Chapter 11 Pediatric Neuroimaging
Ultrasonography is used extensively for prenatal evaluation and in the neonatal period for evaluation of suspected intraventricular hemorrhage, hydrocephalus, and other developmental anomalies, with CT or MRI used to clarify uncertain findings [van Wezel-Meijler et al., 2010; Soetaert et al., 2009].
During late infancy, childhood, and adolescence, when ultrasound is no longer practical, CT is used in the emergency setting for the evaluation of trauma, stroke, and raised intracranial pressure and for examination after placement of ventricular shunts [Ketonen and Valanne, 2008; Abdelhalim and Alberico, 2009]. In most centers, CT is used as the initial examination for evaluation of closed-head or spine injury because of its superior ability to demonstrate acute hemorrhage or osseous injury. CT is also more commonly used than MRI as the initial method for evaluating children presenting with seizures in the emergency department.
An overview of the strength and weaknesses of pediatric ultrasound, CT, and MRI is provided here. A discussion of the neuroimaging changes associated with normal brain development is beyond the scope of this chapter, but several excellent monographs are available [Barkovich, 2005; Tortori-Donati and Rossi, 2005; Provenzale, 2009].
Cranial Ultrasound
Problematic areas in early diagnosis include the cause of ventriculomegaly, the seriousness of isolated ventriculomegaly, the implications of variations of morphology of Dandy–Walker variants, and the nature of underlying cortex in cases of cerebral dysgenesis [Levine et al., 1997].
Real-time sonography can display echogenic (bright) structures, such as choroid plexus, hemorrhage (Figures 11-1 to 11-5), some tumors, and focal areas of cerebritis; it can also show sonolucent structures, such as cerebrospinal fluid in the ventricles (Figure 11-6), subarachnoid spaces, cysts (Figure 11-7 and Figure 11-8), and cystic lesions (Figure 11-9). Normal brain gives rise to fairly uniform echogenicity.
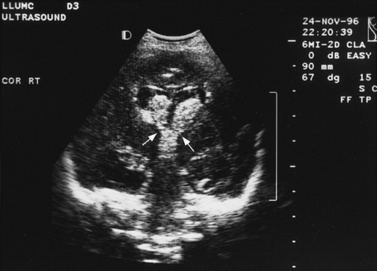
Fig. 11-2 Ultrasound scan of the head of a 1-day-old infant born at 31 weeks’ estimated gestational age.
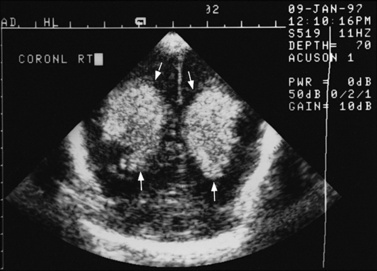
Fig. 11-3 Ultrasound scan of the head of a 3-day-old newborn at 25 weeks’ estimated gestational age.
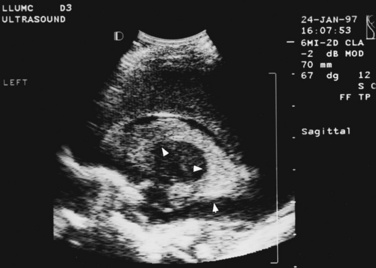
Fig. 11-4 Ultrasound scan of the head of an 8-day-old newborn of 26 weeks’ estimated gestational age.
Detection of fetal CNS anomalies has significantly increased in the last 10–15 years because of the availability of high-resolution ultrasound equipment and the improvement in scanning techniques [Iruretagoyena et al., 2010; Nyberg et al., 2006; Angtuaco et al., 1994]. Transabdominal and endovaginal ultrasound can document early first trimester development and compare it with known embryologic landmarks. The wide array of CNS pathology detected by prenatal sonography includes fetal seizures via abnormal movements [Abrams and Balducci, 1996], holoprosencephaly [Stagiannis et al., 1995], encephalocele [Budorick et al., 1995], schizencephaly, lissencephaly, hydrocephaly (see Figure 11-6) [Holzgreve et al., 1993; Roume et al., 1990], Dandy–Walker malformation (see Figure 11-9) [Cowles et al., 1993], spina bifida [Thiagarajah et al., 1990], and congenital astrocytoma [Heckel et al., 1995].
Postnatally, sonography is helpful in the evaluation of hypoxic-ischemic encephalopathy [van Wezel-Meijler et al., 2010; Kirimi et al., 2002; Anand et al., 1994], including complications of hemorrhage into the germinal matrix, ventricles, or surrounding parenchyma [Sehgal et al., 2009; Müller et al., 2007]. For all infants younger than 30 weeks’ gestation, routine cranial ultrasonography screening should be performed once between 7 and 14 days of age, and should be repeated optimally between 36 and 40 weeks’ postmenstrual age [Ment et al., 2002]. This strategy detects lesions such as intraventricular hemorrhage, which influences clinical care, and those such as periventricular leukomalacia and ventriculomegaly, which provide information about long-term neurodevelopmental outcome [Ment et al., 2002]. Ultrasound can also screen for intrauterine infections and to determine the cause of an enlarging or enlarged head [Yikilmaz and Taylor, 2008; Smith et al., 1998]. Ischemic white matter injury, periventricular leukomalacia (see Figure 11-7 and Figure 11-8), and cystic encephalomalacias can be assessed by ultrasound [Rodriguez et al., 1990], but this approach may not reveal the full extent of injury [Huppi, 2004; Inder et al., 2003; Miller et al., 2003a]. Progression or resolution of intracranial hemorrhage and some of its sequelae, such as hydrocephalus, can be monitored using sonography (see Figure 11-5). Lesions containing cerebrospinal fluid or cerebrospinal fluid-like contents are well delineated because of the echo lucency of water. Examples include ventriculomegaly (see Figure 11-6), porencephaly, Dandy–Walker syndrome (see Figure 11-9), arachnoid cyst, and encephalocele. Vascular malformations, especially aneurysms of the vein of Galen, can be well demonstrated with color Doppler ultrasound. Subtle differences between certain types of lesions, such as differentiation of hydranencephaly from severe hydrocephalus, may not be detected by ultrasound. Major migrational anomalies, such as agyria-pachygyria and lissencephaly, may be delineated by ultrasound; however, smaller heterotopias or other subcortical dysplasias may be overlooked. Ultrasound is helpful in the evaluation of a newborn suspected of having tuberous sclerosis, as it can detect the intracranial hamartomas or subependymal nodules seen in this condition [Tatsuno et al., 1993].
Sonography can be used to screen for myelodysplasia in early infancy, to demonstrate the level of the conus medullaris, and to evaluate newborns suspected of having closed spinal dysraphism (see Chapter 19 for ultrasound examples) [Toma and Rossi, 2001]. It can also reveal the presence or absence of cord pulsations, a sign of tethering, when viewed through the cartilaginous spinal laminae and the infraspinous gaps, which act as sonographic windows. Other dysraphic lesions, such as meningocele, lipoma, hydromyelia, Arnold–Chiari II malformation, diastematomyelia, and lipomyelomeningocele, also can be delineated. Occasionally, sonography is necessary postoperatively to determine the absence of cord pulsations, a finding that suggests cord retethering.
Doppler sonography is used intraoperatively for monitoring resection of arteriovenous malformations during surgery and at the bedside or in the clinic to evaluate arterial patency, direction, and volume of flow and to calculate a resistive index [Lindegaard et al., 1993; Manchola et al., 1993]. Doppler sonography can also be used to evaluate the intracranial circulation during or after extracorporeal membrane oxygenation to detect ischemic or hemorrhagic injury. In patients with subarachnoid hemorrhage, transcranial Doppler ultrasound can be used to detect vasospasm before the patient suffers ischemic neurologic deficit or infarct [Topceuoglu et al., 2002]. It is a standard technique to determine the absence of cerebral blood flow in patients for whom the diagnosis of brain death is suspected.
Computed Tomography
CT has been available since the 1970s for clinical use in children. Ionizing radiation due to x-ray CT is effectively restricted to the immediate body part of interest by the tight collimation used to create the thin fan beam used for scanning. Considerable technical developments have taken place with CT in the past three decades. Several generations of scanners are available, including the helical CT introduced in the early 1990s and the multidetector or multislice scanner. This availability has resulted in an increased number of computed tomographic scans of children, increasing the potential for inappropriate use and excessive radiation dosing [Frush et al., 2003]. Some data indicate that low-dose radiation, similar to the amount received with CT, may be associated with a significant risk of cancer, especially in young children. Children have a longer lifetime in which to manifest radiation-related cancer, and the cancer risk from CT is cumulative over a lifetime. Each computed tomographic examination contributes to the lifetime exposure. It is necessary to limit radiation from CT in children and follow the ALARA (“as low as reasonably achievable”) principle. Several strategies can be followed toward this goal, including performing only necessary examinations, considering alternative imaging modalities such as ultrasonography or MRI when possible, limiting the region of coverage, and adjusting individual imaging parameters, depending on indication, region imaged, and size of the child [Frush et al., 2003; Singh et al., 2009; Strauss et al., 2010].
Abnormalities can be characterized with CT as having low density, isodensity, or high density in relation to the brain. Lesions that appear lower in density include edema, necrosis, infarction (Figure 11-10), neoplasms, leukodystrophies, inflammation, and cysts. Loss of gray–white matter differentiation may be seen with diffuse brain edema after hypoxic-ischemic injury or a demyelinating process. Fat-containing lesions usually appear less dense than water or of mixed density, as in patients with a teratodermoid type of tumor. Air appears as the lowest density, and can be seen with pneumocephalus after trauma or surgery. Lesions isodense to normal tissues are difficult to recognize unless there are changes that demonstrate displacement or replacement of normal anatomic structures or intravenous contrast material that helps to separate the lesion from normal structures. High-density lesions identified on CT usually indicate hemorrhage or the presence of calcium (Figure 11-11). Pathologic intracranial calcifications can be seen with congenital infections, neurocysticercosis, intracranial tumors, tuberous sclerosis, Sturge–Weber syndrome, neurofibromatosis, Cockayne’s syndrome, hypoparathyroidism, arteriovenous malformations, vein of Galen malformations, encephalomalacia, cerebral infarction, or the sequelae of perinatal asphyxia [Erdem et al., 1994]. Hypercellular neoplasms with high nuclear to cytoplasmic ratios (e.g., medulloblastomas, other primitive neuroectodermal tumors, germinomas, lymphomas) may also appear as high-density lesions on CT examination. In young children, CT usually requires sedation and occasionally enhancement using intravenous contrast material. CT myelography and cisternography can be accomplished with intrathecal contrast material introduced into the subarachnoid space. Computed tomographic imaging can be used for precise localization during needle-biopsy procedures or volumetric resection of pediatric brain tumors [Kelly, 1991]. In the evaluation of neonatal disorders, a non-contrast-enhanced computed tomographic examination after ultrasound is helpful in the evaluation of acute hemorrhage (Figure 11-12 and Figure 11-13), developmental anomalies (Figure 11-14 and Figure 11-15), acute trauma (Figure 11-16 and Figure 11-17), suspected infarction (Figure 11-10A), and hydrocephalus, or to demonstrate atrophy and mineralization.
In childhood neurodegenerative diseases, CT may reveal decreased attenuation in the basal ganglia and cerebral white matter or focal or generalized cerebral atrophy [Mirowitz et al., 1991]. Non-contrast-enhanced CT can be helpful in evaluating neurocutaneous syndromes to detect calcifications as in tuberous sclerosis (see Figure 11-15) and for the evaluation of the patient with acute onset of seizures. After closed-head injury, CT without contrast material is usually optimal for evaluation of the skull, intracranial hemorrhage, orbits, sinuses, facial or temporal bones, and osseous spine for fractures or other types of injuries. Evaluation of these regions is of particular importance in the assessment of young children who are victims of nonaccidental trauma. Non-contrast-enhanced CT of the head can rapidly evaluate possible skull fracture and intracranial hemorrhage [Dashti et al., 1999]. Imaging patterns of intracranial hemorrhage in children younger than 3 years have been reported to predict whether the injury was intentional [Wells et al., 2002]. These patterns include subdural hemorrhage located over the cerebral convexities, hemorrhage within the interhemispheric subdural space, hygroma (i.e., nonhemic subdural fluid) with intracranial hemorrhage, and absence of a skull fracture with intracranial hemorrhage [Wells et al., 2002]. If there is a high-risk mechanism of injury, a normal neurologic examination and maintenance of consciousness do not preclude significant intracranial injury in a pediatric trauma patient. A liberal policy of computed tomographic scanning is warranted for such patients [Simon et al., 2001].
Contrast-enhanced CT is helpful in the evaluation of suspected or known vascular malformation, neoplasms (see Figure 11-11B), abscesses, and empyemas. In infants and children with chronic subdural hematomas, contrast enhancement may reveal an enhancing membrane. Contrast-enhanced CT also can be used to differentiate infarction from neoplasm or abscess. Follow-up computed tomographic studies may help distinguish developing infarction from neoplasm. Contrast-only CT may be obtained for serial evaluations of patients with neoplasms, vascular malformations, or suppurative collections. In patients with suspected metastases or seeding of neoplasms, contrast-only CT scan may be sufficient. However, in all the clinical situations previously described, MRI is considered more definitive.
Magnetic Resonance Imaging
On T2-weighted (T2W) images, tissues or structures with long T2 relaxation times, such as cerebrospinal fluid, edema, many tumors, extracellular methemoglobin, infarcts, and multiple sclerosis plaques, are bright, whereas tissues or substances such as muscle, cortical bone, deoxyhemoglobin, and hemosiderin are dark as a result of short T2 relaxation times (see Figure 11-20, Figure 11-23, and Figure 11-25 below). Inversion recovery sequences are typically used to nullify or eliminate signal from a particular tissue. For example, fluid-attenuated inversion recovery (FLAIR) sequences nullify signal from fluids, causing CSF to show up dark rather than bright on T2W images. This sequence is used to increase conspicuity of lesions that are bright on T2 (multiple sclerosis plaques, infarcts) and located near the ventricles, and is particularly useful for identifying subacute subdural hemorrhage. Another sequence called short tau inversion recovery (STIR) nullifies signal from fat and can be used to identify lesions in fatty areas such as the orbits or neck. GRE sequences are routinely used to provide T2*W contrast in images to identify hemorrhage. Since the increased use of higher field strength clinical imaging magnets at 3T, GRE is being used routinely to provide T1W contrast since GRE sequences have less energy deposition than SE sequences and can be performed faster to provide imaging during breath hold. Routinely, T1- and T2-weighted images in various planes are obtained and followed by T1W images with contrast, if needed. The most commonly used contrast agent for MRI contains gadolinium, which shortens the T1 relaxation time of tissues that take up gadolinium contrast agent and thus produce a bright or hyperintense signal on T1W images.
MRI should be used as the primary imaging modality for screening patients in whom neurologic disease is suspected because it is generally the most sensitive and specific of the imaging techniques. MRI is the only modality that provides accurate assessment of brain myelination [Harbord et al., 1990]. Contrast-enhanced MRI is also more sensitive than contrast-enhanced CT for detection of encephalitis [Koelfen et al., 1996], brain abscess (Figure 11-18), ventriculitis, or small foci of leptomeningeal involvement. MRI is often better than CT in demonstrating traumatic injuries, particularly diffuse axonal shearing injury [Mittle et al., 1994], post-traumatic olfactory injury [Yousem et al., 1996], brainstem contusion, subacute hemorrhage, encephalomalacia, and gliosis. Certain pathologies – including migrational anomalies such as gray-matter heterotopias, closed-lip schizencephaly, lissencephaly, pachygyria (Figure 11-19), and hemimegalencephaly, and neurocutaneous disorders such as neurofibromatosis [Es et al., 1996] – that are not seen well on CT are better demonstrated with MRI. In children with severe head injury who are comatose, the presence of traumatic brainstem lesions seen on MRI has a high correlation with outcome. CT fails to demonstrate these lesions [Woischneck et al., 2003].
MRI is useful in the evaluation of patients with movement disorders, such as Wilson’s disease [King et al., 1996; Magalhaes et al., 1994], because it demonstrates abnormal T2 signal in the basal ganglia, especially the outer rim of the putamen and in the substantia nigra; in juvenile Huntington’s disease, it reveals atrophy of the caudate nuclei and increased T2 signal in atrophic caudate nuclei [Ho et al., 1995]. In pantothenate kinase deficiency (formerly designated as Hallervorden–Spatz syndrome), MRI demonstrates areas of symmetric low-signal intensity in T2-weighted images with high signal intensity in the anteromedial aspect of the globus pallidus, the so-called eye of the tiger sign [Ou et al., 1994; Savoiardo et al., 1993]. MRI shows multifocal cortical infarctions and the presence of lactate peaks in proton MRS in mitochondrial disorders, such as MELAS syndrome (i.e., mitochondrial encephalopathy with lactic acidosis and strokelike episodes) [Castillo et al., 1995; Barkovich et al., 1993; Kim et al., 1996; Wycliffe and Skeen, 1997]. In other mitochondrial disorders, such as MERRF syndrome (i.e., myoclonus, epilepsy, and red ragged fibers), Kearns–Sayre syndrome, Leigh’s syndrome, Alpers’ disease, and Menkes’ disease, symmetric white matter T2 hyperintensities with involvement of deep cerebral nuclei are observed on MRI. Patients with Menkes’ disease demonstrate rapidly progressive atrophy accompanied by large subdural hematomas [Barkovich et al., 1993].
For focal (Figure 11-20) and diffuse white matter disease, T2-weighted MRI is more sensitive than CT in demonstrating lesions. MRI should preferentially be used to evaluate children with acute disseminated encephalomyelitis (Figure 11-21) [Lee et al., 1996], human immunodeficiency virus (HIV) encephalitis, sickle cell disease, vasculitis such as systemic lupus erythematosus, Lyme disease [Oksi et al., 1996], progressive multifocal leukoencephalopathy, and multiple sclerosis [Guilhoto et al., 1995]. Recognizable patterns of white matter involvement can be seen with MRI in certain inherited leukodystrophies. For example, early, diffuse involvement of the peripheral subcortical white matter is seen in Pelizaeus–Merzbacher disease [Andre et al., 1990], Canavan’s disease, and Alexander’s disease. In Canavan’s disease, patients have macrocephaly, and on proton MRS, a larger than normal N-acetyl-aspartate peak is produced. In Alexander’s disease, there is predilection for T2 lengthening in frontal white matter and enhancement after contrast administration. A predilection for occipital white matter involvement is seen with adrenoleukodystrophy. Follow-up MRI examinations have been used for patients with adrenoleukodystrophy to monitor progression of disease symptoms [Loes et al., 1994]. In globoid cell leukodystrophy (i.e., Krabbe’s disease), T2 hyperintensity can be seen in the cerebellum and deep cerebral white matter [Sasaki et al., 1991], whereas the thalami and basal ganglia may be hypointense [Osborn and Tong, 1996].
MRI can demonstrate mesial temporal sclerosis (Figure 11-22) in patients with epilepsy [Kuzniecky et al., 1996], and can reveal small tumors in the aqueduct, sella turcica, or brainstem (Figure 11-23) even when the CT is normal. Children with mesial temporal sclerosis may have dual pathology with mild to moderate cortical dysplasia and with hippocampal sclerosis that can be visualized with MRI [Mohamed et al., 2001]. Continuing technical advances are reported using higher field strengths (3T) and/or multiple channel coils that provide higher signal to noise to obtain high-resolution images of the brain capable of detecting lesions not previously identified with MRI, including hippocampal dysplasia, hippocampal atrophy, and dual pathology with cortical dysplasia. These high-resolution MRI scans are improving the diagnosis of patients who are candidates for surgical treatment for refractory epilepsy [Goyal et al., 2004]. MRI is also the preferred procedure when children present with symptoms and signs that suggest a CNS tumor (Figure 11-24; see also Figure 11-11 and Figure 11-23). In the evaluation of pediatric head and neck lesions, MRI provides valuable additional information concerning lesions that are difficult to resolve with CT, and it is essential for assessment of possible intracranial extension of disease [Jones and Koch, 1999].
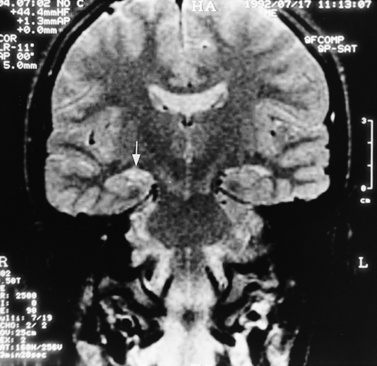
Fig. 11-22 T2-weighted, coronal image through the hippocampus in a child with temporal lobe seizures.
For vascular and hemorrhagic lesions, MRI is more specific than CT or ultrasound. MRA has added a new dimension to the evaluation of pediatric cerebrovascular disease. This noninvasive imaging technique can be used to evaluate vascular malformations, vaso-occlusive disease (see Figure 11-13C and Figure 11-20B), and vascular neoplasms. MRA may demonstrate occlusions or stenoses of the supraclinoid internal carotid arteries, the proximal anterior and middle cerebral arteries, collateral vessels, and parenchymal lesions in moyamoya disease [Yamada et al., 1995], as well as proximal large-vessel stenotic lesions in children with sickle cell disease [Wiznitzer et al., 1990].
MRI may detect silent infarctions in children with Kawasaki’s disease [Fujiwara et al., 1992] or sickle cell disease. In cases of childhood migraine and in sickle cell disease (see Figure 11-20), MRI may demonstrate small white matter and basal ganglia infarctions not seen on CT. MRI is more specific than CT in the identification and staging of hemorrhage and clot formation as a function of the evolution of hemoglobin breakdown products (Figure 11-25) and can differentiate arterial from venous occlusive disease. Sinovenous thrombosis in children is a serious condition that can result in neurologic impairment or death in approximately half the patients [deVeber and Andrew, 2001]. The presence of a venous infarct or dural sinus thrombosis seen with MR venography predicts a poor outcome. In evaluating intracranial hemorrhage caused by angiographically occult lesions such as cavernous angiomas (see Figure 11-25), MRI is the procedure of choice. MRI can help to differentiate between various infantile extra-axial fluid collections and may distinguish effusions and hygromas from subdural hematomas, as in child abuse. Special MRI sequences (FLAIR) can be used to differentiate cerebrospinal fluid-containing lesions (e.g., arachnoid cysts) from other lesions (e.g., epidermoid tumors) that are often difficult to visualize on CT. Sagittal MRI images have better resolution than ultrasound and also cannot be obtained using CT. MRI is excellent in delineating midline abnormalities including lesions in the sella, aqueduct, foramen magnum, and pineal region (Figure 11-26). Absence of T1 hyperintensity in the posterior pituitary on a sagittal MR image is diagnostic of central diabetes insipidus in children [Maghnie et al., 2000]. For evaluation of hypopituitarism in children, MRI of the head is critical [Geffner, 2002]. Hydrocephalus in children, unexplained by ultrasound and CT, may be evaluated satisfactorily with an MRI examination (Figure 11-27). Likewise, in patients with CNS tumors and cerebrospinal fluid seeding, MRI with gadolinium enhancement is more sensitive than a CT examination. MRI is also being used for planning surgery, radiotherapy, and chemotherapy, as well as for treatment follow-up. Contrast enhancement adds to the usefulness of MRI in these settings.
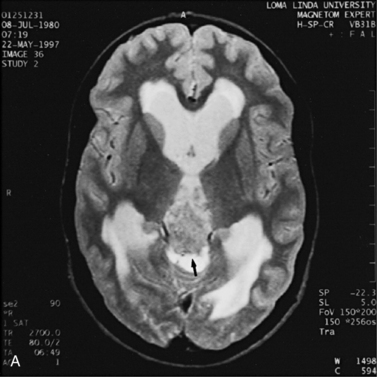
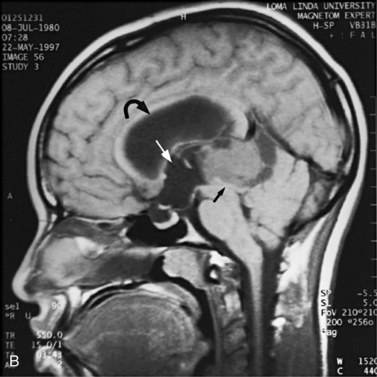
Fig. 11-26 A 16-year-old female with 2 months of blurred vision, who was found to have bilateral papilledema.
Magnetic Resonance Spectroscopy
MRS is a clinically useful, noninvasive tool for identifying the biochemical state of the CNS [Novotny et al., 1998; Panigrahy et al., 2010]. It can provide information about certain metabolites in normal and pathologic conditions [Ross and Michaelis, 1994]. Certain atomic nuclei such as 1H (protons) are magnetic, and when exposed to a strong magnetic field, they align in a particular orientation until equilibrium is reached [Prichard, 1993]. If the nuclei are then excited by a radiofrequency pulse at their resonant frequency, they produce a detectable signal during relaxation back to equilibrium. During relaxation, because of their local chemical environment, each proton produces a signal at a slightly different frequency, called a chemical shift. After a Fourier transform analysis, the plots of the resulting nuclear magnetic resonance spectra appear as peaks of signal intensity versus signal frequency or chemical shift. Peaks at a particular chemical shift represent signal from protons of a particular metabolite or biochemical compound while the area underneath the peak represents the relative concentration of the metabolite.
MRI uses the strong signals from 1H nuclei of water and their spatial location to reconstruct anatomic images. In contrast, proton MRS is interested in the much weaker signals from nonwater protons to obtain quantitative information about biologic molecules within tissue. Special techniques, such as chemical shift selective saturation (CHESS), are used to suppress the overwhelming signal produced because of the large concentration of water. Small concentrations of brain metabolites can be detected using acquisition sequences, such as stimulated-echo acquisition mode and point-resolved spectroscopy. The principles underlying this spectral editing are quite complex and are discussed in several reviews [Bolinger and Insko, 1996; Novotny et al., 1998; Ross and Michaelis, 1994].
Initial studies in children used phosphorus (31P) MRS to investigate spectral changes seen after neonatal asphyxia [Martin et al., 1996]. Reduced phosphocreatine to inorganic phosphate (PCr/Pi) ratios suggested that impairment of energy metabolism occurred late rather than early after asphyxia, and this ratio correlated with poor neurologic outcome, as did the presence of lactate in other studies [Groenendaal et al., 1994; Martin et al., 1996].
Spectral Metabolites Using Proton Magnetic Resonance Spectroscopy
Proton MRS (1H-MRS) is being used to investigate a wide range of neurologic disorders. Unlike 31P MRS, 1H-MRS can be obtained using the same coils as imaging. Metabolites measured with 1H-MRS include N-acetyl-aspartate (NAA), a neuronal marker; creatine (Cre) composed of phosphocreatine and creatine, which are bioenergetic metabolites; choline-containing compounds (Cho), including free choline and phosphoryl and glycerophosphoryl choline that are released during membrane disruption; lactate (Lac), which accumulates in response to tissue damage or anaerobic glycolysis; and other metabolites only seen when acquired with short echo time sequences, such as the neurotransmitters glutamate and immediately formed glutamine (Glx) and myoinositol (mI or Ins), an osmolyte and astrocyte marker [Prichard, 1991; Sappey et al., 1992; Tunggal et al., 1990]. During normal maturation of the brain, NAA, Cre, and Glx levels increase, and Cho and Ins levels decrease [Kreis et al., 1993; Ross et al., 1992] (Figure 11-28). The newborn brain has high water content, and the high Cho and Ins levels decline as myelination progresses [Kreis et al., 1993]. It is not uncommon to detect small lactate peaks in spectra from normal newborn brain, but if lactate levels are high or appear after the neonatal period, it may indicate possible hypoxic-ischemic injury [Holshouser et al., 1997], a metabolic disorder, or infection.
Diseases Studied with Proton Magnetic Resonance Spectroscopy
Characteristic proton spectra in adults have been described in stroke, hepatic encephalopathy, dementia, diabetic ketoacidosis, tumor, multiple sclerosis, acquired immunodeficiency syndrome-related leukodystrophies, and trauma [Ross and Michaelis, 1994]. In children, abnormalities on 1H-MRS have been observed in a variety of metabolic [Chabrol et al., 1995; Kruse et al., 1994] and mitochondrial encephalopathies [Abe et al., 2004], brain tumors [Arle et al., 1997; Byrd et al., 1996; Sutton et al., 1992; Taylor et al., 1998; Warren et al., 2000], and certain neurodegenerative conditions [van der Knapp et al., 1992; Wang et al., 1996]. Since metabolite levels change with brain maturation, metabolite levels and ratios must be compared to normal age-matched control values acquired with the same sequence and parameters at the same field strength for proper interpretation of the results.
Proton MRS has been used after acute traumatic and nontraumatic injuries. Adult patients with severe closed-head injuries or stroke with long-term neurologic sequelae demonstrate elevated Lac peaks, reduced NAA peaks, and reduced NAA/Cre ratios [Choe et al., 1995; Matthews et al., 1995]. After closed-head injury in children, Lac has been reported in regions of contusion and infarction but not in areas of diffuse axonal injury [Sutton et al., 1995]; Lac elevation is more common in children with nonaccidental trauma [Holshouser et al., 1997; Aaen et al., 2010]. Other researchers have found that reduced occipital NAA/Cre ratios or the presence of cerebral Lac correlated with poor outcomes [Ashwal et al., 2000; Shu et al., 1997]. Increased NAA/Cho and reduced Cho/Cre ratios correlate with impaired neuropsychologic functioning after traumatic brain injury [Brenner et al., 2003; Babikian et al., 2006; Hunter et al., 2005]. Spectral sampling in areas of brain that did not appear to be visibly injured has shown altered metabolite ratios that suggested definite injury. Later studies have examined the role of mI, a marker for post-traumatic astrogliosis or disturbed osmotic regulation, using quantitative short echo time MRS, and have found that occipital gray matter mI levels are increased after traumatic brain injury and correlate with poor outcomes [Ashwal et al., 2004b]. The Glx and Cho levels are elevated for all traumatic brain injury patients compared with control subjects, whereas NAA was not [Ashwal et al., 2004a]. The time interval before MRS acquisition after injury and location of sample within the brain might affect findings. Overall, these studies suggest the strong potential for using MRS to provide accurate estimates of long-term neurologic and neuropsychologic function after traumatic brain injury in infants and children. More recently, a longitudinal study using more advanced MR imaging techniques such as susceptibility weighted imaging (SWI) and diffusion tensor imaging (DTI) (discussed in later sections) along with MRS has shown these techniques to be capable of detecting post-traumatic hemorrhagic lesions and the corresponding abnormalities on DTI, as well as metabolite abnormalities on MR spectroscopic imaging (MRSI) (Figure 11-29).
MRS with diffusion-weighted imaging (DWI) has been used to evaluate children with stroke and hypoxic-ischemic injury. Confirmation of the death of tissue is provided on proton MRS by a rise in Lac from anaerobic glycolysis, and a loss of NAA from neuronal death [Zimmerman, 2000]. The combination of MRI and MRS can provide prognostic information in children after near-drowning [Dubowitz et al., 1998]. MRI and MRS have been found particularly useful in the evaluation of neonates with perinatal encephalopathy. Reduced NAA-derived metabolite ratios, elevated Cho or Ins, as well as abnormalities on DWI, have been shown to reflect the severity of injury and to correlate with long-term developmental and neurologic outcomes [Bartha et al., 2004; Cady et al., 1997; Robertson et al., 2001; Zarifi et al., 2002; Miller et al., 2005]. Patients with elevated brain Lac levels and decreased NAA/Cre ratios have been significantly more likely to have a poor outcome compared with age-matched control subjects (Figure 11-30) [Holshouser et al., 1997].
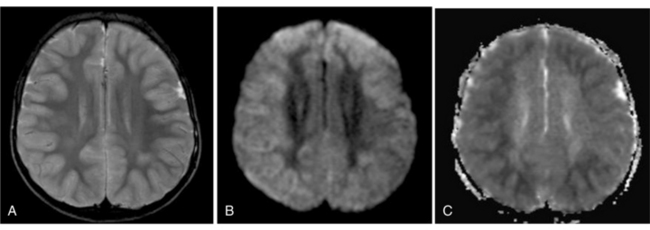
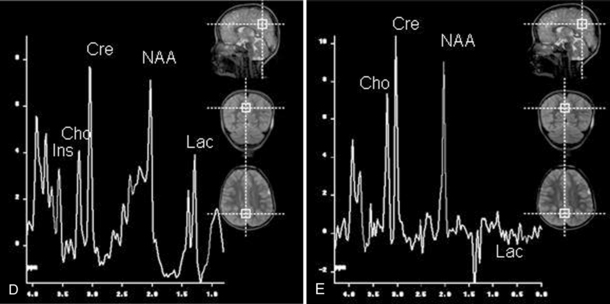
Fig. 11-30 A 30-month-old male after cardiopulmonary arrest following a near-drowning incident.
A, T2-weighted, axial MRI. Diffuse T2 hyperintensities are seen along the cortical margin of both cerebral hemispheres involving the gray matter, which is consistent with diffuse cytotoxic edema from hypoxic-ischemic insult. B, Diffusion-weighted image (b = 1000) shows diffuse abnormal increased signal in the cortical gray matter and subcortical region of both cerebral hemispheres corresponding to the areas of T2 abnormality. C, Apparent diffusion coefficient (ADC) map shows relative low signal in the subcortical region of both cerebral hemispheres extending into the cortical margin. D, Single-voxel proton MR spectrum (TR = 3000 msec, TE = 20 msec, stimulated-echo acquisition mode [STEAM]) from occipital gray matter obtained 4 days after injury reveals a prominent lactate (Lac) peak and a markedly reduced N-acetyl-aspartate/creatine ratio (NAA/Cr = 0.78) compared with age-matched controls (NAA/Cr = 1.5 ± 0.14) (see Figure 11-28A–D). E, Proton MR spectrum (TR = 3000 msec, TE = 144 msec, point-resolved spectroscopy [PRESS]) of the same voxel area obtained immediately after the spectrum depicted in B also reveals a prominent lactate peak and a markedly reduced NAA peak, both of which indicate a poor prognosis. This patient had minimal neurologic response and was withdrawn from life-support 7 days after incident.
Proton MRS combined with MRI is useful in screening patients for metabolic and mitochondrial disorders based on the detection of increased cerebral lactate or the presence of other elevated metabolite peaks [Ashwal et al., 1997; Barkovich et al., 1993; Chabrol et al., 1995; Cross et al., 1993; Matthews et al., 1993]. Abnormalities have been reported in patients with glutaric aciduria type 2 [Shevell et al., 1995]; pyruvate dehydrogenase deficiency [Cross et al., 1994]; Leigh’s syndrome [Krageloh et al., 1993]; X-linked adrenoleukodystrophy [Kruse et al., 1994], MELAS [Abe et al., 2004; Kaufmann et al., 2004], and a variety of other conditions. In phenylketonuria, elevated phenylalanine levels can be seen with MRS of the brain [Osborn and Tong, 1996]. In Canavan’s disease, MRS shows marked elevation of the NAA peaks [Osborn and Tong, 1996]. In addition to an elevated NAA peak, patients with Canavan’s disease have abnormally increased NAA/Cre and NAA/Cho ratios [Barker et al., 1992]. In Leigh’s disease, as in other mitochondrial or metabolic disorders, MRS reveals an abnormally high Lac peak and a decreased NAA peak in the basal ganglia and occipital gray matter [Osborn and Tong, 1996] (Figure 11-31). In some children with metabolic diseases, Lac is present during the acute illness, and with improvement, serial spectra can demonstrate its resolution [Kreis et al., 1995]. Elevated cerebral Lac has also been detected when blood Lac levels have been normal in symptomatic patients [Krageloh et al., 1993]. In children with global developmental delay, proton MRS has also been useful in detecting creatine deficiency associated with disorders of creatine synthesis or transport [Newmeyer et al., 2005].
Proton MRS has also been used in the evaluation of children with epilepsy [Duncan, 1996; Jackson, 1994; Kuzniecky, 2004; Novotny, 1995; Suhy et al., 2002]. Studies using localized proton MRS have indicated decreased NAA, increased Cho, and increased Lac levels in epileptic foci compared with nonictal or contralateral regions [Leite et al., 2010; Aydin-Ozemir et al., 2010; Connelly et al., 1994]. Proton spectroscopic imaging studies have demonstrated similar metabolite changes, but with the added capability of spatially defining the regions with metabolite abnormalities [Hetherington et al., 1995; Hugg et al., 1993]. Over the next decade, it is likely that additional studies will demonstrate a role for proton MRS in the evaluation and selection of patients with intractable seizures for surgery.
The use of proton MRS has been examined in children and adults with CNS tumors [Byrd et al., 1996; Kinoshita et al., 1994; Negendank et al., 1996; Sutton et al., 1992; Tzika et al., 1997; Wang et al., 1995; Warren, 2004]. Malignant pediatric brain tumors are characterized by an increase in Cho due to an increase in active cell proliferation and a decrease in NAA because of replacement of neurons with tumor cells [Sutton et al., 1992; Byrd et al., 1996; Tzika et al., 1997]. In one study, the pretreatment total Cho peak was the most reliable indicator of malignancy [Taylor et al., 1998]. In another multicenter study, Cho signal intensities were highest in astrocytomas and anaplastic astrocytomas, and creatine signal intensities were lowest in glioblastomas [Negendank et al., 1996]. However, tumoral metabolic characteristics exhibited large variations within each subtype of glial tumor, precluding diagnostic accuracy of MRS in differentiating low- and high-grade tumors. In children, it has been suggested that proton MRS may be useful in differentiating various types of cerebellar tumors, such as primitive neuroectodermal tumors (PNET), low-grade astrocytomas, and ependymomas [Wang et al., 1995]. Single-voxel quantitative 1H-MRS performed in patients with untreated pediatric brain tumors showed that the taurine concentration was significantly elevated in PNETs and was useful in the differentiation of PNETs from other tumors [Kovanlikaya et al., 2005]. An example of a short echo time spectrum of a medulloblastoma showing a taurine peak is demonstrated in Figure 11-32. In another report, a computer-based neural network that combined MRI, MRS, and clinical data to predict tumor histology in pediatric patients with posterior fossa tumors was able to identify 95 percent of tumors in 33 children correctly [Arle et al., 1997]. Other spectroscopy features reported include increased Lac and lipids, particularly in high-grade tumors [Byrd et al., 1996].
MRS is useful in differentiating tumor recurrence from radiation necrosis. Patients with postirradiation necrosis are more likely to have reduced NAA, Cre, and Cho peaks with presence of lactate and lipids, compared with patients with tumor recurrence in which Cho is increased [Usenius et al., 1995]. MRS has prognostic significance in predicting outcome in children with relevant primary brain tumors. Patients with a Cho/NAA ratio greater than 4.5 were found to have a higher mortality rate [Warren et al., 2000]. In adults, proton MRS has been used to determine whether metastatic CNS tumors from distal primary sites are present [Sijens et al., 1995]. However, MRS data cannot be used alone because of significant overlap and nonspecificity of the results. It is difficult to distinguish non-neoplastic lesions such as hamartomas, histiocytic lesions, or dysplastic lesions from low-grade neoplasms by MRS [Cecil and Jones, 2001].
Diffusion-Weighted Imaging
Diffusion-weighted imaging (DWI) is a technique that uses MRI to measure the diffusion of water through tissues [Ashwal and Holshouser, 1997; Buxton et al., 1996; Fisher et al., 1992; Warach, 1996]. Random displacements of water molecules (i.e., diffusion) are modified by structural and physiologic factors in a medium. In a medium in which diffusion of water molecules is identical in all directions the process is called isotropic diffusion. When the process depends on direction, it is called anisotropic diffusion [Ito et al., 2002]. In brain white matter, diffusivity of water molecules is not the same in all directions of three-dimensional space and is anisotropic at an imaging voxel scale because of its characteristic microscopic and macroscopic features such as myelin sheath, axon, and fiber tract [Klingberg et al., 1999]. DWI has been used to investigate stroke and hypoxic-ischemic injury in children [Barkovich et al., 2001; Hunter, 2002; Phillips and Zimmerman, 1999], to differentiate solid (e.g., epidermoid tumors) [Chen et al., 2001] from cystic CNS lesions, and to evaluate patients with demyelinating disease. DWI is based on the ability to detect subtle changes in the diffusion of water molecules. DWI measures the apparent diffusion of water (i.e., the self-diffusion of water among other water molecules within tissues) [Buxton et al., 1996; Warach, 1996]. The degree of diffusion depends on many factors, but those demonstrated to be most important include tissue and fluid viscosity, membrane barriers to the free movement of water, chemical interaction of water with macromolecules, and local tissue temperature. MRI can be used to measure apparent diffusion by using an additional pair of strong gradient pulses to the standard pulse sequence, such that the first pulse dephases and the second pulse completely rephases spinning protons. Such data are used to calculate apparent diffusion coefficient (ADC) maps for each brain slice acquired. On an ADC map, the signal intensity corresponds to the ADC value, thus low ADC values (representing restricted diffusion) correspond to a dark signal on an ADC map. The same area of restricted diffusion would show up bright on a corresponding diffusion-weighted image.
Because diffusion is also affected by axonal orientation, DWI has been used to study aspects of myelination [Nomura et al., 1994]. In white matter, diffusion has been found to be anisotropic; there is greater water mobility along myelinated fiber tracts than perpendicular to them. By measuring these differences, DWI allows investigation of myelin orientation and development. This finding may be of particular interest in evaluating children with closed-head injuries. In one report, DWI was found to have increased utility in detection of brain injury in children with nonaccidental trauma [Suh et al., 2001]. In this study, DWI revealed more extensive brain injury than was demonstrated on conventional MRI sequences. DWI may also identify evidence of corpus callosal and subcortical white matter injury, and restricted diffusion in white matter has been associated with poor neurological and neuropsychological outcomes [Galloway et al., 2008; Babikian et al., 2009]. DWI should be obtained in children in whom nonaccidental trauma is suspected, even if acquired several days after the insult [Chan et al., 2003].
During early brain myelination, anisotropy in DWI precedes myelination changes and is helpful in evaluating the normal progression of myelination in infants [Engelbrecht et al., 2002]. DWI obtained during the acute phase of periventricular leukomalacia is more sensitive than conventional MRI and ultrasound in detecting hyperintensities that evolve to damaging lesions at follow-up MRI and ultrasound studies [Bozzao et al., 2003]. In X-linked adrenoleukodystrophy, significant abnormalities with diffusion tensor imaging, an extension of DWI discussed later, that are not seen on conventional MRI are reported and are consistent with demyelination [Schneider et al., 2003]. In a case of vitamin B12-deficiency leukoencephalopathy, reduced anisotropy occurred within white matter lesions with T2 abnormalities and normal-appearing adjacent white matter [Kealey and Provenzale, 2002]. DWI can detect larger areas of involvement than conventional MRI in this demyelinating process. In osmotic myelinolysis, cytotoxic [Chu et al., 2001] and extracellular [Chua et al., 2002] edema is reported with DWI, sometimes before abnormal signals are seen with conventional MRI [Ruzek et al., 2004]. In neonates, DWI is useful in the evaluation of acute ischemic brain injury and seizures. In the acute setting, DWI provides information not available with CT and conventional MRI [Krishnamoorthy et al., 2000]. In children younger than 2 years, DWI is useful for evaluating acute ischemic injuries, metabolic disorders, and leukodystrophies [Gelal et al., 2001]. In patients with sickle cell disease and an acute CNS event, DWI is an essential part of the investigation [Kirkham and DeBaun, 2004]. DWI is helpful for earlier identification of the changes in acute carbon monoxide poisoning [Teksam et al., 2002]. In infants and children with dural sinus thrombosis, DWI shows both increased and decreased ADC mapping of nonhemorrhagic edema, consistent with extracellular and cytotoxic edema. Areas of increased ADC values may return to normal [Keller et al., 1999], whereas areas with normal or decreased ADC values occasionally show imaging sequelae [Ducreux et al., 2001]. Venous infarcts are frequently hemorrhagic. In patients with posterior reversible encephalopathy syndrome, DWI shows reversible vasogenic edema and, in severe cases, its conversion to cytotoxic edema, which is associated with a poorer outcome. Areas of hyperintensities on DWI can progress to infarction [Covarrubias et al., 2002].
Children with new-onset prolonged seizures can develop unilateral hippocampal sclerosis. The presence of diffusion restriction in the affected hippocampal region can herald subsequent development of mesial temporal sclerosis [Farina et al., 2004]. DWI can be helpful in the evaluation of CNS tumors. It may show diffusion restriction in solid portions of primitive neuroectodermal tumors, a finding that is unusual in nonprimitive neuroectodermal tumor lesions [Erdem et al., 2001]. Glioblastomas are relatively uncommon in children, and they can be difficult to differentiate from other brain tumors such as primitive neuroectodermal tumors, ependymomas, or cystic astrocytomas. However, hyperintensity on DWI has been observed in solid portions of glioblastomas, and in combination with spectroscopy, it may be helpful in determining an appropriate site for biopsy and in evaluating tumor recurrence [Chang et al., 2003]. Hyperintensity also can be seen in lymphomas on DWI [Stadnik et al., 2001].
DWI is useful for evaluating children with intracranial infection. Cerebral abscesses, tuberculomas, subdural empyemas, and epidural abscesses demonstrate hyperintensity with DWI [Lim et al., 2003]. Neurocysticercosis cysts and encephalitis appear hyperintense on DWI, whereas toxoplasmosis lesions produce variable signal intensity. DWI hyperintensity is observed in neonates with brain abscesses [Sener, 2004]. DWI may differentiate abscesses from necrotic tumors, although both appear as ring-enhancing lesions on MRI [Leuthardt et al., 2002]. In pyogenic ventriculitis, DWI shows signal intensity in dependent intraventricular fluid [Pezzullo et al., 2003]. DWI may be of some prognostic value in children with herpes simplex encephalitis [Sener, 2001]. These lesions can exhibit low or high signal intensity indicating vasogenic or cytotoxic edema. Patients with cytotoxic edema have fulminating disease and are more likely to have a complicated clinical course and poorer outcome. Patients with vasogenic edema are less seriously ill and more likely to have a good outcome with prompt therapy [Sener, 2001]. In children who undergo middle-ear and mastoid surgery for cholesteatomas, MRI with DWI can detect recurrent or residual cholesteatomas if they are more than 5 mm in diameter [Aikele et al., 2003].
DWI has assumed an important role in the early evaluation of patients with stroke [Fisher et al., 1992; Lutsep et al., 1997; Warach et al., 1995]. DWI reveals hyperintensity in an acute infarct soon after the onset of ischemia. Significant DWI lesions have been associated with little clinical recovery, with follow-up MRI scans demonstrating fixed lesions. In almost all previous reports of adults with stroke, regions with early DWI hyperintensity eventually demonstrated infarction. DWI also has been evaluated in pediatric stroke patients [Connelly et al., 1997]. In some patients, regions of DWI hyperintensity did not uniformly progress to infarction as determined by serial MRI studies, and this suggests that, in children, DWI can demonstrate tissue that is compromised but not to the point of irreversibility. Stroke in children is an unexpected event, and the causes are more diverse than in older populations. Imaging plays an important role in the evaluation and management of children with stroke (see Chapter 85).
The combination of MRI, DWI, diffusion tensor imaging, perfusion-weighted imaging, MRA, and MR venography, as clinically indicated, offers the best sensitivity for the detection of the lesion, determination of its extent, and evaluation of the degree of underlying vascular compromise [Uggetti, 2003]. In neonates, DWI is helpful for the early diagnosis of stroke, and findings may be more pronounced than those seen on conventional MRI. It is not unusual in the evaluation of neonatal strokes to have positive findings on DWI and MRI but negative findings on MRA on MR venography. Venous infarcts are common in newborns. They are usually adjacent to the dural sinuses and show hemorrhagic components (Figure 11-33). Stroke in the newborn can also be hemorrhagic (Figure 11-34 and Figure 11-35). Evaluating DWI as soon as possible after the onset of ischemic or traumatic symptoms holds promise for early detection of potentially reversible ischemic injury. Future outcome studies will determine whether resolution of DWI lesions corresponds with clinical recovery or persistence of deficits.
Diffusion Tensor Imaging
With diffusion tensor imaging (DTI) it is possible to have in vivo localization of neuronal fiber tracts [Basser et al., 2000; Yamada et al., 2009]. This was not possible previously by any other in vivo neuroimaging technique.
FA is calculated from the Eigen values (γ1, γ2, γ3) of the diffusion tensor.
Reconstructed from the tensors of the white matter tracts, three-dimensional maps of the macroscopic fiber orientations in the brain can be obtained. Various algorithms are used to depict the longest axis of the tensor (v1) into white matter trajectories. One of them is fiber assignment by continuous tracking (FACT). Neural connections are mapped by designating at least two arbitrary regions of interest (ROI) in three-dimensional space. Tracking starts at a pixel or ROI. The fiber assignment tracks the ellipsoids as long as the adjacent vectors are strongly aligned. Tracking is terminated when a pixel with threshold FA is reached or a predetermined trajectory curvature between two contiguous vectors is reached. In summary, DTI provides information on diffusion anisotrophy, which can be expressed with three-dimensional white matter tractography [Kunimatsu et al., 2003].
DTI is increasingly used to evaluate normal brain development and changes associated with a wide variety of neonatal and pediatric neurological disorders, as well as the brain’s response to injury [Feldman et al., 2010; Hüppi and Dubois, 2006; Miller et al., 2003b]. DTI has been highly instrumental in advancing knowledge regarding early structural cerebral organization and maturation. DTI has been used in preterm and term infants to evaluate changes in cortical connectivity after white matter injury, particularly in preterm infants [Ment et al., 2009]. Tractography is also of particular value in examining aberrant connectivity in structural brain malformations [Mukherjee and McKinstry, 2006].
DTI has been found to be valuable in surgical planning for resection of brain tumors or temporal lobectomy for intractable epilepsy. Eloquent white matter tracts, such as the pyramidal tracts and optic radiations [Yamamoto et al., 2005; Taoka et al., 2008], can be assessed in relation to the tumor. DTI for assessment of the optic radiations appears to underestimate their anterior extent. This may prove to be a limitation of its use [Yamamoto et al., 2005]. When temporal lobectomy is contemplated for epilepsy surgery, DTI may be used to predict visual field deficits following temporal lobe resection [Taoka et al., 2008]. DTI has been found to be valuable in imaging of stroke to assess the relation between eloquent fiber tracts and infarcts, and in the prognosis of gross motor functions following an infarct [Kunimatsu et al., 2003; Yamada et al., 2003]. It also has been found to be useful in the evaluation of adult degenerative diseases, such as Alzheimer’s disease and other forms of dementia [Taoka et al., 2006; Matsuo et al., 2008]. DTI shows decreased diffusion anisotropy and increased diffusivity of white matter in patients with Alzheimer’s disease. Such changes in diffusion anisotropy and diffusivity reflect decreased fiber attenuation, which can involve disruption and loss of axonal membranes or myelin. This decreased fiber attenuation may be secondary to the loss of neurons in the cortex. Other forms of neurodegenerative diseases where DTI has been found to be useful include multiple sclerosis [Roosendaal et al., 2009], amyotrophic lateral sclerosis [Senda et al., 2009], spinocerebellar atrophy [Mandelli et al., 2007], and Parkinson’s disease [Lee et al., 2010]. In multiple sclerosis patients, low FA, increased radial diffusivity, and a less pronounced increase in axial diffusivity have been found in the fornices, coronal radiata, inferior longitudinal fasciculus, optic radiations, and parts of the corpus callosum [Roosendaal et al., 2009]. Widespread white matter decreased FA values have been found in DTI of amyotrophic lateral sclerosis patients in the corpus callosum, internal capsule, frontal, parietal, and temporal white matter, and posterior cingulum [Senda et al., 2009]. In spinocerebellar atrophy, DTI has shown reduced FA in the middle cerebellar peduncles, cerebellar hemispheric white matter, transverse pontine fibers, and corticospinal tracts at the level of the pons and middle cerebellar peduncles [Mandelli et al., 2007]. Significantly low FA values have been reported with Parkinson’s disease dementia in frontal, temporal, and parietal white matter [Lee et al., 2010]. It has been useful in evaluation of spinal cord lesions [Vargas et al., 2008]. In fourteen cases of spinal cord lesions of traumatic, neoplastic, and inflammatory causes, DTI showed clear displacement and deformation of the white matter tracts at the level of the pathological lesions in the spinal cord [Vargas et al., 2008]. In pediatric cases of ischemic brain insults, DTI has been found valuable in prediction of outcome in neonates and infants with periventricular leukomalacia [Murakami et al., 2008] or developmental anomalies [Sundaram et al., 2008; Herweh et al., 2009]. DTI performed in 10 patients with periventricular leukomalacia showed abnormal FA values in the motor tract [Murakami et al, 2008]. DTI has also been useful in the evaluation of trauma and diffuse axonal injury [Sugiyama et al., 2009; Jang et al., 2009; Chu et al., 2010]. In one study, all 14 patients with diffuse axonal injury showed multiple foci of corticospinal tract injury, with low FA values below 2 standard deviations from normal at the point of corticospinal tract injury. The mean number of corticospinal tract injuries was 3.6 (range: 2–7). The corticospinal tracts were involved at the following locations: the pons (61 percent), the cerebral peduncle (39 percent), the corona radiata (21 percent), the medulla (14 percent), and the posterior limb (11 percent). The pons was the most prevalent area of corticospinal tract injury in patients with diffuse axonal injury at the subcortical level. Another example from the same severely injured trauma patient shown in Figure 11-29 shows another hemorrhagic lesion on SWI in the right basal ganglia with corresponding diffusion abnormalities on DWI, DTI abnormalities on the FA map, and tract deficits on the right compared to the left on tractography images (Figure 11.36). DTI is reported to be a useful technique for detecting diffuse axonal injury of the corticospinal tract at the subcortical level [Jang et al., 2009]. Six patients with Arnold–Chiari II malformation underwent DTI, which showed reduced FA in the corpus callosum; it was also reduced in the area compared to the controls. There was a strong correlation between the size and FA of the corpus callosum in patients. In contrast, the thickness of the anterior commissure was significantly increased and was associated with higher FA in the Chiari II patients. DTI abnormalities seen in the corpus callosum in Chiari II patients point to developmental white matter damage in the corpus callosum beside that exerted by hydrocephalus [Herweh et al., 2009]. Sundaram and colleagues reported their DTI findings in 20 children (age 18–83 months) with global developmental delay [Sundaram et al., 2008]. In 9 out of 20 patients, the arcuate fasciculus was absent bilaterally, and in another 2 patients it was absent in the left cerebral hemisphere. In contrast, the arcuate fasciculus was present bilaterally in all typically developing children. FA was significantly reduced in right inferior longitudinal fasciculus in developmentally delayed children compared with controls. DTI can be used to identify absence of the arcuate fasciculus and inadequate maturation of inferior longitudinal fasciculus in children with global developmental delay of unknown etiology.
Challenges for tractography remain. Validations of the tractography images are mostly based on known neuroanatomy. This remains a limitation of this imaging technique. Intraoperative electrophysiological testing has been used to correlate preoperative tractography, which indicated that tractography might underestimate the fiber tracts [Kinoshita et al., 2005]. On-going development of tractography must be pursued to increase the signal to noise ratio while shortening the scan time, improving the in-plane image resolution, and reducing partial volume averaging due to slice thickness [Yamada et al., 2009]. Higher-field-strength scanners using multichannel head coils and parallel imaging techniques are being used to provide some of these crucial improvements. DTI has been one of the most remarkable advances in the field of neuroimaging in the past decade; however, it has to be used with caution, and an understanding that this imaging technique only represents a fraction of reality [Yamada et al., 2009].
Perfusion Magnetic Resonance Imaging
Perfusion-weighted imaging (PWI) is an extension of MR technology that allows evaluation of blood volume, blood transit time, and blood flow as relative measures [Warach, 1996]. PWI is primarily being investigated for its use in the evaluation of patients early after the onset of symptoms of cerebral ischemia, although other clinical applications are being investigated [Ashwal and Holshouser, 1997; Dunst et al., 2001; Tanner et al., 2003; Warach et al., 1996].
Two techniques have been developed to accomplish PWI on the basis of the magnetic susceptibility effect of gadolinium-containing contrast agents on the brain and the noninvasive magnetic labeling of arterial blood [Buxton et al., 1996; Warach, 1996]. The first, referred to as dynamic contrast-enhanced susceptibility-weighted perfusion imaging (CE-PWI), can be used to image relative differences in blood volume over time. Paramagnetic compounds such as gadolinium (Gd-DTPA), which are used as MRI contrast agents, possess strong magnetic susceptibility. The susceptibility differences between the contrast agent and the surrounding tissue create local magnetic field gradients that can be used to acquire images after contrast injection. Images can be viewed dynamically to assess entry and clearance of the contrast through the tissue, and the transit time can be calculated. Cerebral blood volume can be estimated, as can cerebral blood flow from the ratio of the cerebral blood volume and mean transit time through the brain.
The second method involves blood flow imaging by magnetically labeled protons of the arterial inflow using a radiofrequency pulse and following the label through the brain’s circulation. This method is known as arterial spin labeling (ASL). Deoxyhemoglobin contained within erythrocytes is paramagnetic, and on deoxygenation, the magnetic susceptibility of deoxyhemoglobin is increased and creates local field gradients around these cells. The blood oxygen level-dependent technique takes advantage of this phenomenon to quantify cerebral blood flow [Patel et al., 1995]. PWI can demonstrate regions of acute ischemia before lesions are detectable by MRI. ASL studies can be done rapidly, and they do not involve contrast injection.
Several studies have suggested that PWI, like DWI, can aid in the evaluation of acute ischemic injury, particularly by determining which patients have the potential for recovery and which may benefit from thrombolytic therapy [Ahmed and Masaryk, 2004; Grandin, 2003; Rowley and Roberts, 2004; Warach et al., 1995, 1996]. In patients with sickle cell disease, abnormalities on perfusion MRI are associated with neurologic symptoms although the areas of abnormality may not be seen in conventional MRI, MR angiography, or transcranial Doppler examination. Perfusion imaging is helpful in guiding management in such patients [Kirkham et al., 2001]. PWI has also been used to differentiate tumor types. In one study of adult and pediatric patients using relative cerebral blood volume maps calculated for assessment of tumor vascularity, differences were observed in patients with glioblastomas, anaplastic gliomas, and low-grade gliomas [Lee et al., 2001; Lacerda and Law, 2009]. Combined with spectroscopy, PWI findings can help differentiate progression from stable tumors [Tzika et al., 2004]. Another area of interest for DWI and PWI is in children with acute disseminated encephalomyelitis [Bernarding et al., 2002]. In children with cerebral arteriovenous malformation and other proliferative angiopathies, PWI may be helpful in determining areas of hyperperfusion, hypoperfusion, or venous congestion adjacent to the lesion [Ducreux et al., 2004].
Susceptibility-Weighted Imaging
A sequence using a high-spatial-resolution, three-dimensional, fast, low-angle MRI technique that is extremely sensitive to susceptibility has been described. This sequence, which can be performed on conventional scanners, was originally designed for MR venography using the paramagnetic property of intravascular deoxyhemoglobin [Lee et al., 1999a; Reichenbach et al., 1997].
This technique, known as susceptibility-weighted imaging (SWI), has been very useful in detecting hemorrhagic lesions associated with diffuse axonal injury, and it has depicted significantly more small hemorrhagic lesions than conventional T2*-weighted, gradient-echo techniques [Tong et al., 2003a]. In children with traumatic brain injury and diffuse axonal injury, the number and volume of hemorrhagic lesions detected with SWI have correlated with the initial severity of injury as measured by the Glasgow Coma Scale scores, duration of coma, and long-term outcome [Tong et al., 2004]. SWI can also be used to categorize tissue as normal-appearing or with nonhemorrhagic or hemorrhagic injury [Holshouser et al., 2005]. Using proton MR spectroscopic imaging, voxels with visibly injured (hemorrhagic) brain may demonstrate a significant decrease in NAA/Cre and increase in Cho/Cre compared with voxels with normal-appearing brain. Metabolite ratios from normal-appearing brain better predicted outcome than using ratios from visibly injured brain.
Neonatal strokes, which are sometimes related to dural sinus thrombosis, can be hemorrhagic (see Chapter 17). SWI is more sensitive than CT or conventional MRI sequences (including GRE T2*) in detecting hemorrhage in patients with acute strokes [Wycliffe et al., 2004]. This blood-sensitive sequence has been found to be extremely valuable in detection of hemorrhage in children with accidental or nonaccidental trauma [Tong et al., 2004; Colbert et al., 2010], infarctions [Wycliffe et al., 2004], tumors [Barth et al., 2003], proliferative angiopathies, and vascular malformations [Essig et al., 1999], including Sturge–Weber syndrome [Tong et al., 2003b], cavernous angioma [Lee et al., 1999a] (Figure 11-37), and in patients with hypertensive encephalopathy (Figure 11-38). In the examples of SWI (Figure 11-37 and Figure 11-38), both cases exhibit a markedly increased number and more intense abnormal signal on SWI than with other MRI sequences.
Functional Magnetic Resonance Imaging
Functional MRI is a technique that measures changes in tissue perfusion based on changes in blood oxygenation. It is used to study regional brain activity in response to sensory, motor, and cognitive stimulation [Ashwal and Holshouser, 1997; Rivkin, 2000; Strainer et al., 1997; Warach, 1996]. Functional MRI can be used to study the distribution of cerebral hemodynamic changes associated with various sensory stimuli, including vision [Zeki et al., 1991], sensorimotor [Rao et al., 1993], olfactory [Zatorre et al., 1992b], and auditory function [Binder et al., 1994; Zatorre, 1997]. Functional MRI has been used to investigate complex cognitive functions, such as speech and language processing and reading [Klein et al., 1995; McCarthy et al., 1993; Zatorre et al., 1992a, 1996] (see Chapter 46). Functional MRI allows investigators to study human cerebral activity in vivo as a function of perceptual or psychological variables.
Echoplanar functional MRI combined with appreciation of the blood oxygen level-dependent (BOLD) contrast mechanism can be used to image cortical responses to peripheral stimuli or cognitive task performance [Kwong, 1995; Ogawa and Lee, 1990; Ogawa et al., 1990a, 1990b]. The BOLD contrast mechanism relies on the fact that an increase in regional cortical blood flow occurs in response to task performance or stimulation but is not accompanied by a concomitant increase in local tissue oxygen extraction [Kwong, 1995]. The presence of any substance in a magnetic field alters that field to some degree [Mosley and Glover, 1995], and certain elements, such as iron, have a strong influence in altering the surrounding magnetic field. Products of reduced hemoglobin, such as deoxyhemoglobin, are stronger in altering the surrounding magnetic field than oxyhemoglobin. Cortical activation causes local vasodilatation with increased regional blood flow and oxyhemoglobin. Because of this inflow of oxyhemoglobin, the amount of deoxyhemoglobin in the stimulated cortex does not significantly increase. This regional imbalance of oxyhemoglobin and deoxyhemoglobin reduces the net “susceptibility effect” of deoxyhemoglobin, which can be detected by magnetic susceptibility-sensitive imaging techniques using gradient-recalled echo methods. MRI scanners equipped with echoplanar imaging can obtain gradient images of the stimulated cortex within seconds of stimulation and display increased signal in the stimulated cortex. More-detailed technical descriptions and applications of this technique are beyond the scope of this chapter, but several sources provide excellent reviews of this technology [Ashwal and Holshouser, 1997; Buxton et al., 1996; Fisher et al., 1992; Kwong, 1995; Mosley and Glover, 1995; Rivkin, 2000; Warach, 1996].
MRS can be used for functional imaging to detect lactate in eloquent brain cortex (i.e., cortex controlling sensorimotor, language, and visual function) during activation after a stimulus. An increase in lactate concentration has been observed in the occipital and auditory cortex after stimulation [Prichard, 1991; Sappey et al., 1992; Singh, 1992]. One publication implied that lactate is the preferred substrate for the tricarboxylic acid cycle in neurons [Tsacopoulos and Magistretti, 1996]. Using a model of metabolic compartmentalization, these investigators describe glucose as being taken up by astrocytes and converted to lactate, which is then released into the extracellular space and used by neurons. Functional MRS of the auditory cortex may be helpful in the detection of stimulus-induced and stable-biochemical spectroscopic changes in patients with hearing loss, and may be useful in assessing disease activity [Richards et al., 1997].
Neuronal structures underlying specific perceptual and cognitive processes can be delineated by functional MRI. With the aid of functional MRI, the development of brain function can be followed, and deviation from the normal pattern can be established [Billingsley-Marshall et al., 2004]. Early diagnosis of functional deficits has the potential to reduce residual deficits because of the earlier implementation of cognitive or other forms of rehabilitation [Martin and Marcar, 2001]. Acquisition of functional MRI data from children is associated with a number of methodological challenges, primarily compliance and head motion; however, good data can be obtained. Conditioning and personal interactions can improve compliance, and motion reduction techniques can successfully reduce artifacts resulting from movement [Poldrack et al., 2002].
Functional MRI is being used in patients with various neurologic diseases, including medically refractory epilepsy and brain tumors [Hunter et al., 1996; Lin et al., 1997]. Preoperative evaluation of such patients includes MRI and scalp, subdural, or depth electrode electroencephalography (EEG), and often includes endovascular amobarbital testing to determine which cerebral hemisphere is dominant (Wada test) [Brassel et al., 1996]. In epilepsy surgery candidates or patients with tumors, preoperative functional MRI [Garcia et al., 1995] has been used to determine the feasibility of a proposed surgical resection and to select patients for invasive surgical functional mapping [Lee et al., 1999b]. Functional MRI appears to be as sensitive and specific as the Wada test for speech localization, preoperative memory lateralization, and intraoperative cortical mapping [Lin et al., 1997; Sabbah et al., 2003]. Functional MRI performed immediately before surgery can help determine the location of neighboring eloquent functional area of concern in reference to a targeted lesion [Liu et al., 2003]. Some have used functional MRI and DTI together with anatomical imaging to improve functional outcomes after tumor resection [Wu et al., 2007]. An example of a patient with an arteriovenous malformation in the parietal lobe, who underwent functional MRI to map motor cortex and DTI to show white matter tracts, is shown in Figure 11-39. Information provided by functional MRI has contributed significantly to making optimal surgical decisions before craniotomy. In the future, functional MRI may replace the Wada test because it is much less invasive.
Magnetic Source Imaging
Magnetic source imaging uses magnetoencephalography (MEG). When source localizations modeled from the magnetoencephalographic signal are registered with high-resolution MRI, the resulting magnetic source images display functional information in an anatomic context [Gallen et al., 1993; Roberts et al., 1995]. In functional mapping of the sensorimotor cortex, a combination of magnetic source imaging and functional MRI allows improved sensitivity, fewer false-positive results, and high spatial and temporal resolution [Roberts and Rowley, 1997]. One of the important clinical applications of functional brain imaging is pretreatment mapping to allow definition of eloquent cortex in relation to mass lesions that may be surgically resected or treated with focused irradiation (e.g., gamma knife, protons) or combinations of irradiation and chemotherapy. Magnetic source imaging may also have future applications in studying developmental plasticity after injury. Functional reorganization (i.e., functional plasticity) of the brain can occur after unilateral brain infarction [Lewine et al., 1994], and a similar phenomenon is also found in patients with tumors [Takashi et al., 1997] and arteriovenous malformations [Hasson et al.,1997].
MEG is a powerful and accurate tool for the presurgical evaluation of children with refractory epilepsy [Chuang et al., 1995; Minassian et al., 1999; Stefan et al., 2000]. MEG is also helpful in evaluating patients with dyslexia. Studies have demonstrated aberrant activation maps consisting of reduced activity in left temporoparietal areas (including the posterior part of the superotemporal, angular, and supratemporal gyri) and increased activity in homologous cortex from the right hemisphere [Simos et al., 2000]. Studies using MEG in patients with normal MRI scans who had acquired aphasia and suspected Landau–Kleffner syndrome have demonstrated unilateral and bilateral perisylvian MEG spikes, as well as spikes from nonsylvian regions [Sobel et al., 2000]. MEG was considered helpful for diagnosis and preoperative localization of epileptiform activity [Sobel et al., 2000]. It also has been used in patients with tumors, and provides additional information regarding the spatial relation between brain lesions and functional cortex [Alberstone et al., 2000; Taniguchi et al., 1998]. MEG is also being used in association with three-dimensional MRI in neuronavigational systems. The method of incorporating functional data into neuronavigational systems is a promising tool that can potentially be used in more extensive surgical procedures to lessen injury to eloquent brain areas [Ganslandt et al., 1999].
Among the advantages of functional MRI, magnetic source imaging (MEG), and MRS are noninvasiveness, absence of ionizing radiation, and superior spatial resolution. Functional MRI is also complementary to anatomic MRI because functional imaging takes into account normal individual variations in the functional topography of the brain. It can detect altered topography or functional plasticity, which may present because of functional reorganization in patients with various forms of cerebral injury [Weiller et al., 1992]. MEG provides noninvasive localization data that are not otherwise available with MRI or conventional scalp ictal EEG [Knowlton et al., 1997].
Spinal Imaging
Initial assessment of spinal abnormalities in children includes plain films or, in the very young child, ultrasound. CT is usually indicated for acute spine injuries to determine whether a fracture, dislocation, or spondylolysis is present. CT is also helpful for the evaluation of benign tumors or vertebral body anomalies (see Chapter 78). For most infants and children, MRI has replaced myelography and CT myelography as the definitive procedure for spinal neuroaxis imaging, including spinal dysraphism, other developmental anomalies of the spine (Figure 11-40 and Figure 11-41), and acquired processes, such as neoplasms. CT myelography may have an advantage over MRI in patients with spinal dysraphism for demonstrating the location of cord tethering and for demonstrating nerve root avulsions after trauma. In the evaluation of the spinal bone marrow for metastatic disease, MRI is the procedure of choice (Figure 11-42). Detailed information on spine imaging of children is beyond the scope of this chapter, but several excellent reviews are available [Egloff et al., 2009; Gore et al., 2009; Roche and Carty, 2001; Khanna and El-Khoury, 2007].
Angiography
The role of angiography has diminished since the emergence of CT, MRI, and MRA in pediatric neuroimaging. Contrast angiography in children requires sedation and anesthesia, and may have complications such as death, stroke, and thrombosis of the femoral artery. It is usually restricted to the evaluation of vascular malformations, vasculopathy, vasculitis, vaso-occlusive disease (see Figure 11-10E), and occasionally to CNS tumors (see Chapters 18 and 85) [Eleftheriou et al., 2010].
Single-Photon Emission Computed Tomography and Positron Emission Tomography
Single photon emission computed tomography (SPECT) uses one or more gamma cameras rotating around the patient and acquiring numerous transmission scans after injection of a labeled radiopharmaceutical. Reconstruction techniques using filtered back projection reconstruction produce images in any orthogonal or oblique plane. There is widespread availability of multidetector SPECT. The oblique transverse or temporal lobe view is a useful adjunctive display, particularly in the presence of temporal lobe pathology. Significant advances in SPECT brain imaging radiopharmaceuticals have occurred in the past decade. Technetium 99m ethylene cysteinate dimer, a radiopharmaceutical used for brain perfusion, and Tc 99m hexamethylpropyleneamine oxime are the main radiotracers used for clinical brain SPECT imaging. These radiotracers have the advantage of a Tc 99m label with its optimal imaging characteristics. The indications for brain SPECT imaging in children [Gordon, 1998] include planning for epilepsy surgery [Cross, 2002], evaluation of brain death, acute neurologic loss (including stroke), language disorders, hypertension resulting from renal vascular disease, traumatic brain injury, and migraine. Pediatric psychological conditions in which regional cerebral blood flow studies are occasionally obtained include anorexia nervosa, autism, Gilles de la Tourette syndrome, and attention-deficit hyperactivity disorder [Gordon, 1998]. Considerable research is taking place in neuroreceptor imaging using iodinated- and technetium-labeled neuroligands to measure certain critical components of neurotransmission. On-going research is using SPECT and positron emission tomography (PET) in the study of psychiatric disorders such as schizophrenia, anxiety disorders, depression [Frankle and Laruelle, 2002; Smith et al., 2003], eating disorders [Frank et al., 2004], and stress and mood disorders [Kennedy and Zubieta, 2004]. Iodine131-labeled metaiodobenzylguanidine (MIBG) localizes in adrenergic neurons. In one study, it was used to evaluate the uptake of this radiopharmaceutical in the cerebral tissue in patients with neural crest tumors who had no evidence of CNS disease [Bomanji et al., 1991]. Focal uptake was seen in the cerebellum, basal nuclei, and thalamic region. SPECT with radiolabeled MIBG may prove to be useful for mapping the adrenergic receptors in the human brain [Bomanji et al., 1991]. Increased uptake of MIBG by the brain is reported, especially in cerebellum after therapeutic iodine 131 MIBG in patients with pheochromocytoma [Dwamena et al., 1998]. MIBG scans are used to evaluate and monitor children with neuroblastoma, along with CT or MRI scans, bone scans, bone marrow examination, and urine catecholamine measurements [Kushner, 2004].
Studies using PET scanning have made major contributions during the past decade to understanding the developmental neurobiology of the nervous system, particularly with reference to cerebral and cerebellar glucose, and oxidative metabolism and blood flow [Kim et al., 2010]. However, because of its limited availability, a comprehensive review of the technology and its clinical applications is not included here; several good sources are available [Townsend, 2008; Mawlawi and Townsend, 2009; Hicks and Lau, 2009; Kumar et al., 2005].
PET has been used to study patients with many different pediatric neurologic disorders, including various forms of epilepsy [Zupanc, 1996], Rasmussen’s encephalitis [Caplan et al., 1996], autism [Schifter et al., 1994], language disorders [Semrud-Clikeman, 1997], attention-deficit disorders [Chabot and Serfontein, 1996], movement disorders [Anouti and Koller, 1996], neonatal hypoxic-ischemic encephalopathy [Chugani, 1993], moyamoya disease [Ikezaki et al., 1994], tuberous sclerosis [Rintahaka and Chugani, 1997], Langerhans cell histiocytosis [Calming et al., 2002], and CNS tumors [Hoffman et al., 1992].
The most common clinical application is for pediatric epilepsy, particularly for patients who have not responded to pharmacologic management or the ketogenic diet. Such patients frequently have infantile spasms, focal ictal abnormalities in the frontal or temporal regions, or CNS malformations. These conditions are reviewed in greater detail in the chapters on epilepsy, particularly Chapter 61, on the surgical evaluation and treatment. PET is most useful for patients who have normal neuroimaging studies but clinically and electrographically have a focal seizure disorder or a generalized seizure disorder with significant asymmetries of activity [Chugani et al., 1994; Ferrie et al., 1996; Holopainen et al., 1997; Kramer et al., 1997; Sankar et al., 1995]. Interictal PET studies demonstrate areas of hypometabolic glucose metabolism using 2-deoxy-[18F] fluoro-d-glucose in areas considered epileptogenic. Ictal PET scans demonstrate more variable metabolic changes. Chugani and colleagues [1994] reported three major metabolic ictal PET patterns based on the degree and type of subcortical involvement: asymmetric activity in the striatum and thalamus; unilateral cortical hypermetabolism that always involved frontal cortex; and symmetric striatal and thalamic metabolic abnormalities, accompanied by hippocampal or insular cortex hypermetabolism, diffuse neocortical hypometabolism, and absence of any cerebellar abnormality.
In patients with refractory partial complex seizures, temporal lobe abnormalities can frequently be detected with PET [Adelson et al., 1992]. Studies have indicated congruence between focal temporal EEG abnormalities, hippocampal atrophy on MRI, hypometabolism in corresponding interictal regions with PET, and reductions in the metabolite ratios using proton MRS [Holopainen et al., 1997].
Patients with infantile spasms may also have significant EEG and PET cortical or subcortical asymmetries that have been shown useful in the evaluation for epilepsy surgery [Chugani, 1993; Kramer et al., 1997]. PET may reveal lateralized or localized regions of hypometabolic glucose use that corresponds to localized EEG abnormalities and cortical dysplasias or other structural abnormalities on neuropathologic examination. Surgical resection of regions with one hypometabolic area usually results in improved seizure control; patients with multiple hypometabolic regions are unlikely to benefit from such treatment.
PET has also been of particular value in children with intractable seizures who have subtle or major CNS malformations. These cases include children with tuberous sclerosis [Rintahaka and Chugani, 1997], Sturge–Weber syndrome [Juhász et al., 2007], hemimegalencephaly [Rintahaka et al., 1993], and focal cortical dysplasias and other migrational disorders [Chugani, 1992; Kim et al., 2000; Sankar et al., 1995; Zupanc, 1996]. Identification of such areas of hypometabolic activity in conjunction with EEG and clinical correlates usually provides sufficient evidence to consider surgical resection. PET imaging of children with CNS tumors is useful [Wegner et al., 2004]. Brain tumors can be histologically heterogeneous, and stereotactic biopsies may lead to inaccurate diagnosis or grading. Increased uptake with fluoro-d-glucose–PET may represent more malignant or aggressive tumors [Etzl et al., 2002]. By combining PET and MRI in the planning of a stereotactic brain biopsy, it may be possible to improve the diagnostic yield and reduce sampling from high-risk or functional areas [Massager et al., 2000; Pirotte et al., 2003].
References
The complete list of references for this chapter is available online at www.expertconsult.com.
Aaen G.S., Holshouser B.A., Sheridan C., et al. Magnetic resonance spectroscopy predicts outcome in children with nonaccidental trauma. Pediatrics. 2010;125(2):295-303.
Abdelhalim A.N., Alberico R.A. Pediatric neuroimaging. Neurol Clin. 2009;27(1):285-301.
Abe K., Yoshimura H., Tanaka H., et al. Comparison of conventional and diffusion-weighted MRI and proton MR spectroscopy in patients with mitochondrial encephalomyopathy, lactic acidosis, and stroke-like events. Neuroradiology. 2004;46:113.
Abrams L.A., Balducci J. Fetal seizures: A case study. Obstet Gynecol. 1996;88:61.
Adelson P.D., Peacock W.J., Chugani H.T., et al. Temporal and extended temporal resections for the treatment of intractable seizures in early childhood. Pediatr Neurosurg. 1992;18:169.
Ahmed M., Masaryk T.J. Imaging of acute stroke: State of the art. Semin Vasc Surg. 2004;17:181.
Aikele P., Kittner T., Offergeld C., et al. Diffusion-weighted MR imaging of cholesteatoma in pediatric and adult patients who have undergone middle ear surgery. Am J Roentgenol. 2003;181:261.
Alberstone C.D., Skirboll S.L., Benzel E.C., et al. Magnetic source imaging and brain surgery: Presurgical and intraoperative planning in 26 patients. J Neurosurg. 2000;92:79.
Anand N.K., Gupta A.K., Lamba I.M. Neurosonographic abnormalities in neonates with hypoxic ischemic encephalopathy. Indian Pediatr. 1994;31:767.
Andre M., Monin P., Moret C., et al. Pelizaeus-Merzbacher disease. Contribution of magnetic resonance imaging to an early diagnosis. J Neuroradiol. 1990;17:216.
Angtuaco E.E., Angtuaco T.L., Angtuaco E.J. Prenatal diagnosis of central nervous system abnormalities. Curr Probl Diagn Radiol. 1994;23:69.
Anouti A., Koller W.C. Diagnostic testing in movement disorders. Neurol Clin. 1996;14:169.
Arle J.E., Morriss C., Wang Z.J., et al. Prediction of posterior fossa tumor type in children by means of magnetic resonance image properties, spectroscopy, and neural networks. J Neurosurg. 1997;86:755.
Ashwal S., Holshouser B.A. New neuroimaging techniques and their potential role in patients with acute brain injury. J Head Trauma Rehab. 1997;12:13.
Ashwal S., Holshouser B.A., Tomasi L.G., et al. 1H-MRS determined cerebral lactate is associated with poor neurologic outcomes in children with central nervous system disease. Ann Neurol. 1997;41:470.
Ashwal S., Holshouser B.A., Shu S.K., et al. Predictive value of proton magnetic resonance spectroscopy in pediatric closed head injury. Pediatr Neurol. 2000;23:114.
Ashwal S., Holshouser B.A., Tong K., et al. Proton MR spectroscopy detected glutamate/glutamine is increased in children with traumatic brain injury. J Neurotrauma. 2004;21:1539.
Ashwal S., Holshouser B.A., Tong K., et al. Proton spectroscopy detected myoinositol in children with traumatic brain injury. Pediatr Res. 2004;56:1.
Aydin-Ozemir Z., Terzibasioglu E., Altindag E., et al. Magnetic resonance spectroscopy findings in photosensitive idiopathic generalized epilepsy. Clin EEG Neurosci. 2010;41:42.
Babikian T., Freier M.C., Ashwal S., et al. MR spectroscopy: predicting long-term neuropsychological outcome following pediatric TBI. J Magn Reson Imaging. 2006;24(4):801-811.
Babikian T., Tong K.A., Galloway N.R., et al. Diffusion-weighted imaging predicts cognition in pediatric brain injury. Pediatr Neurol. 2009;41(6):406-412.
Barker P.B., Bryan R.N., Kumar A.J., et al. Proton NMR spectroscopy of Canavan’s disease. Neuropediatrics. 1992;23:263.
Barkovich A.J. Pediatric neuroimaging, ed 4. New York: Lippincott Williams & Wilkins; 2005.
Barkovich A.J., Good W.V., Koch T.K., et al. Mitochondrial disorders: Analysis of their clinical and imaging characteristics. Am J Neuroradiol. 1993;14:1119.
Barkovich A.J., Westmark K.D., Bedi H.S., et al. Proton spectroscopy and diffusion imaging on the first day of life after perinatal asphyxia: Preliminary report. Am J Neuroradiol. 2001;22:1786.
Barth M., Nobauer-Huhmann I.M., Reichenbach J.R., et al. Enhanced BOLD MR-venography (CE-MRV) of brain tumors at 3 Tesla: First clinical experience. Invest Radiol. 2003;38:409.
Bartha A.I., Foster-Barber A., Miller S.P., et al. Neonatal encephalopathy: Association of cytokines with MR spectroscopy and outcome. Pediatr Res. 2004;56:960.
Basser P.J., Pajevic S., Pierpaoli C., et al. In vivo fiber tractography using DT-MRI data. Magn Reson Med. 2000;44:625.
Bernarding J., Braun J., Koennecke H.C. Diffusion- and perfusion-weighted MR imaging in a patient with acute demyelinating encephalomyelitis (ADEM). J Magn Reson Imaging. 2002;15:96.
Billingsley-Marshall R.L., Simos P.G., Papanicolaou A.C., et al. Reliability and validity of functional neuroimaging techniques for identifying language-critical areas in children and adults. Dev Neuropsychol. 2004;26:541.
Binder J.R., Rao S.M., Hammeke T.A., et al. Functional MRI of human auditory cortex. Ann Neurol. 1994;35:662.
Bolinger L., Insko E.K.. Spectroscopy: Basic principles and techniques. Edelman R.R., Zlatkin M.B., Hesselink J.R., editors. Clinical magnetic resonance imaging, 1. Philadelphia: WB Saunders, 1996.
Bomanji J., Moyers J., Huneidi A.H., et al. Cerebral uptake of MIBG: Adrenoreceptors visualized? Nucl Med Commun. 1991;12:3.
Bozzao A., Di Paolo A., Mazzoleni C., et al. Diffusion-weighted MR imaging in the early diagnosis of periventricular leukomalacia. Eur Radiol. 2003;13:1571.
Brassel F., Weissenborn K., Ruckert N., et al. Superselective intra-arterial Amytal (Wada test) in temporal lobe epilepsy: Basics for neuroradiological investigations. Neuroradiol. 1996;38:417.
Brenner T., Freier M.C., Holshouser B.A., et al. Predicting neuropsychologic outcome after traumatic brain injury in children. Pediatr Neurol. 2003;28:104.
Budorick N.E., Pretorius D.H., McGahan J.P., et al. Cephalocele detection in utero: Sonographic and clinical features. Ultrasound Obstet Gynecol. 1995;5:77.
Buxton R.B., Frank L.R., Prasad P.V.. Principles of diffusion and perfusion MRI. Edelman R.R., Zlatkin M.B., Hesselink J.R., editors. Clinical magnetic resonance imaging, 1. Philadelphia: WB Saunders, 1996.
Byrd S.E., Tomita T., Palka P.S., et al. Magnetic resonance spectroscopy (MRS) in the evaluation of pediatric brain tumors. Part II. Clinical analysis. J Natl Med Assoc. 1996;88:717.
Cady E.B., Amess P., Penrice J., et al. Early cerebral-metabolite quantification in perinatal hypoxic-ischaemic encephalopathy by proton and phosphorus magnetic resonance spectroscopy. Magn Reson Imaging. 1997;15:605.
Calming U., Bemstrand C., Mosskin M., et al. Brain 18-FDG PET scan in central nervous system Langerhans cell histiocytosis. J Pediatr. 2002;141:435.
Caplan R., Curtiss S., Chugani H.T., et al. Pediatric Rasmussen encephalitis: Social communication, language, PET and pathology before and after hemispherectomy. Brain Cogn. 1996;32:45.
Castillo M., Kwock L., Green C. MELAS syndrome: Imaging and proton MR spectroscopic findings. Am J Neuroradiol. 1995;16:233.
Cecil K.M., Jones B.V. Magnetic resonance spectroscopy of the pediatric brain. Top Magn Reson Imaging. 2001;12:435.
Chabot R.J., Serfontein G. Quantitative electroencephalographic profiles of children with attention deficit disorder. Biol Psychiatry. 1996;40:951.
Chabrol B., Vion-Dury J., Confort-Gouny S., et al. Magnetic resonance spectroscopy: A new technique for the exploration of brain metabolism in pediatrics. Arch Pediatr. 1995;2:783.
Chang Y.W., Yoon H.K., Shin H.J., et al. MR imaging of glioblastoma in children: Usefulness of diffusion/perfusion-weighted MRI and MR spectroscopy. Pediatr Radiol. 2003;33:836.
Chan Y.L., Chu W.C., Wong G.W., et al. Diffusion-weighted MRI in shaken baby syndrome. Pediatr Radiol. 2003;33:574.
Chen S., Ikawa F., Kurisu K., et al. Quantitative MR evaluation of intracranial epidermoid tumors by fast fluid-attenuated inversion recovery imaging and echo-planar diffusion-weighted imaging. Am J Neuroradiol. 2001;22:1089.
Choe B.Y., Suh T.S., Choi K.H., et al. Neuronal dysfunction in patients with closed head injury evaluated by in vivo 1H magnetic resonance spectroscopy. Invest Radiol. 1995;30:502.
Chu K., Kang D.W., Ko S.B., et al. Diffusion-weighted MR findings of central pontine and extrapontine myelinolysis. Acta Neurol Scand. 2001;104:385.
Chu Z., Wilde E.A., Hunter J.V., et al. Voxel-based analysis of diffusion tensor imaging in mild traumatic brain injury in adolescents. Am J Neuroradiol. 2010;31(2):340-346. Epub 2009 Dec 3
Chua G.C., Sitoh Y.Y., Lim C.C., et al. MRI findings in osmotic myelinolysis. Clin Radiol. 2002;57:800.
Chuang S.H., Otsubo H., Hwang P., et al. Pediatric magnetic source imaging. Neuroimaging Clin N Am. 1995;5:289.
Chugani H.T. Positron emission tomography scanning: Applications in newborns. Clin Perinatol. 1992;20:395.
Chugani H.T., Shewmon D.A., Shields W.D. Surgery for intractable infantile spasms: Neuroimaging perspectives. Epilepsia. 1993;34:764.
Chugani H.T., Rintahaka P.J., Shewmon D.A. Ictal patterns of cerebral glucose utilization in children with epilepsy. Epilepsia. 1994;35:813.
Colbert C., Holshouser B.A., Aaen G., et al. Value of Cerebral Microhemorrhages detected using Susceptibility Weighted Imaging for prediction of Long-term Outcome in Children with Non-accidental Trauma. Radiology. 2010. In Press
Connelly A., Jackson G.D., Duncan J.S., et al. Magnetic resonance spectroscopy in temporal lobe epilepsy. Neurology. 1994;44:1411.
Connelly A., Chong W.K., Johnson C.L., et al. Diffusion weighted magnetic resonance imaging of compromised tissue in stroke. Arch Dis Child. 1997;77:38.
Covarrubias D.J., Luetmer P.H., Campeau N.G. Posterior reversible encephalopathy syndrome: Prognostic utility of quantitative diffusion-weighted MR images. Am J Neuroradiol. 2002;23:1038.
Cowles T., Furman P., Wilkins I. Prenatal diagnosis of Dandy-Walker malformation in a family displaying X-linked inheritance. Prenat Diagn. 1993;13:87.
Cross J.H. Epilepsy surgery in childhood. Epilepsia. 2002;43(Suppl 3):65.
Cross J.H., Gadian D.G., Connelly A., et al. Proton magnetic resonance spectroscopy studies in lactic acidosis and mitochondrial disorders. J Inherit Metab Dis. 1993;16:800.
Cross J.H., Connelly A., Gadian D.G., et al. Clinical diversity of pyruvate dehydrogenase deficiency. Pediatr Neurol. 1994;10:276.
Dashti S.R., Decker D.D., Razzaq A., et al. Current patterns of inflicted head injury in children. Pediatr Neurosurg. 1999;31:302.
deVeber G., Andrew M., Canadian Pediatric Ischemic Stroke Study Group. Cerebral sinovenous thrombosis in children. N Engl J Med. 2001;345:417.
Dubowitz D.J., Bluml S., Arcinue E., et al. MR of hypoxic encephalopathy in children after near drowning: Correlation with quantitative proton MR spectroscopy and clinical outcome. Am J Neuroradiol. 1998;19:1617.
Ducreux D., Oppenheim C., Vandamme X., et al. Diffusion-weighted imaging patterns of brain damage associated with cerebral venous thrombosis. Am J Neuroradiol. 2001;22:261.
Ducreux D., Meder J.F., Fredy D., et al. MR perfusion imaging in proliferative angiopathy. Neuroradiology. 2004;46:105.
Duncan J.S. Magnetic resonance spectroscopy. Epilepsia. 1996;37:598.
Dunst J., Ahrens S., Paulussen M., et al. Prognostic impact of tumor perfusion in MR-imaging studies in Ewing tumors. Strahlenther Onkol. 2001;177:153.
Dwamena B.A., Zempel S., Klopper J.F., et al. Brain uptake of iodine-131 metaiodobenzylguanidine following therapy of malignant pheochromocytoma. Clin Nucl Med. 1998;23:441.
Egloff A.M., Kadom N., Vezina G., et al. Pediatric cervical spine trauma imaging: a practical approach. Pediatr Radiol. 2009;39(5):447-456. Epub 2008 Nov 12
Eleftheriou D., Cox T., Saunders D., et al. Investigation of childhood central nervous system vasculitis: magnetic resonance angiography versus catheter cerebral angiography. Dev Med Child Neurol. 2010. Jan 28
Engelbrecht V., Scherer A., Rassek M., et al. Diffusion-weighted MR imaging in the brain in children: Findings in the normal brain and in the brain with white matter diseases. Radiology. 2002;222:410.
Erdem E., Agildere M., Eryilmaz M., et al. Intracranial calcification in children on computed tomography. Turk J Pediatr. 1994;36:111.
Erdem E., Zimmerman R.A., Haselgrove J.C., et al. Diffusion-weighted imaging and fluid attenuated inversion recovery imaging in the evaluation of primitive neuroectodermal tumors. Neuroradiology. 2001;43:927.
Es S.V., North K.N., McHugh K., et al. MRI findings in children with neurofibromatosis type I: A prospective study. Pediatr Radiol. 1996;26:478.
Essig M., Reichenbach J.R., Schad L.R., et al. High-resolution MR venography of cerebral arteriovenous malformations. Magn Reson Imaging. 1999;17:1417.
Etzl M.M.Jr, Kaplan A.M., Moss S.D., et al. Positron emission tomography in three children with pleomorphic xanthoastrocytoma. J Child Neurol. 2002;17:522.
Farina L., Bergqvist C., Zimmerman R.A., et al. Acute diffusion abnormalities in the hippocampus of children with new-onset seizures: The development of mesial temporal sclerosis. Neuroradiology. 2004;46:251.
Feldman H.M., Yeatman J.D., Lee E.S., et al. Diffusion tensor imaging: a review for pediatric researchers and clinicians. J Dev Behav Pediatr. 2010;31(4):346-356.
Ferrie C.D., Maisey M., Cox T., et al. Focal abnormalities detected by 18FDG PET in epileptic encephalopathies. Arch Dis Child. 1996;75:102.
Fisher M., Sotak C.H., Minematsu K., et al. New magnetic resonance techniques for evaluating cerebrovascular disease. Ann Neurol. 1992;32:115.
Frank G.K., Bailer U.F., Henry S., et al. Neuroimaging studies in eating disorders. CNS Spectr. 2004;9:539.
Frankle W.G., Laruelle M. Neuroreceptor imaging in psychiatric disorders. Ann Nucl Med. 2002;16:437.
Frush D.P., Donnelly L.F., Rosen N.S. Computed tomography and radiation risks: What pediatric health care providers should know. Pediatrics. 2003;112:951.
Fujiwara S., Yamano T., Hattori M., et al. Asymptomatic cerebral infarction in Kawasaki disease. Pediatr Neurol. 1992;8:235.
Gallen C.C., Sobel D.F., Schwartz B., et al. Magnetic source imaging: Present and future. Invest Radiol. 1993;3:S153.
Galloway N.R., Tong K.A., Ashwal S., et al. Diffusion-weighted imaging improves outcome prediction in pediatric traumatic brain injury. J Neurotrauma. 2008;25(10):1153-1162.
Ganslandt O., Fahlbusch R., Nimsky C., et al. Functional neuronavigation with magnetoencephalography: Outcome in 50 patients with lesions around the motor cortex. J Neurosurg. 1999;91:73.
Garcia P.A., Laxer K.D., Ng T. Application of spectroscopic imaging in epilepsy. Magn Reson Imaging. 1995;13:1181.
Geffner M.E. Hypopituitarism in childhood. Cancer Control. 2002;9:212.
Gelal F.M., Grant P.E., Fischbein N.J., et al. The role of isotropic diffusion MRI in children under 2 years of age. Eur Radiol. 2001;11:1006.
Gordon I. Cerebral imaging in pediatrics. Q J Nucl Med. 1998;42:126.
Gore P.A., Chang S., Theodore N. Cervical spine injuries in children: attention to radiographic differences and stability compared to those in the adult patient. Semin Pediatr Neurol. 2009;16(1):42-58.
Goyal M., Bangert B.A., Lewin J.S., et al. High-resolution MRI enhances identification of lesions amenable to surgical therapy in children with intractable epilepsy. Epilepsia. 2004;45:954.
Grandin C.B. Assessment of brain perfusion with MRI: Methodology and application to acute stroke. Neuroradiology. 2003;45:755.
Groenendaal F., Veenhoven R.H., van der Grond J., et al. Cerebral lactate and N-acetyl-aspartate/choline ratios in asphyxiated full-term neonates demonstrated in vivo using proton magnetic resonance spectroscopy. Pediatr Res. 1994;35:148.
Guilhoto L.M., Osorio C.A., Machado L.R., et al. Pediatric multiple sclerosis: Report of 14 cases. Brain Dev. 1995;17:9.
Harbord M.G., Finn J.P., Hall-Craggs M.A., et al. Myelination patterns on magnetic resonance of children with developmental delay. Dev Med Child Neurol. 1990;32:295.
Hasson R.M., Tarr R.W., Lewin J.R., et al. Clinical application of BOLD functional magnetic resonance imaging in patients with cerebral arteriovenous malformations. In: Proceedings of the American Society of Neuroradiology (ASNR)/American Society of Head and Neck Radiology (ASHNR). Oak Brook, Ill: American Society of Neuroradiology; 1997:176.
Heckel S., Favre R., Gasser B., et al. Prenatal diagnosis of a congenital astrocytoma: A case report and literature review. Ultrasound Obstet Gynecol. 1995;5:63.
Herweh C., Akbar M., Wengenroth M., et al. DTI of commissural fibers in patients with Chiari II-malformation. Neuroimage. 2009;44:306.
Hetherington H.P., Kuzniecky R.I., Pan J.W., et al. Application of high field spectroscopic imaging in the evaluation of temporal lobe epilepsy. Magn Reson Imaging. 1995;13:1175.
Hicks R.J., Lau E.W. PET/MRI: a different spin from under the rim. Eur J Nucl Med Mol Imaging. 2009;36(Suppl 1):S10-S14. PubMed PMID: 19104800
Ho V.B., Chuang H.S., Rovira M.J., et al. Juvenile Huntington disease: CT and MR features. Am J Neuroradiol. 1995;16:1405.
Hoffman J.M., Hanson M.W., Friedman H.S., et al. FDG-PET in pediatric posterior fossa brain tumors. J Comput Assist Tomogr. 1992;16:62.
Holopainen I.E., Lundbom N.M., Metsahonkala E.L., et al. Temporal lobe pathology in epilepsy: Proton magnetic resonance spectroscopy and positron emission tomography study. Pediatr Neurol. 1997;16:98.
Holshouser B.A., Ashwal S., Luh G.Y., et al. Proton MR spectroscopy after acute central nervous system injury: Outcome prediction in neonates, infants, and children. Radiology. 1997;202:487.
Holshouser B.A., Tong K.A., Ashwal S. Proton magnetic resonance spectroscopic imaging detects diffuse axonal injury in pediatric brain trauma. Am J Neuroradiol. 2005;26:1276-1285.
Holzgreve W., Feil R., Louwen F., et al. Prenatal diagnosis and management of fetal hydrocephaly and lissencephaly. Child Nerv Syst. 1993;9:408.
Hugg J.W., Laxer K.D., Matson G.B., et al. Neuron loss localizes human temporal lobe epilepsy by in vivo proton magnetic resonance spectroscopic imaging. Ann Neurol. 1993;34:788.
Hunter J.V. Magnetic resonance imaging in pediatric stroke. Top Magn Reson Imaging. 2002;13:23.
Hunter J.V., Brown L., Haselgrove J., et al. Comparison of hemispheric dominance for language as identified by Wada testing versus functional MR imaging in a pediatric population. In: Proceedings of the American Society of Neuroradiology. Oak Brook, Ill: American Society of Neuroradiology; 1996:86.
Hunter J.V., Thornton R.J., Wang Z.J., et al. Late proton MR spectroscopy in children after traumatic brain injury: correlation with cognitive outcomes. Am J Neuroradiol. 2005;26(3):482.
Huppi P.S. Immature white matter lesions in the premature infant. J Pediatr. 2004;145:575.
Hüppi P.S., Dubois J. Diffusion tensor imaging of brain development. Semin Fetal Neonatal Med. 2006;11(6):489-497.
Ikezaki K., Matsushima T., Kuwabara Y., et al. Cerebral circulation and oxygen metabolism in childhood moyamoya disease: A perioperative positron emission tomography study. J Neurosurg. 1994;81:843.
Inder T.E., Anderson N.J., Spencer C., et al. White matter injury in the premature infant: A comparison between serial cranial sonographic and MR findings at term. Am J Neuroradiol. 2003;24:805.
Iruretagoyena J.I., Trampe B., Shah D. Prenatal Diagnosis of Chiari Malformation with syringomyelia in the second trimester. J Matern Fetal Neonatal Med. 2010;23:184.
Ito R., Mori S., Melhem E.R. Diffusion tensor brain imaging and tractography. Neuroimaging Clin N Am. 2002;12:1.
Jackson G.D. New techniques in magnetic resonance and epilepsy. Epilepsia. 1994;35(Suppl 6):S2.
Jang S.H., Kim S.H., Kim O.L., et al. Corticospinal tract injury in patients with diffuse axonal injury: a diffusion tensor imaging study. NeuroRehabilitation. 2009;25:229.
Jones B.V., Koch B.L. Magnetic resonance imaging of the pediatric head and neck. Top Magn Reson Imaging. 1999;10:348.
Juhász C., Haacke E.M., Hu J., et al. Multimodality imaging of cortical and white matter abnormalities in Sturge-Weber syndrome. Am J Neuroradiol. 2007;28(5):900-906.
Kaufmann P., Shungu D.C., Sano M.C., et al. Cerebral lactic acidosis correlates with neurological impairment in MELAS. Neurology. 2004;62:1297.
Kealey S.M., Provenzale J.M. Tensor diffusion imaging in B12 leukoencephalopathy. J Comput Assist Tomogr. 2002;26:952.
Keller E., Flacke S., Urbach H., et al. Diffusion- and perfusion-weighted magnetic resonance imaging in deep cerebral venous thrombosis. Stroke. 1999;30:1144.
Kelly P.J. Computer assisted stereotactic biopsy and volumetric resection of pediatric brain tumors. Neurol Clin. 1991;9:317.
Kennedy S.E., Zubieta J.K. Neuroreceptor imaging of stress and mood disorders. CNS Spectr. 2004;9:292.
Ketonen L.M., Valanne L. Neuroimaging of pediatric diseases. Semin Neurol. 2008;28(4):558-569. Epub 2008 Oct 8
Khanna G., El-Khoury G.Y. Imaging of cervical spine injuries of childhood. Skeletal Radiol. 2007;36(6):477-494. Epub 2006 Oct 24
Kim I.O., Kim J.H., Kim W.S., et al. Mitochondrial myopathy-encephalopathy-lactic acidosis- and stroke-like episodes (MELAS) syndrome: CT and MR findings in seven children. Am J Roentgenol. 1996;166:641.
Kim S.K., Na D.G., Byun H.S., et al. Focal cortical dysplasia: Comparison of MRI and FDG-PET. J Comput Assist Tomogr. 2000;24:296.
Kim S., Salamon N., Jackson H.A., et al. PET imaging in pediatric neuroradiology: current and future applications. Pediatr Radiol. 2010;40(1):82-96.
King A.D., Walshe J.M., Kendall B.E., et al. Cranial MR imaging in Wilson’s disease. Am J Roentgenol. 1996;167:1579.
Kinoshita M., Yamada K., Hashimoto N. Fiber-tracking does not accurately estimate size of fiber bundle in pathological condition: initial neurosurgical experience using neuronavigation and subcortical white matter stimulation. Neuroimage. 2005;25:424.
Kinoshita Y., Kajiwara H., Yokota A., et al. Proton magnetic resonance spectroscopy of brain tumors: An in vitro study. Neurosurgery. 1994;35:606.
Kirimi E., Tuncer O., Atas B., et al. Clinical value of color Doppler ultrasonography measurements of full-term newborns with perinatal asphyxia and hypoxic ischemic encephalopathy in the first 12 hours of life and long-term prognosis. Tohoku J Exp Med. 2002;197:27.
Kirkham F.J., DeBaun M.R. Stroke in children with sickle cell disease. Curr Treat Options Neurol. 2004;6:357.
Kirkham F.J., Calamante F., Bynevelt M., et al. Perfusion magnetic resonance abnormalities in patients with sickle cell disease. Ann Neurol. 2001;49:477.
Klein D., Milner B., Zatore R.J., et al. The neural substrates underlying word generation: A bilingual functional imaging study. Proc Natl Acad Sci USA. 1995;92:2899.
Klingberg T., Vaidya C.J., Gabrieli J.D., et al. Myelination and organization of the frontal white matter in children: A diffusion tensor MRI study. Neuroreport. 1999;10:2817.
Knowlton R.C., Laxer K.D., Aminoff M.J., et al. Magnetoencephalography in partial epilepsy: Clinical yield and localization accuracy. Ann Neurol. 1997;42:622.
Koelfen W., Freund M., Guckel F., et al. MRI of encephalitis in children: Comparison of CT and MRI in the acute stage with long-term follow-up. Neuroradiology. 1996;38:73.
Kovanlikaya A., Ashok Panigrahy A., Krieger M.D., et al. Untreated pediatric primitive neuroectodermal tumor in vivo: Quantitation of taurine with MR Spectroscopy. Radiology. 2005;236:1020.
Krageloh M.I., Grodd W., Schoning M., et al. Proton spectroscopy in five patients with Leigh’s disease and mitochondrial enzyme deficiency. Dev Med Child Neurol. 1993;35:769.
Kramer U., Sue W.C., Mikati M.A. Focal features in West syndrome indicating candidacy for surgery. Pediatr Neurol. 1997;16:213.
Kreis R., Ernst T., Ross B.D. Development of the human brain: In vivo quantification of metabolite and water content with proton magnetic resonance spectroscopy. Magn Reson Med. 1993;30:424.
Kreis R., Pfenninger J., Herschkowitz N., et al. In vivo proton magnetic resonance spectroscopy in a case of Reye’s syndrome. Intensive Care Med. 1995;21:266.
Krishnamoorthy K.S., Soman T.B., Takeoka M., et al. Diffusion-weighted imaging in neonatal cerebral infarction: Clinical utility and follow-up. J Child Neurol. 2000;15:592.
Kruse B., Barker P.B., van Zijl P.C., et al. Multislice proton magnetic resonance spectroscopic imaging in X-linked adrenoleukodystrophy. Ann Neurol. 1994;36:595.
Kumar S., Rajshekher G., Prabhakar S. Positron emission tomography in neurological diseases. Neurol India. 2005;53(2):149-155.
Kunimatsu A., Aoki S., Masutani Y., et al. Three-dimensional white matter tractography by diffusion tensor imaging in ischaemic stroke involving the corticospinal tract. Neuroradiology. 2003;45:532.
Kushner B.H. Neuroblastoma: A disease requiring a multitude of imaging studies. J Nucl Med. 2004;45:1172.
Kuzniecky R. Clinical applications of MR spectroscopy in epilepsy. Neuroimaging Clin N Am. 2004;14:507.
Kuzniecky R.I., Burgard S., Bilir E., et al. Qualitative MRI segmentation in mesial temporal sclerosis: Clinical correlations. Epilepsia. 1996;37:433.
Kwong K.K. Functional magnetic resonance imaging with echo planar imaging. Magn Reson Q. 1995;11:1.
Lacerda S., Law M. Magnetic resonance perfusion and permeability imaging in brain tumors. Neuroimaging Clin N Am. 2009;19(4):527-557.
Lee W.T., Wang P.J., Liu H.M., et al. Acute disseminated encephalomyelitis in children: Clinical, neuroimaging and neurophysiologic studies. Zhonghua Min Guo Xiao Er Ke Yi Xue Hui Za Zhi. 1996;37:197.
Lee B.C., Vo K.D., Kido D.K., et al. MR high-resolution blood oxygenation level-dependent venograph of occult (low-flow) vascular lesions. Am J Neuroradiol. 1999;20:1239.
Lee C.C., Ward H.A., Sharbrough F.W., et al. Assessment of functional MR imaging in neurosurgical planning. Am J Neuroradiol. 1999;20:1511.
Lee S.J., Kim J.H., Kim Y.M., et al. Perfusion MR imaging in gliomas: Comparison with histologic tumor grade. Korean J Radiol. 2001;2:1.
Lee J.E., Park H.J., Park B., et al. A comparative analysis of cognitive profiles and white-matter alterations using voxel-based diffusion tensor imaging between patient’s with Parkinson’s disease dementia and dementia with Lewy bodies. J Neurol Neurosurg Psychiatry. 2010;81:320.
Leite R.A., Otaduy M.C., e Silva G.E., et al. Diagnostic methods for extra-temporal neocortical focal epilepsies: present and future. Arq Neuropsiquiatr. 2010;68:119.
Leuthardt E.C., Wippold F.J.2nd, Oswood M.C., et al. Diffusion-weighted MR imaging in the preoperative assessment of brain abscesses. Surg Neurol. 2002;58:395.
Levine D., Barnes P.D., Madsen J.R., et al. Fetal Central Nervous System Anomalies: MR imaging Augments Sonographic Diagnosis. Radiology. 1997;204:635.
Lewine J.D., Astur R.S., Davis L.E., et al. Cortical organization in adulthood is modified by neonatal infarct. A case study. Radiology. 1994;190:93.
Lim C.C., Lee W., Chung S.M., et al. Diffusion-weighted MR imaging in intracranial infections. Ann Acad Med Singapore. 2003;32:446.
Lin J.C., Gates J.R., Ritter F.J., et al. Preoperative functional MR imaging to assess language and memory lateralization in patients with surgical brain disease. In: Proceedings of the American Society of Neuroradiology (ASNR)/American Society of Head and Neck Radiology (ASHNR). Oak Brook, Ill: American Society of Neuroradiology; 1997:173.
Lindegaard K.F., Sorteberg W., Normes H. Transcranial Doppler in neurosurgery. Avd Tech Stand Neurosurg. 1993;20:39.
Liu H., Hall W.A., Truwit C.L. The roles of functional MRI in MR-guided neurosurgery in a combined 1.5 Tesla MR-operating room. Acta Neurochir Suppl. 2003;85:127.
Loes D.J., Hite S., Moser H., et al. Adrenoleukodystrophy: A scoring method for brain MR observations. Am J Neuroradiol. 1994;15:1761.
Lutsep H.L., Albers G.W., DeCrespigny A., et al. Clinical utility of diffusion-weighted magnetic resonance imaging in the assessment of ischemic stroke. Ann Neurol. 1997;41:574.
Magalhaes A.C., Caramelli P., Menezes J.R., et al. Wilson’s disease: MRI with clinical correlation. Neuroradiol. 1994;36:97.
Maghnie M., Cosi G., Genovese E., et al. Central diabetes insipidus in children and young adults. N Engl J Med. 2000;343:998.
Manchola I.F., DeSalles A.A., Foo T.K., et al. Arteriovenous malformation hemodynamics: A transcranial Doppler study. Neurosurgery. 1993;33:556.
Mandelli M.L., De Simone T., Minati L., et al. Diffusion tensor imaging of spinocerebellar ataxias types 1 and 2. Am J Neuroradiol. 2007;28:1996.
Martin E., Marcar V.L. Functional MR imaging in pediatrics. Magn Reson Imaging Clin N Am. 2001;9:231.
Martin E., Buchli R., Ritter S., et al. Diagnostic and prognostic value of cerebral 31P magnetic resonance spectroscopy in neonates with perinatal asphyxia. Pediatr Res. 1996;40:749.
Massager N., David P., Goldman S., et al. Combined magnetic resonance imaging- and positron emission tomography-guided stereotactic biopsy in brainstem mass lesions: Diagnostic yield in a series of 30 patients. J Neurosurg. 2000;93:951.
Matsuo K., Mizuno T., Yamada K., et al. Cerebral white matter damage in frontotemporal dementia assessed by diffusion tensor tractography. Neuroradiology. 2008;50:605.
Matthews P.M., Andermann F., Silver K., et al. Proton MR spectroscopic characterization of differences in regional brain metabolic abnormalities in mitochondrial encephalomyopathies. Neurology. 1993;43:2484.
Matthews V.P., Barker P.B., Blackband S.J., et al. Cerebral metabolites in patients with acute and subacute strokes: Concentrations determined by quantitative proton MR spectroscopy. Am J Roentgenol. 1995;165:633.
Mawlawi O., Townsend D.W. Multimodality imaging: an update on PET/CT technology. Eur J Nucl Med Mol Imaging. 2009;36(Suppl 1):S15-S29.
McCarthy G., Blamire A.M., Rothman D.L., et al. Echo-planar magnetic resonance imaging studies of frontal cortex activation during word generation in humans. Proc Natl Acad Sci USA. 1993;90:4952.
Ment L.R., Bada H.S., Barnes P., et al. Practice parameter: Neuroimaging of the neonate. Neurology. 2002;58:1726.
Ment L.R., Hirtz D., Hüppi P.S. Imaging biomarkers of outcome in the developing preterm brain. Lancet Neurol. 2009;8(11):1042-1055. Epub 2009 Sep 30
Miller J.H., McKinstry R.C., Philip J.V., et al. Diffusion-tensor MR imaging of normal brain maturation: a guide to structural development and myelination. Am J Roentgenol. 2003;180(3):851-859.
Miller S.P., Cozzio C.C., Goldstein R.B., et al. Comparing the diagnosis of white matter injury in premature newborns with serial MR imaging and transfontanel ultrasonography findings. Am J Neuroradiol. 2003;24:1661.
Miller S.P., Ramaswamy V., Michelson D., et al. Patterns of Brain Injury in Term Neonatal Encephalopathy. J Pediatr. 2005;146:453-460.
Minassian B.A., Otsubo H., Weiss S., et al. Magnetoencephalographic localization in pediatric epilepsy surgery: Comparison with invasive intracranial electroencephalography. Ann Neurol. 1999;46:627.
Mirowitz S.A., Santor K., Prensky A.J., et al. Neurodegenerative diseases of childhood: MR and CT evaluation. J Comput Assist Tomogr. 1991;15:210.
Mittle R.I., Grossman R.I., Hiehle J.R., et al. Prevalence of MR evidence of diffuse axonal injury in patients with mild head injury and normal head CT findings. Am J Neuroradiol. 1994;15:1583.
Mohamed A., Wyllie E., Ruggieri P., et al. Temporal lobe epilepsy due to hippocampal sclerosis in pediatric candidates for epilepsy surgery. Neurology. 2001;56:1643.
Mosley M.E., Glover G.H. Functional MR imaging capabilities and limitations. Neuroimaging Clin N Am. 1995;5:161.
Mukherjee P., McKinstry R.C. Diffusion tensor imaging and tractography of human brain development. Neuroimaging Clin N Am. 2006;16(1):19-43. vii
Müller H., Beedgen B., Schenk J.P., et al. Intracerebellar hemorrhage in premature infants: sonographic detection and outcome. J Perinat Med. 2007;35(1):67-70.
Murakami A., Morimoto M., Yamada K., et al. Fiber-tracking techniques can predict the degree of neurologic impairment for periventricular leukomalacia. Pediatrics. 2008;122:656.
Negendank W.G., Sauter R., Brown T.R., et al. Proton magnetic resonance spectroscopy in patients with glial tumors: A multicenter study. J Neurosurg. 1996;84:449.
Newmeyer A., Cecil K.M., Schapiro M., et al. Incidence of brain creatine transporter deficiency in males with developmental delay referred for brain magnetic resonance imaging. J Dev Behav Pediatr. 2005;26(4):276-282.
Nomura Y., Sakuma H., Takeda K., et al. Diffusional anisotropy of the human brain assessed with diffusion-weighted MR: Relation with normal brain development and aging. Am J Neuroradiol. 1994;15:231.
Novotny E.J.Jr. Overview—the role of NMR spectroscopy in epilepsy. Magn Reson Imaging. 1995;13:1171.
Novotny E., Ashwal S., Shevell M. Proton magnetic resonance spectroscopy: An emerging technology in pediatric neurology research. Pediatr Res. 1998;44:1.
Nyberg D.A., Hyett J., Johnson J.A., et al. First-trimester screening. Radiol Clin North Am. 2006;44:837.
Ogawa S., Lee T.M. Magnetic resonance imaging of blood vessels at high fields: In vivo and in vitro measurements and image simulation. Magn Reson Med. 1990;16:9.
Ogawa S., Lee T.M., Kay A.R., et al. Brain magnetic resonance imaging with contrast dependent on blood oxygenation. Proc Natl Acad Sci USA. 1990;87:9868.
Ogawa S., Lee T.M., Nayak A.S., et al. Oxygenation sensitive contrast in magnetic resonance image of rodent brain at high magnetic fields. Magn Reson Med. 1990;14:68.
Oksi J., Kalimo H., Marttila R.J., et al. Inflammatory brain changes in Lyme borreliosis. A report on three patients and review of literature. Brain. 1996;119:2143.
Osborn A.G., Tong K.A. Handbook of neuroradiology: Brain and skull, ed 2. St. Louis: Mosby; 1996.
Ou S.F., Chi C.S., Shian W.J., et al. Clinical and MRI study of the Hallervorden-Spatz syndrome: Long-term follow-up of one case. Chung Hua Min Kuo Hsiao Erh Ko I Hsueh Hui Tsa Chih. 1994;35:439.
Panigrahy A., Nelson M.D.Jr, Blüml S. Magnetic resonance spectroscopy in pediatric neuroradiology: clinical and research applications. Pediatr Radiol. 2010;40(1):3-30.
Patel M.R., Siewert B., Warach S., et al. Diffusion and perfusion imaging techniques. Magn Reson Imaging Clin N Am. 1995;3:425.
Pezzullo J.A., Tung G.A., Mudigonda S., et al. Diffusion-weighted MR imaging of pyogenic ventriculitis. Am J Roentgenol. 2003;180:71.
Phillips M.D., Zimmerman R.A. Diffusion imaging in pediatric hypoxic ischemia injury. Neuroimaging Clin N Am. 1999;9:41.
Pirotte B., Goldman S., Salzberg S., et al. Combined positron emission tomography and magnetic resonance imaging for the planning of stereotactic brain biopsies in children: Experience in 9 cases. Pediatr Neurosurg. 2003;38:146.
Poldrack R.A., Pare-Blagoev E.J., Grant P.E. Pediatric functional magnetic resonance imaging: Progress and challenges. Top Magn Reson Imaging. 2002;13:61.
Prichard J.W. What the clinician can learn from MRS lactate measurements. NMR Biomed. 1991;4:99.
Prichard J.W.. Magnetic resonance spectroscopy of cerebral metabolism in vivo. Asbury A.K., McKhann G.M., McDonald W.I., editors. Disease of the nervous system: Clinical neurobiology, 2. New York: WB Saunders, 1993.
Provenzale J.M. Advances in pediatric neuroradiology: highlights of the recent medical literature. Am J Roentgenol. 2009;192(1):19-25.
Rao S.M., Binder J.R., Bandetinni P.A., et al. Functional magnetic resonance imaging of complex human movements. Neurology. 1993;43:2311.
Reichenbach J.R., Venkatesan R., Schillinger D.J., et al. Small vessels in the human brain: MR venography with deoxyhemoglobin as an intrinsic contrast agent. Radiology. 1997;204:272.
Richards T.L., Gates G.A., Gardner J.C., et al. Functional MR spectroscopy of the auditory cortex in healthy subjects and patients with sudden hearing loss. Am J Neuroradiol. 1997;18:611.
Rintahaka P.J., Chugani H.T. Clinical role of positron emission tomography in children with tuberous sclerosis complex. J Child Neurol. 1997;12:42.
Rintahaka P.J., Chugani H.T., Messa C., et al. Hemimegalencephaly: Evaluation with positron emission tomography. Pediatr Neurol. 1993;9:21.
Rivkin M.J. Developmental neuroimaging of children using magnetic resonance techniques. Ment Retard Dev Disabil Res Rev. 2000;6:68.
Roberts P.L., Rowley H.A. Mapping of sensorimotor cortex: Functional MR and magnetic source imaging. Am J Neuroradiol. 1997;18:871.
Roberts T., Rowley H., Kucharczyk J. Applications of magnetic source imaging to presurgical brain mapping. Neuroimaging Clin N Am. 1995;5:251.
Robertson N.J., Lewis R.H., Cowan F.M., et al. Early increases in brain myo-inositol measured by proton magnetic resonance spectroscopy in term infants with neonatal encephalopathy. Pediatr Res. 2001;50:692.
Roche C., Carty H. Spinal trauma in children. Pediatr Radiol. 2001;31(10):677-700.
Rodriguez J., Claus D., Verellen G., et al. Periventricular leukomalacia: Ultrasonic and neuropathological correlations. Dev Med Child Neurol. 1990;32:347.
Roosendaal S.D., Geurts J.J., Vrenken H., et al. Regional DTI differences in multiple sclerosis patients. Neuroimage. 2009;44:1397.
Ross B., Michaelis T. Clinical applications of magnetic resonance spectroscopy. Magn Reson Q. 1994;10:191.
Ross B., Kreis R., Ernst T. Clinical tools for the 90s: Magnetic resonance spectroscopy and metabolite imaging. Eur J Radiol. 1992;14:128.
Roume J., Larroche J.C., Razavi-Encha F., et al. Fetal hydrocephalus. Clinical significance of associated anomalies and genetic counseling: A pathological approach. Genet Couns. 1990;1:185.
Rowley H.A., Roberts T.P. Clinical perspectives in perfusion: Neuroradiologic applications. Top Magn Reson Imaging. 2004;15:28.
Ruzek K.A., Campeau N.G., Miller G.M. Early diagnosis of central pontine myelinolysis with diffusion-weighted imaging. Am J Neuroradiol. 2004;25:210.
Sabbah P., Chassoux F., Leveque C., et al. Functional MR imaging in assessment of language dominance in epileptic patients. Neuroimage. 2003;18:460.
Sankar R., Curran J.G., Kevill J.W., et al. Microscopic cortical dysplasia in infantile spasms: Evolution of white matter abnormalities. Am J Neuroradiol. 1995;16:1265.
Sappey M.D., Calabrese G., Fein G., et al. Effect of photic stimulation on human visual cortex lactate and phosphates using 1H and 31P magnetic resonance spectroscopy. J Cereb Blood Flow Metab. 1992;12:584.
Sasaki M., Sakuragawa N., Takashima S., et al. MRI and CT findings in Krabbe disease. Pediatr Neurol. 1991;7:283.
Savoiardo M., Halliday W.C., Nardocci N., et al. Hallervorden-Spatz disease: MR and pathologic findings. Am J Neuroradiol. 1993;14:155.
Schifter T., Hoffman J.M., Hatten H.P.Jr, et al. Neuroimaging in infantile autism. J Child Neurol. 1994;9:155.
Schneider J.F., Il’yasov K.A., Boltshauser E., et al. Diffusion tensor imaging in cases of adrenoleukodystrophy: Preliminary experience as a marker for early demyelination? Am J Neuroradiol. 2003;24:819.
Sehgal A., El-Naggar W., Glanc P., et al. Risk factors and ultrasonographic profile of posterior fossa haemorrhages in preterm infants. J Paediatr Child Health. 2009;45:215.
Semrud-Clikeman M. Evidence from imaging on the relationship between brain structure and developmental language disorders. Semin Pediatr Neurol. 1997;4:117.
Senda J., Ito M., Watanabe H., et al. Correlation between pyramidal tract degeneration and widespread white matter involvement in amyotrophic lateral sclerosis: a study with tractography and diffusion-tensor imaging. Amyotroph Lateral Scler. 2009;10:288.
Sener R.N. Herpes simplex encephalitis: Diffusion MR imaging findings. Comput Med Imaging Graph. 2001;25:391.
Sener R.N. Diffusion MRI findings in neonatal brain abscess. J Neuroradiol. 2004;31:69.
Shevell M.I., Didomenicantonio G., Sylvain M., et al. Glutaric acidemia type II: Neuroimaging and spectroscopy evidence for developmental encephalomyopathy. Pediatr Neurol. 1995;12:350.
Shu S.K., Knierim D., Ashwal S., et al. Predictive value of proton magnetic resonance spectroscopy in closed head injury in children. Ann Neurol. 1997;42:528.
Sijens P.E., Knopp M.V., Brunetti A., et al. 1H MR spectroscopy in patients with metastatic brain tumors: A multicenter study. Magn Reson Med. 1995;33:818.
Simon B., Letourneau P., Vitorino E., et al. Pediatric minor head trauma: Indications for computed tomographic scanning revisited. J Trauma. 2001;51:231.
Simos P.G., Breier J.I., Fletcher J.M., et al. Brain activation profiles in dyslexic children during non-word reading: A magnetic source imaging study. Neurosci Lett. 2000;290:61.
Singh M. Toward proton MR spectroscopic imaging of stimulated brain function. IEEE Trans Nucl Sci. 1992;39:1161.
Singh S., Kalra M.K., Moore M.A., et al. Dose reduction and compliance with pediatric CT protocols adapted to patient size, clinical indication, and number of prior studies. Radiology. 2009;252(1):200-208. Epub 2009 May 12
Smith R., Leonidas J.C., Maytal J. The value of head ultrasound in infants with macrocephaly. Pediatr Radiol. 1998;28:143.
Smith G.S., Koppel J., Goldberg S. Applications of neuroreceptor imaging to psychiatry research. Psychopharmacol Bull. 2003;37:26.
Sobel D.F., Aung M., Otsubo H., et al. Magnetoencephalography in children with Landau-Kleffner syndrome and acquired epileptic aphasia. Am J Neuroradiol. 2000;21:301.
Soetaert A.M., Lowe L.H., Formen C. Pediatric cranial Doppler sonography in children: non-sickle cell applications. Curr Probl Diagn Radiol. 2009;38(5):218-227.
Stadnik T.W., Chaskis C., Michotte A., et al. Diffusion-weighted MR imaging of intracerebral masses: Comparison with conventional MR imaging and histologic findings. Am J Neuroradiol. 2001;22:969.
Stagiannis K.D., Sepulveda W., Bower S. Early prenatal diagnosis of holoprosencephaly: The value of transvaginal ultrasonography. Eur J Obstet Gynecol Reprod Biol. 1995;61:175.
Stefan H., Hummel C., Hopfengartner R., et al. Magnetoencephalography in extratemporal epilepsy. J Clin Neurophysiol. 2000;17:190.
Strainer J.C., Ulmer J.L., Yerkin F.Z., et al. Functional MR of the primary auditory cortex: An analysis of pure tone activation and tone discrimination. Am J Neuroradiol. 1997;18:601.
Strauss K.J., Goske M.J., Kaste S.C., et al. Image gently: Ten steps you can take to optimize image quality and lower CT dose for pediatric patients. Am J Roentgenol. 2010;194(4):868-873.
Sugiyama K., Kondo T., Oouchida Y., et al. Clinical utility of diffuson tensor imaging for evaluating patients with diffuse axonal injury and cognitive disorders in the chronic stage. J Neurotrauma. 2009;26:1879.
Suh D.Y., Davis P.C., Hopkins K.L., et al. Nonaccidental pediatric head injury: Diffusion-weighted imaging findings. Neurosurgery. 2001;49:309.
Suhy J., Laxer K.D., Capizzano A.A., et al. 1H MRSI predicts surgical outcome in MRI-negative temporal lobe epilepsy. Neurology. 2002;58:821.
Sundaram S.K., Sivaswamy L., Makki M.I., et al. Absence of arcuate fasiculus in children with global developmental delay of unknown etiology: a diffusion tensor imaging study. J Pediatr. 2008;152:250.
Sutton L.N., Wang Z., Gusnard D., et al. Proton magnetic resonance spectroscopy of pediatric brain tumors. Neurosurgery. 1992;31:195.
Sutton L.N., Wang Z., Duhaime A.C., et al. Tissue lactate in pediatric head trauma. A clinical study using 1H-NMR spectroscopy. Pediatr Neurosurg. 1995;22:81.
Takashi Y., Hasuo K., Mihara F., et al. Increased activity of the ipsilateral motor cortex during a hand motor task in patients with brain tumor and paresis. Am J Neuroradiol. 1997;18:865.
Taniguchi M., Yoshimine T., Kato A., et al. Dysembryoplastic neuroepithelial tumor in the insular cortex. Three dimensional magnetoencephalographic localization of epileptic discharges. Neurol Res. 1998;20:433.
Tanner S.F., Cornette L., Ramenghi L.A., et al. Cerebral perfusion in infants and neonates: Preliminary results obtained using dynamic susceptibility contrast enhanced magnet resonance imaging. Arch Dis Child Fetal Neonatal Ed. 2003;88:F525.
Taoka T., Iwasaki S., Sakamoto M., et al. Diffusion anisotrophy and diffusivity of white matter tracts within the temporal stem in Alzheimer disease: evaluation of the “tract of interest” by diffusion tensor tractography. Am J Neuroradiol. 2006;27:1040.
Taoka T., Sakamoto M., Nakagawa H., et al. Diffusion tensor tractography of the Meyer loop in cases of temporal lobe resection for temporal lobe epilepsy: correlation between postsurgical visual field defect and anterior limit of Meyer loop on tractography. Am J Neurol. 2008;29:1329.
Tatsuno M., Haegawa M., Okuyama K. Ventriculitis in infants: Diagnosis by color Doppler flow imaging. Pediatr Neurol. 1993;9:127.
Taylor J.S., Ogg R.J., Langston J.W. Proton MR spectroscopy of pediatric brain tumors. Neuroimaging Clin N Am. 1998;8:753.
Teksam M., Casey S.O., Michel E., et al. Diffusion-weighted MR imaging findings in carbon monoxide poisoning. Neuroradiology. 2002;44:109.
Thiagarajah S., Henke J., Hogge W.A., et al. Early diagnosis of spina bifida: The value of cranial ultrasound markers. Obstet Gynecol. 1990;76:54.
Toma P.L., Rossi U.G. Paediatric ultrasound. II. Other applications. Eur Radiol. 2001;11:2369.
Tong K.A., Ashwal S.A., Holshouser B.A., et al. Hemorrhagic shearing lesions in children and adolescents with posttraumatic diffuse axonal injury: Improved detection and initial results. Radiology. 2003;227:332.
Tong K.A., Kido D., Ashwal S. Elucidating the Sturge-Weber pial angioma using noncontrast high-resolution susceptibility-weighted imaging: Visualization of deep venous shunting and possible increased oxygen extraction in the underlying brain: A case report. In: Proceedings of the American Society of Neuroradiology. Oak Brook, Ill: American Society of Neuroradiology; 2003:75.
Tong K.A., Ashwal S., Holshouser B.A., et al. Diffuse axonal injury in children: Clinical correlation with hemorrhagic lesions. Ann Neurol. 2004;56:36.
Topceuoglu M.A., Pryor J.C., Ogilvy C.S., et al. Cerebral vasospasm following subarachnoid hemorrhage. Curr Treat Options Cardiovasc Med. 2002;4:373.
Tortori-Donati, Rossi. Pediatric Neuroradiology. Berlin Heidelberg: Springer-Verlag; 2005.
Townsend D.W. Positron emission tomography/computed tomography. Semin Nucl Med. 2008;38(3):152-166. Review. PubMed PMID: 18396176
Tsacopoulos M., Magistretti P.J. Metabolic coupling between glia and neurons. J Neurosci. 1996;16:877.
Tunggal B., Hofmann K., Stoffel W. In vivo 13C nuclear magnetic resonance investigations of choline metabolism in rabbit brain. Magn Reson Med. 1990;13:90.
Tzika A.A., Vajapeyam S., Barnes P.D. Multivoxel proton MR spectroscopy and hemodynamic MR imaging of childhood brain tumors: Preliminary observations. Am J Neuroradiol. 1997;18:203.
Tzika A.A., Astrakas L.G., Zarifi M.K., et al. Spectroscopic and perfusion magnetic resonance imaging predictors of progression in pediatric brain tumors. Cancer. 2004;15:1246.
Uggetti C. Stroke in young people: Imaging. Neurol Sci. 2003;24(Suppl 1):S15.
Usenius T., Usenius J.P., Tenhunen M., et al. Radiation-induced changes in human brain metabolites as studied by 1H nuclear magnetic resonance spectroscopy in vivo. Int J Radiat Oncol Biol Phys. 1995;33:719.
van der Knapp M.S., van der Grond J., Luyen P.R., et al. 1H and 31P magnetic resonance spectroscopy of the brain in degenerative cerebral disorders. Ann Neurol. 1992;31:202.
van Wezel-Meijler G., Steggerda S.J., Leijser L.M. Cranial ultrasonography in neonates: role and limitations. Semin Perinatol. 2010;34:28.
Vargas M.I., Delavelle J., Jlassi H., et al. Clinical applications of diffusion tensor tractography of the spinal cord. Neuroradiology. 2008;50:25.
Wang Z., Sutton L.N., Cnaan A., et al. Proton MR spectroscopy of pediatric cerebellar tumors. Am J Neuroradiol. 1995;16:1821.
Wang Z., Zimmerman R.A., Sauter R., et al. spectroscopy of the brain: Clinically useful information obtained in assessing CNS diseases in children. Am J Roentgenol. 1996;167:191.
Warach S.. Diffusion and perfusion MRI: Functional brain imaging. Edelman R.R., Zlatkin M.B., Hesselink J.R., editors. Clinical magnetic resonance imaging, 1. Philadelphia: WB Saunders, 1996.
Warach S., Gaa J., Siewert B., et al. Acute human stroke studied by whole brain echoplanar diffusion-weighted magnetic resonance imaging. Ann Neurol. 1995;37:231.
Warach S., Dashe J.F., Edelman R.R. Clinical outcome in ischemic stroke predicted by early diffusion-weighted and perfusion magnetic resonance imaging: A preliminary analysis. J Cereb Blood Flow Metab. 1996;16:53.
Warren K.E. NMR spectroscopy and pediatric brain tumors. Oncologist. 2004;33:312.
Warren K.E., Frank J.A., Black J.L., et al. Proton magnetic resonance spectroscopic imaging in children with recurrent primary brain tumors. J Clin Oncol. 2000;18:1020.
Wegner E.A., Barrington S.F., Kingston J.E., et al. The impact of PET scanning on management of paediatric oncology patients. Eur J Nucl Med Mol Imaging. 2004;32:23.
Weiller C., Chollet F., Friston K.J., et al. Functional reorganization of the brain in recovery from striatocapsular infarction in man. Ann Neurol. 1992;31:463.
Wells R.G., Vetter C., Laud P. Intracranial hemorrhage in children younger than 3 years: Prediction of intent. Arch Pediatr Adolesc Med. 2002;156:252.
Wiznitzer M., Ruggieri P.M., Masaryk T.J., et al. Diagnosis of cerebrovascular disease in sickle cell anemia by magnetic resonance angiography. J Pediatr. 1990;117:551.
Woischneck D., Klein S., Reissberg S., et al. Prognosis of brain stem lesion in children with head injury. Childs Nerv Syst. 2003;19:174.
Wu J.S., Zhou L.F., Tang W.J., et al. Clinical evaluation and follow-up outcome of diffusion tensor imaging-based functional neuronavigation: a prospective, controlled study in patients with gliomas involving pyramidal tracts. Neurosurgery. 2007;61(5):935-948.
Wycliffe N.D., Skeen M.B. MR imaging findings in mitochondrial myopathy, encephalopathy with lactic acidosis, and stroke (MELAS) syndrome showing migratory pattern of cortical infarcts. In: Proceedings of the American Society of Neuroradiology (ASNR)/American Society of Head and Neck Radiology (ASHNR). Oak Brook, Ill: American Society of Neuroradiology; 1997:226.
Wycliffe N.D., Choe J., Holshouser B., et al. Reliability in detection of hemorrhage in acute stroke by a new three-dimensional gradient recalled echo susceptibility-weighted imaging technique compared with computer tomography: A retrospective study. J Magn Reson Imaging. 2004;20:372.
Yamada I., Suzuki S., Matsushima Y. Moyamoya disease: Diagnostic accuracy of MRI. Neuroradiology. 1995;37:356.
Yamada K., Mori S., Nakamura H., et al. Fiber-tracking method reveals sensorimotor pathway involvement in stroke patients. Stroke. 2003;34:159.
Yamada K., Sakai K., Akazawa K., et al. MR Tractography: A Review of its Clinical Applications. Magn Reson Med Sci. 2009;8:165.
Yamamoto T., Yamada K., Nishimura T., et al. Tractography to depict three layers of visual field trajectories to the calcarine gyri. Am J Opthalmol. 2005;140:781.
Yikilmaz A., Taylor G.A. Sonographic findings in bacterial meningitis in neonates and young infants. Pediatr Radiol. 2008;38:129.
Yousem D.M., Geckle R.J., Bilker W.B., et al. Posttraumatic olfactory dysfunction: MR and clinical evaluation. Am J Neuroradiol. 1996;17:1171.
Zarifi M.K., Astrakas L.G., Poussaint T.Y., et al. Prediction of adverse outcome with cerebral lactate level and apparent diffusion coefficient in infants with perinatal asphyxia. Radiology. 2002;225:859.
Zatorre R.J. Functional neuroimaging in the study of the human auditory cortex. Am J Neuroradiol. 1997;18:621.
Zatorre R.J., Evans A.C., Meyer E., et al. Lateralization of phonetic and pitch processing in speech perception. Science. 1992;256:846.
Zatorre R.J., Jones-Gotman M., Evans A.C., et al. Functional localization and lateralization of human olfactory cortex. Nature. 1992;360:339.
Zatorre R.J., Meyer E., Gjedde A., et al. PET studies of phonetic processing of speech: Review, replication, and re-analysis. Cereb Cortex. 1996;6:21.
Zeki S., Watson J.D.G., Lueck C.J., et al. A direct demonstration of functional specialization in human visual cortex. J Neurosci. 1991;11:641.
Zimmerman R.A. Pediatric cerebrovascular disease. JBR-BTR. 2000;83:245.
Zupanc M.L. Update on epilepsy in pediatric patients. Mayo Clin Proc. 1996;71:899.