Chapter 18 Neonatal Brain Injury
Although neonatal stroke and trauma are not uncommon, data about risk factors, causes, and outcomes are still scarce because of a paucity of population-based studies. However, emerging data are changing previous concepts regarding pathophysiology, especially for neonatal stroke. In the past, outcomes for these conditions have been primarily focused on the major neurologic residua of cerebral palsy (see Chapter 69). However, data from the Canadian Pediatric Stroke Registry and the German Childhood Stroke Study Group [deVeber et al., 2000; Kurnik et al., 2003] and from recent longitudinal studies [Westmacott et al., 2009a, b; Ballantyne et al., 2008; Ricci et al., 2008] suggest that cognitive deficits only become apparent during early childhood. This chapter reviews what is known regarding the incidence, causes, risk factors, and outcome of stroke and traumatic brain injury, with attention to the clinical presentations and methods of diagnosis and management after these conditions occur. Pathophysiology is discussed in more detail in Chapter 17 because hypoxic-ischemic injury and focal ischemia-reperfusion injury in the setting of stroke are thought to have common pathogenetic mechanisms. However, pathophysiology of trauma in the newborn period is relatively unstudied, and only broad assumptions can be made for these conditions.
Perinatal Arterial Ischemic Stroke
Definitions and Epidemiology
A recent National Institute of Neurological Disorders and Stroke workshop on perinatal stroke provided consensus recommendations on the definition and classification of ischemic perinatal stroke (Raju et al., 2007). Agreement about the following working definition was obtained during the workshop: “a group of heterogeneous conditions in which there is a focal disruption of cerebral blood flow secondary to arterial or cerebral venous thrombosis or embolization, occurring between 20 weeks of fetal life through the 28th postnatal day and confirmed by neuroimaging or neuropathology studies.” The two major subtypes, ischemic (including cerebral venous thrombosis) and hemorrhagic perinatal stroke, will be discussed separately. Time of onset can be considered as “late fetal” (28 weeks to birth), “perinatal” (28 weeks to 7 days after birth), “neonatal” (0–28 days after birth), and “presumed perinatal” (symptoms presenting beyond day 28 of life). This part of the chapter will focus on perinatal arterial ischemic stroke (PAIS).
Brain infarction due to occlusion of a major artery or one of its territorial branches is now recognized in an increasing number of full-term infants, with an incidence of approximately 1 per 2300–4000 live births, not dissimilar to that of stroke in the elderly [Estan and Hope, 1997; Nelson and Lynch, 2004; Schulzke et al., 2005; Schneider et al., 2004; Kirton and deVeber, 2009]. Data in preterm infants are scarce, but have recently been reported with an estimated incidence of 0.7 percent in a hospital-based case-control study [Benders et al., 2007].
Stroke in newborns is reported to have a male predominance [Chabrier et al., 2009], but this was not seen in a recent study from the International Pediatric Stroke Study (IPSS) registry data, of 249 infants aged less than 29 days with arterial ischemic stroke. Just over half of the patients (57 percent) were male, with a 1.3:1 male:female ratio, which is not significant [Golomb et al., 2009]. There is a tendency toward involvement of left-sided lesions within territories of the middle cerebral artery [Golomb et al., 2009; Trauner et al., 1993]. This is thought to be due to hemodynamic differences in cerebral blood flow between the right and left carotid arteries due to the hemodynamic effects of a patent ductus arteriosus or possibly due to preferential flow of placental emboli into the left-sided cerebral vessels.
Despite relatively low mortality (3–10 percent in the Canadian Stroke Register) [deVeber et al., 2000] and low recurrence rates (1.1–5 percent) [deVeber et al., 2000; Fullerton et al., 2007; Kurnik et al., 2003], there is a significant risk of developing adverse neurologic sequelae. Unilateral spastic cerebral palsy develops in about one-third of infants, and impairments in vision, cognition, language, and behavior are common [Lee et al., 2005; Westmacott et al., 2009].
The infant mortality rate in the United States for 1995–1998 due to stroke (ICD-9 CM 430–437) is reported to be 5.33 deaths per 100,000 per year, the perinatal mortality rate is 2.21 per 100,000, and the neonatal mortality rate is 3.49 per 100,000 live births per year [Lynch and Nelson, 2001]. The National Hospital Discharge Survey (1980–1998) determined that, for infants younger than 30 days of age, the hospital mortality rate for neonatal stroke is 10.1 percent, or 2.67 deaths per 100,000 live births [Lynch and Nelson, 2001; Lynch et al., 2002], which is similar to a more recent review, in which the infant death rate due to cerebrovascular disorders was 3 per 100,000 in the United States in 2005 [Kung et al., 2008].
Pathophysiology and Risk Factors
Many maternal, fetal, and neonatal risk factors are associated with neonatal arterial ischemic strokes, but evidence for true causation is lacking in most cases. Often, more than one potential risk factor can be identified (Figure 18-1).
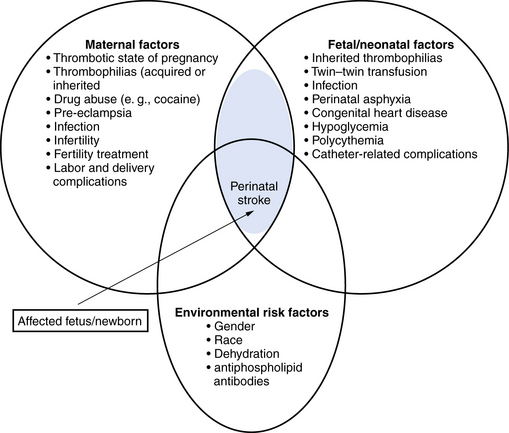
Fig. 18-1 Risk factors for perinatal arterial stroke.
(Adjusted from Raju TN, Nelson KB, Ferriero D et al. Ischemic perinatal stroke: summary of a workshop sponsored by the National Institute of Child Health and Human Development and the National Institute of Neurological Disorders and Stroke. Pediatrics 2007;120:609.)
Maternal Risk Factors
A history of infertility was identified as a risk factor in two recent studies (7–11 percent) [Lee et al., 2005; Curry et al., 2007]. An assumption is made that ovarian stimulation drugs would result in a hypercoagulable state, leading to placental thrombosis, but this still has to be confirmed with placental examination. Other maternal conditions, such as thyroid disease, diabetes mellitus, and gestational diabetes, were noted, but have not been identified as independent risk factors.
Antepartum Risk Factors
Pre-eclampsia was identified as a consistent independent risk factor in several studies (15–25 percent) [Lee et al., 2005; Curry et al., 2007]. This condition is known to affect uteroplacental blood flow. Oligohydramnios and decreased fetal movements have also been identified as risk factors, but both have no longer found to be significant in multiple regression analyses [Lee et al., 2005; Curry et al., 2007].
Intrapartum Risk Factors
While Ramaswamy et al. [2004] found only six cases with neonatal arterial ischemic stroke among 124 infants admitted with neonatal encephalopathy, the birth process tends to be reported as being ‘complicated’ and an intervention to deliver the infant is often required. Infants were not often severely depressed at birth, however, and were often well enough to stay with their mothers rather than in a neonatal intensive care unit. Factors that emerged after multivariate analysis were a prolonged second stage of labor (OR = 8.8; P = 0.001) and prolonged rupture of membranes (OR = 3.4; P = 0.05) [Lee et al., 2005]. More general signs of fetal distress (fetal heart-rate abnormalities, meconium-stained amniotic fluid, an Apgar score <7 at 5 minutes), cord abnormalities (tight nuchal cord), and need for intervention during delivery (instrumental delivery or need for emergent cesarean section) were more common in cases compared to controls, but were not identified as independent risk factors. A combination of three or more risk factors led to an odds ratio of 25.3. This implied a risk of 1 in 200 when three or more of the following risk factors were present: primiparity, infertility, oligohydramnios, pre-eclampsia, chorioamnionitis, prolonged rupture of membranes, decreased fetal movements, prolonged second stage of labour, and fetal heart-rate abnormalities [Lee et al., 2005].
Although the placenta is the most likely source of emboli to the fetal brain, data about the placenta are scarce, mainly because the placenta is often unavailable by the time the infant develops symptoms. Several placental abnormalities have been reported in infants with perinatal stroke, such as placental thrombosis, infarction, chorioamnionitis, funisitis, and placental chorioangiomas [Benders et al., 2007; Burke et al., 1997; Kraus et al., 1999; Elbers et al., 2011].
Postnatal Risk Factors
Prothrombotic risk factors
The peripartum period is a prothrombotic state in both the mother and fetus. Prothrombotic factors have been reported to play a possible role in the child, and more recently, it also has been shown that a prothrombotic factor is more often present in both mother and child [Simchen et al., 2009]. Gunther et al. [2000] performed a case-control study in 91 cases and showed that the percentage of prothrombotic risk factors was significantly higher in affected infants (68 percent compared to 24 percent; OR = 6,7, CI = 3,8–11,7). The most commonly found prothrombotic factors were elevated lipoprotein(a) and factor V Leiden, but hyperhomocysteinemia and mutations in the methyltetrahydrofolate reductase (MTHFR) gene also have been identified as associated factors. In two recent studies, high percentages of prothrombotic factors were found in both the neonate and the mother, as well as in mother–infant pairs, demonstrating the importance of also performing a thrombophilia screen in the mother [Curry et al., 2007; Simchen et al., 2009]. Simchen et al. [2009] noted a 8.5-fold increased risk for arterial ischemic stroke in a mother carrying factor V G1691A mutation (FVL), and a 2.1- and 3.8-fold increased risk in mothers with factor II G20210A mutation, or acquired antiphospholipid antibodies (APA) as compared to controls. In the study by Curry et al. [2007], a heterozygous or homozygous MTHFR C677T mutation was most common, seen in 41 of 60 neonates (68 percent). However, no significant differences between frequencies of prothrombotic coagulation defects were found in another recent cohort study [Miller et al., 2006].
Other neonatal risk factors
Arterial ischemic stroke is found in almost 40 percent of a series of infants preceding repair of congenital heart disease, and this is especially common in those infants who require atrial septostomy [McQuilllen et al., 2006]. In a more recent study, 10 percent of 122 patients with congenital heart disease were diagnosed with stroke, and in 6 patients the stroke occurred preoperatively. Stroke was clinically silent in 11 of the 12 infants [Chen et al., 2009]. Newborn infants undergoing extracorporeal membrane oxygenation are also at increased risk for developing arterial ischemic stroke (Wu et al., 2005).
Pais in the preterm infant
Independent risk factors identified in a case-control study of 31 preterm infants with stroke, using multivariate risk factor analysis, were different from risk factors identified in full-term infants. Twin-to-twin transfusion syndrome (TTTS) (OR = 31,2; 95 percent CI = 2.9–340.00), abnormal heart rate pattern (5,2; 1,5–17,6), and hypoglycaemia (<2 mmol/L) (3.9, 1,2–12,6) were independently associated with PAIS [Benders et al., 2007].
Perinatal Hemorrhagic Stroke
In a population-based cohort over a period of 10 years, a prevalence of 6.2 in 100,000 live births was observed for perinatal hemorrhagic stroke (PHS) [Armstrong-Wells et al., 2009]. A case-control study was performed with three controls per case. In contrast to what is known in infants with PAIS, all newborn infants with PHS presented with encephalopathy and more than half with seizures (65 percent). PHS was typically unifocal (74 percent) and unilateral (83 percent). Etiologies included thrombocytopenia (n = 4) and cavernous malformation (n = 1); 15 (75 percent) were idiopathic. Fetal distress and postmaturity were identified as independent predictors in a multivariate analysis.
Clinical Presentation and Diagnosis
PAIS in the newborn often manifests with seizures. The infants are initially well and have stayed with their mother on the ward, or can even be admitted from home. Whereas seizures associated with other forms of neonatal encephalopathy tend to be multifocal or myoclonic, seizures associated with arterial ischemic stroke tend to be persistently focal motor, usually involving only one upper extremity. Seizures tend to occur later than in neonates with hypoxic-ischemic encephalopathy (HIE) [Rafay et al., 2009]. Using multivariate analysis in 27 with PAIS and 35 with HIE, delayed seizure onset (≥12 hrs after birth) (p <0.0001; OR = 39.7; CI = 7.3, 217) and focal motor seizures (p = 0.007; OR = 13.4; 95 percent CI = 2.1,87.9) predicted stroke. Other more subtle symptoms observed in a large cohort of 215 newborn infants included poor feeding, hypotonia, and apnea [Kurnik et al., 2003; Redline et al., 2008].
In a population-based study of PAIS in children with motor impairment, most of the children presented after 3 months of life with hemiparesis (first recognized as early hand preference) or seizures [Wu et al., 2004]. These children are considered to have so-called “presumed perinatal ischemic stroke” (PPIS) [Kirton et al., 2008], which is diagnosed as a term (>36 weeks) infant older than 28 days with a normal neonatal neurologic history presenting with a neurological deficit or seizure referable to focal, chronic infarction on neuroimaging. Focal infarction specifies stroke (without limiting lesions to arterial territories) and includes unilateral/multiple/bilateral infarcts while excluding global injuries (HIE, watershed infarction, and periventricular leukomalacia [PVL]). Lesion chronicity is implied by imaging (restricted diffusion absent, encephalomalacia, gliosis, and atrophy of connected structures or Wallerian degeneration).
Infants with PPIS are less likely to have cortical involvement and more often show involvement of one or more of the lenticulostriate arteries. In a recent study, only 40 percent of late-diagnosed cases showed cortical involvement [Laugesaar et al., 2007]. In a large series of 59 cases with PPIS, venous periventricular venous infarction (PVI) was included in the spectrum of presumed perinatal stroke. Arterial proximal middle cerebral artery M1 segment infarction was most common (n = 19; 35 percent), and venous PVI was second (n = 12; 22 percent) and accounted for 75 percent of subcortical injuries [Kirton et al., 2008]. When risk factors are studied and outcome is reported, it is better to report children who are diagnosed in the neonatal period separately from those diagnosed later, referred to as PPIS.
Imaging
Cranial ultrasound studies (Box 18-1) may be normal if the stroke is superficial and ischemic, or they may reveal a wedge-shaped area of increased echogenicity with a linear demarcation line, usually within the territory of the middle cerebral artery. The echogenicity tends to become visible during the second half of the first week. Magnetic resonance imaging (MRI), including diffusion-weighted imaging (DWI), and MR angiography, is superior to head ultrasound, and should be performed in any newborn presenting with focal neonatal seizures.
Box 18-1 Evaluation of Neonates with Arterial Ischemic Stroke or Cerebral Sinovenous Thrombosis*
* There are few studies in this area, and there are no universally accepted guidelines.
† Normal levels (determined by antigen measurement and activity) for neonates are lower than for older children and adults. Levels may be temporarily low in the setting of acute illness; the significance of this is unclear.
(Data from Debus OM et al. The factor V G1691A mutation is a risk for porencephaly: A case-control study. Ann Neurol 2004;56:287; Manco-Johnson MJ, Grabowski EF, Hellgreen M et al. Laboratory testing for thrombophilia in pediatric patients. On behalf of the Subcommittee for Perinatal and Pediatric Thrombosis of the Scientific and Standardization Committee of the International Society of Thrombosis and Haemostasis [ISTH]. Thromb Haemost 2002;88:155–156.)
The role of DWI in the prediction of motor outcome was first shown by Mazumdar et al. [2003] and subsequently by others [de Vries et al., 2005; Kirton et al., 2007], showing restricted diffusion within the descending corticospinal tracts. Restricted diffusion at the level of the internal capsule and especially the middle part of the cerebral peduncle is now referred to as “pre-Wallerian degeneration,” as it is followed by Wallerian degeneration at 6–12 weeks and beyond (Figure 18.2).
A simple classification has been used, based on the primary artery involved [de Vries et al., 1997; Mercuri et al., 2004]. Infarcts in the territory of the middle cerebral artery were further subdivided into main branch, cortical branch, and lenticulostriate branch infarctions. Others have used a different classification, making a distinction between proximal M1 (PM1) of the middle cerebral artery that includes the lateral lenticulostriate (LLS) arteries, associated with infarction of the basal ganglia or distal M1 (DM1), in which compromised flow occurs distal to LLS and spares the basal ganglia while infarcting distal (cortical) middle cerebral artery territory. A third type of occlusion involves the anterior trunk of the middle cerebral artery, which is a superior middle cerebral artery division infarction that includes the frontal lobe (anterior-to-central sulcus) and the anterior temporal lobe (distal anterior trunk branch occlusions included). A fourth type involves the posterior trunk of the middle cerebral artery and is an inferior middle cerebral artery division infarction that includes parietal (posterior-to-central sulcus) and posterior temporal lobes (distal posterior trunk branch occlusions included). Finally, a fifth type, LLS infarcts, affects the basal ganglia (putamen and caudate body) and posterior limb of internal capsule (PLIC), with sparing of the subcortical white matter and cortex [Kirton et al., 2008]. Comparison of full-term and preterm infants showed that main branch involvement is rare in preterm infants, who tend to have LLS infarcts and less often show cortical involvement [Benders et al., 2009].
Newly developed techniques, such as diffusion tensor imaging (DTI), which allows quantification and visualization of white matter pathways in vivo, are more frequently being used in the evaluation of neonatal stroke [Lequin et al., 2009]. DTI characterizes the three-dimensional spatial distribution of water diffusion in each MRI voxel (see Chapter 11). Water diffuses preferentially along the direction of the axons and is restricted perpendicular to axons by myelin. This directional dependency is referred to as anisotropy. Directionality-encoded color maps (red–green–blue, RGB) or fiber tracking are commonly used. A fractional anisotropy (FA) map can show asymmetry of the PLIC as early as the neonatal period. In a study of 15 patients with congenital hemiparesis of different etiologies, studied at a median age of 2 years and compared with 17 age-matched controls, the clinical severity of hemiparesis was noted to correlate with asymmetry in FA (p <0.0001), transverse diffusivity (p <0.0001), and mean diffusivity (p <0.03) [Glenn et al., 2007]. Another promising technique is volumetric determination of stroke volumes, which was noted to predict motor outcome in animal studies [Ashwal et al., 2009]. Functional MRI (fMRI) tends to be used in childhood or adolescence to study reorganization of the sensorimotor cortex [Staudt et al., 2002; Seghier et al., 2005], but it was recently shown that passive unilateral sensorimotor stimulation is feasible, even in the preterm infant, resulting in bilateral activation of the sensorimotor cortex [Arichi et al., 2009; Heep et al., 2009].
Management/Treatment
Acute Period
For all types of perinatal stroke, supportive care with particular attention to monitoring of intracranial pressure is essential [Roach et al., 2008]. The use of continuous EEG monitoring or amplitude integrated EEG (aEEG) may be helpful for recognizing and treating neonatal seizures. Unilateral ictal discharges can be identified using two-channel aEEG, often suggesting a unilateral parenchymal lesion, usually PAIS or PHS before an MRI can be acquired [van Rooij et al., 2009]. Guidelines on the management and treatment of PAIS were published in 2008 [Roach et al., 2008; Monagle et al., 2008]. The American College of Chest Physicians (ACCP) only recommends administration of unfractionated heparin (UFH) or low molecular weight heparin (LMWH) in neonates with their first PAIS and an on-going documented cardioembolic source. Thrombolytic agents are not recommended.
Outcomes
Motor Effects
Arterial ischemic stroke may lead to permanent motor impairment or cerebral palsy. Estimates of motor impairment after neonatal arterial ischemic stroke range from 9 percent to 91 percent [Clancy et al., 1985; deVeber et al., 2000; de Vries et al., 1997; Golomb et al., 2008, Koelfen et al., 1995; Lee et al., 2005; Mercuri et al., 2004; Sran and Baumann, 1988; Trauner et al., 1993]. In a study by Lee et al. [2005], 58 percent of 40 children developed cerebral palsy and a delayed presentation was associated with increased risk for cerebral palsy (relative risk [RR], 2.2; 95 percent CI = 1.2–4.2). Cerebral palsy occurred in 68 percent of the largest cohort studied so far, and 87 percent of these children developed hemiplegia. This large cohort also consisted of infants with PAIS, with 47 percent developing cerebral palsy, as well as infants with PPIS, with 91 percent developing cerebral palsy. Most children with ischemic stroke diagnosed in the neonatal period attain independent walking, with the median time to taking first steps for the whole cohort, including infants with cerebral sinovenous thrombosis, being 13 months [Golomb et al., 2003]. In a study looking specifically at children with PPIS, motor outcomes (mean follow-up, 5.3 years) that were predicted by basal ganglia involvement included leg hemiparesis, spasticity, and need for assistive devices (p <0.01) [Kirton et al., 2008].
Sensory Deficits
Children with hemiplegic cerebral palsy are known to have sensory impairments but there are few detailed studies, in part because it is difficult to assess sensory function in young children [Cooper et al., 1995]. Thalamic atrophy has been seen in children with neonatal middle cerebral artery infarction, but whether this atrophy has long-term implications for sensory perception or memory is unclear [Giroud et al., 1995]. When children are carefully studied for visual field defects, hemianopia or quadrantanopia is not uncommon. Six (28 percent) of 16 school-age children with perinatal stroke had impaired visual function [Mercuri et al., 2003]. Children with unilateral pre- or perinatal stroke have more difficulty with facial recognition [Ballantyne and Trauner, 1999] and other visuospatial tasks [Schatz et al., 2000; Stiles et al., 1996, 1997].
Cognitive Effects
In some studies, most children with PAIS were noted to have intelligence within the normal range [Ricci et al., 2008; Trauner et al., 1993]. Cognitive impairment tends to be more common in the presence of hemiplegia and/or epilepsy. In a larger study of 46 infants, studied at a mean age of 42 months, cognitive impairment was present in 41 percent [Sreenan et al., 2000]. Cognitive outcome has only more recently been studied into childhood, showing more problems than intitially reported in small groups of infants studied early in life. Westmacott et al. [2009] studied 26 children with a history of acutely diagnosed unilateral PAIS as preschoolers (3 years 6 months to 5 years 11 months) and again as grade-school students (6 years 1 month to 12 years 5 months). While patients’ performance did not differ from the normative sample for full-scale IQ, verbal IQ, or performance IQ, and there were no significant differences associated with infarct laterality as preschoolers, performance was significantly lower than the normative sample for full-scale IQ working memory and processing speed, but not for verbal IQ or performance IQ at school age. Contrasts between preschool evaluation and grade-school evaluation revealed a significant decline in full-scale IQ, which reflected emerging deficits in nonverbal reasoning, working memory, and processing speed. Individual subject analyses revealed that 69 percent of the children showed significant declines in one or more IQ index measures. There were no significant differences in cognitive performance associated with lesion laterality, although males performed more poorly than females on several cognitive measures at grade school. These data are not in agreement with a study by Ballantyne et al. [2008], who found no evidence of a decline in cognitive function over time in children with perinatal unilateral brain damage, suggesting that there was sufficient on-going plasticity in the developing brain following early focal damage to result in the stability of cognitive functions over time. The time span between the two tests was shorter than in the study by Westmacott et al. [2009], and the time at first test was later, which could explain some of the differences. In another study by the same group, comparing effects of age at stroke and lesion location, it was found that the perinatal group performed more poorly than the childhood group, regardless of lesion location [Westmacott et al., 2009].
Language delay is not uncommon after perinatal stroke and was found in 25 percent of 36 survivors of the Kaiser Permanente study [Lee et al., 2005]; lesion laterality does not appear to predict degree of language impairment, but development of postneonatal epilepsy was a major contributor to language outcome [Ballantyne et al., 2008]. Above-average academic performance is, however, also possible in the setting of large middle cerebral artery strokes and hypsarrhythmia [Golomb et al., 2005]. In children with presumed perinatal stroke, nonmotor outcomes were associated with cortical involvement, including cognitive/behavioral outcomes, visual deficits, and epilepsy (p <0.01) [Kirton et al., 2008].
Epilepsy
Data about rates of childhood epilepsy after PAIS are still scarce. The longer the follow-up period, the greater the risk that there has been a recurrence of epilepsy following seizures seen in the neonatal period. During the first year there is a subset of children who will develop hypsarrhythmia, usually associated with cerebral palsy and cognitive impairment [Golomb et al., 2006]. Infants with hypsarrhythmia following “presumed perinatal stroke” appeared to have a better cognitive outcome. The highest rate of epilepsy after the neonatal period for neonates with arterial ischemic stroke reported is 67 percent, established in a group of 64 children, with resolution of their epilepsy in 32 percent [Golomb et al., 2007]. In a series of 46 children with a mean follow-up duration of 42.1 months (range 18–164 months), 46 percent developed epilepsy [Sreenan et al., 2000]. In a series of 67 preterm and full-term infants with PAIS, epilepsy occurred in 16 percent and was seen most often in children following main branch middle cerebral artery infarction (7/15, 47 percent) [Benders et al., 2009].
Recurrence
The recurrence rate after PAIS is low. The largest prospective study, which followed 215 neonates with arterial ischemic stroke for a median of 3.5 years, found that only 1.8 percent of children developed a recurrent stroke and 3.3 percent a recurrent symptomatic thromboembolism. Prothrombotic risk factors and the presence of additional morbidities, such as complex congenital heart disease or dehydration, were associated with an increased recurrence risk [Kurnik et al., 2003]. More recently a similar 5-year cumulative recurrence rate of 1.2 percent was reported (1 of 84 infants with PAIS) [Fullerton et al., 2007]. Also observed was the fact that children whose stroke occurred later in childhood had a 16-fold increased recurrence risk compared with the neonates (HR = 16; 95 percent CI = 2.1–120; p = 0.008).
Predictors of Outcomes
Vascular territory
The vascular territory that is infarcted aids in predicting outcome but is not highly accurate. One study of 24 children with perinatal stroke reported that concomitant involvement of cortex, basal ganglia, and internal capsule on MRI predicted hemiplegia more strongly than involvement of just one of these territories [Mercuri et al., 1999]. Two studies of initially 23 and subsequently 54 preterm and term neonates with middle cerebral artery ischemic stroke found that involvement of the main branch territory was predictive of hemiplegia [de Vries et al., 1997; Benders et al., 2009]. In a study of 62 children with neonatal arterial ischemic stroke, the presence of bilateral infarctions predicted a lower probability of walking [Golomb et al., 2003]. Another study found that large stroke size (RR = 2.0; 95 percent CI = 1.2–3.2) and injury to Broca’s area (RR = 2.5; 95 percent CI = 1.3–5.0), internal capsule (RR = 2.2; 95 percent CI = 1.1–4.4), Wernicke’s area (RR = 2.0; 95 percent CI = 1.1–3.8), or basal ganglia (RR = 1.9; 95 percent CI = 1.1–3.3) were predictors of hemiplegia [Lee et al., 2005]. Using DWI, “pre-Wallerian degeneration” can be recognized as restricted diffusion involving the descending corticospinal tracts. Restricted diffusion of the internal capsule and especially the cerebral peduncles is now considered to be the best and earliest predictor of hemiplegia [de Vries et al., 2005; Kirton et al., 2007]. In the small series of 14 infants studied by Kirton et al. [2007], percentage of peduncle being affected (p = 0.002), length of descending corticospinal tracts (p <0.001), and volume of descending corticospinal tracts (p = 0.002) were significantly associated with subsequent development of hemiplegia.
Neonatal seizures and early electroencephalogram
In one study of 46 neonates with arterial ischemic stroke, the presence of seizures in the neonatal period was predictive of one or more disabilities in the first years of life [Sreenan et al., 2000]. The presence of an abnormal background EEG pattern was a predictor of hemiplegia in another study [Mercuri et al., 1999].
Prothrombotic coagulation factors
Two studies showed an association between the presence of prothrombotic coagulation factors and neurological outcome. Among the 24 children studied by Mercuri et al. [2001], 9 developed hemiplegia and 8 had at least one prothrombotic coagulation factor (FVL or increased factor VIIIc), compared to only 2 (factor VIIIc) of the 13 with a normal outcome. In the study by Suppiej et al. [2008], inherited thrombophilia was significantly more common in patients with a poor neurologic outcome (Fisher’s exact test, p = 0.002), but this only applied to the children with PPIS.
Rehabilitation
As PAIS usually involves the middle cerebral artery, the upper extremity tends to be more severely affected than the lower extremity. Most children will be able to walk and first steps were taken at a mean age of 13 months, but this cohort consisted of children with PAIS (n = 62) or cerebral sinovenous thrombosis (n = 25) [Golomb et al., 2003]. Performing the assisting hand assessment (AHA) at 18 months can, to a certain degree, predict function of the assisting hand [Holmefur et al., 2009]. Until recently, treatment has been aimed at reducing spasticity and improving range of motion and function, using serial casting or botulinum toxin injections to reduce increased tone [Russo et al., 2007]. More recently, novel strategies have been used, such as constraint-induced movement therapy (CIMT), which involves restraining the unaffected arm to force use of the plegic arm, and this treatment strategy has been shown to be promising for improving function in hemiplegic cerebral palsy [Taub et al., 2004; Deluca et al., 2006]. In 4 out of 10 patients (age range 10–30 years) with hemiplegia following PAIS and preserved corticospinal projections from the affected hemisphere, increases in fMRI activation at the level of the primary sensorimotor cortex, as well as in the supplementary motor areas, were seen [Juenger et al., 2007]. In subsequent studies by the same group it was noted that improvement only occurred in patients with preserved crossed projections, with increased amplitude shown with transcranial magnetic stimulation (TMS) when stimulating the ipsilesional hemisphere [Kuhnke et al., 2008; Walther et al., 2009]. These studies, involving only small groups of children, do suggest that CIMT in PAIS with preserved crossed corticospinal projections induces neuroplastic changes in the primary motor cortex of the lesioned hemisphere, detected as increased cortical excitability (TMS) and increased task-related cortical activation (fMRI).
Neonatal Cerebral Sinovenous Thrombosis
Epidemiology
Population-based studies of neonatal cerebral sinovenous thrombosis (CSVT) are rare. Based on data from the Canadian Pediatric Stroke Registry, the incidence of CSVT in children is 0.67 per 100,000, and in neonates is 0.41 per 100,000 [deVeber et al., 2001]. This study included 69 neonates and is one of the larger studies in this population. A study from the Netherlands included 52 infants, admitted to five neonatal intensive care units [Berfelo et al., 2010]. The largest study to date, which was not population-based, included 84 neonates with symptomatic CSVT [Jordan et al., 2010]; 61 percent presented during the first week of life.
Pathophysiology and Risk Factors
The cerebral veins drain into the dural venous sinuses and this slowly flowing blood is presumably more susceptible to thrombosis. The dural venous sinuses are near suture lines and this location may result in trauma at the time of birth [Shroff et al., 2003]. Occlusion of venous sinuses results in cerebral venous congestion and persumably is the cause of brain injury [Adams, 2007]. Increased venous pressure may cause cerebral edema, venous infarction with or without hemorrhagic conversion, and intraventricular hemorrhage [Wu et al., 2002]. Wu et al. first pointed out that CSVT should always be considered in the presence of an intraventricular hemorrhage (IVH) occurring at term, especially when this is associated with a unilateral thalamic hemorrhage. Thirty-one percent of 29 infants born > 36 weeks’ gestation, who were diagnosed with CSVT, presented with an IVH. Thalamic hemorrhage was diagnosed in 16 percent of infants with CSVT [Wu et al., 2002]. Intracranial hypertension is often present due to venous congestion and to impaired cerebrospinal fluid reabsorption through the arachnoid granulations that are within the venous sinuses [Adams, 2007].
Risk factors for CSVT during the first 28 days of life include dehydration, infection, maternal fever/chorioamnionitis, hypoxic-ischemic injury, and thrombophilia [Fitzgerald et al., 2006; Kenet et al., 2007]. Often, neonates have more than one risk factor [Wu et al., 2002].
Clinical Presentation and Diagnosis
Seizures are the most common presentation in a neonate, followed by nonspecific signs such as lethargy, hypotonia, feeding difficulties, respiratory distress, and apnea [deVeber et al., 2001; Fitzgerald et al., 2006; Wasay et al., 2008]. Focal deficits are present in very few neonates: 6 percent in two different studies [deVeber et al., 2001; Jordan et al., 2010]. With an extensive CSVT, neonates will present with tense or bulging fontanels, splaying of the cranial sutures, and prominent scalp veins. These are signs of poor cerebral venous drainage and increased intracranial pressure.
Cranial ultrasound may detect CSVT, particularly in the presence of a midline thrombus in the superior sagittal sinus, or a unilateral thalamic hemorrhage. Power Doppler may be superior to color Doppler when available [Govaert et al., 1992a; Tsao et al., 1999]. Additional imaging is required to exclude CSVT in more peripheral locations and to confirm the extent of the thrombus. Unenhanced CT may detect a thrombus, and contrast-enhanced CT may show the “empty delta” sign which is a filling defect in the posterior portion of the superior sagittal sinus due to thrombus. Radiologically based false-positive or missed diagnoses have been reported in up to 40 percent of children with CSVT [Davies and Slavotinek, 1994]. MRI of the brain and MR or computed tomography (CT) venography are needed to confirm the diagnosis [Eichler et al., 2007] (Figure 18-3 and Figure 18-4). Susceptibility-weighted imaging (SWI) has recently been reported as another useful sequence in confirming the presence of CSVT and for following to establish progression or resolution [Takekawa et al., 2008; Kawabori et al., 2009].
Management/Treatment
Published consensus-based guidelines for antithrombotic treatment of neonatal CSVT are not in agreement. The American College of Chest Physicians guidelines, published in 2004 [Monagle et al., 2004] and updated in 2008 [Monagle et al., 2008], suggest anticoagulation for neonates without significant intracranial hemorrhage, while the American Heart Association (AHA) guidelines, published in 2008 [Roach et al., 2008], recommend anticoagulation only when there is evidence of thrombus propagation, multiple cerebral or systemic emboli, or a severe prothrombotic state. From the Canadian Pediatric Ischemic Stroke Registry, only 25 of 69 neonates (36 percent) with CSVT, diagnosed between 1992 and 1998, were anticoagulated [deVeber et al., 2001]. Most treated neonates received low molecular weight heparin (LMWH; 20 of 25). None had hemorrhagic complications associated with death or neurologic deterioration. Hemorrhagic infarction was reported in 24 of 69 neonates (35 percent), while ischemic infarction was reported in 5 of 69 (7 percent). Similar data were obtained in the Netherlands, where 23 of 52 infants received anticoagulation without any hemorrhagic complications [Berfelo et al., 2010]. In a European collaborative study of childhood CSVT, 75 neonates were included [Kenet et al., 2007]. Specific data for neonates were not reported in detail, but there was no significant difference in treatment for children younger versus those older than age 2 years. If anticoagulation is deferred in a neonate with a CSVT, then follow-up neuroimaging should be performed after 5–7 days of supportive care to assess for thrombus propagation. If anticoagulation is used, the duration of therapy is typically 6 weeks to 3 months [Shroff et al., 2003; Moharir et al., 2010].
A recent small series (n = 10) of neonates with CSVT and intracerebral hemorrhage (unilateral thalamic hemorrhage) showed that 7 out of 10 neonates who were treated with anticoagulation did not show an increase of the thalamic hemorrhage [Kersbergen et al., 2009]. There are insufficient data to recommend thrombolysis; however, there are case reports of thrombolysis in the setting of progressive CSVT despite anticoagulation [Wasay et al., 2006; Wong et al., 1987].
Outcomes
There is significant risk of adverse outcome. There are few studies with detailed long-term outcomes, but even in younger children, motor and cognitive impairments, as well as epilepsy, are frequent. In series of 42 neonates with CSVT, only 6 of 29 (21 percent) children available for follow-up were neurologically normal at a median age of 2 years. The others had some impairment, such as persistent seizures or motor or cognitive problems [Fitzgerald et al., 2006]. Other studies have reported developmental delays or neurologic deficits in 35–50 percent of individuals [Carvalaho et al., 2001; Jordan et al., 2010]. Not surprisingly, the presence of cerebral venous infarction is one predictor of poor outcome, particularly when infarction is bilateral [deVeber et al., 2001; Golomb et al., 2003].
Data on recurrence risk after neonatal CSVT are limited. In the Canadian Pediatric Stroke Registry, 5 of 69 (7 percent) neonates had a recurrent systemic or cerebral thrombosis at a mean follow-up time of 1.6 years [deVeber et al., 2001].
Motor Effects
Hemiparesis, cerebral palsy, and nonspecific motor effects have all been reported after neonatal CSVT, with estimates ranging from 6 to 67 percent [deVeber et al., 2000, 2001; Fitzgerald et al., 2006]. The median length of follow-up in these studies ranged from 1.6 to 2 years. Most children with a history of perinatal ischemic stroke (including CSVT) eventually walk. In a Canadian cohort of 88 term or near-term neonates with CSVT or arterial ischemic stroke, 100 percent of those with CSVT without parenchymal infarction walked [Golomb et al., 2003]. Of those with infarction (due to arterial ischemic stroke or CSVT), 95 percent with unilateral infarcts and 67 percent with bilateral infarcts walked. The median age of first steps for the cohort was 13 months.
Cognitive Effects
Many studies combined PAIS and CSVT when looking at outcomes. In one study that focused specifically on CSVT, 1 of 19 neonates had a learning disability, and generalized developmental delays were reported in up to 58 percent; the median length of follow-up was 5 years [Carvalho et al., 2001].
Perinatal Birth Trauma
Perinatal traumatic injuries were first reported almost 2 centuries ago. In 1819, the occurrence of subgaleal hematoma was described by Naegele [Amar et al., 2003]. Salmonsen described cranial birth injuries after normal spontaneous vaginal deliveries in 1928 [Pollina et al., 2001]. Perinatal spinal cord injury was reported as early as 1869, when Parrot described an infant with difficult delivery requiring significant traction to the head, which resulted in rupture of the spinal cord at C6–7. In the 1920s, several classic articles on spinal cord injury were published [Blount et al., 2004; Filippigh et al., 1994; Rossitch and Oakes, 1992]. With recognition of the risk of significant perinatal central nervous system (CNS) injuries and improvements in obstetrical techniques, the incidence of such injuries has decreased but remains a significant cause of neonatal morbidity and mortality.
Definition and Epidemiology
Although cranial birth injuries in newborn infants are rare, they are potentially life-threatening. Major birth trauma, defined as “any condition that affects the fetus adversely during delivery” [Hughes et al., 1999], occurs in approximately 3.2 percent of births. Mechanical forces and hypoxia can contribute to birth injury. Mechanical trauma can result in damage to the extracranial, cranial, or intracranial structures; spinal cord; or peripheral nerves. Most birth injuries are clavicular fractures, accounting for more than 94 percent of injuries [Medlock and Hanigan, 1997]. However, 2 percent of all neonatal deaths and 10 percent of all neonatal deaths of term infants are the result of birth injury [Hughes et al., 1999; Pollina et al., 2001]. Injury-related neonatal death can result from intracranial hemorrhage, massive blood loss as a result of subgaleal hemorrhage, or cervical spinal cord injury.
Risk Factors
A variety of factors predisposing infants to birth trauma have been reported. These include fetal macrosomia, breech or footling presentation, shoulder dystocia, prolonged labor, primiparity, and forceps- or vacuum-assisted delivery [Hughes et al., 1999; Medlock and Hanigan, 1997; Perrin et al., 1997; Pollina et al., 2001]. There also have been reports of significant perinatal injury, even in the setting of seemingly uncomplicated delivery [Chamnanvanakij et al., 2002; Medlock and Hanigan, 1997; Pollina et al., 2001]. During the normal birth process, the fetal head is subjected to significant intrauterine pressure, ranging from 38 to 390 mmHg, with an average of 158 mmHg [Medlock and Hanigan, 1997]. These pressures can result in overriding sutures, which may cause direct cerebellar trauma, and rupture or tearing of large venous channels, bridging veins, or tentorial leaflets [Chamnanvanakij et al., 2002; Hayashi et al., 1987; Perrin et al., 1997]. Traction and torsion injuries may result in cervical spinal cord and brachial plexus injury [Filippigh et al., 1994; Menticoglou et al., 1995; Rehan and Seshia, 1993; van Ouwerkerk et al., 2000].
There is controversy about the effect of mode of delivery on the incidence of significant neonatal injury. In a large study of 583,340 live-born singleton infants born to nulliparous women and weighing between 2500 and 4000 g, Towner et al. [1999] found that the incidence of neonatal intracranial injury (including subdural, intracerebral, intraventricular, and subarachnoid hemorrhage) was significantly higher with vacuum extraction, forceps delivery, and cesarean delivery during labor (odds ratios of 1.9–3.4), compared with spontaneous vaginal delivery or cesarean section with no labor. The investigators concluded that the increased risk of injury resulted from abnormal labor requiring intervention, rather than vacuum extraction or forceps delivery [Towner et al., 1999]. In another study, Pollina et al. [2001] conducted a retrospective chart review of 41 children with cranial birth injuries (i.e., skull fracture, epidural or subdural subarachnoid intracerebral intraventricular hemorrhage, or intraparenchymal brain injury) over a 9-year period. They found that birth weight, Apgar scores, and mode of delivery were independently associated with cranial injury. However, in contrast with the previous study, these investigators found that the incidence of cranial trauma was only slightly higher with delivery by cesarean section compared with spontaneous vaginal delivery, whereas it was markedly higher for infants delivered by means of forceps- or vacuum-assisted delivery [Pollina et al., 2001].
Clinical Presentation and Management by Diagnosis
The clinical presentation of neonatal traumatic CNS injury varies according to the type of injury. Most commonly, cranial birth injuries manifest with apnea and seizures [Pollina et al., 2001]. Clinical presentations of the different types of injuries are discussed in more detail in later sections. A neonate presenting with signs and symptoms of cranial birth injury should be evaluated for anemia, thrombocytopenia, and coagulopathy. Laboratory tests should include complete blood count, prothrombin time, partial thromboplastin time, fibrinogen, and blood typing in anticipation of possible transfusion in more severe cases. Because of the difficulty in distinguishing between neonatal sepsis and cranial trauma on presentation, blood and urine cultures and a lumbar puncture are often performed during the initial evaluation [Chamnanvanakij et al., 2002]. Results of these initial evaluations usually reveal predisposing factors, such as vitamin K deficiency, hemophilia, thrombocytopenia, sepsis, or disseminated intravascular coagulopathy [Haase et al., 2003].
Extracranial Injury
Cephalohematoma is the term for hemorrhage into the subperiosteum as a result of separation of the pericranium from the skull during birth. It does not cross suture lines and does not transilluminate (in contrast to caput succedaneum). The incidence ranges from 0.4 to 2.49 percent of live births [Hughes et al., 1999]. Ultrasound may distinguish between cephalohematomas and subgaleal hematomas, and detect coexisting intracranial hemorrhage. Spontaneous resolution usually occurs, with calcification appearing as a rim of curvilinear new bone that resorbs within 2–8 weeks (Figure 18-5). Rarely, a cephalohematoma may become infected [King and Boothroyd, 1998].
Skull fracture is associated with cephalohematoma in up to 25 percent of neonates [Hughes et al., 1999], and skull radiography or CT is indicated when a fracture is suspected. Significant cephalohematoma may result in symptomatic anemia or hyperbilirubinemia, or both. Aspiration of a cephalohematoma is indicated only in the presence of increasing size, increasing erythema, or suspected infection [Medlock and Hanigan, 1997].
Neonates with subgaleal hematomas present initially with fluctuant scalp swelling that may progress to skin discoloration of the frontal and suboccipital areas, and obscuration of the fontanel. In contrast to cephalohematoma, subgaleal hematomas may cross suture lines and extend to the nape of the neck. Most subgaleal hematomas are benign and do not require treatment [Medlock and Hanigan, 1997]. If the hematoma is large enough to cause cerebral injury, irritability, lethargy, poor feeding, and other signs of CNS depression may ensue [Amar et al., 2003]. A rough estimate of blood loss can be made by equating a 1-cm increase in head circumference to a 40-mL blood loss. In severe cases, it is a potentially fatal condition. Diffuse swelling of the head occurs and there is evidence of hypovolemic shock; mortality rate is estimated at 20–25 percent. Subgaleal hemorrhages are caused by rupture of the emissary veins, which are connections between the dural sinuses and the scalp veins. Blood accumulates between the epicranial aponeurosis of the scalp and the periosteum. Most cases of subgaleal hemorrhage reported have been associated with use of a vacuum extractor. The incidence of subgaleal hemorrhage is estimated to occur in 4–6 of 10,000 spontaneous vaginal deliveries and in 46–59 of 10,000 vacuum-assisted deliveries [Chadwick et al., 1996; Uchil et al., 2003]. In a study by Kilani et al. [2006], associated intracranial hemorrhage was present in half of the 34 infants studied, and four (11.8 percent) of infants died. In yet another study, 31 percent had a poor outcome (five died, four had epilepsy, three with severe auditory dysfunction, two with cerebral palsy, and one with renal vein thrombosis) [Chang et al., 2007]. The group with the poor outcome had significantly more patients who had been transferred from other hospitals (p <0.001). Those with a poor outcome had significantly more hypotension (p <0.001) and seizures (p <0.05). Other reported risk factors are vacuum extraction, prematurity, male gender, African lineage, primiparity, macrosomia, positional dystocia, and hypoxia [Amar et al., 2003]. Prompt and aggressive administration of blood products and treatment of associated coagulopathy are recommended to improve outcome.
Cranial Injuries
Skull fractures should be suspected in any neonate with a cephalohematoma or subarachnoid hemorrhage. Skull fractures may be linear, most commonly involving the parietal bone, or depressed, resulting in the so-called “ping-pong” fracture. Although fractures due to fetal compression against the bones surrounding the birth canal may occur during normal delivery, they are often associated with application of forceps or other assisted deliveries [Hughes et al., 1999; Medlock and Hanigan, 1997; Papaefthymiou et al., 1996]. Isolated depressed skull fractures can be diagnosed on physical examination as a shallow depression, usually in the parietal region [Tavarez et al., 1989]. The presence of a concomitant caput, cephalohematoma, or subgaleal hematoma may mask the fracture, which can be diagnosed by radiographic imaging.
Depressed skull fractures should prompt neurosurgical evaluation, although not all fractures require elevation [Medlock and Hanigan, 1997; Tavarez et al., 1989]. Surgical intervention is indicated in the setting of associated complications, such as increased intracranial pressure, cortical compression, or underlying hematoma [Tavarez et al., 1989]. Other complications of depressed skull fractures include sagittal sinus thrombosis associated with an occipital depressed skull fracture [Medlock and Hanigan, 1997], osteodiastasis (i.e., separation between the temporal squama and occipital bone at the lambdoid suture) associated with breech presentation and significant neck hyperextension [Pollina et al., 2001], and “growing skull fracture,” or leptomeningeal cyst. This latter entity occurs as a result of a dural tear allowing free communication of the subarachnoid space and herniation of the meninges through the bony defect accompanied by rapid growth of the brain. Neurologic symptoms, such as seizures, behavioral disturbances, and motor weakness, may appear months to years after the injury. Some investigators advocate close follow-up of conservatively managed fractures with serial radiographs and CT [Papaefthymiou et al., 1996]. Ultrasound and MRI are also useful for evaluation of a leptomeningeal cyst [King and Boothroyd, 1998].
Intracranial Injuries
Pathogenesis
The mechanisms by which cell death occurs following traumatic brain injury, at the cellular level, are in some ways similar to those processes occurring after an hypoxic-ischemic event in the immature brain and are referred to in greater detail in Chapter 17. Direct impact to the developing brain as a result of trauma, the release of blood, and contusive disruption of cell membranes leads to the release of excitatory amino acids and calcium, and inflammatory responses that characterize those processes, leading to cell death along the necrotic/apoptotic continuum [Lea and Faden, 2001].
Each of these responses to injury is influenced significantly by the effects of age and maturational capacity of the developing nervous system. In this regard, it is known that the nervous system of the newborn infant has a reduced capacity for glutamate clearance from the synaptic cleft due to the decreased expression of glutamate transporters, near-adult levels of NMDA receptors, and increased permeability to calcium influx [Statler, 2006].
In addition, the immature brain is less able to quench free radicals. Traumatic brain injury, resulting in tissue disruption, results in the release of transition metals, such as iron and heme, originating from the degradation of intracellular and extracellular heme proteins. Iron is in very high concentrations in human developing oligodendroglia and is a huge source for the production of free radicals [Hussain and Juurlink, 1995; Thorburne and Juurlink, 1996]. Metallothioneins are among a number of proteins that reduce redox activity of transition metals; however, they are inadequately expressed in the developing brain, compared to adults. Similarly, the breakdown of heme is catalyzed by heme oxygenases (HO) found in neurons and glia, but in lower concentrations in the newborn than in the adult. Indeed, essentially all of the endogenous free radical scavenging proteins, including superoxide dismutase and glutathione reductase, are in reduced concentrations in the newborn compared to the adult brain [Bavir et al., 2006; Robertson et al., 2006].
Enhanced exposure to the putative amino acids, increased permeability to calcium influx, and the reduced capacity to scavenge free radicals, likely make the newborn brain more sensitive to the damaging effects of trauma than the adult brain, although this remains controversial. Additional potential contributing factors include the rapid and extensive brain swelling and edema that is seen in the younger infant, compared to the adult [Duhaime and Durham, 2007; Vannucci et al., 1993]. Although the cause for these age-related changes remains unknown, several theories have been proposed. Studies point to the relatively compliant skull and membranous suture properties of the newborn skull that are associated with an increased likelihood of brain distortion. Other studies have suggested that the blood–brain barrier is more permeable in the immature compared to the adult brain after injury [Grundl et al., 1994], and that inflammatory responses and diffusion of excitotoxic neurotransmitter release are greater in the developing brain [van Lookeren Campagne et al., 1994]. Increased brain swelling in newborns can cause secondary injury due to patchy cerebral hypoperfusion and surrounding ischemia, presumably augmenting the region of vulnerability. Duhaime et al. [2007] describe the MRI appearance of the “big black brain” in infants following nonaccidental traumatic injury as being due to excessive edema formation seen with subdural hemorrhages in contrast to the adult. This was thought to be secondary to a combination of clinical events, including apnea, seizures, and patchy areas of ischemia. Several pathologic studies have pointed to an increased number of “ischemic” changes in these postmortem brains that may contribute to the production of cell swelling [Geddes et al., 2001a; Geddes et al., 2001b].
The question as to whether the immature brain is more sensitive or resistant to injury compared to the adult continues to be debated [Kochanek, 2006]. The developing brain may well be more sensitive to injury, simply based on the immature nature of its endogenous protective mechanisms. Pragmatically, several laboratories have shown that the immature brain is more sensitive to injury. Following a focal contusion in newborn rats between 3 (preterm human equivalent) and 30 (juvenile human equivalent) postnatal days, investigators found widespread cell death which first appeared at 6 hours post injury, peaked at 24 hours, and subsided by 5 days after the injury. The extent of injury, which was uniformly apoptotic in nature, peaked at 3–7 postnatal days (term newborn human equivalent), and subsequently diminished [Bittigau et al., 1999; Pohl et al., 1999]. The authors concluded that the prognosis for traumatic brain injury is age-dependent, with the newborn being most sensitive. Similar findings were shown in an excitotoxic model of cell death. McDonald et al. [1988, 1992] injected NMDA into the brains of rat pups aged 7–42 postnatal days, and found that the volume of injury in the younger, immature rat was 21 times greater than that seen in their adult counterparts. Kolb et al. (1987, 2003) found that, after surgically removing sections of the frontal lobe in rats of varying ages, both neurogenesis and functional recovery were age-dependent. Therefore, 1-day-old rats (very preterm human equivalent) had more extensive behavioral impairments than any of the other older groups of animals. Ten-day-old rats (term human equivalent) showed the greatest degree of behavioral sparing’ and adult animals were less affected. Yager et al. (1996, 1997) showed that the newborn rat brain was more sensitive to injury following hypoxia-ischemia than older rats given the same insult. However, during recovery, younger rats showed a greater degree of behavioral functional recovery than did the adult animals, suggesting a greater degree of plasticity in the younger rats [Yager et al., 2005, 2006; Saucier et al., 2007].
Pathophysiologically, there is substantive evidence to suggest that the newborn brain, following traumatic injury, suffers the consequences of profound edema, leading to secondary ischemic injury and early extensive apoptotic cell death. The immature brain also appears to have a greater capacity for plasticity and potential recovery than the adult’s. However, these relationships are clearly not fully elucidated; nor do they behave in a linear fashion [Giza and Prins, 2006]. Much research needs to be done before we fully understand the effect of traumatic brain injury in the newborn and how age influences outcomes and approaches to therapy.
Recent literature has further elucidated biomarkers of injury that may be used as surrogates for outcome determination and indicators for therapy. Berger et al. (2007) have determined serum levels of neuron-specific enolase (NSE), S100B, and myelin basic protein in groups of children following hypoxic-ischemic injury, inflicted traumatic brain injury (iTBI), and noninflicted traumatic brain injury (nTBI). This group found significant correlation between the three markers and outcome at 6 months of age [Beers et al., 2007], and between iTBI and hypoxic-ischemic insults [Berger et al., 2007]. Similarly, new neuroimaging techniques involving SWI, MR spectroscopy (MRS), and DTI are being used to determine the extent and diversity of early injury as markers of blood deposition (SWI) with greater sensitivity, metabolic perturbations (MRS), and long-term plasticity (DTI). These techniques, although being used in older children at present, have great capacity for use in the newborn [Ashwal et al., 2004a, b, 2006a, b].
Epidural hematomas
Epidural hematomas in the neonate occur only rarely, even in the setting of intracranial hemorrhage [Park et al., 2006]. In one postmortem examination series, only 2 percent of neonatal intracranial hemorrhages were epidural in location. In a series of 41 patients with cranial injuries monitored over an 8-year period, no epidural hematomas were reported. The investigators postulated that this was because most epidural hematomas are asymptomatic and therefore unrecognized [Pollina et al., 2001]. Moreover, the bleeding is venous, resulting in the slow development of a hematoma and less dramatic presentation. Others have postulated that, because the dura mater does not separate from the skull as easily in neonates and the middle meningeal artery has not yet developed, neonates are at lower risk for epidural hematomas. Unlike epidural hematomas in older children, associated skull fractures are often absent [Park et al., 2006].
Clinical presentation can include increasing head circumference, altered level of consciousness, or focal neurologic signs. Evaluation consists of obtaining a complete blood count, coagulation studies, and head CT or MRI (Figure 18-6). Neurosurgical evacuation of symptomatic epidural hematoma is required by craniotomy or by ultrasound-guided needle aspiration [Vachharajani and Mathur, 2002]. Neonates with isolated epidural hematomas have a good prognosis. In a review of several case series, Akiyama et al. [2001] found that, of 18 children with neonatal epidural hematomas, 5 (28 percent) died, and 3 of those who died had associated injuries, including subdural hematoma, intraventricular hemorrhage, or subarachnoid hemorrhage. The survivors all had good outcomes, with the exception of one child who had psychomotor delay. However, in this report, the length of follow-up and method of developmental assessment were not enumerated.
Subdural hematomas
Subdural hematomas are the most common intracranial birth injury, accounting for 73 percent of neonatal intracranial hemorrhage [Pollina et al., 2001]. In a study of 583,340 live births of singleton newborns weighing between 2500 and 4000 g and excluding breech presentation, the incidence of subdural or cerebral hemorrhage was 2.9 per 10,000 for spontaneous vaginal delivery, 4.1 per 10,000 for cesarean section without labor, 21.3 per 10,000 with combined vacuum- and forceps-assisted delivery, and 25.7 per 10,000 for cesarean section after failed vaginal delivery [Towner et al., 1999]. Antenatal subdural hematomas and subdural hematomas after normal spontaneous vaginal delivery have been described [Chamnanvanakij et al., 2002]. While a significant subdural hemorrhage is most often related to birth trauma, a small subdural was noted to be common when MRI was performed routinely in 111 consecutive, asymptomatic full-term infants [Whitby et al., 2004]. Nine infants had a subdural hemorrhage: three following a normal vaginal delivery (6.1 percent) and five following a forceps after an attempted ventouse delivery (27.8 percent), and only one after a traumatic ventouse (i.e., vacuum device) delivery (7.7 percent). A similar incidence of a 26 percent prevalence rate for intracranial hemorrhage was determined in 88 newborns following vaginal births. However, in this group, no association was seen with traumatic or assisted birth compared to an uncomplicated vaginal birth. All newborns were asymptomatic [Looney et al., 2007]. Risk factors include primiparity, vacuum- or forceps-assisted delivery, traumatic or breech delivery, asphyxia, shock, and coagulopathy [Chamnanvanakij et al., 2002; Haase et al., 2003; Hayashi et al., 1987]. Occipital diastasis can occur during a vaginal breech delivery with excessive extension of the newborn’s neck. Vaginal breech deliveries are not commonly performed as a consequence of results of multicenter, randomized studies [Hofmeyr et al., 2003].
Neonatal subdural hematomas are thought to result from tearing of the falx and tentorium or bridging cortical veins due to stretching associated with labor. Hemorrhage is therefore most commonly seen in tentorial or interhemispheric areas [Haase et al., 2003; Perrin et al., 1997; Pollina et al., 2001]. Intraventricular, subarachnoid, or intraparenchymal hemorrhage may occur [Chamnanvanakij et al., 2002; Medlock and Hanigan, 1997]. Secondary cerebral infarction has also been reported and has been related to prolonged arterial compression [Govaert et al., 1992b]. The majority of the subdural hemorrhages are infratentorial (Figure 18-7), but a supratentorial location can also be noted and is sometimes associated with a lobar hemorrhage, which can be large and associated with a midline shift, requiring neurosurgical intervention.
Clinical presentation may vary from asymptomatic to severe mass effect with neurologic symptoms. When symptomatic, patients may present with irritability, poor feeding, apnea, seizures, or bradycardia [Perrin et al., 1997]. In one series of 26 infants with subdural hematoma, 60 percent had respiratory symptoms (e.g., cyanotic episodes, apnea), and the remainder had neurologic symptoms, including seizures, hypotonia, or both [Chamnanvanakij et al., 2002]. An overlying cephalohematoma, subgaleal hematoma, or skull fracture may be present. Other birth injuries, such as a brachial plexopathy (e.g., Erb’s palsy) or clavicular fracture, may be present and are indicative of a difficult labor and delivery [Perrin et al., 1997]. Rarely, an infant with a subdural hematoma may present with signs of impending herniation or cerebral infarction [Govaert et al., 1992b; Haase et al., 2003; Perrin et al., 1997].
Appropriate laboratory evaluation includes a complete blood count and coagulation profile. Subdural hematomas may be diagnosed by head ultrasound, but definitive diagnosis, including the presence of intracerebral hemorrhage, cerebral edema, hydrocephalus, or cerebral infarction, requires CT or MRI [Chamnanvanakij et al., 2002; Hayashi et al., 1987; Perrin et al., 1997]. MRI angiography or cerebral angiography may be considered as secondary imaging studies [Perrin et al., 1997]. MRI provides superior imaging of tentorium tears, as well as better delineation of posterior fossa injury [King and Boothroyd, 1998].
Management is generally supportive, with maintenance of circulating blood volume, correction of significant anemia and coagulopathy, and treatment of seizures. Most often, neurosurgical intervention is unnecessary. If there are signs of transtentorial herniation, percutaneous needle aspiration of the subdural hematoma may be attempted, which was successful in 5 out of 7 neonates and was recommended as the treatment of choice [Vinchon et al., 2005]. In one series of 26 neonates with subdural hematomas, only 1 required surgery for elevation of a depressed skull fracture [Chamnanvanakij et al., 2002]. Perrin et al. [1997] studied a series of 15 neonates with posterior fossa subdural hematomas for whom neurosurgical consultation was obtained. In this series, 8 of 15 patients underwent surgical evacuation. Indication for surgery included development of signs of progressive brainstem compression [Perrin et al., 1997]. In another case series of 48 neonates with subdural hematomas, 13 underwent neurosurgical intervention, consisting of burr holes, craniotomy, or placement of a ventriculoperitoneal shunt. The investigators recommend surgical intervention for increased intracranial pressure, obstructive hydrocephalus, or accompanying intracerebral hemorrhage [Hayashi et al., 1987].
Short-term outcome in children with an isolated infratentorial subdural hemorrhage, and also in those with a lobar hemorrhage, has often been reported to be more favourable than expected [Govaert et al., 1992b; Hanigan et al., 1995].
Neurologic sequelae after perinatal subdural hematoma depend on the location of the hematoma, associated cerebral injury, and accurate diagnosis and management. In one study of 26 infants with subdural hematoma, 9 (34.6 percent) had an abnormal neurologic examination at discharge, with 7 infants exhibiting mild hypotonia and 2 infants with Erb’s palsy. There were no deaths in this series [Chamnanvanakij et al., 2002]. In another series, Perrin et al. [1997] retrospectively reviewed charts of 15 children with perinatal subdural hematoma. Follow-up ranged from 2 to 10 years, with a mean of 4.5 years. There were no deaths in this series, but 3 children were profoundly delayed, 2 were moderately delayed, 3 were mildly delayed, and 7 were neurodevelopmentally normal. Hayashi et al. [1987] found that 8 (17 percent) of 46 neonates with subdural hematomas had some developmental abnormalities 6 months to 3 years after injury.
Intracerebral hemorrhage may occur in isolation or in association with other cranial birth injuries, including subdural hematomas or cerebral vascular injuries. Possible mechanisms of injury include direct compression of the brain as a result of molding, forcing the frontal bone under the parietal bone during passage through the birth canal, occipital osteodiastasis resulting in intracerebellar hemorrhage, asphyxia inducing hemorrhagic arterial infarct, or venous infarct due to sagittal sinus thrombosis [Govaert et al., 1992a; Hayashi et al., 1987].
Intracerebral hemorrhage occurs in 7–20 percent of full-term infants with intracranial hemorrhage [Hanigan et al., 1995; Pollina et al., 2001; Sandberg et al., 2001]. In one report, 31 percent of infants had risk factors for intracerebral hemorrhage, including perinatal asphyxia, coagulopathy, complicated delivery, or hyperviscosity. However, up to 50 percent of neonates with intracerebral hemorrhage in this series did not have any identifiable risk factors [Hanigan et al., 1995]. The most common presentation for intracerebral hemorrhage is seizures within 48 hours of birth [Govaert et al., 1992b; Hanigan et al., 1995; Medlock and Hanigan, 1997]. In a recent study by Armstrong-Wells et al. [2009], this lesion was referred to as perinatal hemorrhagic stroke (PHS). They identified 20 cases among 323,532 live births (19 intracerebral hemorrhage and 1 subarachnoid hemorrhage), which gave a prevalence of 6.2 in 100 000 live births. Infants presented with encephalopathy (100 percent) and seizures (65 percent). PHS was typically unifocal (74 percent) and unilateral (83 percent). Etiologies included thrombocytopenia (n = 4) and cavernous malformation (n = 1), but most 15 (75 percent) were idiopathic. Fetal distress and postmaturity were identified as independent risk factors. Antenatal onset of parenchymal hemorrhage can be associated with a mutation in the COL4A1 gene [de Vries et al., 2009; Meuwissen et al., 2011]. The lesions can be severe, with almost complete destruction of both hemispheres, and the cerebellum can be involved as well (Figure 18-8). In the study by Sandberg et al. [2001], only 11 infants were identified from January 1960 to February 2000, and most presented within the first 2 days of life (6 of 11 patients); the most common presenting sign was seizure (7 of 11 patients). No cause was identified in 6 of 11 patients; the remainder were attributed to coagulopathy (n = 3), ruptured intracranial aneurysm (n = 1), or hemorrhagic infarction (n= 1). Eight patients underwent surgical hematoma evacuation on the basis of radiographic evidence of significant mass effect, evidence of signs of elevated intracranial pressure, or both. Four patients had normal neurologic outcomes, 4 had motor deficits (one of whom additionally demonstrated cognitive delay), and 3 had delayed speech. Outcome is often reported to be better, even in the context of large intracerebral lesions [Brouwer et al., 2010; Huang et al., 2004; Sandberg et al., 2001]. In a subgroup of infants with intracerebral lesions, the temporal lobe is affected; these infants tend to present with apnea as the initial manifestation [Sirsi et al., 2007; Slaugther et al., 2009; Hoogstraate et al., 2009]. Slaughther et al. [2009] suggested that neonatal temporal lobe hemorrhagic infarct could be secondary to suspected superficial temporal venous thrombosis but this was not confirmed in all patients with MR venography. In spite of often dramatic imaging abnormalities, a good clinical outcome was noted. The frontal lobe has been recognized as another predilection site, often following an uncomplicated vaginal delivery.
In a recent series of 53 infants with a subdural hemorrhage associated with an infra- or supratentorial intracerebral hemorrhage, mortality was high (24.5 percent), with the lowest mortality in infants with a supratentorial intracerebral hemorrhage (10 percent). Three infants with a midline shift required a craniotomy; 6 infants needed a subcutaneous reservoir due to outflow obstruction and 3 subsequently required a ventriculoperitoneal shunt. The group with a poor outcome (death or developmental quotient <85) had a significantly lower 5-minute Apgar score (p = 0.005). Follow-up was available in 36 of 40 survivors, at an age of at least 15 months. Patients were assessed with the Griffiths’ Mental Developmental Scale, and the mean DQ of all survivors was 97. Six infants (17 percent) had a DQ below 85 (2 of them had cerebral palsy). Three infants developed cerebral palsy (8.6 percent); 1 had cerebellar ataxia and 2 a hemiplegia (Brouwer et al., 2010).
Subarachnoid hemorrhage
Subarachnoid hemorrhage is a relatively common neonatal intracranial injury and occurs as a result of tearing of veins traversing the subarachnoid space [Hughes et al., 1999; King and Boothroyd, 1998]. With the increased use of CT and MRI, clinically insignificant subarachnoid hemorrhage has been diagnosed with increasing frequency [Whitby et al., 2004]. In their study of 583,340 singleton live births, Towner et al. [1999] found the incidence of subarachnoid hemorrhage varied from 0.9 per 10,000 infants born by cesarean section to as high as 10.7 per 10,000 infants born by vacuum- and forceps-assisted delivery. In Pollina’s series of 41 infants with cranial birth injury, 20 percent had subarachnoid hemorrhage [Pollina et al., 2001].
Presenting symptoms include decreased level of consciousness, hypotonia, and seizures [Hughes et al., 1999]. Hydrocephalus may develop because of obstruction of the fourth ventricle by clot formation or by subarachnoid adhesions over the convexities [Hayashi et al., 1987; King and Boothroyd, 1998]. Subarachnoid hemorrhage may cause cerebral vasospasm, leading to infarction [Medlock and Hanigan, 1997] (Figure 18-9).
Cranial Nerve Injuries
Acquired facial nerve palsy occurs in 1.8–7.5 per 1000 deliveries and is more common in males than females (2:1 in one study). Most cases are associated with forceps deliveries, but 33 percent occur after spontaneous delivery. The mechanism of injury in either case is similar, with compression of the facial nerve at the stylomastoid foramen either by the posterior forceps blade or by compression against the maternal sacral promontory [Hughes et al., 1999; Medlock and Hanigan, 1997].
Signs and symptoms include difficulty in closing the eyelid on the affected side, loss of the nasolabial fold, and an asymmetric crying facies. Electromyography and auditory brainstem response testing are recommended for neonates in whom there is no improvement over 3–4 days. Surgical intervention usually is deferred until 1–2 years of age [Hughes et al., 1999].
Other cranial nerve injuries include abducens nerve palsies, hypoglossal nerve palsies, and recurrent laryngeal nerve palsies [Medlock and Hanigan, 1997].
Spinal Cord Injuries
Cervical spinal cord injury is an important, although rare, cause of neonatal morbidity and mortality, for which the diagnosis is often delayed or overlooked during the initial evaluation. The incidence is difficult to ascertain because examination of the spinal cord is not routinely done at postmortem examination. In one series, 45 percent of neonates undergoing postmortem examination had evidence of distortional trauma to the spinal cord [Yates, 1959]; in a second series, the incidence of significant spinal or brainstem injury was greater than 10 percent [Towbin, 1969]. In a Canadian study from 1993, the investigators cite an incidence of 1 case in 29,000 live births [Rehan and Seshia, 1993], whereas another study reported an incidence of upper cervical spinal cord injury of 1 in 80,000 [Menticoglou et al., 1995].
Spinal cord injury may occur as a result of longitudinal traction, hyperextension or hyperflexion of the neck, excessive rotational force, or ischemic damage to the spinal cord [Filippigh et al., 1994; Morota et al., 1992; Rossitch and Oakes, 1992]. Because the interspinous ligaments, posterior joint capsule, and cartilaginous end plates of the neonate are elastic and redundant, the spine is more deformable in the child than in the adult. The facet joints are more horizontally oriented and therefore less stable. These factors make the pediatric spine more susceptible to hyperextension injury. This type of injury may occur in utero and usually results in a high spinal cord lesion [Rossitch and Oakes, 1992]. The neonatal spinal column is quite elastic, but the spinal cord can be stretched only 0.25 inch before rupturing. This difference in elasticity can result in spinal cord injury without radiographic abnormality (SCIWORA) because the bony and ligamentous elements may remain intact, even when the spinal cord has been ruptured. Forceful longitudinal traction during difficult breech delivery may cause this type of injury, and results in lower cervical to high thoracic spinal cord injury [Morgan and Newell, 2001; Rossitch and Oakes, 1992, Vialle et al., 2008]. Torsional forces during instrumentation with forceps, with head rotation of 90° or more, may cause rotational injury during delivery with cephalic presentation [Medlock and Hanigan, 1997]. This type of injury typically results in high cervical cord lesions [Rossitch and Oakes, 1992].
Diagnosis of spinal cord injury is often delayed because of the variable presentation and frequent presence of confounding factors. There is often concomitant perinatal asphyxia, respiratory distress, and brachial plexus injury [Mills et al., 2001; Onal et al., 2002; Rossitch and Oakes, 1992]. Neonates with spinal cord injury are flaccid, with absent spontaneous movements and absent deep tendon reflexes. Complex spinal reflexes may be present and misleading. Apnea may occur in high cervical cord injury, requiring intubation and mechanical ventilation. A sensory level can be detected by lack of facial response to painful stimuli below the level of injury [Filippigh et al., 1994; MacKinnon et al., 1993; Rossitch and Oakes, 1992]. The differential diagnosis includes HIE, spinal muscular atrophy, congenital myopathies, brachial plexus injury, congenital intraspinal tumors, myelodysplasia, and sepsis. Unaffected facial muscles and presence of a sensory level can distinguish spinal cord injury from spinal muscular atrophy and cerebral diplegia [Filippigh et al., 1994]. Radiographic evaluation can elucidate the cause in most neonates.
A variety of radiographic tests can be useful in the setting of suspected spinal cord injury. Plain films of the spine are often normal because of the deformability of the neonatal spine [Rehan and Seshia, 1993; Rossitch and Oakes, 1992]. Ultrasonography is useful in initial evaluation as a noninvasive test that may be done at the bedside. Due to incomplete ossification of the vertebral arch in neonates, the spinal cord may be visualized, and edema, hematomyelia, extraspinal hematoma, and avulsion of a nerve root may be revealed [Filippigh et al., 1994; Rehan and Seshia, 1993; Simon et al., 1999]. CT delineates the spinal column better than the cord itself, but bony abnormalities are relatively uncommon. CT myelography is invasive and may lead to false-negative or false-positive results [MacKinnon et al., 1993]. If the infant is stable enough for transport, MRI is the imaging modality of choice. It provides excellent soft-tissue delineation and can distinguish edema from hemorrhage, infarction, vascular anomalies, or congenital anomalies [Blount et al., 2004; Mills et al., 2001; Onal et al., 2002; Rehan and Seshia, 1993]. Somatosensory-evoked potentials may provide additional useful information [Rehan and Seshia, 1993].
The long-term outcome of neonatal spinal cord injury depends on the location of the injury. Infants with a lesion above C4 who display recovery of breathing movements in the first day with continued rapid recovery generally have a good outcome [MacKinnon et al., 1993]. Perinatal spinal cord injury usually results in some degree of permanent neurologic deficit (Figure 18-10). In one retrospective series of 22 patients, all had at least mild disability. Seven patients with upper cervical cord lesions died, and four with cervicothoracic or thoracolumbar injuries died [MacKinnon et al., 1993]. Postmortem examination of the spinal cord in three neonates with intramedullary cord hemorrhage revealed irreversible spinal cord trauma, whereas a fourth neonate in the same case series, who had presented identically with quadriparesis, had only cord edema without hemorrhage and made a complete recovery. Similar to adult spinal cord injury, edema of the spinal cord without intramedullary hemorrhage on MRI is associated with a good outcome [Mills et al., 2001].
Brachial Plexus Injury
Brachial plexus injury is a relatively common birth injury. The reports of the largest populations found incidences occurring in 0.42 and 1.5 per 1000 live births (Gilbert et al., 1999; Donnelly et al., 2002; Evans-Jones et al., 2003; Andersen et al., 2006; McNeely and Drake, 2003; Medlock and Hanigan, 1997; van Ouwerkerk et al., 2000].
Despite improved perinatal care, the incidence of brachial plexus injury has not declined, possibly because of increasing birth weights. Predisposing factors include macrosomia, breech presentation, shoulder dystocia, and assisted delivery with forceps or vacuum extraction [McNeely and Drake, 2003; van Ouwerkerk et al., 2000]. In the study by Evans-Jones et al. [2003], significant associated risk factors were compared with the normal population: shoulder dystocia (60 percent vs. 0.3 percent); high birth weight, with 53 percent infants weighing more than the 90th percentile; and assisted delivery (relative risk [RR] 3.4, 95 percent confidence interval [CI] 2.9–3.9, p = 0.0001). There was a considerably lower risk of brachial plexus palsy in infants delivered by cesarean section (RR = 7, 95 percent CI 2–56, p = 0.002).
The mechanism of injury is thought to be excessive traction on the brachial plexus caused by lateral flexion and traction on the head during a difficult delivery with shoulder dystocia. In cases of breech presentation, bilateral injury may exist [van Ouwerkerk et al., 2000]. Four types of injury have been described: neuropraxia, or temporary conduction block; axonotmesis, in which the axon is severed but surrounding neural elements are intact; neurotmesis, or complete postganglionic disruption of a nerve; and avulsion, or preganglionic disconnection from the spinal cord [van Ouwerkerk et al., 2000]. Brachial plexus injury may also be classified as complete, upper, or lower palsies [Medlock and Hanigan, 1997].
Diagnosis is generally made by noticing an asymmetric Moro reflex. Erb’s palsy is characterized by injury to C5 and C6, resulting in paralysis of the deltoid, biceps, brachioradialis, and supinator muscles. Grasp is preserved, and the arm is held in the “waiter’s tip” position. This accounts for 90 percent of brachial plexus injuries. Klumpke’s paralysis involves C7 and results in loss of the grasp reflex in addition to the previously described symptoms. With involvement of the sympathetic fibers from the first thoracic root, Horner’s syndrome may be identified [Hughes et al., 1999]. Complete plexus injury results in atonic “flail limb” and a Horner’s sign. Differential diagnosis includes arthrogryposis, sepsis or osteomyelitis of the shoulder joint, fractures of the humerus or clavicle, upper motor neuron injury, and spinal cord injury [van Ouwerkerk et al., 2000]. Electromyography and nerve conduction studies may be considered to assess the degree of injury and to guide therapy for future management [Hughes et al., 1999; Medlock and Hanigan, 1997]. MRI can delineate the extent of injury and distinguish between brachial plexus and spinal cord injury or congenital anomalies [Medlock and Hanigan, 1997]. MR neurography enables localization of injured nerves and characterization of associated pathology, and is a useful adjunct in treatment planning [Smith et al., 2008].
Management is controversial. Most injuries resolve spontaneously and require only supportive care with physical therapy and application of dynamic splints [Hughes et al., 1999]. In a systematic review of brachial plexus surgery, McNeely and Drake [2003] conclude that there is no definitive advantage to surgery over conservative management. Results of surgical intervention were variable, and patients frequently did not regain normal function. They recommend waiting at least 3–6 months before proceeding with surgical intervention. Andersen et al. [2006] reviewed data from nonrandomized studies and these indicated that children with severe injuries do better with surgical repair. Malessy and Pondaag [2009] recommend restricting surgery to severe cases in which spontaneous restoration of function is unlikely to occur (i.e., in neurotmesis or root avulsions).
Brachial plexus injuries often resolve spontaneously, but in 5–25 percent of children, there is residual extremity weakness [Andersen et al., 2006; Hughes et al., 1999; McNeely and Drake, 2003; van Ouwerkerk et al., 2000]. In a prospective observational study of the natural history of brachial plexus injuries, Noetzel et al. [2001] found that, for infants who had not recovered antigravity movement at the shoulder at 2 weeks of age, the degree of residual weakness at 6 months was highly predictive of eventual recovery. Permanent weakness or disability was highly likely in those children who had less than antigravity strength in the biceps, triceps, and deltoid muscles, or absence of active wrist extension. From the review by Andersen et al. [2006], it was noted that 75 percent of affected infants recover completely within the first month of life. Permanent impairment and disability are experienced by 25 percent of affected infants. Andersen et al. recommended referral to a multidisciplinary brachial plexus team if a physical examination demonstrated an incomplete recovery by the end of the first month.
References
The complete list of references for this chapter is available online at www.expertconsult.com.
Adams H.P. Venous thrombosis, pituitary apoplexy and vascular disease of the spinal cord. In Principles of cerebrovascular disease, ed 1, New York: McGraw-Hill; 2007:347-348.
Akiyama Y., Moritake K., Maruyama N., et al. Acute epidural hematoma related to cesarean section in a neonate with Chiari II malformation. Childs Nerv Syst. 2001;17:290.
Amar A.P., Aryan H.E., Meltzer H.S., et al. Neonatal subgaleal hematoma causing brain compression: Report of two cases and review of the literature. Neurosurgery. 2003;52:1470.
Andersen J., Watt J., Olson J., et al. Perinatal brachial plexus palsy. Paediatr Child Health. 2006;11:93.
Arichi T., Moraux A., Melendez A., et al. Somatosensory cortical activation identified by functional MRI in preterm and term infants. Neuroimage. 2009. Oct 23
Armstrong-Wells J., Johnston S.C., Wu Y.W., et al. Prevalence and predictors of perinatal hemorrhagic stroke: results from the Kaiser pediatric stroke study. Pediatrics. 2009;123:823.
Ashwal S., Holshouser B., Tong K., et al. Proton MR spectroscopy detected glutamate/glutamine is increased in children with traumatic brain injury. J Neurotrauma. 2004;21:1539.
Ashwal S., Holshouser B., Tong K., et al. Proton spectroscopy detected myoinositol in children with traumatic brain injury. Pediatr Res. 2004;56:630.
Ashwal S., Babikian T., Gardner-Nichols J., et al. Susceptibility-weighted imaging and proton magnetic resonance spectroscopy in assessment of outcome after pediatric traumatic brain injury. Arch Phys Med Rehabil. 2006;87(12 Suppl 2):S50.
Ashwal S., Holshouser B.A., Tong K.A. Use of advanced neuroimaging techniques in the evaluation of pediatric traumatic brain injury. Dev Neurosci. 2006;28:309.
Ashwal S., Obenaus A., Snyder E.Y. Neuroimaging as a basis for rational stem cell therapy. Pediatr Neurol. 2009;40:227.
Ballantyne A.O., Spilkin A.M., Trauner D.A. Language outcome after perinatal stroke: does side matter? Child Neuropsychol. 2007;13:494.
Ballantyne A.O., Trauner D.A. Facial recognition in children after perinatal stroke. Neuropsychiatry Neuropsychol Behav Neurol. 1999;12:82.
Ballantyne A.O., Spilkin A.M., Hesselink J., et al. Plasticity in the developing brain: intellectual, language and academic functions in children with ischaemic perinatal stroke. Brain. 2008;131:2975.
Bayir H., Kochanek P.M., Kagan V.E. Oxidative stress in immature brain after traumatic brain injury. Dev Neurosci. 2006;28:420.
Beers S.R., Berger R.P., Adelson P.D. Neurocognitive outcome and serum biomarkers in inflicted versus non-inflicted traumatic brain injury in young children. J Neurotrauma. 2007;24:97.
Benders M.J., Groenendaal F., De Vries L.S. Preterm arterial ischemic stroke. Semin Fetal Neonatal Med. 2009;14:272.
Benders M.J.N.L., Groenendaal F., Uiterwaal C.S.P.M., et al. Maternal and infant characteristics associated with perinatal arterial stroke in the preterm infant. Stroke. 2007;38:1759-1765.
Berfelo FJ, Kersbergen KJ, van Ommen CH, et al: Neonatal Cerebral Sinovenous Thrombosis: Clinical symptoms, imaging, risk factors, treatment and outcome, Stroke 41(70):1382–1388
Berger R.P., Beers S.R., Richichi R., et al. Serum biomarker concentrations and outcome after pediatric traumatic brain injury. J Neurotrauma. 2007;24:1793.
Bittigau P., Sifringer M., Pohl D., et al. Apoptotic neurodegeneration following trauma is markedly enhanced in the immature brain. Ann Neurol. 1999;45:724.
Blount J., Doughty K., Tubbs R.S., et al. In utero spontaneous cervical thoracic epidural hematoma imitating spinal cord birth injury. Pediatr Neurosurg. 2004;40:23.
Brouwer A.J., Groenendaal F., Koopman C., et al. Intracranial hemorrhage in full term newborns: a hospital-based cohort study. Neuradiology. 2010;52:567.
Burke C.J., Tannenberg A.E., Payton D.J. Ischaemic cerebral injury, intrauterine growth retardation and placental infarction. Dev Med Child Neurol. 1997;39:726.
Carvalho K.S., Bodensteiner J.B., Connolly P.J., et al. Cerebral venous thrombosis in children. J Child Neurol. 2001;16:574.
Chabrier S., Saliba E., Nguyen The Tich S., et al. Obstetrical and neonatal characteristics vary with birthweight in a cohort of 100 term newborns with symptomatic arterial ischemic stroke. Eur J Paediatr Neurol. 2009. epub21
Chadwick L.M., Pemberton P.J., Kurinczuk J.J. Neonatal subgaleal haematoma: associated risk factors, complications and outcome. J Paediatr Child Health. 1996;32:228-232.
Chamnanvanakij S., Rollins N., Perlman J.M. Subdural hematoma in term infants. Pediatr Neurol. 2002;26:301.
Chang H.Y., Peng C.C., Kao H.A., et al. Neonatal subgaleal hemorrhage: clinical presentation, treatment, and predictors of poor prognosis. Pediatr Int. 2007;49:903.
Chen J., Zimmerman R.A., Jarvik G.P., et al. Perioperative stroke in infants undergoing open heart operations for congenital heart disease. Ann Thorac Surg. 2009;88:823.
Clancy R., Malin S., Laraque D., et al. Focal motor seizures heralding stroke in full-term neonates. Am J Dis Child. 1985;139:601.
Cooper J., Majnemer A., Rosenblatt B., et al. The determination of sensory deficits in children with hemiplegic cerebral palsy. J Child Neurol. 1995;10:300.
Curry C.J., Bhullar S., Holmes J., et al. risk factors for perinatal arterial stroke: a study of 60 mother-child pairs. Pediatr Neurol. 2007;37:99.
Davies R.P., Slavotinek J.P. Incidence of the empty delta sign in computed tomography in the paediatric age group. Australas Radiol. 1994;38:17.
Deluca S.C., Echols K., Law C.R., et al. Intensive pediatric constraint-induced therapy for children with cerebral palsy: randomized, controlled, crossover trial. J Child Neurol. 2006;21:931.
deVeber G.A., MacGregor D., Curtis R., et al. Neurologic outcome in survivors of childhood arterial ischemic stroke and sinovenous thrombosis. J Child Neurol. 2000;15:316.
deVeber G., Andrew M., Adams C., et al. Cerebral sinovenous thrombosis in children. N Engl J Med. 2001;345:417.
de Vries L.S., Groenendaal F., Eken P., et al. Infarcts in the vascular distribution of the middle cerebral artery in preterm and fullterm infants. Neuropediatrics. 1997;28:88.
de Vries L.S., Van der Grond J., Van Haastert I.C., et al. Prediction of outcome in new-born infants with arterial ischaemic stroke using diffusion-weighted magnetic resonance imaging. Neuropediatrics. 2005;36:12.
de Vries L.S., Koopman C., Groenendaal F., et al. COL4A1 mutation in two preterm siblings with antenatal onset of parechymal hemorrhage. Ann Neurol. 2009;65:12.
Donnelly V., Foran A., Murphy J., et al. Neonatal brachial plexus palsy: an unpredictable injury. Am J Obstet Gynecol. 2002;187:1209.
Duhaime A.C., Durham S. Traumatic brain injury in infants: the phenomenon of subdural hemorrhage with hemispheric hypodensity (“Big Black Brain”). Prog Brain Res. 2007;161:293.
Eichler F., Krishnamoorthy K., Grant P.E. Magnetic resonance imaging evaluation of possible neonatal sinovenous thrombosis. Pediatr Neurol. 2007;37:317.
Elbers J., Viero S., MacGregor D., et al. Placental pathology in neonatal stroke. Pediatrics. 2011;127:e722.
Estan J., Hope P. Unilateral neonatal cerebral infarction in full term infants. Arch Dis Child. 1997;76:F88.
Evans-Jones G., Kay S.P., Weindling A.M., et al. Congenital brachial palsy: Incidence, causes, and outcome in the United Kingdom and Republic of Ireland. Arch Dis Child Fetal Neonatal Ed. 2003;88:F185.
Filippigh P., Clapuyt P., Debauche C., et al. Sonographic evaluation of traumatic spinal cord lesions in the newborn infant. Pediatr Radiol. 1994;24:245.
Fitzgerald K.C., Williams L.S., Garg B.P., et al. Cerebral sinovenous thrombosis in the neonate. Arch Neurol. 2006;63:405.
Fullerton H.J., Wu Y.W., Sidney S., et al. Risk of recurrent childhood arterial ischemic stroke in a population-based cohort: the importance of cerebrovascular imaging. Pediatrics. 2007;119:495.
Geddes J.F., Hackshaw A.K., Vowles G.H., et al. Neuropathology of inflicted head injury in children. I. Patterns of brain damage. Brain. 2001;124(Pt 7):1290.
Geddes J.F., Vowles G.H., Hackshaw A.K., et al. Neuropathology of inflicted head injury in children. II. Microscopic brain injury in infants. Brain. 2001;124(Pt 7):1299.
Gilbert W.M., Nesbitt T.S., Danielsen B. Associated factors in 1611 cases of brachial plexus injury. Obstet Gynecol. 1999;93:536.
Giroud M., Fayolle H., Martin D., et al. Late thalamic atrophy in infarction of the middle cerebral artery territory in neonates. A prospective clinical and radiological study in four children. Childs Nerv Syst. 1995;11:133.
Giza C.C., Prins M.L. Is being plastic fantastic? Mechanisms of altered plasticity after developmental traumatic brain injury. Dev Neurosci. 2006;28:364.
Glenn O.A., Ludeman N.A., Berman J.I., et al. Diffusion tensor MR imaging tractography of the pyramidal tracts correlates with clinical motor function in children with congenital hemiparesis. Am J Neuroradiol. 2007;28:1796.
Golomb M.R., deVeber G.A., MacGregor D.L., et al. Independent walking after neonatal arterial ischemic stroke and sinovenous thrombosis. J Child Neurol. 2003;18:530.
Golomb M.R., Carvalho K.S., Garg B.P. A 9-year-old boy with a history of large perinatal stroke, infantile spasms, and high academic achievement. J Child Neurol. 2005;20:444.
Golomb M.R., Garg B.P., Williams L.S. Outcomes of children with infantile spasms after perinatal stroke. Pediatr Neurol. 2006;34:291.
Golomb M.R., Garg B.P., Carvalho K.S., et al. Perinatal stroke and the risk of developing childhood epilepsy. J Pediatr. 2007;151:409.
Golomb M.R., Garg B.P., Saha C., et al. Cerebral palsy after perinatal arterial ischemic stroke. Child Neurol. 2008;23:279.
Golomb M.R., Fullerton H., Novak-Gottl U., et al. Male predominance in childhood ischemic stroke: findings from the International Pediatric Stroke Study. Stroke. 2009;40:52.
Govaert P., Voet D., Achten E., et al. Noninvasive diagnosis of superior sagittal sinus thrombosis in a neonate. Am J Perinatol. 1992;9:201.
Govaert P., Vanhaesebrouck P., de Praeter C. Traumatic neonatal intracranial bleeding and stroke. Arch Dis Child. 1992;67:840.
Grundl P.D., Biagas K.V., Kochanek P.M., et al. Early cerebrovascular response to head injury in immature and mature rats. J Neurotrauma. 1994;11:135.
Gunther G., Junker R., Strater R., et al. Childhood Stroke Study Group. Symptomatic ischemic stroke in full-term neonates: role of acquired and genetic prothrombotic risk factors. Stroke. 2000;31:2437.
Haase R., Kursawe I., Nagel F., et al. Acute subdural hematoma after caesarean section: A case report. Pediatr Crit Care Med. 2003;4:246.
Hanigan W.C., Powell F.C., Miller T.C., et al. Symptomatic intracranial hemorrhage in full-term infants. Childs Nerv Syst. 1995;11:698.
Hayashi T., Hashimoto T., Fukuda S., et al. Neonatal subdural hematoma secondary to birth injury. Clinical analysis of 48 survivors. Childs Nerv Syst. 1987;3:23.
Heep A., Scheef L., Jankowski J., et al. Functional magnetic resonance imaging of the sensorimotor system in preterm infants. Pediatrics. 2009;123:294.
Hofmeyr G.J., Hannah M.E.. Planned caesarean section for term breech delivery. CD000166 Cochrane Database Syst Rev. 2003(3). Review
Holmefur M., Krumlinde-Sundholm L., Bergstrom J., et al. Longitudinal development of hand function in children with unilateral cerebral palsy. Dev Med Child Neurol. 2009. epub
Hoogstraate S.R., Lequin M.H., Huysman M.A., et al. Apnoea in relation to neonatal temporal lobe haemorrhage. Eur J Paediatr Neurol. 2009;13:356. Epub 2008 Aug 30
Huang A.H., Robertson R.L. Spontaneous superficial parenchymal and leptomeningeal hemorrhage in term neonates. Am J Neuroradiol. 2004;25:469.
Hughes C.A., Harley E.H., Milmoe G., et al. Birth trauma in the head and neck. Arch Otolaryngol Head Neck Surg. 1999;125:193.
Husain J., Juurlink B.H. Oligodendroglial precursor cell susceptibility to hypoxia is related to poor ability to cope with reactive oxygen species. Brain Res. 1995;698:86.
Jordan LC, Rafay MF, Smith SE, et al: Antithrombotic treatment in neonatal cerebral sinovenous thrombosis: results of the international pediatric stroke study, J Pediatr in press
Juenger H., Linder-Lucht M., Walther M., et al. Cortical neuromodulation by constraint-induced movement therapy in congenital hemiparesis: an FMRI study. Neuropediatrics. 2007;38:130.
Kawabori M., Kuroda S., Kudo K., et al. Susceptibility-weighted magnetic resonance imaging detects impaired cerebral hemodynamics in the superior sagittal sinus thrombosis–case report. Neurol Med Chir (Tokyo). 2009;49:248.
Kenet G., Kirkham F., Niederstadt T., et al. Risk factors for recurrent venous thromboembolism in the european collaborative paediatric database on cerebral venous thrombosis: A multicentre cohort study. Lancet Neurol. 2007;6:595.
Kersbergen K.J., de Vries L.S., van Straaten H.L., et al. Anticoagulation therapy and imaging in neonates with a unilateral thalamic hemorrhage due to cerebral sinovenous thrombosis. Stroke. 2009;40:2754.
Kilani R.A., Wetmore J. Neonatal subgaleal hematoma: presentation and outcome–radiological findings and factors associated with mortality. Am J Perinatol. 2006;23:41.
King S.J., Boothroyd A.E. Cranial trauma following birth in term infants. Br J Radiol. 1998;71:233.
Kirton A., deVeber G. Advances in perinatal ischemic stroke. Pediatr Neurol. 2009;40:205.
Kirton A., Shroff M., Visvanathan T., et al. Quantified corticospinal tract diffusion restriction predicts neonatal stroke outcome. Stroke. 2007;38:974.
Kirton A., Deveber G., Pontigon A.M., et al. Presumed perinatal ischemic stroke: vascular classification predicts outcomes. Ann Neurol. 2008;63:436.
Kochanek P.M. Pediatric traumatic brain injury: quo vadis? Dev Neurosci. 2006;28:244.
Koelfen W., Freund M., Varnholt V. Neonatal stroke involving the middle cerebral artery in term infants: Clinical presentation, EEG and imaging studies, and outcome. Dev Med Child Neurol. 1995;37:204.
Kolb B. Overview of cortical plasticity and recovery from brain injury. Phys MedRehabil Clin N Am. 2003;14(1 Suppl):S7-S25. viii
Kolb B. Recovery from early cortical damage in rats. I. Differential behavioral andanatomical effects of frontal lesions at different ages of neural maturation. BehavBrain Res. 1987;25:205.
Kraus F.T., Acheen V.I. Fetal thrombotic vasculopathy in the placenta: cerebral thrombi and infarcts, coagulopathies, and cerebral palsy. Hum Pathol. 1999;30:759.
Kuhnke N., Juenger H., Walther M., et al. Do patients with congenital hemiparesis and ipsilateral corticospinal projections respond differently to constraint-induced movement therapy? Dev Med Child Neurol. 2008;50:898.
Kung H.C., Hoyert D.L., Xu J., et al. Deaths: final data for 2005. Natl Vital Stat Rep. 2008;56:37.
Kurnik K., Kosch A., Strater R., et al. Childhood Stroke Study Group. (2003) Recurrent thromboembolism in infants and children suffering from symptomatic neonatal arterial stroke: a prospective follow-up study. Stroke. 2003;34:2887.
Laugesaar R., Kolk A., Tomberg T. Acutely and retrospectively diagnosed perinatal stroke: a population-based study. Stroke. 2007;38:2234.
Lea PMt, Faden A.I. Traumatic brain injury: developmental differences in glutamate receptor response and the impact on treatment. Ment Retard Dev Disabil Res Rev. 2001;7:235.
Lee J., Croen L.A., Lindan C., et al. Predictors of outcome in perinatal arterial stroke: a population-based study. Ann Neurol. 2005;58:303.
Lequin M.H., Dudink J., Tong K.A., et al. Magnetic resonance imaging in neonatal stroke. Semin Fetal Neonatal Med. 2009;14:299.
Looney C.B., Smith J.K., Merck L.H., et al. Intracranial hemorrhage in asymptomatic neonates: prevalence on MR images and relationship to obstetric and neonatal risk factors. Radiology. 2007;242:535.
Lynch J.K., Nelson K.B. Epidemiology of perinatal stroke. Curr Opin Pediatr. 2001;13:499.
Lynch J.K., Hirtz D.G., DeVeber G., et al. Report of the National Institute of Neurological Disorders and Stroke workshop on perinatal and childhood stroke. Pediatrics. 2002;109:116.
MacKinnon J.A., Perlman M., Kirpalani H., et al. Spinal cord injury at birth: Diagnostic and prognostic data in twenty-two patients. J Pediatr. 1993;122:431.
Malessy M.J., Pondaag W. Obstetric brachial plexus injuries. Neurosurg Clin N Am. 2009;20:1-14. Review
Mazumdar A., Mukherjee P., Miller J.H., et al. Diffusion-weighted imaging of acute corticospinal tract injury preceding Wallerian degeneration in the maturing human brain. Am J Neuroradiol. 2003;24:1057.
McDonald J.W., Silverstein F.S., Johnston M.V. Neurotoxicity of N-methyl-D-aspartate is markedly enhanced in developing rat central nervous system. Brain Res. 1988;459:200.
McDonald J.W., Trescher W.H., Johnston M.V. Susceptibility of brain to AMPA induced excitotoxicity transiently peaks during early postnatal development. Brain Res. 1992;583:54.
McNeely P.D., Drake J.M. A systematic review of brachial plexus surgery for birth-related brachial plexus injury. Pediatr Neurosurg. 2003;38:57.
McQuillen P.S., Hamrick S.E., Perez M.J., et al. Balloon atrial septostomy is associated with preoperative stroke in neonates with transposition of the great arteries. Circulation. 2006;113:280.
Medlock M.D., Hanigan W.C. Neurologic birth trauma. Intracranial, spinal cord, and brachial plexus injury. Clin Perinatol. 1997;24:845.
Menticoglou S.M., Perlman M., Manning F.A. High cervical spinal cord injury in neonates delivered with forceps: Report of 15 cases. Obstet Gynecol. 1995;86:589.
Mercuri E., Rutherford M., Cowan F., et al. Early prognostic indicators of outcome in infants with neonatal cerebral infarction: A clinical, electroencephalogram, and magnetic resonance imaging study. Pediatrics. 1999;103:39.
Mercuri E., Cowan F., Gupte G., et al. Prothrombotic disorders and abnormal neurodevelopmental outcome in infants with neonatal cerebral infarction. Pediatrics. 2001;107:1400.
Mercuri E., Anker S., Guzzetta A., et al. Neonatal cerebral infarction and visual function at school age. Arch Dis Child Fetal Neonatal Ed. 2003;88:F487.
Mercuri E., Barnett A., Rutherford M., et al. Neonatal cerebral infarction and neuromotor outcome at school age. Pediatrics. 2004;113:95.
Meuwissen M.E.C., de Vries L.S., Verbeek H.A., et al. Sporadic COL 4A1 mutations with extensive prenatal porencephaly resembling hydraencephaly. Neurology. 2011;76:844.
Miller S.P., Wu Y.W., Lee J., et al. Candidate gene polymorphisms do not differ between newborns with stroke and normal controls. Stroke. 2006;37:2678.
Mills J.F., Dargaville P.A., Coleman L.T., et al. Upper cervical spinal cord injury in neonates: The use of magnetic resonance imaging. J Pediatr. 2001;138:105.
Moharir M.D., Shroff M., Stephens D., et al. Anticoagulants in pediatric cerebral sinovenous thrombosis: a safety and outcome study. Ann Neurol. 2010;67:590.
Monagle P., Chan A., Massicotte P., et al. Antithrombotic therapy in children: The seventh ACCP conference on antithrombotic and thrombolytic therapy. Chest. 2004;126:645S.
Monagle P., Chalmers E., Chan A., et al. Antithrombotic therapy in neonates and children: American college of chest physicians evidence-based clinical practice guidelines (8th edition). Chest. 2008;133:887S.
Morgan C., Newell S.J. Cervical spinal cord injury following cephalic presentation and delivery by Caesarean section. Dev Med Child Neurol. 2001;43:274.
Morota N., Sakamoto K., Kobayashi N. Traumatic cervical syringomyelia related to birth injury. Childs Nerv Syst. 1992;8:234.
Nelson K.B., Lynch J.K. Stroke in newborn infants. Lancet Neurol. 2004;3:150.
Noetzel M.J., Park T.S., Robinson S., et al. Prospective study of recovery following neonatal brachial plexus injury. J Child Neurol. 2001;16:488.
Onal C., Yakinci C., Kocak A., et al. Cervical hematomyelia: A rare entity in a neonate with cesarean section and surgical recovery. Pediatr Neurosurg. 2002;36:90.
Papaefthymiou G., Oberbauer R., Pendl G. Craniocerebral birth trauma caused by vacuum extraction: A case of growing skull fracture as a perinatal complication. Childs Nerv Syst. 1996;12:117.
Park S.H., Hwang S.K. Surgical treatment of subacute epidural hematoma caused by a vacuum extraction with skull fracture and cephalohematoma in a neonate. Pediatr Neurosurg. 2006;42:270.
Perrin R.G., Rutka J.T., Drake J.M., et al. Management and outcomes of posterior fossa subdural hematomas in neonates. Neurosurgery. 1997;40:1190.
Pohl D., Bittigau P., Ishimaru M.J., et al. N-Methyl-D-aspartate antagonists and apoptotic cell death triggered by head trauma in developing rat brain. Proc Natl Acad Sci USA. 1999;96:2508.
Pollina J., Dias M.S., Li V., et al. Cranial birth injuries in term newborn infants. Pediatr Neurosurg. 2001;35:113.
Rafay M.F., Cortez M.A., deVeber G.A., et al. Predictive value of clinical and EEG features in the diagnosis of stroke and hypoxic-ischemic encephalopathy in neonates with seizures. 2009.
Raju T.N., Nelson K.B., Ferriero D., et al. Ischemic perinatal stroke: summary of a workshop sponsored by the National Institute of Child Health and Human Development and the National Institute of Neurological Disorders and Stroke. Pediatrics. 2007;120:609.
Ramaswamy V., Miller S.P., Barkovich A.J., et al. Perinatal stroke in term infants with neonatal encephalopathy. Neurology. 2004;62:2088.
Redline R.W., Sagar P., King M.E., et al. Case records of the Massachusetts General Hospital. Case 12-2008. A newborn infant with intermittent apnea and seizures. N Engl J Med. 2008;358:1713.
Rehan V.K., Seshia M.M. Spinal cord birth injury—diagnostic difficulties. Arch Dis Child. 1993;69:92.
Ricci D., Mercuri E., Barnett A., et al. Cognitive outcome at early school age in term-born children with perinatally acquired middle cerebral artery territory infarction. Stroke. 2008;39:403.
Roach E.S., Golomb M.R., Adams R., et al. Management of stroke in infants and children: A scientific statement from a special writing group of the American heart association stroke council and the council on cardiovascular disease in the young. Stroke. 2008;39:2644.
Robertson C.L., Soane L., Siegel Z.T., et al. The potential role of mitochondria in pediatric traumatic brain injury. Dev Neurosci. 2006;28:432.
Rossitch E.Jr, Oakes W.J. Perinatal spinal cord injury: Clinical, radiographic and pathologic features. Pediatr Neurosurg. 1992;18:149.
Russo R.N., Crotty M., Miller M.D., et al. Upper-limb botulinum toxin A injection and occupational therapy in children with hemiplegic cerebral palsy identified from a population register: a single-blind, randomized, controlled trial. Pediatrics. 2007;119:e1149.
Sandberg D.I., Lamberti-Pasculli M., Drake J.M., et al. Spontaneous intraparenchymal hemorrhage in full-term neonates. Neurosurgery. 2001;48:1042. discussion 1048
Saucier D.M., Yager J.Y., Armstrong E.A., et al. Enriched environment and the effect of age on ischemic brain damage. Brain Res. 2007;1170:31.
Schatz A.M., Ballantyne A.O., Trauner D.A. A hierarchical analysis of block design errors in children with early focal brain damage. Dev Neuropsychol. 2000;17:75.
Schneider A.T., Kissela B., Woo D., et al. Ischemic stroke subtypes: a population based study of incidence rates among blacks and whites. Stroke. 2004;35:1552.
Schulzke S., Weber P., Luetschg J., et al. Incidence and diagnosis of unilateral arterial cerebral infarction in newborn infants. J Perinat Med. 2005;33:170.
Seghier M.L., Lazeyras F., Zimine S., et al. Visual recovery after perinatal stroke evidenced by functional and diffusion MRI: case report. BMC Neurol. 2005;26(5):17.
Shevell M.I., Silver K., O’Gorman A.M., et al. Neonatal dural sinus thrombosis. Pediatr Neurol. 1989;5:161.
Shroff M., Deveber G. Sinus thrombosis in children. Neuroimaging Clin N Am. 2003;13:115.
Simchen M.J., Goldstein G., Lubetsky A., et al. Do Factor v Leiden and antiphospholipid antibodies in either mothers or infants increase the risk for perinatal arterial stroke? Stroke. 2009;40:65.
Simon L., Perreaux F., Devictor D., et al. Clinical and radiological diagnosis of spinal cord birth injury. Arch Dis Child Fetal Neonatal Ed. 1999;81:F235.
Sirsi D., Nadiminti L., Packard M.A., et al. Apneic seizures: a sign of temporal lobe hemorrhage in full-term neonates. Pediatr Neurol. 2007;37:366.
Slaughter L., Egelhoff J., Balmakund T. Neurologic Outcome in Neonatal Temporal Lobe Hemorrhagic Venous Infarcts. J.Child Neurol. 2009;24:1236.
Smith A.B., Gupta N., Strober J., et al. Magnetic resonance neurography in children with birth-related brachial plexus injury. Pediatr Radiol. 2008;38:159.
Sran S.K., Baumann R.J. Outcome of neonatal strokes. Am J Dis Child. 1988;142:1086.
Sreenan C., Bhargava R., Robertson C.M. Cerebral infarction in the term newborn: Clinical presentation and long-term outcome. J Pediatr. 2000;137:351.
Statler K.D. Pediatric posttraumatic seizures: epidemiology, putative mechanisms of epileptogenesis and promising investigational progress. Dev Neurosci. 2006;28:354.
Staudt M., Grodd W., Gerloff C., et al. Two types of ipsilateral reorganization in congenital hemiparesis: A TMS and fMRI study. Brain. 2002;125:2222.
Stiles J., Stern C., Trauner D., et al. Developmental change in spatial grouping activity among children with early focal brain injury: Evidence from a modeling task. Brain Cogn. 1996;31:46.
Stiles J., Trauner D., Engel M., et al. The development of drawing in children with congenital focal brain injury: Evidence for limited functional recovery. Neuropsychologia. 1997;35:299.
Suppiej A., Franzoi M., Gentilomo C., et al. High prevalence of inherited thrombophilia in ‘presumed peri-neonatal’ ischemic stroke. Eur J Haematol. 2008;80:71.
Takekawa H., Tanaka H., Ogawa T., et al. Usefulness of echo-planar T2* susceptibility-weighted imaging for reliable diagnosis of cerebral venous sinus thrombosis. Intern Med. 2008;47:2101.
Taub E., Ramey S.L., DeLuca S., et al. Efficacy of constraint-induced movement therapy for children with cerebral palsy with asymmetric motor impairment. Pediatrics. 2004;113:305.
Tavarez L.A., Kottamasu S.R., Ezhuthachan S.G., et al. Neonatal skull depression: Review of four cases. J Perinatol. 1989;9:423.
Thorburne S.K., Juurlink B.H. Low glutathione and high iron govern the susceptibility of oligodendroglial precursors to oxidative stress. J Neurochem. 1996;67:1014.
Towbin A. Latent spinal cord and brain stem injury in newborn infants. Dev Med Child Neurol. 1969;11:54.
Towner D., Castro M.A., Eby-Wilkens E., et al. Effect of mode of delivery in nulliparous women on neonatal intracranial injury. N Engl J Med. 1999;341:1709.
Trauner D.A., Chase C., Walker P., et al. Neurologic profiles of infants and children after perinatal stroke. Pediatr Neurol. 1993;9:383.
Tsao P.N., Lee W.T., Peng S.F., et al. Power doppler ultrasound imaging in neonatal cerebral venous sinus thrombosis. Pediatr Neurol. 1999;21:652.
Uchil D., Arulkumaran S. Neonatal subgaleal hemorrhage and its relationship to delivery by vacuum extraction. Obstet Gynecol Surv. 2003;58:687.
Vachharajani A., Mathur A. Ultrasound-guided needle aspiration of cranial epidural hematoma in a neonate: Treating a rare complication of vacuum extraction. Am J Perinatol. 2002;19:401.
van Lookeren Campagne M., Verheul J.B., et al. Early evolution and recovery from excitotoxic injury in the neonatal rat brain: a study combining magnetic resonance imaging, electrical impedance, and histology. J Cereb Blood Flow Metab. 1994;14:1011.
Vannucci R.C., Christensen M.A., Yager J.Y. Nature, time-course, and extent of cerebral edema in perinatal hypoxic-ischemic brain damage. Pediatr Neurol. 1993;9:29.
van Ouwerkerk W.J., van der Sluijs J.A., Nollet F., et al. Management of obstetric brachial plexus lesions: State of the art and future developments. Childs Nerv Syst. 2000;16:638.
van Rooij L.G., de Vries L.S., van Huffelen A.C., et al. Additional value of 2-channel amplitude integrated EEG recording in full-term infants with unilateral brain injury. Arch Dis Child Fetal Neonatal Ed. 2009. epub Oct 8
Vialle R., Piétin-Vialle C., Vinchon M., et al. Birth-related spinal cord injuries: a multicentric review of nine cases. Childs Nerv Syst. 2008;24:79.
Vinchon M., Pierrat V., Tchofo P.J., et al. Traumatic intracranial hemorrhage in newborns. Childs Nerv Syst. 2005;21:1042.
Walther M., Juenger H., Kuhnke N., et al. Motor cortex plasticity in ischemic perinatal stroke: a transcranial magnetic stimulation and functional MRI study. Pediatr Neurol. 2009;41:171.
Wasay M., Bakshi R., Dai A., et al. Local thrombolytic treatment of cerebral venous thrombosis in three paediatric patients. J Pak Med Assoc. 2006;56:555.
Wasay M., Dai A.I., Ansari M., et al. Cerebral venous sinus thrombosis in children: A multicenter cohort from the united states. J Child Neurol. 2008;23:26.
Westmacott R., Askalan R., Macgregor D., et al. Cognitive outcome following unilateral arterial ischaemic stroke in childhood: effects of age at stroke and lesion location. Dev Med Child Neurol. 2009. Aug 20. Epub
Westmacott R., MacGregor D., Askalan R., et al. Late emergence of cognitive deficits after unilateral neonatal stroke. Stroke. 2009;40:2012.
Whitby E.H., Griffiths P.D., Rutter S., et al. Frequency and natural history of subdural haemorrhages in babies and relation to obstetric factors. Lancet. 2004;363:846.
Wong V.K., LeMesurier J., Franceschini R., et al. Cerebral venous thrombosis as a cause of neonatal seizures. Pediatr Neurol. 1987;3:235.
Wu Y.W., Lynch J.K., Nelson K.B. Perinatal arterial stroke: understanding mechanisms and outcomes. Semin Neurol. 2005;25:424.
Wu Y.W., Miller S.P., Chin K., et al. Multiple risk factors in neonatal sinovenous thrombosis. Neurology. 2002;59:438.
Wu Y.W., March W.M., Croen L.A., et al. Perinatal stroke in children with motor impairment: A population-based study. Pediatrics. 2004;114:612.
Yager J.Y., Thornhill J.A. The effect of age on susceptibility to hypoxic-ischemic brain damage. Neurosci Biobehav Rev. 1997;21:167.
Yager J.Y., Shuaib A., Thornhill J. The effect of age on susceptibility to brain damage in a model of global hemispheric hypoxia-ischemia. Brain Res Dev Brain Res. 1996;93:143.
Yager J.Y., Wright S., Armstrong E.A., et al. A new model for determining the influence of age and sex on functional recovery following hypoxic-ischemic brain damage. Dev Neurosci. 2005;27(2–4):112.
Yager J.Y., Wright S., Armstrong E.A., et al. The influence of aging on recovery following ischemic brain damage. Behav Brain Res. 2006;173:171.
Yates P.O. Birth trauma to the vertebral arteries. Arch Dis Child. 1959;34:436.