Aerosol Drug Therapy
After reading this chapter you will be able to:
Describe how particle size, motion, and airway characteristics affect aerosol deposition.
Describe how aerosols are generated.
List the hazards associated with aerosol drug therapy.
Describe how to select the best aerosol drug delivery system for a patient.
Describe how to initiate and modify aerosol drug therapy.
State the information patients need to know to self-administer drug aerosol therapy properly.
Describe how to assess patient response to bronchodilator therapy at the point of care.
Describe how to apply aerosol therapy in special circumstances.
Describe how to protect patients and caregivers from exposure to aerosolized drugs.
An aerosol is a suspension of solid or liquid particles in gas. Aerosols occur in nature as pollens, spores, dust, smoke, smog, fog, and mist.1 A primary function of the upper airway and respiratory tract is to protect the lungs from invasion by these aerosols. In the clinical setting, medical aerosols are generated with atomizers, nebulizers, and inhalers—devices that physically disperse matter into small particles and suspend them into a gas. Aerosols can be used to deliver bland water solutions to the respiratory tract (see Chapter 35) or to administer drugs to the lungs, throat, or nose for local and systemic effect. This chapter focuses on the principles of aerosol drug therapy.
The aim of medical aerosol therapy is to deliver a therapeutic dose of the selected agent (drug) to the desired site of action. The indication for any specific aerosol is based on the need for the specific drug and the targeted site of delivery.1 For patients with pulmonary disorders, administration of drugs by aerosol offers higher local drug concentrations in the lung with lower systemic levels compared with other forms of administration. Improved therapeutic action with fewer systemic side effects provides a higher therapeutic index.2
Characteristics of Therapeutic Aerosols
Particle Size
Aerosol particle size depends on the substance for nebulization, the method used to generate the aerosol, and the environmental conditions surrounding the particle.3 It is impossible to determine visually whether a nebulizer is producing an optimal particle size. The unaided human eye cannot see particles less than 50 to 100 µm in diameter (equivalent to a small grain of sand). The only reliable way to determine the characteristics of an aerosol suspension is laboratory measurement. The two most common laboratory methods used to measure medical aerosol particle size distribution are cascade impaction and laser diffraction. Cascade impactors are designed to collect aerosols of different size ranges on a series of stages or plates. The mass of aerosol deposited on each plate is quantified by drug assay, and a distribution of drug mass across particle sizes is calculated. In laser diffraction, a computer is used to estimate the range and frequency of droplet volumes crossing the laser beam.
Deposition
When aerosol particles leave suspension in gas, they deposit on (attach to) a surface. Only a portion of the aerosol generated and emitted from a nebulizer (emitted dose) may be inhaled (inhaled dose). A fraction of the inhaled dose is deposited in the lungs (respirable dose). Inhaled mass is the amount of drug inhaled. The proportion of the drug mass in particles that are small enough (fine-particle fraction) to reach the lower respiratory tract is the respirable mass. Not all aerosol delivered to the lung is retained, or deposited. A small percentage (1% to 5%) of inhaled drug may be exhaled. Whether aerosol particles that are inhaled into the lung are deposited in the respiratory tract depends on the size, shape, and motion of the particles and on the physical characteristics of the airways and breathing pattern. Key mechanisms of aerosol deposition include inertial impaction, gravimetric sedimentation, and brownian diffusion.1,3
Inertial Impaction
Inertial impaction occurs when suspended particles in motion collide with and are deposited on a surface; this is the primary deposition mechanism for particles larger than 5 µm. The greater the mass and velocity of a moving object, the greater its inertia, and the greater the tendency of that object to continue moving along its set path (Figure 36-1). When a particle of sufficient (large) mass is moving in a gas stream and that stream changes direction, the particle tends to remain on its initial path and collide with the airway surface.
Sedimentation
Sedimentation occurs when aerosol particles settle out of suspension and are deposited owing to gravity. The greater the mass of the particle, the faster it settles (Figure 36-2). During normal breathing, sedimentation is the primary mechanism for deposition of particles 1 to 5 µm. Sedimentation occurs mostly in the central airways and increases with time, affecting particles 1 µm in diameter. Breath holding after inhalation of an aerosol increases the residence time for the particles in the lung and enhances distribution across the lungs and sedimentation. A 10-second breath hold can increase aerosol deposition 10% and increase the ratio of aerosol deposited in lung parenchyma to central airway by fourfold.4
Diffusion
Figure 36-3 summarizes the relationships between particle size and aerosol deposition in the respiratory tract. The depth of penetration and deposition of a particle in the respiratory tract tend to vary with size and tidal volume (VT).5 With this knowledge, it may be possible to target aerosol deposition to specific areas of the lung by using the proper particle size and breathing pattern.
Aging
Aerosol particles can change size as a result of either evaporation or hygroscopic water absorption. The relative rate of particle size change is inversely proportional to the size of a particle, so small particles grow or shrink faster than large particles. Small water-based particles shrink when exposed to relatively dry gas. Aerosols of water-soluble materials, especially salts, tend to be hygroscopic, absorbing water and growing when introduced into a high-humidity environment.5
Particle size is not the only determinant of deposition. Inspiratory flow rate, flow pattern, respiratory rate, inhaled volume, ratio of inspiratory time to expiratory time (I : E ratio), and breath holding all influence where a particle of any specific size is deposited. The presence of airway obstruction is one of the greatest factors influencing aerosol deposition. It has been shown that total pulmonary deposition is greater in smokers and patients with obstructive airway disease than in healthy persons (Figure 36-4). Similarly, when inspiratory flow rates are constant, the deposition fraction of monodisperse aerosols increases with increased VT, length of respiratory (inspiratory) period, and particle size (Figure 36-5).
Quantifying Aerosol Delivery
One approach used to quantify aerosol deposition to the human body (in vivo) involves scintigraphy, in which a drug is “tagged” with a radioactive substance (e.g., technetium), aerosolized, and inhaled. A scanner (similar to scanners used in nuclear medicine) measures the distribution and intensity of radiation across the device and the patient’s head and thorax. The result is a radiation map of aerosol deposition in the upper airway, the lungs (central and peripheral airways), and the stomach. This information is used to calculate the percentage of drug retained by the device and delivered to various areas in the patient.6
A less direct approach relates the systemic pharmacokinetic profile of a drug delivered by aerosol to an assay of the drug in a patient’s blood or urine over time. This method does not estimate actual lung delivery, but it provides insight into systemic drug levels achieved after aerosol administration. Care must be taken to differentiate drug absorbed through the lungs from drug absorbed through the gastrointestinal tract. Simple laboratory, or in vitro, models, which simulate a range of VT values, inspiratory flow rates, I : E ratios, and respiratory rates, have been useful in predicting inhaled mass of drug and relative performance of nebulizers.7
Hazards of Aerosol Therapy
The primary hazard of aerosol drug therapy is an adverse reaction to the medication being administered (see Chapter 32). Other hazards to the patient include infection, airway reactivity, systemic effects of bland aerosols, drug concentration, and eye irritation. Care providers and bystanders risk these hazards as a result of exposure to secondhand aerosol drugs.
Infection
Aerosol generators can contribute to nosocomial infections by spreading bacteria by the airborne route.8 The most common sources of bacteria are patient secretions, contaminated solutions (i.e., multiple-dose drug vials), and caregivers’ hands. Offending organisms are primarily gram-negative bacilli, in particular, Pseudomonas aeruginosa and Legionella pneumophila (the cause of the highly virulent legionnaires’ disease).9
Various procedures can help reduce contamination and infection associated with respiratory care equipment. Guidelines from the U.S. Centers for Disease Control and Prevention (CDC) state that nebulizers should be sterilized between patients, frequently replaced with disinfected or sterile units, or rinsed with sterile water (not tap water) and air dried every 24 hours (see Chapter 4).
Airway Reactivity
Cold air and high-density aerosols can cause reactive bronchospasm and increased airway resistance, especially in patients with preexisting respiratory disease.10 Medications such as acetylcysteine, antibiotics, steroids, cromolyn sodium, ribavirin, and distilled water have been associated with increased airway resistance and wheezing during aerosol therapy. Administration of bronchodilators before or with administration of these agents may reduce the risk or duration of increased airway resistance.
The risk of inducing bronchospasm always should be considered when aerosols are administered. Monitoring for reactive bronchospasm should include peak flow measurements or percentage forced expiratory volume in 1 second (%FEV1) before and after therapy; auscultation for adventitious breath sounds; observation of the patient’s breathing pattern and overall appearance; and, most essential, communicating with the patient during therapy to determine the perceived work of breathing.11
Pulmonary and Systemic Effects
Preliminary assessment should balance the need versus the risk of aerosol therapy, especially among patients at high risk, such as infants, patients who are prone to fluid and electrolyte imbalances, and patients with atelectasis or pulmonary edema. For patients unable to clear their own secretions, suctioning or other airway clearance techniques may be indicated as an adjunct to aerosol therapy. Care must be taken to ensure that patients are capable of clearing secretions when the secretions are mobilized by aerosol therapy. Appropriate airway clearance techniques should accompany any aerosol therapy designed to help mobilize secretions (see Chapter 40).
Drug Concentration
During nebulization, the evaporation, heating, baffling, and recycling of drug solutions undergoing jet or ultrasonic nebulization increase solute concentrations.12 This process can expose the patient to increasingly higher concentrations of the drug over the course of therapy and result in a larger concentration of drug remaining in the nebulizer at the end of therapy. This increase in concentration usually is time-dependent; the greatest effect occurs when nebulization of medications occurs over extended periods, as in continuous aerosol drug delivery.
Eye Irritation
Aerosol administration via a face mask may deposit drug in the eyes and cause eye irritation. In very rare cases, anticholinergic medications (see Chapter 32) have been suspected to worsen preexisting eye conditions, such as forms of glaucoma. Caution should be exercised when a face mask is used during aerosol drug therapy. In addition, special mask designs that have been shown to reduce drug deposition in the eyes or mouthpieces should be considered for at-risk patients.13,14
Secondhand Exposure to Aerosol Drugs
Workplace exposure to aerosol may be detectable in the plasma of bystanders and health care providers. Repeated secondhand exposure to bronchodilators is associated with increased risk of occupational asthma. Institutions should develop and implement an occupational health and safety policy to minimize the risk of secondhand aerosol exposure for care providers and bystanders.15–17 Unless filters are placed in the expiratory limb, 40% of aerosol produced during mechanical ventilation is exhausted to the air of the intensive care unit.18 Implementation of an occupational health and safety policy could include using systems that introduce less aerosol to the atmosphere (pressurized metered dose inhalers [pMDIs], dry powder inhalers [DPIs], and breath-actuated nebulizers), filtering exhalation to contain aerosol, and using environmental controls.
Aerosol drug Delivery Systems
Effective aerosol therapy requires a device that quickly delivers sufficient drug to the desired site of action with minimal waste and at a low cost.19 Aerosol generators in use include pMDIs with or without spacers or holding chambers, DPIs, small and large volume (jet) nebulizers, hand-bulb atomizers (including nasal spray pumps), ultrasonic nebulizers (USNs), and vibrating mesh (VM) nebulizers as well as numerous emerging technologies.2
Clinicians are often exposed to competing and sometimes conflicting claims about the different delivery systems and may not be provided the information they need to select the correct system for a given situation. Because device selection can make the difference between successful and unsuccessful therapy, clinicians must have in-depth knowledge of the operating principles and performance characteristics of these various systems and how best to select and apply them.20
Metered Dose Inhalers
The pMDI is used to administer bronchodilators, anticholinergics, and steroids. More formulations of these drugs are available for use by pMDIs than for use with nebulizers. Properly used, pMDIs are at least as effective as other nebulizers for drug delivery. For this reason, pMDIs often are the preferred method for delivering bronchodilators to spontaneously breathing patients and patients who are intubated and undergoing mechanical ventilation.21
Most pMDIs are “press and breathe,” but there is increasing presence of a variation known as breath-actuated pMDIs. The basic components of pMDI are similar regardless of type, manufacturer, or active ingredient; commonly used pMDIs are shown in Figure 36-6.
A pMDI is a pressurized canister that contains the prescribed drug (a micronized powder or aqueous solution) in a volatile propellant combined with a surfactant and dispersing agent (Figure 36-7). When the canister is inverted (nozzle down) and placed in its actuator, or “boot,” the volatile suspension fills a metering chamber that controls the amount of drug delivered. Pressing down on the canister aligns a hole in the metering valve with the metering chamber. The high propellant vapor pressure quickly forces the metered dose out through this hole and through the actuator nozzle.
From their inception in the mid-1950s to the beginning of the twenty-first century, chlorofluorocarbons (CFCs) such as Freon were the propellants used in pMDIs. Manufacture of CFCs for most applications was prohibited because of the effect of these compounds on global warming, with a period of transition provided for pMDIs. A consortium of eight pharmaceutical companies developed hydrofluoroalkane (HFA)-134a to be more environment-friendly and possibly clinically safer than CFCs.22 Redesign of key components of the pMDI has resulted in improved performance.23
Before initial use and after storage, every pMDI should be primed by shaking and actuating the device to atmosphere one to four times (see label for the specific device). Without priming, the initial dose actuated from a new pMDI canister contains less active substance than subsequent actuations.20 This “loss of dose” from a pMDI occurs when drug particles rise to the top of the canister over time (“cream”). A reduction in emitted dose with the first actuation commonly occurs with a pMDI after storage, particularly with the valve pointed in the downward position. Loss of prime is related to valve design and occurs when propellant leaks out of the metering chamber during periods of nonuse (e.g., 4 hours). The result is reduced pressure and drug released with the next actuation.20 Improved designs of metering valves developed for use with HFA propellants reduce these losses. It is recommended that a single dose be wasted before the next dose is inhaled when a CFC pMDI has not been used for 4 to 6 hours. An HFA pMDI requires no wasting of dose for periods exceeding 2 days.
Breath-Actuated Pressurized Metered Dose Inhaler
A variation of a pMDI is a breath-actuated model, which incorporates a trigger that is activated during inhalation. This trigger theoretically reduces the need for the patient or caregiver to coordinate MDI actuation with inhalation.24 However, patients may stop breathing when the MDI is actuated (“cold Freon effect”) or have suboptimal inspiration. Evaluation of the efficacy of breath-actuated pMDIs in children younger than 6 years is limited, and their use should be restricted to older children and adults. Oropharyngeal deposition of steroids using these devices is still very high.
The Aerocount Autohaler is a flow-triggered pMDI developed and marketed by the 3M Corporation (St. Paul, MN) (Figure 36-8). The device is designed to eliminate the need for hand-breath coordination by automatically triggering in response to the patient’s inspiratory effort.24 To use the Autohaler, the patient cocks a lever on the top of the unit, which sets in motion a downward spring force. Using the closed-mouth technique, the patient draws through the mouthpiece. When the patient’s flow rate exceeds 30 L/min, a vane releases the spring, which forces the canister down and triggers the pMDI. In the United States, the Autohaler is available only with pirbuterol, a bronchodilator similar to albuterol. Current data indicate that the device reduces pharyngeal impaction and enhances lung deposition. A possible limitation of the device is that it can be breath actuated only. Patients experiencing an acute exacerbation of bronchospasm may be unable to generate sufficient flows to trigger the Autohaler. This theoretical concern has not been widely observed in clinical studies of patients with severe exacerbation of asthma receiving treatment in emergency departments. Nevertheless, caution may be appropriate in ordering breath-triggered pMDIs for small children and patients prone to severe levels of airway obstruction.
The Easihaler (GlaxoSmithKline, Philadelphia) is a breath-actuated pMDI that has been developed with a range of medications and is currently available in Europe and Canada. Release in the United States is anticipated in the near future. A new generation of pMDIs such as the Tempo (MAP Pharmaceuticals, Mountain View, CA) have been designed to be breath actuated with lower force of the plume exiting the mouthpiece, reducing oropharyngeal deposition and increasing lung dose (Figure 36-9).
Dose Counters
A serious limitation of pMDIs is the lack of a “counter” to indicate the number of doses remaining in the canister. After the number of label doses have been administered, the pMDI may seem to give another 20 to 60 doses, which may deliver little or no medications as the doses “tail-off.” Tail-off effect refers to variability in the amount of drug dispensed toward the end of the life of the canister. The result of tail-off is swings from normal to almost no dose emitted from one breath to the next with no reliable indicator to the user. Without a dose counter, there is no viable method to determine remaining drug in a pMDI other than manually keeping a log of every dose taken. The U.S. Food and Drug Administration (FDA) is requiring all new pMDIs to have a counter technology to track pMDI actuations remaining. Third-party dose counters may be added to older pMDI models but may not have the accuracy of built-in technology (Figure 36-10).
Factors Affecting Pressurized Metered Dose Inhaler Performance and Drug Delivery
Temperature
Decreased temperature (<10° C) has been shown to decrease the output of CFC pMDIs. Patients with cold air–induced bronchospasm who keep their pMDIs in outer coat pockets when outside in cold winter weather may receive only a small percentage of drug compared with that administered with the same pMDI at 25° C. This problem has been less serious with the newer HFA pMDIs.20
Timing of Actuation Intervals
Manufacturers recommend 30 seconds to 1 minute between actuations. When propellants are released, the device cools, changing aerosol output. The pause allows the device to return to room temperature and recover normal output. However, Fink and colleagues25 showed that pMDI output is similar at 15-second intervals. Very rapid actuation of multiple puffs per breath reduces inhaled drug per puff.
Aerosol Delivery Characteristics
Although pMDIs can produce particles in the respirable range (MMAD 2 to 6 µm),20 the initial velocity and dispersion of the aerosol plume generate larger particles that decrease in size as they leave the pMDI, resulting in approximately 80% of the dose leaving the actuator to impact and become deposited in the oropharynx. A significant proportion of this oropharyngeal deposition is swallowed and may be a factor in systemic absorption of some drugs. Pulmonary deposition ranges from 10% to 20% in adults and larger children (less in infants).26 The exact amount of drug delivered to an individual patient is unpredictable because of high variability between patients and because pMDI drug administration is technique-dependent.
Technique
The successful administration of aerosol drugs by pMDI is highly technique-dependent. Two-thirds of patients and health care professionals who should teach pMDI use do not perform the procedure properly.27 Box 36-1 outlines the recommended steps for self-administering a bronchodilator by simple pMDI. Thorough preliminary patient instruction can last 10 to 30 minutes and should include demonstration, practice, and confirmation of patient performance (demonstration pMDIs with placebo are available from manufacturers for this purpose). Repeated instruction improves performance; repeat instruction is done most appropriately with follow-up clinic or home visits. Demonstration and return demonstration must occur several times for best patient adherence to device use.
Most pMDI labels call for placing the mouthpiece between the lips. However, research has shown that positioning the outlet of the pMDI approximately 4 cm (two fingerbreadths) in front of the mouth improves lung deposition by decreasing oropharyngeal impaction.28 Holding the canister outside the open mouth (at two fingerbreadths) provides a space for the particles to decelerate while evaporating, allowing particle size to reduce to respirable size. Use of the open-mouth technique with a low inspiratory flow rate can result in a doubling of the dose delivered to the lower respiratory tract of an adult from approximately 7% to 10% to 14% to 20%. However, this technique is more difficult for patients to perform reliably than the closed-mouth technique. Although it may reduce oropharyngeal deposition, the technique has not been shown to improve the clinical response to pMDI bronchodilators.
The high percentage of oropharyngeal drug deposition with use of steroid pMDIs can increase the incidence of opportunistic oral yeast infection (thrush) and changes in the voice (dysphonia). Rinsing the mouth after steroid use can help avoid this problem, but most pMDI steroid aerosol impaction occurs deep in the hypopharynx, which cannot be easily rinsed with gargling. For this reason, steroid pMDIs should not be used alone but always in combination with a spacer or valved holding chamber. See Box 36-2 for instructions for determining dosage left in the pMDI.
Pressurized Metered Dose Inhaler Accessory Devices
Spacers and Holding Chambers
Basic concepts for spacer devices include (1) small volume adapters, (2) open tube designs, (3) bag reservoirs, and (4) valved holding chambers (Figure 36-11). More than a dozen different devices with volumes ranging from 15 to 750 ml have been developed over the past 30 years.
A spacer is a simple valveless extension device that adds distance between the pMDI outlet and the patient’s mouth. This distance allows the aerosol plume to expand and the propellants to evaporate before the medication reaches the oropharynx. Larger particles leaving the pMDI tend to impact on the spacer walls. In combination, this phenomenon reduces oropharyngeal impaction and increases pulmonary deposition. Proper use of a simple open-tube spacer still requires some hand-breath coordination because a momentary delay between triggering and inhaling the discharged spray results in a substantial loss of drug and reduced lung delivery. Exhalation into a simple spacer after pMDI actuation clears the aerosol from the device and wastes most of the dose to the atmosphere. This reduction in dose also occurs with small volume reverse-flow design spacers if there is no provision for “holding” the aerosol in the device.30
Holding chambers produce a finer, slower moving, more “respirable” aerosol with less impaction of drug in the oropharyngeal area (1% of dose) than simple spacers (10%) or a pMDI alone (80% of dose). Deposition after inhalation of a radiolabeled pMDI solution aerosol from the AeroChamber, compared with deposition from the same pMDI inhaled with the open-mouth technique, showed a 10-fold to 17-fold decrease in the amount of radioactivity deposited in the oropharyngeal-laryngeal area while a similar lung dose was maintained. This finding was true for both healthy subjects and patients with chronic obstructive pulmonary disease (COPD).31 The advantage of reduced oropharyngeal deposition is fewer side effects from steroid aerosols, as shown in numerous published clinical trials. If multiple actuations of one or more drugs are placed into a spacer, both the total dose and the respirable dose of drug available for inhalation are reduced. The extent of these losses may vary for different drugs and spacer designs.20
Even with a holding chamber, respirable particles containing drug settle out and become deposited within the device, causing a whitish buildup on the inner chamber walls. This residual drug poses no risk to the patient but may be rinsed out periodically. Drug output from plastic spacers has been shown to decrease owing to the presence of an electrostatic charge. With these devices, a buildup of material can be seen on the walls of the chamber. As more material builds up on the wall of the chamber, the charge is dissipated, and more drug is inhaled by the patient. Washing the chamber with water (without soap) causes the electrostatic charge to be reestablished, making the device less effective for the next few puffs, until the static charge in the chamber (which attracts small particles) is again reduced.32 Optimal technique is outlined in Box 36-3.
Use of conductive metal or nonelectrostatic plastic chambers or washing the plastic chamber periodically with deionizing detergent (liquid dishwashing soap) can overcome the loss of fine-particle mass owing to electrostatic charge and increase the inhaled mass from 20% to 50% of the emitted dose of the pMDI, even in children (Figure 36-12).32 The effect of washing the chamber with conventional dishwashing soap reduces this static charge for up to 30 days. All valved holding chambers and spacers should be cleaned regularly, typically monthly, as recommended by the manufacturer. Use of dilute liquid dishwashing soap, with or without rinsing, and allowing to air dry are recommended.
The addition of a one-way valve to convert an open tube into a reservoir for the aerosol, the incorporation of the actuator in the pMDI, the shape of the device, flow of air through the device, edge effects, masks, and manufacturing materials all affect aerosol characteristics and yield. The inhalation valve, which is used to contain the aerosol, also acts as a baffle to reduce oropharyngeal deposition. This valve must be able to withstand the initial pressure from the pMDI when the device is triggered to retain aerosol and have sufficiently low resistance to open readily when the user inhales, in particular, when the user is a child or an infant. Exhalation valves in a face mask attached to a spacer device must also provide low resistance. Issues of spacer volume, VT, frequency of breathing, and mechanical dead space between the spacer and mouth are of particular concern when these devices are used by children.33 Differences of twofold to threefold in the amount of drug available at the mouth have been measured among spacers used at the present time to treat infants. Clinicians should determine the delivery efficiencies of spacer devices before using the devices in the care of a particular population.
Accessory devices are used with either the manufacturer-designed boot that comes with the pMDI or with a “universal adapter” that triggers the pMDI canister. Different formulations of pMDI drugs operate at different pressures and have a different-sized orifice in the boot that is specifically designed by the manufacturer for use exclusively with that pMDI. The output characteristics of a pMDI change when an adapter with a different-sized orifice is used. With HFA pMDIs, the diameter of the actuator orifice is smaller, and the spray is predictably finer. When the HFA pMDI is used in an actuator designed for use with CFC pMDIs, output is reduced. When these HFA formulations are used with any particular spacer, it is important to know how comparable the available dose and particle size distribution are to the dose and particle size from an existing CFC pMDI.20
Dry Powder Inhalers
A DPI is typically a breath-actuated dosing system. With a DPI, the patient creates the aerosol by drawing air though a dose of finely milled drug powder with sufficient force to disperse and suspend the powder in the air. DPIs are inexpensive, do not need propellants, and do not require the hand-breath coordination needed for pMDIs. However, dispersion of the powder into respirable particles depends on the creation of turbulent flow in the inhaler. Turbulent flow is a function of the ability of the patient to inhale the powder with a sufficiently high inspiratory flow rate (Figure 36-13). In terms of both lung deposition and drug response, DPIs are as effective as pMDIs.34
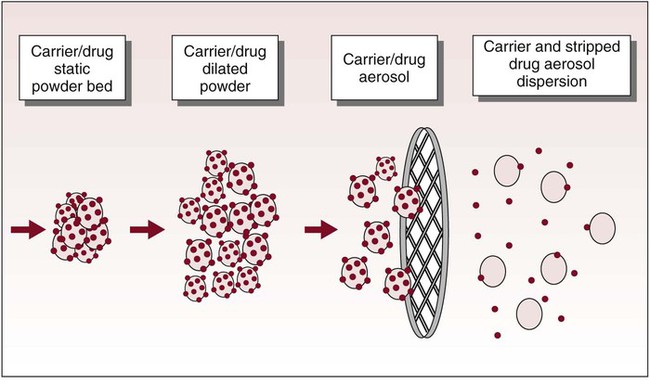