The Respiratory System
After reading this chapter you will be able to:
State the major developmental events of the respiratory system.
Describe how genes control lung development.
Describe the key elements of normal fetal circulation.
State what happens to the respiratory system at birth.
Describe the developmental events in the respiratory system that continue after birth.
Identify the main structures in the thorax and describe their functions.
Identify and describe the primary and accessory muscles of breathing.
Describe how the pulmonary and bronchial circulations are organized and their functions.
Describe how somatic and autonomic nervous systems connect to and control the lungs and respiratory muscles.
Identify the major structures of the upper respiratory tract and how they function.
Describe how the lungs are organized into lobes and segments and the airways that supply them with ventilation.
Describe how and why airways produce and move mucus.
Describe how the structures in the respiratory bronchioles and alveoli are organized.
Development of the Respiratory System
Based on cellular differentiation and tissue architecture, development of the respiratory system has been categorized into various stages.1 Figure 8-1 shows the various stages of lung development, and Table 8-1 summarizes the major developmental events in each phase. Respiratory development begins in the embryonic period on or about day 22 after fertilization, when a small mass of cells, the respiratory primordium, begins to develop near the ventral region of the fourth pharyngeal arch of the primitive pharynx. This mass of cells forms a pouchlike bud, the respiratory diverticulum, on about day 26 (Carnegie stage 9) that continues to grow to form a laryngotracheal tube (Figure 8-2). The laryngotracheal tube forms from a groove in the fourth pharyngeal pouch. From the laryngotracheal tube, a tracheal bud forms by the end of the fourth week of life. The dorsal portion of the primitive foregut develops into the primordial esophagus and is separated from the tracheal bud by the formation of a tracheoesophageal septum. During week 5 of development, the tracheal bud continues to develop and bifurcates into left and right primary bronchial buds. The laryngeal structures develop at the superior end of the laryngotracheal bud.
TABLE 8-1
Developmental Events of the Cardiopulmonary System
Gestational Age | Developmental Event |
Embryonic Period | |
20-22 days | Primordial pharyngeal arches form |
21-23 days | Primordial respiratory cells form on fourth pharyngeal pouch, primordial heart starts forming |
26th day | Laryngotracheal bud forms |
4th wk | Primitive trachea develops |
5th wk | Primary bronchial buds form, laryngeal structures develop |
Fetal Period | |
Pseudoglandular Stage | |
6th wk | Segmental and subsegmental bronchioles form |
7th wk | Diaphragm complete |
8th wk | Heart complete, fetal circulatory pattern begins to develop |
10th wk | Pulmonary lymphatic structures develop |
12th wk | Major arteries formed |
13th wk | Major airway epithelia and mucus-producing cells formed, smooth muscle cells developing |
14th wk | Principal arteries formed |
16th wk | Terminal bronchioles and associated pulmonary vessels form |
Canalicular Stage | |
16th-17th wk | Respiratory bronchioles and immature acini begin to form |
20th-24th wk | Type I and II pneumocytes begin to appear and replicate |
24th-26th wk | Pulmonary capillaries develop at surface of acinus, immature surfactant begins to appear in lung fluid |
Terminal Saccular Stage | |
26th wk-birth | Terminal saccules increase in number, pulmonary capillary density and proximity increase, type I and II pneumocytes continue to multiply, surfactant production increases, extrauterine life possible with support |
Alveolar Stage | |
32th-40th wk | Immature alveoli begin to form and increase in number; surfactant production matures |
40th week | 50 million immature alveoli formed |
Period After Birth | |
Birth | First breath and lung fluid cleared, adult circulatory pattern established |
8-10 yr | 470 million mature alveoli formed |
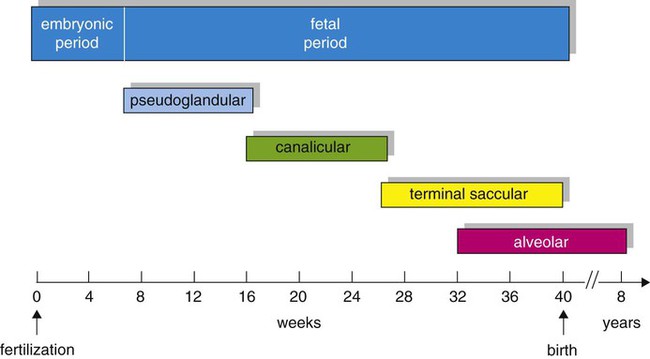
The developmental branching process of the airways and blood vessels of the lung is highly regulated by the timely activation of various genes in different locations. Of the approximate 22,000 genes in the human genome, about 40 are required for normal respiratory development.2–4 Table 8-2 lists many of these genes and the process in which they play an important role. The initial step in the development of the respiratory system is the localized expression of the NKX2-1 gene (also known as thyroid-specific transcription factor, TTF-1) in the anterior wall of the foregut, which stimulates the primary lung bud formation. Failure or mutation of the NKX2-1 gene can lead to failure of lung bud formation and various tracheoesophageal malformations.5 Lung bud elongation and the repetitive airway branching process is stimulated and directed by the highly choreographed expression of other key genes, including FGF10, FGFR2IIB, GATA-6, HNF-3, SPROUTY2, SHH, BMP-4, and NOGGIN as well as numerous other genes (see Table 8-2). Mutations of the FGF10 gene can result in tracheal development but fatal failure of further lung formation.6
TABLE 8-2
Genes Implicated in Pulmonary Development
Event | Factors and Genes |
Early lung bud development and airway branching | Thyroid transcription factor 1 (NKX2-1) |
FGF10 and FGF9 | |
FGFR2IIIB and FGFR2IIIC | |
GATA-6 | |
HNF-3 alpha and beta | |
Vitamin A (retinoic acid) and receptor | |
LEFTY 1 and 2 | |
Sprouty (SPROUTY2) | |
Sonic hedgehog (SHH) | |
Bone morphogenetic protein 4 (BMP-4) | |
NOGGIN | |
N-Acetylglucosaminyltransferase 1 | |
Secondary dichotomous branching | Transforming growth factor alpha and beta (TGF-α and TGF-β) |
WNT 5 and 7 | |
Platelet-derived growth factor (PDGF) | |
Alveolar development | PDGF |
Tropoelastin 1 | |
Fibrillin 1 | |
Cyclin-dependent kinase inhibitors (p57 and p21) | |
Type 1 cell alpha transmembrane protein | |
Ephrin B2 | |
Surfactant formation | Surfactant protein A, B, and C (SFTPA, SFTPB, and SFTPC) |
ATP-binding cassette subfamily (ABCA3) | |
NKX2-1 | |
HNF-3 | |
Pulmonary vascular development | Activin receptor–like kinases |
TGF | |
Vascular endothelial growth factors (VEGF) | |
Forkhead box transcription factors (Fox) | |
Integrin alpha forms | |
Caveolin 1 and 2 |
At approximately 6 weeks of development, lung and airway growth has the appearance of a glandular structure—hence the name of the second phase of development, the pseudoglandular stage (Figure 8-3). For the next 10 weeks, the growth and branching of the tracheobronchial tree and pulmonary vasculature continue, under the direction of the various genes described earlier, and culminate with formation of the terminal and respiratory bronchioles. The distinction between these two types of bronchioles is important. Terminal bronchioles, similar to bronchi and the trachea, are conducting airways only and do not participate in gas exchange with blood. Respiratory bronchioles have much more superficial capillaries and are capable of gas exchange with blood and become more elaborate as development continues.
Branching and dividing of the tracheobronchial tree occur in several ways as the result of differential gene expression. A single bud that develops off of an existing structure is termed a monopodial bud. Airways that divide into two or more airways do so through dichotomous branching. Most of the divisions of airways occur in a nonsymmetric fashion termed irregular dichotomous branching.7,8 The epithelial lining of the airways begins to differentiate into columnar epithelia in the proximal airways and differentiates into cuboidal epithelium in the more distal bronchioles (Figure 8-4, A). Development of cilia, mucous glands, and goblet cells occurs at this time, and these are found lining most of the conducting airways.
The third phase of development is termed the canalicular stage (see Figure 8-4, B). It begins at week 16 and continues until week 26. The canalicular stage overlaps with the pseudoglandular stage because the superior regions of the lung are developing slightly faster than the inferior regions. During this phase, primary changes include the development of two to four more generations of respiratory bronchioles from each terminal bronchiole, the formation of blind tubular alveolar ducts from each respiratory bronchiole, and greater blood vessel development. In the last several weeks of this stage, the region beyond each terminal bronchiole forms the functional structure called the acinus, the basic gas-exchanging unit of the lung. At this time, the two principal epithelial cell types that cover the gas exchange surface begin to appear, type I and type II pneumocytes. At the end of the canalicular period (24 to 26 weeks of gestation), the fetus, if born, is capable of sufficient gas exchange and is viable if supported with supplemental O2, ventilatory support, and surfactant administration.
During the fourth phase, the terminal saccular stage (see Figure 8-4, C), more terminal bronchioles and their associated acini form, and their structure continues to develop from 26 weeks to birth. The formation of the total number of terminal bronchioles is complete at the end of this phase.8 The cuboidal epithelia that line the blind tubules of the acinus continue to differentiate into rounded secretory cells (type II pneumocytes) and flatter squamous epithelial cells (type I pneumocytes). Mounting evidence shows that an important source of type I pneumocytes during both development and after lung injury are type II cells that can proliferate and differentiate.9,10 Capillaries continue to form near and bulge from the surface of the acinus. Although some type II pneumocytes form by 20 weeks’ gestation, they are in such small numbers and of such primitive function that their impact on lung function is marginal. From this point until birth, there is rapid proliferation of alveolar ducts and sacs, formed from the respiratory bronchioles. The type I pneumocytes of the saccule walls thin and elongate to cover the walls of this region. Type I cells become the primary gas-exchange cells in the lung with close approximation to developing pulmonary capillaries. Type II pneumocytes form and secrete the vital pulmonary surfactants that are necessary to alter surface tension and help keep the lungs inflated.
The development of mature alveoli, accompanied by capillary proliferation within the walls, marks the final phase of lung development and is known as the alveolar period (see Figure 8-4, D). This phase begins at about week 32 of gestation and continues for years after birth. During this phase, the terminal saccules develop pouchlike regions called alveoli in their walls that are hexagonal in shape. The process of alveolarization occurs through the formation of crests along the immature airway wall, which develop further into septa that lengthen into the terminal saccule lumen; this effectively divides up the terminal airspace and results in greater numbers of alveoli that enlarge to a mature state with time.
A full-term newborn infant has about 50 million alveoli, and the number continues to increase for about 2 to 3 years after birth.11,12 The alveoli are lined with type I and II pneumocytes covering the pulmonary capillaries that have formed just below the basement membrane.
Human pulmonary surfactant, which promotes lung inflation and protects the alveolar surface, begins to be produced around 24 to 25 weeks of development by type II pneumocytes. It is composed primarily of phospholipids, a small amount of protein (types SP-A, SP-B, and SP-C), and a trace of carbohydrates.13 Early research in pulmonary surfactants centered on the phospholipid components, mainly phosphatidylcholine (lecithin [L] and sphingomyelin [S]) and phosphatidylglycerol (PG). Quantification of these phospholipids (the L/S ratio and PG concentration) provides a predictive index of the lung maturity in a fetus before birth and the risks of the development of respiratory distress.14 An L/S ratio of 2 or more indicates a relatively low risk for the development of respiratory distress syndrome, whereas an L/S ratio of less than 1.5 is associated with a high risk.
Surfactant synthesis is regulated by numerous hormones and factors, including glucocorticoids, prolactin, insulin, estrogens, androgens, thyroid hormones, and catecholamines.15 Glucorticosteroid production increases at the end of gestation and stimulates receptors in type II pneumocytes to increase surfactant production and improve the L/S ratio. Various key genes are also associated with normal surfactant production (surfactant protein genes A, B, C, and D; surfactant protein A, B, C, and D; and an adenosine triphosphate (ATP)–binding cassette transporter, ABCA3), and their failure, owing to mutation, is linked with the development of respiratory distress syndrome and other pulmonary disorders.16
A distinctive function of the developing lung is the formation of relatively large amounts of fetal lung fluid that is passed into amniotic fluid. Fetal lung fluid is a unique combination of plasma ultrafiltrate from the fetal pulmonary microcirculation, components of pulmonary surfactant, and other fluids from pulmonary epithelial cells.7 This fluid is constantly produced and keeps the fetal lung inflated at a slight positive pressure with respect to amniotic fluid pressure; it is important in stimulating normal lung development.17 At term, the fetal lung is filled with about 40 ml, and fluid is produced at a rate that results in replacing it multiple times per day. Conditions that lead to reduced fetal breathing and amniotic fluid formation (oligohydramnios) are linked to incomplete inflation of the lung with fluid and poorly developed (hypoplastic) lungs.
Throughout the developmental period, lung growth is similar in male and female fetuses. There are differences, however. At birth, the lungs of male infants are, on average, larger and have a greater number of respiratory bronchioles than the lungs of female infants when adjusted for gestational age.18 When evaluating breathing efforts and surfactant production at 26 to 36 weeks of gestation, female fetuses have better developed lung function and are slightly less susceptible to the development of respiratory distress syndrome.19,20
Transition from Uterine to Extrauterine Life
Placental Structure and Function
Survival of the embryo and then fetus requires an effective circulatory interface with the circulation of the mother, which is provided by the placenta.21 Within 1 week of uterine implantation, vascular projections called chorionic villi arise from the aorta of the embryo and penetrate the uterine endometrium. As gestation proceeds, the villi increase in number and complexity; erode the endometrium; and create irregular pockets called intervillous spaces in the placenta, which fill with maternal blood. The maternal blood flowing through the intervillous spaces bathes the embryonic villi and creates an O2-rich and nutrient-rich blood environment. As gestation progresses, the villi decrease in size but increase in number and complexity, resulting in an increased surface area that is essential for adequate maternal-fetal gas, nutrient, and waste exchange.
The maternal uterine tissues and blood vessels of the fetal chorionic villi make up the bulk of the placenta. Figure 8-5 shows a cross section of a well-developed placenta. Maternal blood flows into the intervillous space through the spiral arteries, whereas fetal blood is supplied to the villi from two umbilical arteries. Maternal and fetal blood come into close proximity but remain separated by an embryonic membrane that permits the exchange of O2, CO2, water, ions, various metabolic molecules, and hormones. Some maternal cells do move into fetal blood, and some fetal cells move into maternal blood and have been found in various maternal organs.
Various factors enhance the delivery of O2 to fetal tissues. The partial pressure gradient for O2 between maternal blood and fetal blood drives the diffusion of O2 into fetal blood within the chorionic villi capillaries.22,23 The maternal arterial blood has a partial pressure of O2 (PaO2) of approximately 100 mm Hg, which mixes with the blood in the intervillous space to produce a mean PO2 of approximately 50 mm Hg. Fetal blood that enters the villi has a PO2 of approximately 19 mm Hg, and the pressure gradient between maternal and fetal blood PO2 (50 − 19 = 31 mm Hg) causes O2 to diffuse into fetal blood. Blood leaving the villi and entering the umbilical vein has a PO2 of approximately 30 mm Hg. Table 8-3 summarizes the normal gas and acid-base values in normal fetal umbilical arteries and veins and maternal intervillous blood. Assessment of umbilical vein blood gas data shortly after birth is a method of determining the degree of fetal asphyxiation during the birth process.
TABLE 8-3
Approximate Normal Values of Blood Gases and Acid-Base in Fetal and Maternal Blood
Value | Maternal Intervillous Blood | Fetal Umbilical Artery Blood | Fetal Umbilical Venous Blood |
pH | 7.38 | 7.36 | 7.39 |
PCO2 (mm Hg) | 42 | 47 | 43 |
PO2 (mm Hg) | 50 | 19 | 30 |
The O2 content and delivery by fetal blood are almost the same as adult blood despite the much lower PO2; this is due to several factors, including relatively higher content of hemoglobin (18 g/dl) and hematocrit (54%) in fetal blood and the presence of fetal hemoglobin (HbF), which has an increased affinity for O2 and a more pronounced Bohr effect (reduced oxyhemoglobin affinity with acidosis) to enhance O2 release.23 Figure 8-6 illustrates how the increased O2 affinity is manifested by a leftward shift of the fetal oxyhemoglobin dissociation curve. The P50 (PO2 that saturates 50% of the hemoglobin) is 6 to 8 mm Hg less than the P50 for adult hemoglobin (HbA), which indicates the degree of the shift toward higher affinity. At birth, approximately 70% of circulating hemoglobin is HbF. HbA gradually replaces HbF during the first 6 months of extrauterine life as HbA genes in bone marrow switch on and HbF genes in the liver (major site of fetal erythrocyte development) are switched off.
Fetal Circulation
Fetal circulation is different than the circulation of the neonate after birth.24 Three important bypass pathways function in the developing fetus to enhance the flow of blood to the developing organs: ductus venosus, ductus arteriosus, and foramen ovale. Oxygenated blood from the placenta is carried in the umbilical vein back to the fetal circulation via the hepatic circulatory system (Figure 8-7). Approximately one-third of this blood flows to the lower trunk and extremities. The other two-thirds flows through the ductus venosus, which bypasses the liver’s circulation and flows to the inferior vena cava. This better oxygenated blood in the inferior vena cava mixes with the venous blood returning from the lower trunk and extremities and enters the right atrium. Approximately 50% of this blood is shunted from the right atrium into the left atrium through an opening in the interatrial septum called the foramen ovale. Left atrial blood flows to the left ventricle and then to the ascending aorta, where it continues on to the brain, brachiocephalic trunk, and descending aorta. Venous blood from the superior vena cava is directed downward through the right atrium into the right ventricle and then into the main pulmonary artery.
Cardiopulmonary Events at Birth
Various mechanisms work together to reduce and clear the amount of lung fluid at birth in preparation for air inflation.25 Days before birth, the epithelia of the lung stop the production of lung fluid. The lung fluid is actively absorbed back into the fetal circulation. Most of the active lung water absorption is facilitated by active sodium channel activity that is stimulated by fetal and maternal thyroid hormones, glucocorticoids, and epinephrine and increasing fetal lung and blood O2 content. In addition, some evidence suggests that the water channel aquaporin is also active in this process.26 During normal vaginal delivery, approximately one-third of the lung fluid is cleared through compression of the thorax in the birth canal. The pulmonary capillaries and lymphatics clear the remaining fluid.
The newborn infant is stimulated by new tactile and thermal stimuli, all of which stimulate breathing. In addition, as placental gas transfer is suddenly interrupted, the newborn quickly becomes hypoxemic, hypercapnic, and acidotic. This situation triggers strong inspiratory efforts (Figure 8-8). At first, no air enters the newborn lung until the transpulmonary pressure gradient exceeds 40 cm H2O. As lung volume increases in a stepwise fashion with each breath, increasingly less pressure is needed to overcome the opposing forces. The volume trapped in the lung stabilizes quickly and is crucial to adequate gas exchange.
Figure 8-9 summarizes the major cardiopulmonary changes that occur during the transition from a fluid-filled lung to an air-filled lung. As the lung expands with air, and gas exchange starts within the lung, pulmonary blood PO2 increases, PCO2 decreases, and pH increases; this results in pulmonary vasodilation, lower pulmonary vascular resistance, and constriction of the ductus arteriosus, which facilitates greater blood flow through the pulmonary circulation. Ductus arteriosus closure is stimulated further by the loss of maternal prostaglandins. The combination of increasing alveolar air content and constriction of the ductus arteriosus promotes progressive improvement in the matching of ventilation and blood flow, which increases the PO2 and decreases the PCO2 of blood leaving the lungs. After the clamping of the umbilical cord, cessation of umbilical and placental blood flow causes closure of the ductus venosus and a rapid increase in systemic vascular resistance. As systemic vascular resistance increases, left-sided heart pressures increase. Left atrial pressures also increase as a result of increased pulmonary blood flow that returns from the lungs. With left-sided heart pressures now higher than right-sided pressures, the foramen ovale closes.
Postnatal Lung Development
Upper Airway
Figure 8-10 shows the relative differences of the upper airway in relation to body size in an infant and an adult. The greater relative weight of the head can cause acute flexion of the cervical spine in infants with poor muscle tone. Infant neck flexion causes acute airway obstruction. Although the head is larger, an infant’s nasal passages are proportionately smaller than those of an adult. In addition, the infant’s jaw is much rounder, and the tongue is much larger relative to the size of the oral cavity.27 These anatomic differences increase the likelihood of airway obstruction when an infant becomes unconscious and loses muscle tone.
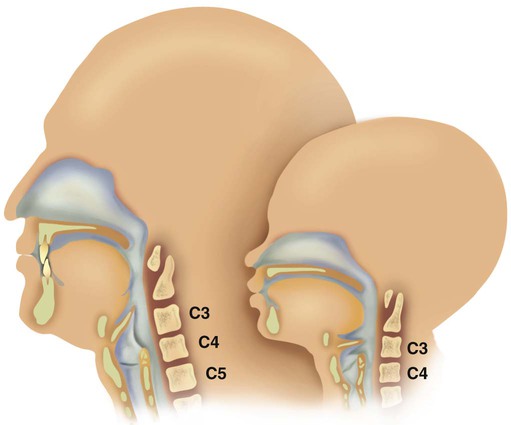
Most infants breathe through the nose. However, most term newborn infants shift to oral breathing in response to nasal occlusion and hypoxia.28 As normal infants mature, they begin to use the oral breathing route more and are more capable of shifting to oral breathing when nasal obstruction is present.29 At approximately 4 to 5 months of age, most infants are capable of full oral ventilation.
A newborn’s larynx lies higher in the neck compared with the larynx of an adult, with the glottis located between C3 and C4, and is more funnel-shaped than that of an adult. In a child, the narrowest region of the upper airway is through the cricoid cartilage, rather than the glottis, as it is in adults. The epiglottis of an infant is longer and less flexible than the epiglottis of an adult and lies higher and in a more horizontal position. During swallowing, the infant’s larynx provides a direct connection to the nasopharynx. This connection creates two nearly separate pathways, one for breathing and one for swallowing, allowing infants to breathe and suckle at the same time. Anatomic descent of the epiglottis begins at to 3 months of age. Mechanical and chemical irritant laryngeal reflexes develop at birth and can initiate protective laryngeal closure; these reflexes can trigger prolonged apnea in some and may be a cause of sudden infant death syndrome.30 In addition, infections in this area or repeated attempts at intubation or suctioning can easily cause swelling and obstruction of this area.
The large conducting airways of infants are shorter and narrower than the airways of adults. The normal newborn trachea is approximately 5 to 6 cm long and 4 mm in diameter, whereas in small preterm infants, it may be only 2 cm long and 2 to 3 mm wide. Because of the smaller airways, a newborn’s anatomic dead space is proportionately smaller than the anatomic dead space of an adult, being approximately 1.5 ml/kg of body weight. Figure 8-11 compares the tracheal anatomy in an adult and a newborn. The main stem bronchi branch off from the trachea in the infant at less acute angles than in the adult. However, similar to adults, the right main stem bronchus of the infant is still more in line with the trachea, which promotes right main stem intubation when airways or suction catheters are inserted to deeply. Mean airway diameter, from main bronchi to respiratory bronchioles, increases about two to three times from birth to adulthood.31
Lower Airway and Alveoli
The human lung continues to develop alveoli for years until it reaches a stable stage, at which the total number has increased to approximately 480 million alveoli.32 All development is generally complete by 10 years of age with most occurring in the first postnatal years.33 This development largely occurs by the formation of increasing numbers of septa in the terminal airspaces that continue to subdivide the airspace into shallow immature alveoli. These immature alveoli enlarge in size and undergo further refinement of pulmonary capillaries over the ensuing months and years.34 By adulthood, the alveolar-capillary membrane has a gas exchange surface area of approximately 140 m2.35
It was previously thought that the above-described alveolar development process ended several years after birth. However, numerous studies in various mammals have shown that compensatory lung growth can rapidly occur in the lung when part or all of the other lung is removed.36–38 Stem cell activation in the lungs, in response to gene and mechanical stretch, appears to be responsible for alveolar development well into adulthood after loss of lung tissue.39
Development of Vascular, Lymphatic, and Nervous Systems
The basic architecture of the pulmonary circulation is complete at birth. The main pulmonary trunk arises from the right ventricle and divides into left and right pulmonary arteries that supply each lung. These arteries divide further to form direct or conventional arteries and supernumerary arteries. Conventional arteries follow the airway branching, whereas supernumerary arteries follow an irregular pattern that allows substantial collateralization of flow between different regions of lung. Both types of pulmonary arteries come together to supply blood to large clusters of alveoli that are supplied by a single bronchiole. Most of the growth in the vascular system that occurs after birth includes further smooth muscle growth within the walls of arteries and arterioles and greater density and refinement of the arterioles and capillaries in the distal airway region.33,34
Chest Wall Development, Diaphragm, and Lung Volume
The thoracic wall in infants is more compliant, and their muscles are less developed than the muscles of adults and provide little structural support. The infant thoracic cage is also more boxlike, with the ribs being horizontally oriented or elevated (Figure 8-12). In addition, the diaphragm inserts into the thoracic cage in a horizontal plane, which decreases the effective ability to enlarge the thorax.
Respiratory System in the Adult
Surface Features of the Thorax
Thoracic shape and dimension vary from individual to individual and are linked to age, gender, and race. At birth, the thorax has a smaller transverse dimension, which widens with the onset of walking. Thoracic size and volume continue to increase throughout childhood and especially during the adolescent growth spurt. However, development of the thorax and lung volume is not equal in both sexes. When evaluating lung size and volume throughout puberty and into adulthood, boys and men are consistently found to have larger lungs than age-matched and height-matched girls and women.40 Some races have a proportionately larger thorax-to-height ratio than others. In females, the location of the nipple varies with the size and shape of the breast. In males, the nipple is usually located in the midclavicular line at the level of the fourth intercostal space.
Imaginary lines are commonly used to establish reference points and identify landmarks on the thorax. These lines and points help identify the location of underlying structures and the location of abnormal findings. On the anterior chest, the midsternal line divides the thorax into equal halves. The left and right midclavicular lines are parallel to the midsternal line. These are drawn through the midpoints of the left and right clavicles (Figure 8-13). The midaxillary line divides the lateral chest into equal halves. The anterior axillary line is parallel to the midaxillary line. It is situated along the anterolateral chest. The posterior axillary line is also parallel to the midaxillary line. It is located on the posterolateral chest wall (Figure 8-14). Three imaginary vertical lines are located on the posterior thorax. The midspinal line divides the posterior chest into two equal halves. The left and right midscapular lines are parallel to the midspinal line. They pass through the inferior angles of the scapulae in a relaxed upright subject (Figure 8-15).
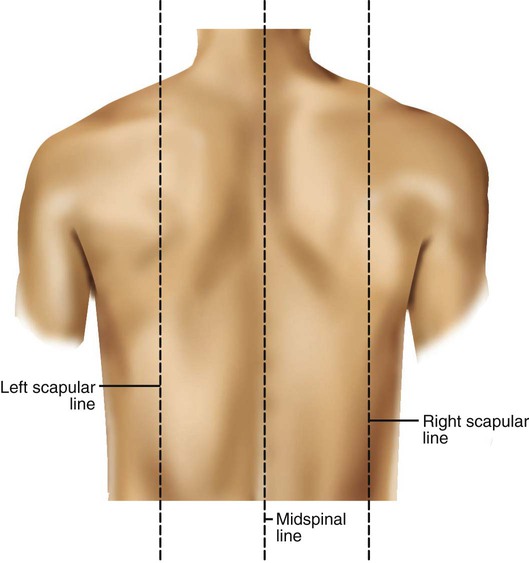
Components of the Thoracic Wall
The thoracic cavity is formed by the tissues of the chest, upper back, and diaphragm.41 It is a cone-shaped cavity that houses the lungs and the contents of the mediastinum (Figure 8-16). It functions to protect the vital organs within and is capable of changing shape to enable air to be moved into and out of the lungs. The thoracic cavity is formed from epithelial, connective, and muscle tissues.
The various parts of the thoracic wall are shown in Figure 8-17. The outer covering of the thorax is formed by the integumentary system, which includes skin, hair, subcutaneous fat, and breast tissues. Skin is a composite of an outer epidermis and an inner connective tissue layer called the dermis. Below the dermis is a layer of subcutaneous fat. Skeletal muscle, encased in a layer of connective tissue called fascia, is found under the subcutaneous fat. Skeletal muscle tissue forms the various muscles of the chest and back and lies over and between the ribs. The ribs of the rib cage lie in the inner portion of the thoracic wall. The inner layer of the thoracic wall is lined with a serous membrane called the parietal pleura. It is apposed by another serous membrane called the visceral pleura, which covers the lung. A thin, fluid-filled pleural space forms between the parietal and visceral pleural membranes.
The rigidity of the thorax is provided by the bone tissue of the rib cage. The bony parts of the rib cage include the sternum, ribs, thoracic vertebral bones, scapula, and clavicle (Figure 8-18). The sternum is a long, vertical flat bone found on the anterior side that is composed of three bones: the manubrium, the body (or gladiolus), and the xiphoid process. The superior edge of the manubrium forms a shallow depression that is known as the suprasternal (or jugular) notch. The fused connection between the manubrium and the body is known as the sternal angle; it is also known as the angle of Louis. The sternal angle is an external marker of the point where the trachea divides into the left and right main stem bronchi. A cartilaginous joint called the costal cartilage is on the lateral edges of the manubrium and sternal body and forms the attachment between the ribs and sternum. This joint allows the rib cage to bend and permits the thorax to increase and decrease in size.
The rib cage is formed by 12 pairs of ribs.41 Rib pairs 1 through 7 are known as the true ribs because they are attached directly to the sternum. The first ribs and the upper sternum form the opening into the thorax that is called the thoracic inlet, or operculum. Ribs 8 through 12 are called false ribs because they are either indirectly attached to the sternum or not attached at all. The vertebrochondral ribs include rib pairs 8, 9, and 10, which are indirectly attached to the sternum through a common cartilaginous strap. Rib pairs 11 and 12 are called floating ribs because they are not attached to the sternum. Each rib has a sternal end; a long, curved, and relatively flat body; and a head that articulates with the thoracic vertebrae (Figure 8-19). Intercostal muscles lie between the ribs and hold them together. Just below each rib is a thoracic artery, vein, and nerve that supply blood flow and nerve communications to that region of the chest wall (see Figure 8-17).
The upper and lateral regions of the thorax house the bones of the pectoral girdles. The pectoral girdle on each side is formed by the clavicle and scapula.41 The scapula forms the socket for the shoulder joint and is stabilized or moved by skeletal muscles of the upper back. The clavicle supports and stabilizes the shoulder joint through a flexible attachment to the manubrium of the sternum.
Rib Movement
The various ribs move in different ways, and some may move more than others at different times. The first rib moves slightly, raising and lowering the sternum. Its slight motion increases the anteroposterior diameter of the chest. This action is not used during quiet breathing and becomes active only under conditions that require increased ventilation or deep breathing. Ribs 2 through 7 move simultaneously about two axes (Figure 8-20). As each rib rotates about the axis of its neck, its sternal end rises and falls. This movement increases the anteroposterior thoracic diameter in what is commonly referred to as a “pump handle”–like motion. At the same time, the rib moves about its long axis from its angle at the sternum. This motion causes the middle part of the rib to move up and down in what is commonly described as a “bucket handle.” The compound action of ribs 2 through 7 changes both the anteroposterior and the transverse dimensions in an upward and outward motion. Ribs 8 through 10 rotate in a pattern similar to that of ribs 2 through 7. However, elevation of the anterior ends of these ribs produces a small backward movement of the lower sternum that slightly reduces the thoracic anteroposterior diameter. Outward rotation of the middle section of these ribs increases the transverse diameter of the thorax. Ribs 11 and 12 participate in changing the contour of the chest in a minor way as they are pulled upward and outward in a “caliper”-like motion.
Respiratory Muscles
Changes in thoracic cavity dimension during breathing are the product of tension developed by various skeletal muscles.42 Collectively, these muscles are known as the respiratory muscles; their origins, insertions, somatic nervous supply, and actions are summarized in Tables 8-4 and 8-5. The diaphragm and intercostal muscles are the primary muscles of ventilation. They are active both while at rest and when the individual exhibits stress-induced increases in breathing. The accessory muscles of ventilation assist the diaphragm and intercostal muscles when ventilatory demand increases. The scalene, sternocleidomastoid, pectoral, and abdominal wall muscles are the predominant accessory muscles. Other abdominal and chest wall muscles may also function as accessory muscles.
TABLE 8-4
Respiratory Muscles That Expand the Thorax During the Inspiratory Phase
Muscle | Origin | Insertion | Innervation | Action |
Diaphragm | Xiphoid process, lower lateral ribs, lumbar vertebra | Central tendon of dome | Phrenic nerves (C3-5) | Diaphragm moves downward, abdominal wall forced outward |
External intercostals | Upper ribs | Lower ribs | Intercostal nerves (T1-12) | Lift ribs upward |
Scalene | Lower 5 cervical vertebrae | Ribs 1 and 2 | Cervical nerves (C5-8) | Lifts ribs 1 and 2 |
Sternocleidomastoids | Manubrium and clavicle | Mastoid process of occipital bone | Accessory nerves (cranial nerve XI) | Lift sternum |
Trapezius | Occipital bone, C7-T12 vertebrae | Scapula and clavicle | Accessory nerves (cranial nerve XI) | Stabilizes head |
Pectoralis minor | Anterior region of ribs 3-5 | Scapula | Pectoral nerves (C6-8) | Lifts upper ribs |
Pectoralis | Clavicle and sternum | Humerus | Pectoral nerves (C5-C8) | Lifts sternum |
TABLE 8-5
Respiratory Muscles That Compress the Thorax During the Expiratory Phase
Muscle | Origin | Insertion | Innervation | Action |
Internal intercostals | Lower ribs | Upper ribs | Intercostal nerves (T1-12) | Pull ribs down |
External oblique | Anterior lower 8 ribs | Linea alba and iliac crest | Lower intercostal and iliohypogastric nerves (T7-12) | Pulls abdominal wall inward |
Internal oblique | Lumbar vertebrae, iliac crest, and inguinal ligaments | Costal region of ribs and pubis | Lower intercostal and iliohypogastric nerves (T10-12 and L1) | Pulls abdominal wall inward |
Transverse abdominis | Costal region of lower ribs, iliac crest, and inguinal crest | Linea alba | Lower intercostal and iliophypogastric (T7-L1) | Pulls abdominal wall inward |
Rectus abdominis | Costal region and ribs 5-7 | Pubis | Lower intercostal and iliophypogastric (T7-12) | Pulls abdominal wall inward |
Serratus anterior | Costal region of upper 8 ribs | Scapula | Long thoracic nerves (T5-7) | Compresses thorax when arm is stabilized |
Serratus, posterior superior | Lower cervical and upper thoracic vertebrae | Posterior ribs 2-5 | Intercostal nerves | Pulls ribs downward |
Serratus, posterior inferior | Lower thoracic and upper lumbar vertebrae | Posterior ribs 9-12 | Thoracic nerves | Pulls ribs downward |
Latissimus dorsi | Lower thoracic, lumbar, sacral vertebrae, ilium, and lower ribs | Humerus | Thoracodorsal nerve (C6-8) | Compresses thorax when arm is stabilized |
The diaphragm is a thin, musculotendinous, dome-shaped structure that separates the thoracic and abdominal cavities (Figure 8-21).43 It originates from the chest and abdominal wall and converges in a central tendon at the top of its dome. The posterior portion arises from the first three lumbar vertebrae. The lateral costal portions arise from the inner surface of ribs 7 through 12 and transverse abdominal muscles on each side. The anterior portion arises from the inner surface of the xiphoid process of the sternum. The best estimates of muscle fiber composition in the adult human diaphragm indicate that there are about 55% slow oxidative–type, 21% fast oxidative–type, and 24% fast glycolytic–type muscle fibers.44 These findings coupled with an abundant blood supply throughout the breathing cycle help to explain in part why the diaphragm is so highly aerobic and fatigue-resistant compared with other skeletal muscles and more capable of long-term rhythmic contraction.
In an upright position and with the diaphragm relaxed, the liver forces the dome of the right hemidiaphragm upward approximately 1 cm higher than the left hemidiaphragm at the end of a quiet exhalation. The highest portion of the right dome sits at the eighth or ninth thoracic vertebra posteriorly and at the fifth rib anteriorly. The left diaphragmatic dome sits at the ninth or tenth thoracic vertebra posteriorly and the sixth rib anteriorly. Movements of the hemidiaphragms are synchronous in healthy subjects. When lying down in a supine position, the weight of the abdominal contents forces the diaphragm farther up into the thoracic cavity. During quiet breathing, the diaphragm is responsible for approximately 75% of the change in thoracic volume.45 When the muscle fibers of the diaphragm are tensed during inspiration, the dome of the diaphragm is pulled down 1 to 2 cm; this results in enlargement of the thoracic cavity and compression of the abdominal contents. During maximal inspiration, the diaphragm can be pulled down approximately 10 cm. Exhalation results when diaphragmatic tension decreases, and the diaphragm returns to its relaxed position.
Functionally, the diaphragm is divided into a right and a left hemidiaphragm. Each hemidiaphragm is innervated by a phrenic nerve that arises from branches of spinal nerves C3, C4, and C5.43 Spinal cord injuries at or above the level of the third cervical vertebrae result in diaphragmatic paralysis. In this situation, the individual has lost all nervous control of the respiratory muscles and is unable to breathe. Unilateral phrenic nerve injury or disease to one side can spare the other nerve and permit unilateral ventilation.
The accessory muscles of inspiration include various muscles in the neck, chest, and upper back. Eleven pairs of intercostal muscles are found between the ribs.46 The external intercostal muscles (Figure 8-22) originate on the upper ribs and attach to the lower ribs. The fibers of these muscles run at an oblique angle between the ribs. When they generate tension, they lift the ribs upward and cause the thoracic cavity to enlarge the thorax (Hamberger mechanism). They receive nerve signals from the intercostal nerves that arise from thoracic spinal nerves (T1-12). They are more active during the inspiratory phase of forceful breathing and are thought to play a role in stabilizing excessive rib motion during forceful breathing.47
Three pairs of scalene muscles (scalenus anterior, scalenus medius, and scalenus posterior) arise from the lower five or six cervical vertebrae and insert on the clavicle and first two ribs (Figure 8-23). They lift the upper chest when active. The scalene muscles are slightly active during resting inhalation and become more active with forceful inspiration, especially when ventilatory demands increase.48 Such instances may occur in healthy subjects during exercise or in patients who have pulmonary disease. In healthy subjects, inspiratory efforts against a closed glottis or obstructed airway activate the scalene muscles. When alveolar pressure decreases to −10 cm H2O, scalene muscles are active in all subjects. The scalene muscles are largely inactive during expiratory efforts but can become active to fixate the ribs as abdominal muscles contract during forceful exhalation such as coughing.
Sternocleidomastoid muscles (Figure 8-24) originate from the manubrium and clavicle and insert on the mastoid process of the temporal bone. Normally, this muscle flexes and rotates the head and is active during shoulder shrugging. When the head is held in an upright position by tensing the trapezius muscle of the upper back and neck, the sternocleidomastoid muscles can function to lift the upper chest. They receive nerve impulses from branches of the accessory nerves (cranial nerve XI) and cervical nerves C1 and C2. These muscles are active during forceful inspiration and become visible as thick bands on either side of the neck during the inspiratory phase in an individual who is in respiratory distress. This motion increases the anteroposterior diameter of the chest.49
The major and minor pectoralis muscles are broad fan-shaped muscles of the upper anterior chest (Figure 8-25). The pectoralis major originates on the humerus and inserts onto the clavicle and sternum. The pectoralis minor originates from the anterior region of the ribs 3 through 5 and inserts onto the scapula. When these muscles receive impulses from the pectoral nerves, they normally function to adduct the arms in a hugging motion. They are also capable of generating some anterior thoracic lift when the arms are braced on a surface in front of a subject. Individuals who have chronic shortness of breath often use these muscles by sitting in a “tripod” position. This position is generated by sitting upright and leaning forward with both arms braced on a table or other stationary object.
The trapezius muscles are flat triangular muscles that are located on the upper back and neck (Figure 8-26). They arise from the occipital bone, seventh cervical vertebra, and all of the thoracic vertebrae. They insert onto the scapulae and lateral third of the clavicles. Their action is to rotate the scapulae, lift the shoulders, and flex the head up and back. They become active during forceful inspiration by helping to brace the head and allowing the sternocleidomastoid muscles to lift the thorax.
The accessory muscles of exhalation become active during forceful breathing (see Table 8-5). Generally, these muscles act to compress the thoracic cavity and facilitate exhalation. The internal intercostal muscles (see Figure 8-22) lie between the ribs and just behind the external intercostal muscles. They originate along the inferior border of the upper ribs and insert into the superior border of the lower ribs. The muscle fibers of the internal intercostal muscles run downward and less obliquely than the external intercostal muscle fibers. This orientation causes these muscles to pull the ribs together, which results in compression of the thoracic cavity. They are stimulated by branches of the intercostal nerves and are most active during forceful exhalation. They also become active toward the end of deep inhalation and act to antagonize the lifting effect of the external intercostal muscles, which effectively stabilizes rib motion during forceful exhalation.49
When the abdominal wall muscles contract, they compress the abdominal cavity. This compression forces the diaphragm upward, compressing the thoracic cavity. The abdominal muscles include pairs of external oblique, internal oblique, transverse abdominis, and rectus abdominis muscles (Figure 8-27).50 The external oblique muscles are the outermost layer of abdominal wall muscle and lie over the lateral aspects of the abdominal cavity. They originate on the anterior surface of the lower eight ribs and abdominal aponeurosis and insert into the linea alba (a connective tissue band on the midanterior surface of the abdomen), iliac crest, and inguinal ligament. The internal oblique muscles lie just underneath the external oblique muscles. They originate on the lumbar vertebrae, iliac crest, and inguinal ligaments and insert into the pubis and costal region of the lower ribs; this results in a fiber orientation that is at right angles to the external oblique muscles. The transverse abdominis muscles lie below the internal oblique muscles. Muscle fibers of the transverse abdominis run around the lateral wall of the abdomen by originating on the lower six ribs, iliac crest, and inguinal ligaments and inserting into the linea alba. The rectus abdominis muscles are a pair of muscular bands that run vertically on the anterior surface of the abdomen. These muscular bands arise from the pubis, travel upward over the abdominal cavity, and insert into the costal region of ribs 5, 6, and 7 and the xiphoid process of the sternum.
Myographic analysis of each of the different abdominal wall muscles reveals that they are active during resting and forceful exhalation.51 They become more active when the elastic recoil of the lung and thorax cannot provide the needed expiratory flow during forceful exhalation, such as coughing, sneezing, talking loudly, and playing wind-powered musical instruments.52 The most active muscle of the group during resting and forceful exhalation in most body positions is the transverse abdominis, and the least active muscles are the rectus abdominis muscles. The abdominal muscles can also contribute to inspiration by contracting at end-exhalation. This contraction reduces end-expiratory lung volume so that the chest wall can recoil outward, assisting the next inspiratory effort.53 Elevating abdominal pressure increases both the length and the radius of curvature of the diaphragm. Both of these effects result in greater transdiaphragmatic pressure for a given contractile tension. In patients with chronic obstructive pulmonary disease, any increase in ventilatory demand significantly increases the use of the abdominal muscles. Loss of effective use of the abdominal wall muscles results in a marked inability to exhale forcefully and to cough effectively.
Pleural Membranes, Space, and Fluid
The thoracic cavity is subdivided into the mediastinum and the left and right pleural cavities. The centrally located mediastinum contains the trachea, esophagus, heart, great vessels, and other organs.54 The left and right pleural cavities contain the lungs. The surfaces of the inner thoracic wall, mediastinum, and lungs are covered with serous membranes called the pleural membranes (see Figure 8-17). The parietal pleural membrane lines the chest wall and mediastinum, whereas the lungs are covered by the visceral pleura. Both membranes are constructed from a thin surface layer of mesothelial cells, and below the layer of mesothelial cells is a layer of connective tissue that houses blood vessels, lymphatic vessels, and nerve fibers.55 Numerous microscopic openings, called stomata, are found in the surface of the pleura and are surrounded by mesothelial cells. The stomata open into the lymphatic drainage system of the pleural membrane. The parietal pleura contains sensory fibers that are responsible for the painful sensation that is associated with inflammation of the pleura—a condition called pleurisy.
The space between the membranes is called the pleural space and is filled with approximately 0.26 ml/kg, or about 18 ml in a 70-kg adult, of pleural fluid.56 Pleural fluid is a clear fluid with a pH of 7.60 to 7.65 that has few cells, a small amount of protein (about 1 g/dl), and glucose and electrolytes in concentrations that approximate those of plasma. The small volume of pleural fluid is spread out over the entire surface of both lungs and functions as a lubricant to reduce friction as the lungs move within the thorax and as an airtight seal that adheres together the two pleural membranes. Pleural fluid is secreted and reabsorbed by the two pleural membranes. A little more than half of the pleural fluid is thought to be produced by the parietal pleura according to Starling forces of filtration. Pleural fluid is formed from the systemic blood flow to each pleura. Blood pressure–driven filtration is supplied to the parietal pleura by blood flow from the intercostal arteries, and the bronchial circulation of the lung supplies most of the blood flow to the visceral pleura.
It is estimated that the pleurae produce 150 to 250 ml of pleural fluid per day.57 Most of the fluid is thought to be absorbed by the visceral pleura capillaries (according to Starling forces), and the rest is cleared by drainage through the lymphatic stomata of parietal pleura, by solute-coupled liquid absorption, and through some transcytosis. Fluid and solutes or cells cleared by lymphatic drainage are carried by the pulmonary lymphatics to the hilar region, where they enter the major lymphatic vessels that drain back to the subclavian veins and right heart.
Mediastinum
The mediastinum lies between the left and right pleural cavities that contain the lungs (see Figure 8-16). The mediastinum is bounded on either side by the pleural cavities, anteriorly by the sternum, posteriorly by the thoracic vertebrae, inferiorly by the diaphragm, and superiorly by the thoracic inlet. The mediastinum can be subdivided into three subcompartments.54 Between the sternum and pericardium is an anterior compartment, which contains the thymus gland and lymph nodes. The middle compartment contains the pericardium, heart, great vessels, phrenic and upper portions of the vagus nerves, trachea, portions of the right and left main stem bronchi, and lymph nodes. The posterior compartment contains the thoracic aorta, esophagus, and thoracic duct. Also found in the posterior mediastinum are the sympathetic nervous system ganglionic chains and lower portions of the vagus nerve and lymph nodes.
Lungs
The lungs are multilobed, cone-shaped, spongelike organs that lie within the pleural cavities (Figure 8-28). They are pink at birth and develop a gray coloration with age. Average adult lungs are hollow low-density organs that occupy a volume of approximately 3.5 L and weigh approximately 900 g.55 The organs within the mediastinum bulge into the left hemithorax, resulting in a narrower and slightly smaller left lung. The liver below the right lung elevates the right diaphragm and results in a slightly shorter right lung.
The anterior, lateral, and posterior lung surfaces lie and move against the thoracic inner wall. The medial surfaces of the lungs lie in close contact to the mediastinal surfaces. Figure 8-29 shows the medial surfaces of the lungs and the opening in this region, which is called the hilum. The main stem bronchi, blood vessels, lymphatics, and nerves that enter or exit the lung all pass through the hilum.
Each lung is divided into two or three lobes (see Figure 8-28), which are separated by one or more fissures. The right lung has upper, middle, and lower lobes. The left lung has only an upper and a lower lobe. Both lungs have an oblique fissure that begins on the anterior chest at approximately the sixth rib at the midclavicular line. These fissures extend laterally and upward until they cross the fifth rib on the lateral chest in the midaxillary line. The fissures continue to the posterior chest to approximately the third thoracic vertebra. The right lung also has a horizontal or “minor” fissure that separates the upper and middle lobes. This horizontal fissure extends from the fourth rib at the sternal border to the fifth rib at the midaxillary line.
The lungs are elastic organs that can expand when inflated with air and recoil back to their resting volume when exhalation occurs. Lung elasticity stems from surface tension forces in the alveoli and from the elastic properties of the tissues and various connective tissue fibers. Three different fiber systems form a scaffold that supports the structure of the lungs as tension develops in them with inflation.58 The axial system, primarily composed of collagen and reticulin fibers, originates in the hilum and extends outward in all of the airway walls almost all the way to the alveolar region. The septal fiber system, composed of collagen, reticulin, and elastin, supports the alveolar walls and capillaries. The peripheral fiber system, primarily composed of collagen, originates in the outer viscera and extends into the lung tissue to divide up the lung tissue effectively into interlobular regions.
Pulmonary Vascular, Lymphatic, and Nervous Systems
Pulmonary Circulation
The pulmonary circulation is supplied with blood from the right heart (Figure 8-30) at a flow rate that is equal to the entire blood volume each minute at rest.59 O2-reduced systemic venous blood flows to the right heart via the inferior and superior venae cavae. This blood is pumped to the lungs by the right ventricle through the pulmonic semilunar valve and on to the trunk of the pulmonary artery. The trunk of the pulmonary artery passes upward and divides into right and left pulmonary arteries just below the point of tracheal bifurcation into left and right main stem bronchi (the carina). The pulmonary arteries accompany the right and left main stem bronchi through the hilar opening into the lungs and continue to divide along with the airways. The pulmonary arteries divide to form two types of arteries: conventional arteries, which continue to follow the airway branching, and supernumerary arteries, which branch at 90-degree angles from the conventional arteries and travel outside the common path. Supernumerary arteries account for about 25% of the cross-sectional area of the pulmonary arterial system. As the arteries continue to divide and become more numerous, they become smaller in diameter and possess greater smooth muscle in their medial walls. Both sets of arteries form arterioles that connect to and supply blood to the microcirculation of the respiratory zone of the lung.
The pulmonary arterial system continues to divide into increasing numbers all the way to the distal airspaces, where they subdivide and form dense “sheetlike” beds of alveolar capillaries that are located within the walls of the alveoli and just below approximately 90% of the alveolar surface (Figure 8-31). The wall of the pulmonary capillary is formed by endothelial cells. At rest, the pulmonary capillary bed contains 60 to 80 ml of blood and can expand to 200 ml through dilation and recruitment of collapsed capillaries during conditions of higher cardiac output (e.g., exercise).60 Pulmonary blood is collected from the capillaries by the pulmonary venules, which combine into larger veins. Similar to their arterial counterparts, the veins also form conventional and supernumerary types of veins that drain blood from the pulmonary capillary beds. The pulmonary veins possess less smooth muscle in their medial walls and have thinner walls than similar-sized pulmonary arteries. The veins follow the same connective tissue path that houses the bronchi and arteries and coalesce into larger and fewer vessels. Four major pulmonary veins—superior and inferior veins from each lung—exit through the hila and return arterialized blood to the left atrium of the heart for delivery to the systemic circulation (see Figure 8-30).
Respiratory Function of Pulmonary Circulation
The pulmonary circulation has several different functions.59 The primary function of the pulmonary circulation is to deliver blood to the alveolar-capillary bed for the exchange of O2 and CO2 with alveolar gas and then to deliver it to the left heart. The second function is to serve as a barrier between the interstitial spaces and airspaces of the lung on one side and the blood within the capillaries on the other. Although less than 0.3 µm thick, the endothelial capillary membrane is an active barrier that controls the exchange of fluid and solutes that cross it. In doing so, it plays a crucial role in the regulation of the fluid balance within the lungs. Injury to the pulmonary capillary often disrupts the fluid balance and can result in excessive fluid leaks and the formation of pulmonary edema. The third function is nonrespiratory and involves the production, processing, and clearance of a large variety of chemicals and blood clots.
Table 8-6 compares the hemodynamics of the systemic and pulmonary circulatory systems.61 Although the entire cardiac output passes through both pulmonary and systemic circuits, the pulmonary circulation offers much lower resistance and consequently has a much lower blood pressure. The low vascular pressures within the pulmonary circuit are essential in maintenance of fluid balance at the alveolar-capillary interface. The pulmonary capillaries are exposed to vascular pressures of about 7 to 10 mm Hg. Increased pressure in the pulmonary circulation, which can occur with mitral valve disease or congestive heart failure, can disrupt fluid balance and lead to excessive fluid leakage, fluid accumulation, and alveolar congestion, which can impair gas exchange and lead to hypoxia.
TABLE 8-6
Resting Hemodynamic Values in Adult Systemic and Pulmonary Vascular Systems
Systemic Circuit | Pulmonary Circuit | |
Blood flow (cardiac output, L/min) | 5 | 5 |
Arterial blood pressure (mm Hg) | 120/80 | 25/10 |
Vascular resistance (dynes/sec/cm−5) | 1200 | 120 |
The low vascular pressures of the pulmonary circulation result in regional blood flow within the lungs that is highly influenced by gravity, airway pressure, and gas exchange.59 In the upright lung, blood pressure in the pulmonary arteries increases approximately 1 cm H2O for each 1 cm traversed downward from the apex to the base. As a consequence of having a low blood pressure and being susceptible to gravity, blood flow is much higher in the lung bases in resting upright subjects. Gravity-related effects also occur in recumbent positions but are less pronounced. The distribution of pulmonary blood flow is also closely related to local airway gas pressure and pulmonary gas exchange. Areas that experience higher airway pressure (e.g., during positive pressure ventilation) that equals or exceeds local arteriole and capillary pressure have reduced blood flow as a result of the opposing airway pressure (zone 1 airways). Regions where blood pressure is greater than the surrounding air pressure, such as in the bases of the upright lung during spontaneous breathing, have greater blood flow (zone 3 airways). Areas of regional lung hypoxia, because of reduced ventilation, congestion, or airway obstruction, can result in local pulmonary arterial vasoconstriction and cause blood flow to be shifted from these areas toward areas of higher O2 content and pulmonary vasodilation.60
Nonrespiratory Function of the Pulmonary Circulation
The pulmonary circulation also serves as a blood reservoir for the left ventricle.59,60 This reservoir maintains stable left ventricular volumes despite small changes in cardiac output. The pulmonary blood volume (approximately 600 ml) is sufficient to maintain normal left ventricle filling for several cardiac cycles. This reservoir is important if filling of the right heart is temporarily decreased or interrupted.
The pulmonary circulation also acts as a filter for the systemic circulation. The capillaries have an inner diameter of about 7 to 10 µm and theoretically trap particles (e.g., blood clots) down to this size before they enter the systemic circulation, where blockages could be life-threatening. Studies in animals have shown, however, that glass beads that are 500 µm in diameter can pass through the pulmonary circulation and probably do so through pulmonary arteriovenous shunts.62
The lungs also play an active role in the clearance and activation and release of various biochemical factors.59,60 They are responsible for synthesis, activation, inactivation, and detoxification of many bioactive substances. Adenosine, norepinephrine, bradykinin, endothelins, atrial natriuretic peptide, and various leukotrienes and certain prostaglandins are removed by the pulmonary circulation. Angiotensin I is converted to its active form (angiotensin II) as it circulates through the lung. Various proinflammatory cytokines are released from the lung when it is injured or repetitively overinflated during mechanical ventilation.63
Bronchial Circulation
A separate arterial supply called the bronchial circulation supplies blood to the airways from the trachea to the bronchioles and to most of the visceral pleurae.64 The metabolic needs of the lung are comparatively low, and much of the lung parenchyma is oxygenated by direct contact with inspired gas. The bronchial circulation is a branch of the systemic circuit and is supplied with blood from the aorta via minor thoracic branches. Blood flow through the bronchial circulation constitutes about 1% to 2% of the total cardiac output.
A single right bronchial artery, which supplies the right lung, arises from the upper intercostal artery, the right subclavian artery, or an internal mammary artery. Two bronchial arteries supply the left lung, and they branch directly from the upper thoracic aorta. Bronchial arteries follow their respective bronchi. Two or three branches accompany each subdivision of the conducting airway. The bronchial arterial circulation terminates in a plexus of capillaries that anastomose with the alveolar-capillary bed. Bronchial venous blood drains through the azygos, hemiazygos, and intercostal veins to the right atrium, and some drains through the pulmonary capillaries to the pulmonary veins and to the right left atrium. Figure 8-32 shows the interrelationship and comingling of the pulmonary and bronchial circulatory systems.
The bronchial and pulmonary circulations share an important compensatory relationship.65 Decreased pulmonary arterial blood pressure tends to cause an increase in bronchial artery blood flow to the affected area. This compensation minimizes the danger of pulmonary infarction, as sometimes occurs when a blood clot (pulmonary embolus) enters the lung. Similarly, loss of bronchial circulation can be partially offset by increases in pulmonary arterial perfusion. The adult lung does not require the bronchial circulation to remain viable, as evidenced by the success of lung transplantation, which does not preserve the bronchial circulation. However, this circulation apparently plays a more important role in lung development, helps to preserve gas exchange during various congenital cardiac conditions, and appears to compensate in certain pulmonary diseases (e.g., pulmonary fibrosis) for the gradual obstruction of the pulmonary circulation.
Lymphatics
The lymphatic system of the lungs is an extensive system of lymphatic vessels, lymph nodes, the tonsils, and the thymus gland.66 The primary function of the lymphatic system is to clear fluid from the interstitial and pleural spaces to help maintain the fluid balance in the lungs. The lymphatic system also plays an important role in the specific defenses of the immune system. It removes bacteria, foreign material, and cell debris via the lymph fluid and through the action of various phagocytic cells (e.g., macrophages) that provide defense against foreign material and cells that are able to penetrate deep into the lung. It also produces various lymphocytes and plasma cells to aid in defense. Both roles are essential for maintaining normal function of the respiratory system.
Most of the pulmonary lymphatic system consists of superficial and deep vessels.67 The superficial (pleural) vessels that drain the lung surface and pleural space are more numerous over the lower half of the upright lung. Many of these vessels are broad ribbon-like, reservoir-type vessels that are closely associated with the blind lymphatic capillaries. The deep (peribronchovascular) conduit-like vessels contain bicuspid valves to direct flow and travel through the connective tissue tracts that house the larger pulmonary vessels in the deeper lung tissue. Both drain the blind lymphatic capillaries in the respective regions. The deeper lymph vessels are closely associated with the small airways but do not extend into the walls of the alveolar-capillary membranes. The lymphatic vessels are thin-walled vessels that contain little connective and muscle tissue in their walls.
Lymph fluid is collected by the loosely formed lymphatic capillaries and drains through the lymph vessels toward the hilum. The fluid is propelled through the lymphatic system by the collective actions of the valves that direct flow toward the hilum and by the combined milking actions of smooth muscle contractions in the deeper conduit-like vessels and ventilation, which squeezes the lymphatic vessels.68 Lymph fluid flow from the lungs can be increased after an injury to the pulmonary capillaries that results in increased leakage (e.g., acute respiratory distress syndrome) or from pulmonary capillary hypertension secondary to heart disease (e.g., left-sided heart failure).
The lymph vessels emerge from the hilum of each lung and drain lymph fluid through a series of lymph nodes that are clustered about each hilum and the mediastinum. From there, lymph travels through various inferior, superior tracheobronchial and paratracheal lymph nodes within the mediastinum (Figure 8-33). The lymph fluid rejoins the general circulation after passing through the right lymphatic or thoracic duct, which drains into the jugular, subclavian, or innominate veins. The lymph fluid mixes with blood and returns to the heart.
Nervous Control of the Lungs
All of the major structures of the respiratory system are innervated by branches of the peripheral nervous system: the autonomic and somatic branches (Figure 8-34).69 The somatic system provides voluntary and automatic motor control and sensory innervation to the chest wall and respiratory muscles. Most of the major motor nerves that carry nervous signaling to the respiratory muscles are summarized in Tables 8-4 and 8-5. The autonomic nervous system signaling to and from the lungs is carried through efferent and afferent pathways. These pathways carry unconscious autonomic nervous system motor signals to smooth muscles and glands and various sensory signals back to the brain.
Autonomic innervation of the lungs is carried from the brainstem through branches of the right and left vagus nerves (cranial nerve X) and from the spinal cord to four or five thoracic sympathetic ganglia that lie just laterally to the spinal cord.70 Both contribute fibers to the anterior and posterior pulmonary plexus at the root of each lung. From these plexus, sympathetic and parasympathetic fibers enter the lung through the hilum and innervate various structures.
Efferent Pathways
After forming ganglia and postganglionic nerve fibers, parasympathetic and sympathetic nerve fibers enter the lung through the hilum and run parallel to the airways as they branch (Figure 8-35). Parasympathetic fibers form their ganglia much closer to the target tissues (e.g., bronchioles, glands, and blood vessels) and have much shorter postganglionic nerve fibers. Most of the sympathetic fibers form their ganglia along the spinal cord and then form longer postganglionic fibers that penetrate the lungs and end on the airway smooth muscle and glands. The largest branches accompany the bronchi. The smallest nerve fibers parallel the pulmonary veins. Both sympathetic and parasympathetic postganglionic efferents innervate the smooth muscle and glands of the airways and the smooth muscles of the pulmonary arterioles. They influence the diameter of the airway by causing more or less tension in the smooth muscles that wrap around the airway and influence glandular secretion. The smooth muscles in the medial wall of the pulmonary arterioles cause constriction when tensed and dilation when relaxed. The combined effects of the parasympathetic and sympathetic nervous activity, which generally oppose each other’s action, result in a balanced control of airway and vessel diameter and glandular secretion.
The airways are provided with a third autonomic pathway that is neither parasympathetic nor sympathetic in action.58 The nonadrenergic, noncholinergic (NANC) system nerve fibers travel within the vagus nerve to each lung. When active, the NANC nerve endings release a neurotransmitter that promotes the production of nitric oxide, which causes the relaxation of airway smooth muscle and dilation. The NANC system is also thought to be capable of causing bronchoconstriction through the local reflex release of substance P and neurokinin A.
Afferent Pathways
Most afferent fibers follow pathways from the lungs to the central nervous system in the vagus nerve. The vagus afferent pathways are activated by a variety of different receptors within the lung that are sensitive to inflation, deflation, and chemical stimulation.71 Slow adapting stretch receptors (SARs) are concentrated in the small and medium-sized airways and are closely associated with the airway smooth muscle. Lung inflation and airway stretch stimulate the SARs, and they continue to signal and do not adapt and drop their signaling rate—hence their name. In the mucosal layer of the airway, rapid adapting receptors (RARs) sense changes in tidal volume, respiratory rate, and changes in lung compliance and respond to a wide variety of mechanical and chemical irritants. In addition, a variety of other chemical and congestion sensors, when active, seem to modify the sensation of breathing and modify the breathing pattern (e.g., cough reflex and response to alveolar congestion). Additional receptors are located outside the lungs; they include respiratory muscle proprioceptors that sense the stretch state of the muscles and peripheral chemoreceptors that sense the chemical condition of blood (e.g., O2, CO2, and H+ concentration) that are involved in the control of ventilation.
Pulmonary stretch SARs and RARs progressively discharge during lung inflation and are linked to inhibition of further inflation. This is a type of negative feedback known as the inflation reflex. It was originally described by Hering and Breuer and continues to bear their names. The inflation reflex is thought to be actively involved with controlling the depth of breathing. Studies in animals indicate that these receptors influence the duration of the expiratory pause between breaths. The inflation reflex is probably very weak or absent during quiet breathing in healthy adults, but there appears to be evidence of its activity in newborns.72
Another reflex that is associated with SAR and RAR activity is the Head paradoxical reflex.73 This reflex stimulates a deeper breath rather than inhibiting further inspiration. It may be the basis for occasional deep breaths or gasps. Deep breaths or sighs occur with normal breathing, presumably preventing alveolar collapse. Head reflex may also be responsible for gasping in newborn infants as they progressively inflate their lungs.
Irritant or mechanical RARs are found mainly in the posterior wall of the trachea and at bifurcations of the larger bronchi. These receptors respond to various mechanical, chemical, and physiologic stimuli and behave as irritant receptors. The stimuli include physical manipulation or irritation, inhalation of noxious gases, histamine-induced bronchoconstriction, asphyxia, and microembolization of the pulmonary arteries. Stimulation of the irritant RARs can result in bronchoconstriction, hyperpnea, glottic closure, cough, and sneeze.74 Stimulation of these receptors can also cause a reflex slowing of the heart rate (bradycardia). This response is referred to as the vagovagal reflex. It may occur during tracheobronchial suctioning, intubation of the airway, or bronchoscopy. These procedures can cause significant mechanical irritation of the airway.
Unmyelinated slow-conducting C-fiber endings (also known as juxtacapillary or J receptors), which are present in the walls of the bronchial and terminal airway region, have been linked to a breathing reflex pattern associated with mechanical stretch, pulmonary congestion, and exposure to various chemicals (e.g., capsaicin, phenylbiguanide, CO2, and autacoids).75,76 When C-fibers become activated, signals are sent back to the brainstem via the vagus nerve. Rapid, shallow breathing results. C-fiber activation has also been shown to cause bradycardia, hypotension, bronchoconstriction, mucus production, and apnea in experimental animals.77 Stimulation of these receptors may contribute to the sensation of dyspnea and, in severe cases, the vagovagal reflex, which can complicate pulmonary edema, pulmonary embolism, and pneumonia.
Anatomy of the Respiratory Tract
Upper Respiratory Tract
The upper respiratory tract is defined as the airways that start at the nose and mouth and extend down to the trachea (Figure 8-36).78,79 The upper airway is open to the outside environment through the external nares or nostrils of the nose and the mouth of the oral cavity. Most of the air moved through the respiratory tract during resting breathing enters through the nares and nasal cavity. Mouth breathing is used during exercise to reduce the resistance to gas flow at higher ventilation rates. The functions of the upper airway are summarized in Box 8-1.
Nasal Cavity and Sinuses
Within the skull bones and around the nasal cavity are the sinuses (Figure 8-37). These hollow spaces are named for the bones in which they are found.80 The sinuses are lined with a mucous membrane and drain into the nasal cavity through numerous ducts. They function to reduce the weight of the skull, to strengthen the skull, and to modify the voice during phonation.
Oral Cavity
Air can also enter and exit from the respiratory tract through the oral cavity (Figure 8-38). The anterior roof of the oral cavity is called the hard palate and is formed by the maxillary bone. The posterior portion is known as the soft palate because of its soft tissue composition and ability to move upward to seal off the nasal cavity. The end of the soft palate hangs down into the posterior portion of the oral cavity. This part of the soft palate is called the uvula. The walls of the oral cavity are formed by the cheeks, and the floor is dominated by the tongue.
The mucosal surfaces of the oral cavity also provide humidification and warming of inspired air. These surfaces are much less efficient than the nose. Saliva is produced by major and minor salivary glands. Saliva functions primarily as a wetting and digestive agent for food but provides some humidification of inspired gas. The oral cavity ends at a double web on each side, called the palatine folds. The palatine tonsils sit between these folds on each side (see Figure 8-38). The palatine tonsils are vascularized lymphoidal tissues that play an immunologic role, especially in childhood.
Reflexes of the mouth, pharynx, and larynx help to protect the lower respiratory tract during swallowing.81 These protective functions can be severely compromised during anesthesia or unconsciousness. Loss or compromise of these important reflexes can result in aspiration of bacteria-colonized saliva or food and can cause pulmonary infection and asphyxiation in severe cases.
Pharynx
The posterior portion of the nasal and oral cavities opens into a region called the pharynx. The entire pharynx is lined with stratified squamous epithelium. The pharynx is subdivided into the nasopharynx, oropharynx, and hypopharynx, or laryngopharynx. The nasopharynx lies at the posterior end of the nasal cavity and extends to the tip of the uvula. Numerous foreign particles impact the surface of the nasopharynx. Located in this region are the two pharyngeal tonsils (also called the adenoids) that are on either side of the lateral and posterior walls of the pharynx. They function to monitor and interact with the particles inhaled through the actions of the lymphoid cells located here. In the same region, there are two openings into the left and right eustachian tubes that link the upper airway with the middle ear (see Figure 8-36). The eustachian tubes drain fluid out of the middle ear and allow gas to move in or out of the middle to equalize pressure on either side of the tympanic membrane.
Larynx
The larynx lies below the hypopharynx and is formed by a complex arrangement of nine cartilages and numerous muscles (Figure 8-39).82 Generally, it functions to protect the respiratory tract during eating and drinking and in phonation. The thyroid cartilage forms most of the upper portion of the larynx and is generally referred to as the Adam’s apple. This cartilage is named for the thyroid gland that lies over its outer surface. Just below the thyroid cartilage is the cricoid cartilage, which is the only laryngeal structure that forms a complete ring of cartilage around the airway and is the most narrow region of the upper airway in infants. A membrane of connective tissue called the cricothyroid ligament spans the space between the thyroid and cricoid cartilage. This membrane is occasionally used as the location for placement of an emergency prosthetic airway in patients who have a life-threatening blockage of the upper airway.
The cartilaginous and leaf-shaped epiglottis lies within and is attached to the thyroid cartilage by a flexible joint. In adults, it is 2 to 4 cm long, 2 to 3 cm wide, and 2 to 5 mm deep. It is not easily visualized in adults, but it can be seen in small children and crying infants because of its higher position. While air breathing, the thyroid cartilage slides down and remains apart from the epiglottis, allowing air to move in and out of the respiratory tract. The epiglottis functions to help prevent liquids and food from entering the respiratory tract by forming a tight seal with the thyroid cartilage during swallowing. The act of swallowing is a complex series of muscular contractions that results in early closure of the vocal cords, upward motion of the thyroid cartilage, and movement of the epiglottis down and back to form a tight seal as food is propelled to the back of the mouth and toward the esophagus.82,83
The inlet to the larynx lies below and behind the base of the tongue. Figure 8-40 shows the inlet as it appears when viewed with a laryngoscope. The base of the tongue is attached to the epiglottis by three folds. These folds form a space between the tongue and the epiglottis called the vallecula, which is a key landmark in oral intubation (see Figure 8-36).
Speech
The laryngeal component of speech is called phonation. It requires the adjustment of vocal cord tension and position relative to one another.84 The action of the posterior cricoarytenoid muscles causes the arytenoid cartilages to rotate and opens the vocal cords. Closure of the vocal cords is accomplished by rotating the arytenoids in the opposite direction through the action of the lateral cricoarytenoid and oblique arytenoid muscles. On closure of the vocal cords, the expiratory muscles of breathing (e.g., abdominal wall muscle group) compress the thoracic cavity and can increase intrapulmonary pressures to 35 cm H2O during forceful speech. To form sound, the cricothyroid muscles tilt the cricoid and arytenoid cartilages posteriorly with respect to the thyroid cartilage, and this elongates and tenses the vocal cords. Simultaneously, this action is opposed by the thyroarytenoid muscles, which act to pull the arytenoid cartilages anteriorly and relax vocal cord tension. Release of pressurized airflow through the tensed vocal cords causes vocal cord vibration and the production of audible sound waves, which resonate in the upper airway and sinuses. By careful adjustment of thyroarytenoid muscle tension and mandible and tongue position, fine control over sound production is achieved. Swelling of the vocal cords or the adjacent tissues increases their mass and disturbs their ability to vibrate; this can result in hoarseness and the inability to phonate.
Patent Upper Airway
The relative positions of the oral cavity, pharynx, and larynx are crucial to the patency of the upper airway in unconscious patients. In upright subjects, the head and neck form a 90-degree angle with the axis of the pharynx and larynx (Figure 8-41, B). With loss of consciousness, the head flexes forward and decreases this angle (see Figure 8-41, A). This positional change can partially or completely obstruct the upper airway. Extension of the head and lower jaw into the “sniff” position alleviates this obstruction (see Figure 8-41, C). Extension of the head moves the tongue away from the rear of the pharynx. This technique is used to maintain the airway in unconscious patients and facilitates placement of artificial airways.
Lower Respiratory Tract
The airways of the tracheobronchial tree extend from the larynx down to the airways that participate in gas exchange. Types of airways and their dimensions are summarized in Table 8-7. Each branching of an airway produces subsequent generations of smaller airways. The first 15 generations are known as conducting airways because they function to convey gas from the upper airway to the structures that participate in gas exchange with blood. The microscopic airways beyond the conducting airways that carry out gas exchange with blood are classified as the respiratory airways.
TABLE 8-7
Bronchial and Bronchiolar Divisions
Structure | Trachea | Segmental Bronchus | Terminal Bronchiole | Number | Diameter of Individual Structures | Total Cross-Sectional Area |
Cartilaginous Conducting Structures | ||||||
Trachea | 0 | 1 | 2.5 cm | 5.0 cm2 | ||
Main bronchi | 1 | 2 | 11-19 mm | 3.2 cm2 | ||
Lobar | 2-3 | 5 | 4.5-13.5 mm | 2.7 cm2 | ||
Segmental | 3-6 | 0 | 19 | 4.5-6.5 mm | 3.2 cm2 | |
Subsegmental | 4-7 | 1 | 38 | 3-6 mm | 6.6 cm2 | |
Bronchi | 2-6 | Varies | Varies | Varies | ||
Terminal bronchi | 3-7 | 1000 | 1.0 mm | 7.9 cm2 | ||
No Cartilage in Walls | ||||||
Bronchioles | 5-14 | Varies | Varies | Varies | ||
Terminal bronchioles | 6-15 | 0 | 35,000 | 0.65 mm | 116 cm2 | |
Respiratory bronchioles | 1-8 | Varies | 0.5-0.3 mm | Varies | ||
Terminal respiratory bronchioles | 2-9 | 630,000 | 0.45 mm | 1000 cm2 | ||
Alveolar ducts/sacs | 4-12 | 4 × 106 | 0.40 mm | 1.71 m2 | ||
Alveoli | 300 × 106 | 0.25-0.30 mm | 140 m2 |
Trachea and Bronchi
The trachea extends from its connection to the cricoid cartilage down through the neck and into the thorax to the articulation point between the manubrium and body of the sternum (angle of Louis). At this point, it divides into two main stem bronchi (Figure 8-42). The adult trachea is approximately 12 cm long and has an inner diameter of about 2 cm. Figure 8-43 shows the different layers of tissue that form the trachea. The outermost layer is a thin connective tissue sheath. Below the sheath are numerous C-shaped cartilaginous rings that provide support and maintain the trachea as an open tube. The typical adult trachea has 16 to 20 of these rings. The inner surface of the trachea is covered with a mucous membrane. In the posterior wall of the trachea is a thin band of tissue, called the trachealis muscle, that supports the open ends of the tracheal rings. The esophagus lies just behind the trachea.
The trachea lies midline in the upper mediastinum and branches into right and left main stem bronchi (see Figure 8-42). At the base of the trachea, the last cartilaginous ring that forms the bifurcation for the two bronchi is called the carina. The carina is an important landmark that is used to identify the level where the two main stem bronchi branch off from the trachea; this is normally at the base of the aortic arch. The right bronchus branches off from the trachea at an angle of about 20 to 30 degrees, and the left bronchus branches with an angle of about 45 to 55 degrees (Figure 8-44). The lower angle of branching of the right bronchus results in a greater frequency of foreign body passage into the right lung because of the more direct pathway.
Lobar and Segmental Pulmonary Anatomy
The lungs have an apex and a base and are subdivided by fissures into lobes.55 The lobes are subdivided further into bronchopulmonary segments (Table 8-8 and Figure 8-45). Each segment is supplied with gas from a single segmental bronchus. Controversy exists over the exact number of segments; some anatomists accept that each lung has 10 segments, whereas others maintain that the right has 10 and the left has 8. Knowledge of segmental anatomy is important in the physical examination of a patient to identify the location of a defect such as an infection site or a tumor mass in the lungs.
TABLE 8-8
Segment | Number | Segment | Number |
Right Upper Lobe | Left Upper Lobe | ||
Apical | 1 | Upper division | |
Posterior | 2 | Apical-posterior | 1 and 2† |
Anterior | 3 | Anterior | 3 |
Right Middle Lobe | Lower division (lingula) | ||
Lateral | 4 | Superior lingula | 4 |
Medial | 5 | Inferior lingula | 5 |
Right Lower Lobe | Left Lower Lobe | ||
Superior | 6 | Superior | 6 |
Medial basal | 7 | Anterior basal | 7 and 8 |
Anterior basal | 8 | Lateral basal | 9 |
Lateral basal | 9 | Posterior basal | 10 |
Posterior basal | 10 |
*The subdivisions of the lung and bronchial tree are fairly constant. Slight variations between right and left sides are noted by combined names and numbers.
†Some authors believe that the left lung should be numbered so that there are eight segments, where the apical-posterior is numbered 1 and the anteromedial is numbered 6.
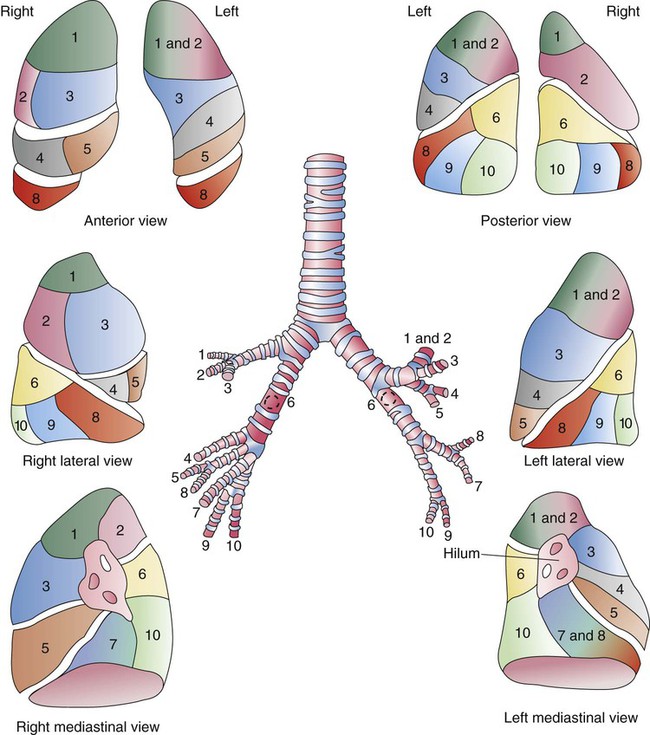
With further divisions, the number of airways increases tremendously. The cross-sectional area of the conducting system increases exponentially. At the level of the terminal bronchioles, the cross-sectional area is approximately 20 times greater than that at the trachea. Gas flow in these airways conforms to the laws of fluid physics. Increased cross-sectional area reduces the velocity of gas flow during inspiration. When inspired gas reaches the level of the terminal bronchiole, its average velocity has fallen to about the same rate as the speed of diffusing gas molecules.85 Low-velocity gas movement at the level of the terminal bronchiole and beyond is physiologically important for two reasons. First, laminar flow develops, which minimizes resistance in the small airways and decreases the work associated with inspiration. Second, low gas velocity facilitates rapid mixing of alveolar gases. This mixing provides a stable partial pressure of O2 and CO2 in the alveolar environment that supports stable diffusion and gas exchange.86
Histology of the Airway Wall
All of the conducting airways from the trachea to the bronchioles have walls that are constructed of three layers (Figures 8-46 and 8-47): an inner layer that forms a mucous membrane called the mucosa, which is primarily composed of epithelia; a submucosa composed of connective tissue, bronchial glands, and smooth fibers that wrap around the airway; and an outer covering of connective tissue called the adventitia.87 The cartilaginous rings and plates found in larger airways are located in the adventitia.
The mucosa is composed of many different types of specialized epithelial cells that sit on top of a basement membrane. The most common type of epithelia are the numerous pseudostratified, ciliated, columnar epithelia.88 The pseudostratified epithelial cells are held together toward their surface or apical end through three types of junctions—apical tight junctions, zonal adherens junctions, and desmosome-type junctions—and they anchored in place to the basement membrane.89 The junctions, especially the tight junctions, play an important role in the maintenance of fluid and electrolyte (e.g., Cl−) transport across the mucous membrane. These junctions prevent the movement of fluids and electrolytes between the apical surface and basal surfaces of the airway. Disturbances in this transport (e.g., Cl− transport malfunction in cystic fibrosis transport receptor membrane channels) can lead to mucus and mucus transport abnormalities.
Near the base of the pseudostratified cells are large numbers of basal cells. The basal cells contribute to the appearance of a “pseudostratified” cellular layer. Basal cells mature into pseudostratified cells and are thought to play an important role in repair of the mucous membrane after diseases and injury. Dispersed between the pseudostratified epithelia are mucus-producing goblet cells and serous cells (in newborns) and the openings of submucosal bronchial glands. The bronchial glands are exocrine glands formed by secretory epithelial cells that sit on the basement membrane, which extends down into the lamina propria and into the submucosa. The bronchial glands are wrapped with myoepithelial cells. Myoepithelial cells contract and squeeze the bronchial gland when they receive signals from parasympathetic nerve fibers. In this region are neuroendocrine cells (also known as Kulchitsky cells) that are often organized into small clusters called neuroepithelial bodies.90 Neuroendocrine cells are connected to the vagus nerve and are thought to function during lung development, are hypoxia and stress-strain sensors, and secrete various bioactive chemicals (e.g., serotonin, calcitonin, and gastrin-releasing peptide). Lymphocytes are also found intermixed with these cells, and it is thought that they may be migratory in nature.
Below the epithelial and basement membrane of the mucosa is the lamina propria.89 The lamina propria is composed of loose fibroelastic connective tissue, lymphoid tissue, and a dense layer of elastic fibers. Below the lamina propria lies the submucosa. The submucosa of large airways contains bronchial glands, a capillary network, smooth muscle, some elastic tissue, and cartilage in larger airways. Bronchial glands vary in size up to 1 mm in length and connect to the bronchial surface via long, narrow ducts. The number of these glands increases significantly in diseases such as chronic bronchitis. Mast cells are also found in the submucosa and release numerous and potent vasoactive and bronchoactive substances such as histamine.91 Histamine causes vasodilation and bronchoconstriction, acting directly on smooth muscle. The triggering of mast cell release of its various substances and the resultant inflammation and bronchospasm of the airway are characteristic of the pathologic changes of asthma.
The various secretory cells (primarily goblet cells) of the mucosa and bronchial glands of the submucosa contribute to the production of mucus.92 Normally, the respiratory tract produces about 100 ml of mucus per day. Most of the mucus formed in the larger airways is produced by the bronchial glands. Goblet cells contribute more in the smaller airways. The amount and composition of mucus produced can increase and change with airway irritation and diseases such as chronic bronchitis and asthma.93 Mucus is spread over the surface of the mucus membrane to a depth of about 7 µm and is propelled by the ciliated epithelia toward the pharynx. The outer layer of mucus is more gelatinous and is called the gel layer. The inner layer is much more fluid-like and is referred to as the sol layer. The mucus normally produced is a nearly clear fluid with greater viscosity than water. It is a mixture of 97% water and 3% solute.92 The solute portion is produced primarily by goblet cells and bronchial glands; it is called mucin and is composed of protein (predominantly glycoproteins, proteoglycans, and IgA and IgG), lipids (primarily neutral lipids), and minerals (mainly inorganic electrolytes). The glycoprotein, lipid, and water content of mucus provides its viscoelastic gel nature. Viscoelastic refers to the ability of mucus to deform and spread when force is applied to it.
Mucus functions to protect the underlying tissue. It helps to prevent excessive amounts of water from moving into and out of the epithelia.92 It shields the epithelia from direct contact with potentially toxic materials and microorganisms. It acts like sticky flypaper to trap particles that make contact with it. This makes mucus an important part of the pulmonary defenses. The production of mucus is stimulated by local mechanical and chemical irritation, release of proinflammatory mediators (e.g., cytokines), and parasympathetic (vagal) stimulation.
The ciliated pseudostratified epithelia play a crucial role in the defense of the respiratory tract by propelling mucus toward the pharynx. Ciliated cells are found in the nasal cavity and all the airways from the larynx to the terminal bronchioles. Each of the pseudostratified cells possesses about 200 cilia on its luminal surface.89 Under the electron microscope, the surface of the mucus membrane looks like a “shag carpet” of cilia with about 1 to 2 billion cilia per square centimeter. Each cilium is an extension of the cell with an average length of about 6 µm and diameter of about 0.2 µm. A cross-sectional view through the cilium reveals it to be constructed of one inner and nine outer pairs of microtubules that are encased in the cell membrane. The outer pairs of microtubules are interlinked by a filamentous protein called nexin. From each of the outer pairs of microtubules, protein filaments called dynein extend toward the adjacent pair of microtubules. Each of the outer pairs also extends a protein spoke toward the central pair of microtubules. The presence of Mg2+ and ATP within the cilium causes the dynein arms and spokes to attach and slide along the outer and inner microtubules, similar to the action of actin and myosin. This action results in rapid bending of the cilium that resembles a whipping motion (Figure 8-48).
The cilia “stroke” at a rate of about 15 times per second, which produces a sequential motion of the cilia called a metachronal wave.94 The metachronal “wavelength” is approximately 20 µm and propels surface material in a specific direction. In the nose, this motion propels material back to the pharynx. From the bronchioles up to the larynx, it moves material toward the pharynx. The stroking action of millions of cilia propels the surrounding mucus at a speed of about 2 cm/min. This action is commonly referred to as the mucociliary escalator. In healthy lungs, this mechanism allows inhaled particles to be removed within 24 hours. The control and coordination of ciliary motion are not totally understood and represent some of the many fascinating properties of pulmonary tissues.
The production of mucus and the rate of ciliary beating are sensitive to various conditions and chemicals. Mucus production increases when the respiratory tract is irritated by particles and by various chemicals and during increased parasympathetic nervous stimulation.93 Ciliary beating can be effectively slowed or stopped if the viscosity of the sol layer is increased by exposure to dry gas. Ciliary motion is also slowed or stopped after exposure to smoke, high concentrations of inhaled O2, and drugs such as atropine.
The cells of the respiratory mucosa change as they progress into the smaller airways (Figure 8-49). As the thickness of the airway walls decreases, bronchial glands become fewer in number. At the bronchiolar level, the number of ciliated cells decreases. Simple columnar and cuboidal epithelial cells begin to predominate and are interspersed with goblet cells. In this region, large numbers of Clara cells, nonciliated cuboidal cells with apical granules, are found. It is thought that these cells play a role in degrading various xenobiotic oxidants via cytochrome P-450, contribute proteins for surfactant production, synthesize various lipids, and play a role in lung repair by being able to differentiate into other important epithelial cells in the mucosa after injury.95
Respiratory Zone Airways
The terminal bronchioles begin about 12 to 15 generations beyond the trachea (Figure 8-50).96 There are about 16,000 terminal bronchioles with airway opening diameters of about 700 µm. This yields a combined cross-sectional area opening that is almost 100 times that of the main stem bronchi. All of the airways down to and including the terminal bronchioles carry or conduct gas flow to and from the airways that participate in gas exchange with blood. The airways from the nares to and including the terminal bronchioles constitute the conducting zone airways, which do not participate in gas exchange. These airways constitute the anatomic dead space of the respiratory system, which is rebreathed with each breath. In an adult human, the volume filling the airways of the anatomic dead space is approximately 2 ml/kg of lean body weight, or about 150 ml in a typical adult.
A single terminal bronchiole supplies a cluster of respiratory bronchioles. Collectively, this unit is referred to as the acinus, or primary lobule. Each acinus comprises numerous respiratory bronchioles, alveolar ducts, and approximately 10,000 alveoli (Figure 8-51). The adult lung is thought to contain more than 30,000 acini. Each acinus is supplied with pulmonary blood flow from a pulmonary arteriole, and blood is drained away from several acini through a pulmonary venule. In addition, each acinus is equipped with a lymphatic drainage vessel and nervous fibers. These features make the primary lobule the functional unit of the lungs. Gas molecule movement in this region is largely via diffusion rather than convective flow, which occurs in larger airways.
Millions of alveolar ducts branch from the respiratory bronchioles (Figure 8-52). Alveolar ducts are tiny airways only 0.3 mm in diameter, and their walls are composed entirely of alveoli. Each alveolar duct ends in a cluster of alveoli, which is frequently referred to as an alveolar sac. Each alveolar sac opens into about 16 or 17 alveoli, and about one-half of the total number of alveoli are found in this region.
Alveoli
More recent estimates suggest that the number of alveoli in adult lungs range from 270 to 790 million, with an average of about 480 million.32 The number of alveoli increases with the height of the subject. Alveolar size varies with lung volume and averages about 0.2 mm in diameter when the lung is inflated to its functional residual volume. Figure 8-53 shows alveoli in a normal rat lung at different states of inflation and how their shapes change. When inflated at and beyond the functional residual volume (see Figure 8-53A-C), alveoli have a polyhedral shape that results from numerous flat walls rather than a curved spherical structure. Alveoli found in the apical regions of the vertical lung have greater diameters than alveoli in the basal regions as a result of the gravitational effects. Alveoli in the basal regions are partially collapsed as a result of the weight of the organ.
The alveolar walls or septa are formed by various cell types that are arranged to provide a thin surface for gas exchange and strength.97 The alveolar septa are covered with extremely flat squamous epithelia called type I pneumocytes (Figure 8-54). Although they represent only about 8% of all the cells found in the alveolar region, type I cells cover about 93% of the alveolar surface.98 These cells form a “patchwork”-like surface that covers the alveolar capillaries and forms the gas exchange surface of the alveolus. At their edges where they meet one another, they form tight junctions that help to limit the movement of material into the alveolar airspace from the interstitial space just below. They are held in place and supported from below by a network of collagen and elastin fibers. They are susceptible to injury and apoptosis (programmed cell death) from inhaled particles (e.g., cigarette smoke), bacterial infection, and excessive concentrations of inhaled O2.
Interspersed on the alveolar surface and concentrated in the corners of the alveolar septa are type II pneumocytes, which are cuboidal epithelia with apical microvilli (Figure 8-55). These cells are twice as numerous as the type I cells, but they occupy only 7% of the alveolar surface.98 Type II cells do not function as gas exchange membranes as the type I cells do. They (along with the Clara cells) manufacture surfactant, store it in vesicles called lamellated bodies, and secrete it onto the alveolar surface.99 Surfactant is primarily composed of phospholipids (dipalmitoylphosphatidylcholine) and proteins (surfactant proteins A through D). It functions to reduce the surface tension of the alveolus, sheds water from the alveolar surface, helps to prevent alveolar surface tension-driven collapse, improves lung compliance, reduces the work of breathing, and protects the alveolar surface. Normally, surfactant is removed from the alveolar space continuously by type II cells and macrophages. The type II cells recycle about 50% of it, whereas the macrophages primarily remove it through catabolism.100
Although the lungs do not have stem cells in the classic sense, the type II cells do have a “stem cell”–like action. They can proliferate and differentiate into type I cells to repopulate and repair the alveolar surface after injury.101 They are also involved in alveolar defense through surfactant production and the release of some cytokines that trigger inflammation.
Macrophages are another common cell found in the alveolar region.98 They can move from the pulmonary capillary circulation by squeezing through openings in the alveolar septa and then move out onto the alveolar surface. They are defensive cells that patrol the alveolar region and phagocytize foreign particles and cells (e.g., bacteria). They can present portions of the foreign particles and bacteria to lymphocytes as part of the immune response and contain various digestive enzymes (e.g., trypsin) that break down the material they engulf.
Within the interalveolar septum is an interstitial space that contains matrix material and the pulmonary capillaries. Also found in the interstitial space are bands of elastin fibers and a collagen fiber matrix.58 These fibers support the alveolar cells and the shape of the alveolus. Small openings are located in the alveolar septa. Some of the openings allow gas to move from one alveolus to another. These are called the pores of Kohn. Other openings connect alveoli with secondary respiratory bronchioles. These passageways are called the canals of Lambert. All of these alveolar openings and passageways facilitate the collateral movement of gas and help maintain alveolar volume.102
Blood-Gas Barrier
Gas exchange between alveolar gas and pulmonary capillary blood occurs across the alveolar-capillary membrane. In a typical adult, this blood-gas barrier stretches over a surface area of approximately 140 m2 and is less than 1 µm thick over most of that area.103 This makes the membrane more than 50 times larger than the area covered by skin and more than 2000 times thinner.
The blood-gas barrier is composed of many different layers through which O2 and CO2 diffuse (Figure 8-56). The outermost layer is a very thin film of fluid composed primarily of surfactant that forms into a tubular myelin matrix. Below the surfactant fluid layer is the thinly stretched type I cell. The delicate structure of type I cells makes them highly susceptible to injury from toxins carried to them by either airborne or blood-borne routes. The interstitial space and its contents lie below. Within this space are basement membranes, matrix material connective tissue fibers, and the alveolar capillary.58 The capillary wall is formed from thin, flat squamous epithelia called endothelial cells that form a thin tube by connecting together at their edges with tight junctions. Within the capillary lies the plasma and, finally, the erythrocytes. Both O2 and CO2 cross through the membrane via partial pressure-driven diffusion.
The blood-gas barrier is not equal in thickness and chemical content from side to side (see Figure 8-56). On one side of the alveolar wall, the type I cells and capillary endothelial cells lie close together with a thin interstitial space. This part of the blood-gas barrier is, on average, 0.2 to 0.3 µm thick, and it is where the alveolar capillary bulges into the alveolar space.103 On the other side, where there is a thicker interstitial space with greater fiber, matrix, and nuclear material content, the barrier can be more than 3 to 10 times thicker. This difference between the two sides functionally results in “faster-weaker” and “slower-stronger” diffusion sides of the blood-gas barrier.
The interstitial space within the alveolar septum contains a network of fibers that form a kind of connective tissue skeleton that holds the alveolar structures in place and together.104 The fibers within the alveolar septum are part of the continuum of connective tissue fibers that are found in the pleural surface and in the airway walls that extends all the way to the root of the lung in the hilar region. Elastin and collagen fiber bands are formed by fibroblasts into a network within the interstitial space into which the capillaries are woven. Also around the fibers and capillaries is a nonliving matrix of fluid and solutes. The weaving path taken by the capillaries passes them from the thick to the thin sides of the blood-gas barrier as they extend through the septum. In the thin side, the basement membranes of the endothelial and type I cells fuse into a structure called the lamina densa, which is formed from type IV collagen.105 In the thick side, thick bands of type I collagen and elastin are found. The type I cells and endothelial cells are attached to either side of the lamina densa by a series of protein fibers collectively known as laminins. Laminins effectively bind together the blood-gas barrier into a three-part laminate that results in a relatively strong and thin structure that can normally, with the additional support offered by the capillary network, withstand the everyday stress of alveolar and capillary stretch.106