Chapter 10 Spinal Fluid Examination
History
In 1891, Wynter first reported the use of LP as a therapeutic measure after using the technique in a patient with tuberculous meningitis. The diagnostic value of LP was first recognized and described by Quincke in 1891. His innovations included using a percutaneous needle with a stylet, a manometer to record the opening pressure, and measurements of CSF cell counts, protein concentration, and sugar content. The technique quickly gained acceptance and had become a routine part of medical care by the turn of the 20th century. Several authors have summarized this early history [Vandam, 1989]. Numerous subsequent investigators have contributed to an ever-expanding understanding of the value of CSF analysis in various neurologic diseases [Fishman, 1992].
Cerebrospinal Fluid Formation, Flow, and Absorption
CSF is principally formed by secretion from the choroid plexus, villous invaginations of the walls of the lateral, third, and fourth ventricles which are richly vascularized and lined by a ciliated epithelium (Figure 10-1). The choroid plexus of the lateral ventricles is continuous through the foramina of Monro with the choroid plexus of the roof of the third ventricle. The arterial supply to this portion of the choroid plexus originates from the anterior choroidal arteries, which branch off the internal carotid artery, and from the posterior choroidal arteries, which are branches of the posterior cerebral arteries. The choroid plexus within the fourth ventricle, which sends extensions through the lateral foramina of Luschka, is usually supplied by the posterior inferior cerebellar arteries. In studies of rats, blood flow to choroidal vessels is almost 10 times greater than that to the cerebral cortex [Szmydynger-Chodobska et al., 1994]. Nerves derived from several sources, including the cervical sympathetic chain, the neural plexus of the internal carotid and posterior cerebral arteries, and the vagal nuclei, provide extensive adrenergic, cholinergic, and peptidergic innervation of the blood vessels and epithelial cells of the choroid plexus. These inputs have an influence on the rate of CSF production that is independent of vasomotor changes [Lindvall and Owman, 1981].
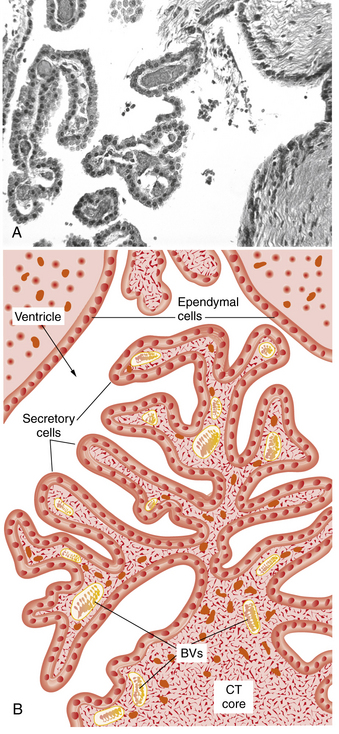
Fig. 10-1 Structure of the choroid plexus.
(A, Courtesy of Dr. Thomas Caceci, Virginia–Maryland College of Veterinary Medicine. B, Adapted from a drawing by Dr. Samir El-Shafey, Virginia–Maryland College of Veterinary Medicine.)
The capillaries of the choroid plexus, unlike those found in most other areas of the brain, have large fenestrations that offer little resistance to the passage of fluid, ions, and small macromolecules [Segal, 1993]. Passage of blood past these capillaries creates an ultrafiltrate of plasma within the interstitial space at the basolateral surface of the epithelial cells. Analysis of the ionic composition of CSF relative to plasma (Table 10-1) reveals that this interstitial fluid is modified considerably before it reaches the ventricular system, and strongly supports CSF formation being an active secretory process [Davson et al., 1987].
Additional studies have found that CSF secretion depends highly on active transport proteins that, as with other secretory epithelia, are differentially localized on the apical and basolateral membranes of the choroid plexus epithelial cells. Water follows the flow of sodium and chloride ions from the interstitial fluid into the CSF. Carbonic anhydrase within the epithelial cells catalyzes the formation of carbonic acid from water and carbon dioxide. Carbon dioxide diffuses freely, but the dissociation products of carbonic acid, bicarbonate and hydrogen, are transported across the basolateral membrane by specific proteins in exchange for sodium and chloride, favoring the passive diffusion of water into the cells [Speake et al., 2001]. Sodium transport out of the cells is principally mediated by sodium–potassium ATPases on the apical membrane, with selectively permeable apical chloride and potassium channels rounding out the complement of transporters thought to be most important to creating a net flow of water into the CSF (Figure 10-2). Water does flow passively along osmotic gradients through the choroid plexus, moving freely through the aquaporin-1 channels which are highly expressed on the apical surface of the epithelial cells [Tait et al., 2008]. However, the contribution to CSF production from osmosis is minimal, as CSF production by experimental animals is unaltered even by instillation of high osmolar fluid into the ventricles [Macaulay and Zeuthen, 2009].
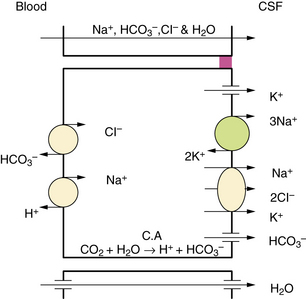
Fig. 10-2 Major processes involved in cerebrospinal fluid (CSF) secretion.
(From Brown PD, Davies SL, Speake T, et al. Molecular mechanisms of cerebrospinal fluid production. Neuroscience 2004;129:957.)
Other specific active transport proteins present along the basolateral membrane allow transport into the CSF of essential hydrophilic micronutrients, including glucose, amino acids, purines, nucleosides, and vitamins. Transport proteins along the apical membrane work to clear the CSF of potentially toxic metabolites, such as organic acids and bases [Spector and Johanson, 1989].
Tight junctions link the choroid plexus epithelial cells, limiting the free diffusion of ionic molecules and creating the blood–CSF barrier. Although protein diffusion is largely restricted, most of the small amount of protein found in the CSF is nevertheless of plasma origin. Maintenance of a relatively stable CSF composition, despite wide variation in the composition of the plasma, reflects the integrity of the blood–CSF barrier and the work of active transporters against concentration gradients [Fishman, 1992].
The choroid plexus produces from 70 to 90 percent of the CSF, with the remainder deriving from movement of brain parenchymal interstitial fluid across the ependyma into the ventricles and across the meninges into the subarachnoid space [Proescholdt, 2000]. The rate of CSF formation in healthy adults averages 0.35 mL per minute, or roughly 500 mL each day. Children produce proportionally less CSF, depending on their height and weight, with as little as 25 mL produced per day in newborns. Postmortem studies have provided estimates that total CSF volume ranges from 50 mL in term neonates to 150 mL in adults, with only a small percentage contained within normal-sized ventricles. The total volume of the CSF undergoes complete replacement 3–4 times each day.
Choroid plexus epithelial cells express a wide range of receptors for hormones and neurotransmitters [Nilsson et al., 1992], but the rate of CSF formation appears to remain fairly constant under most conditions. Many factors have been found to influence the rate of CSF production in animal models, including cholera toxin, norepinephrine, hyperosmolality, hypothermia, atrial natriuretic hormone, vasopressin, serotonin, and dopamine [Rosenberg, 1990]. Cholera toxin and norepinephrine increase CSF production through an increase in cyclic AMP formation, which has been linked to increased bicarbonate transport [Saito and Wright, 1984].
Greatly reduced CSF production has been seen after experimental administration of the sodium–potassium ATPase inhibitor ouabain [Vates et al., 1964]. Carbonic anhydrase inhibitors, such as acetazolamide, can reduce the choroidal production of CSF in rats by 50–100 percent [Fishman, 1992]. Of the seven mammalian isoforms of carbonic anhydrase, however, the CAIII isoform that is most abundant in the human choroid plexus is insensitive to acetazolamide [Nogradi et al., 1993], explaining the somewhat limited clinical effectiveness of this medication [Cowan and Whitelaw, 1991]. Furosemide and other loop diuretics weakly inhibit carbonic anhydrase activity but are thought to reduce CSF production through inhibition of sodium, potassium, and chloride co-transporters [Johanson et al., 1994]. Endogenous vasopressin release and V1 receptor activation appears to mediate some of the reduction in choroidal blood flow and CSF formation that occurs with hypoxia or with increased intracranial pressure [Faraci et al., 1994]. Elevated CSF vasopressin levels have been found in some patients associated with the syndrome of idiopathic intracranial hypertension [Sorensen et al., 1982].
Figure 10-3 illustrates the pathway taken by the CSF. Fluid is secreted into the lateral ventricles, passes through the foramina of Monro into the third ventricle, and flows through the aqueduct of Sylvius into the fourth ventricle. CSF exits the ventricular system through the lateral foramina of Luschka to enter the prepontine cistern and cerebellopontine angles. Alternatively, CSF can leave the fourth ventricle through the midline foramen of Magendie, entering the cisterna magna, from which it can flow upward over the cerebellar hemispheres or downward into the spinal subarachnoid space and around the brainstem into the basal cisterns, including the interpeduncular cistern. CSF flows predominantly downward posterior to the spinal cord and upward anterior to the cord, leading the fluid eventually to reach the basal cisterns, from which flow is mainly upward over the brain convexity. The quality of CSF flow can be visualized noninvasively using spin-echo labeled magnetic resonance imaging (MRI) [Yamada et al., 2008].
In the steady state, the rate of CSF absorption is equal to that of its production. Most CSF is absorbed into the venous sinuses across the arachnoid villi and granulations, arachnoid membrane invaginations through the dural lining of the sinuses that are concentrated near the sagittal sinus. Absorption across the arachnoid villi occurs by vesicular transport. This action demonstrates a dependence on the hydrostatic pressure gradient across the villous surface, such that the villi act as one-way pressure valves that open above a threshold pressure, usually 20–50 mm H2O [Welch and Friedman, 1960]. Some CSF absorption occurs across the capillaries, veins, and lymphatics of the spinal cord and spinal roots [Koh et al., 2005], and evidence suggests that CSF is also reabsorbed through the cribriform plate and nasal lymphatics [Johnston et al., 2004].
Cerebrospinal Fluid Function
The CSF provides buoyancy and physical protection to the brain, absorbing the stretching and compressive forces generated by normal head movement and lessening the impact of the deceleration and rotational forces created by head trauma. A second protective function derives from the ability of the CSF to redistribute in response to acute changes in other intracranial contents, helping to maintain normal intracranial pressures. The CSF is also thought to serve as a route for the transport of centrally acting hormones, such as for the diffusion of hypothalamic releasing factors across the third ventricle [Kozlowski, 1982].
The CSF aids in the excretion of metabolites through bulk absorption of the interstitial fluid of the brain and spinal cord, with which it is in close communication [Go, 1997], prompting speculation that the CSF pathways are analogous in function to the lymphatic channels that these tissues lack [Cserr et al., 1977]. This “sink action,” along with the various mechanisms by which the CSF composition is maintained, suggests that the CSF plays a significant role in normal physiology and in the compensatory response to pathologic situations [Hochwald, 1984].
Diagnostic Sampling of Cerebrospinal Fluid
Contraindications and Cautions
LP through a soft tissue infection such as cellulitis is discouraged out of concern that this can introduce bacteria into deeper tissues and cause an epidural abscess, osteomyelitis, or meningitis [Abolnik et al., 1995]. An increased bleeding tendency is also a contraindication because puncture may incur a greater risk of epidural, subdural, or subarachnoid bleeding [Adler et al., 2001]. However, coagulopathy may be correctable by stopping an anticoagulant medication or by giving an appropriate transfusion of platelets or clotting factors [Silverman et al., 1993]. Before LP is performed, it is believed that the patient should have a platelet count greater than 10,000/mm3 and an international normalized ratio less than 1.5 [Howard et al., 2000]. Anticoagulation therapy should probably be delayed for at least 2 hours after LP [Sadjadpour, 1977].
There is considerable and understandable fear that LP in patients with a CSF obstruction can unbalance the pressure across the area of obstruction and contribute to cerebral herniation or spinal cord compression. Neuroimaging, usually with cranial computed tomography (CT), is often performed before LP, especially in patients who appear ill. Patients are considered to be at an increased risk for cerebral herniation if neuroimaging reveals a focal mass lesion or obliteration of the fourth ventricle or quadrigeminal cistern. In adults with suspected meningitis, patients without clinical signs of increased intracranial pressure (i.e., papilledema or altered level of consciousness) or focal neurologic deficits are very unlikely to have an abnormal CT scan or to herniate after LP [Hasbun et al., 2001]. Children undergoing evaluation for suspected meningitis who are at higher risk of having a cerebral abscess, such as those with a history of cyanotic congenital heart disease, may need neuroimaging prior to LP [Tunkel et al., 2004]. However, herniation has been reported after normal neuroimaging, leading many to continue to debate the appropriate use of neuroimaging before LP [Oliver et al., 2003]. Since it is considered essential that antibiotic administration not be delayed when meningitis is suspected, especially with meningococcal disease, current recommendations are to start antibiotics as soon as possible, before LP if necessary [Tunkel et al., 2004].
Procedure
The lower end of the spinal cord, the conus medullaris, is found by MRI to be at the L1–2 vertebral interspace in most subjects and safely above the L3 vertebral body in all subjects without spinal deformity [Saifuddin et al., 1998]. To avoid damaging the conus, only the interspaces below L3 are used for LP. With a patient lying in the lateral recumbent position, the L3–4 interspace can be found at the level of the superior iliac crests. This area should be carefully palpated and marked to ensure that it can be identified after the patient is cleaned and draped. Having the patient curl with the neck and hips flexed can maximize the size of the interspace. Whether the patient is placed in a lateral recumbent or seated position, care should be taken to maintain the alignment of the shoulders and hips, avoiding rotation of the spine. Having the patient’s back near the edge of the bed and at a proper height can aid in the comfort and success of the physician. The recommended positioning is shown in Figure 10-4.
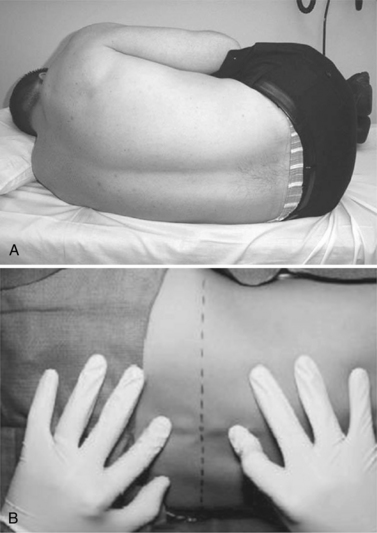
Fig. 10-4 Proper positioning of the patient.
(From Roos KL. Lumbar puncture. Semin Neurol 2003;23:105.)
The patient’s skin should be prepared using a microbicidal agent, such as a 10 percent solution of povidone-iodine. The skin should be given time to dry and then wiped clean and draped with sterile towels. A local anesthetic, such as a 1 percent solution of lidocaine, can be injected into the area to minimize the pain of repeated needlesticks. Anesthesia provides little benefit when the LP can be accomplished with a single needlestick, because the pain of the anesthetic injection often equals or exceeds that of the spinal needle insertion. A reasonable alternative is the use of a topical analgesic cream, such as the 5 percent emulsion of lidocaine and prilocaine commonly referred to as eutectic mixture of local anesthetics (EMLA). EMLA requires 20–30 minutes of application but has been associated with a decreased pain response, even in neonates [Kaur et al., 2003].
The most commonly used spinal needles are 2.5- or 5-cm long, 20- or 22-gauge Quincke needles, which are beveled and fitted with a stylet. The sharply beveled tip of the Quincke needle should be turned to face the patient’s side so that its cutting edge can push between, rather than cut, the longitudinal fibers of the dura. Atraumatic needles, such as the Whitacre and Sprotte needles, have duller tips that make dural injury less likely (Figure 10-5). These needles are also more flexible, encounter more tissue resistance, and require the use of an introducer, making them more difficult to position. Advancing the needle without its stylet in place may increase the chances of successful and nontraumatic CSF collection [Nigrovic et al., 2007], but has been discouraged because of concern that this will introduce cells that might later form a spinal epidermoid tumor [Ziv et al., 2004].
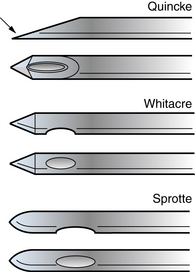
Fig. 10-5 The Quincke, Whitacre, and Sprotte needles.
The arrow indicates the sharp edge of the beveled tip of the Quincke needle.
(From Roos KL. Lumbar puncture. Semin Neurol 2003;23:105.)
The needle is gradually advanced, the ligamentum flavum and dura are pierced, and a slight popping sensation is felt as the subarachnoid space is entered. The stylet is removed to allow CSF to drain to the needle hub. If no fluid appears, the needle should be rotated a quarter-turn in case a nerve rootlet or strand of arachnoid is obstructing the opening. If this does not initiate CSF flow, the needle can be introduced a few millimeters more, sometimes resulting in a second popping sensation. If there is still no CSF, the stylet should be replaced and the needle slowly withdrawn for redirection of the needle toward the same interspace or for selection of another interspace [Roos, 2003].
Normal opening pressures in adults and older children generally range from 120 to 200 mm H2O. Pressures as high as 250 mm H2O in obese adults are also considered normal [Corbett and Mehta, 1983]. In a young, relaxed child who is in the lateral recumbent position, normal CSF pressures range from 50 to 200 mm H2O when the neck and legs are extended, but can rise to 100–280 mm H2O when the neck and legs are flexed [Clark et al., 2000]. Normal pressures in newborns vary between 90 and 120 mm H2O [Volpe, 2001]. Pathologic elevations of the CSF pressure are discussed in Chapter 77.
Replacing the stylet before withdrawing the spinal needle is recommended to avoid pulling a strand of arachnoid through the dura, creating a channel for continued CSF leakage and increasing the risk of postprocedural headache [Strupp et al., 1998]. The clinician should carefully document the details of the LP in the medical record.
Complications
More serious complications of LP do occur, although rarely [Evans, 1998]. These mainly involve subarachnoid, subdural, and epidural hematomas resulting from a breach of the epidural venous plexus, radicular arteries, or radicular veins [Adler et al., 2001]. Patients commonly have signs and symptoms of meningeal irritation or local pain. Severe symptoms, such as sensory, motor, or sphincter dysfunction, suggest the need for surgical hematoma evacuation [Kirkpatrick and Goodman, 1975]. An epidural CSF collection can cause symptoms of spinal compression which can usually be managed conservatively [Aronson and Zonfrillo, 2009].
Infectious complications, including meningitis, epidural abscess, and osteomyelitis, can result from the use of incompletely aseptic technique. Experiments have confirmed that LP in bacteremic animals can cause meningitis, but only with high colony counts and before the administration of antibiotics [Carp and Bailey, 1992]. Concern that patients with acute leukemia may have malignant cells introduced into the CSF by LP has not been supported by experimental or clinical evidence. Neither of these theoretical hazards should cause LP to be withheld from patients with suspected meningitis [Shinefield, 1975].
The most common complications of LP are back pain and postprocedural headache. Postprocedural headache may occur within 72 hours of the puncture, may be worsened by an upright position and relieved by recumbency (orthostatic), and may be associated with neck stiffness, nausea, vomiting, and cranial nerve palsies. Headache complicates LP in approximately one-third of patients [Ebinger et al., 2004a]. Symptoms usually resolve spontaneously after a few days, but protracted and complicated cases occasionally are reported. Postprocedural headache is thought to be caused by on-going CSF leakage through a tear in the dura, low intracranial pressure, and downward displacement of pain-sensitive intracranial structures [Ferrante et al., 2004]. MRI can demonstrate loss of intracranial CSF volume 24 hours after LP. Very large reductions are associated with headache, but many patients are symptomatic despite having neither visible change in CSF volume nor measurable change in brain position [Grant et al., 1991]. MRI studies reveal diffuse meningeal and dural venous sinus contrast enhancement (Figure 10-6), suggesting that a low CSF volume induces a painful compensatory venous sinus engorgement [Hannerz et al., 1999].
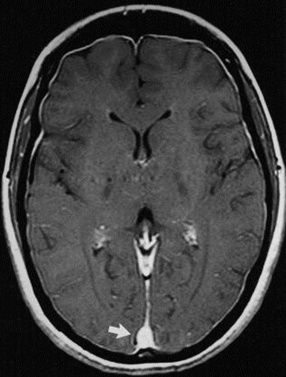
Fig. 10-6 Intracranial hypotension.
(From Nakshi R, Mechtler LL, Kamran S, et al. MRI findings in lumbar puncture headache syndrome. Clin Imaging 1999;23:73.)
An identical clinical and radiologic picture is occasionally seen in patients with a documented low CSF pressure (<60 mm H2O) and no history of an LP or other known source of a dural tear, such as head trauma or surgery. Patients with this syndrome of spontaneous intracranial hypotension are often found to have developed a spontaneous CSF leak, documented by CT or MR myelography or by radioisotope or gadolinium cisternography [Gordon, 2009b]. Spontaneous intracranial hypotension sometimes occurs in patients with the known or suspected connective tissue disorders such as Marfan’s syndrome or Ehlers–Danlos syndrome [Mokri et al., 2002]. Symptomatic CSF leakage and hypovolemia has been associated with normal CSF pressures [Miyazawa et al., 2003]. Spontaneous intracranial hypotension is often confused with other chronic headache disorders, delaying effective therapy [Schievink, 2003]. Although CSF hypovolemia usually runs a benign course, rare, life-threatening complications have been reported, including diencephalic compression and subdural hematomas [Schievink, 2008]. When bed rest is ineffective, treatment options include epidural injection of autologous blood or fibrin glue, continuous epidural saline infusion, and direct surgical repair of an identified site of leakage [Cousins et al., 2004].
Several technical aspects and patient factors appear to lessen the incidence and severity of postprocedural headache in adults, including the use of small-diameter needles [Tourtellotte et al., 1972] or atraumatic needles [Strupp et al., 2001], low body mass index, male gender, and lack of a prior history of headache [Evans et al., 2000]. Bed rest after LP has not been found to prevent headache, but it is effective in reducing pain after it has developed [Ebinger et al., 2004b]. Similarly, prophylactic epidural blood patches do not prevent headaches but are very successful in the treatment of refractory postprocedural headaches [van Kooten et al., 2008]. Studies have been contradictory or negative regarding the use of steroids, hydration, or caffeine for the prevention or treatment of postprocedural headaches [Ahmed et al., 2006; Lee et al., 2007].
Cerebrospinal Fluid Analysis
Appearance
The appearance of the CSF should be studied and recorded. Normal CSF is clear and colorless, indistinguishable from water when held up to white light in identical tubes. The presence of cells can be assessed by looking for Tyndall’s effect, a snowy scattering of direct sunlight when the tube is held against a dark background and the bottom is flicked with a finger [Simon and Abele, 1978]. This test can be positive with cell counts of less than 50 cells/mm3. Higher cell counts (i.e., more than 200 leukocytes/mm3 or 400 red blood cells/mm3) are readily perceived, but the eye cannot easily distinguish between the contribution of leukocytes and red blood cells, and this test cannot replace laboratory analysis. With red blood cell counts between 500 and 6000 cells/mm3, the CSF color becomes pink or xanthochromic. Xanthochromia, a yellowish discoloration of the CSF, is seen with the presence of red blood cell breakdown products such as oxyhemoglobin, bilirubin, and methemoglobin, each of which can be quantified by spectrophotometry. In the context of acute subarachnoid hemorrhage, bilirubin has traditionally been thought to appear first after 10 hours, reach a maximum at 48 hours, and persist for up to 2–4 weeks [Walton, 1956]. Later in vitro studies have added further caution to the interpretation of xanthochromia, suggesting that it can develop in less than 2 hours with the addition to CSF of less than 10,000 red blood cells/mm3, and can develop immediately with the addition of 30,000 red blood cells/mm3 or more [Graves and Sidman, 2004].
Cells
Counting chambers are used for quantitation of red blood cells and white blood cells within the CSF. Such automated devices can also provide fairly accurate differentials for the types of white blood cells present, but a pathologist should review a stained slide to provide confirmation. A small number of red blood cells can be found in the fluid of normal older children and adults, even in the absence of a traumatic puncture. Even higher numbers of red blood cells can be found in normal newborns, with a mean of 120 red blood cells/mm3. Infants without proven CNS pathology have also been found to have a higher number of total white blood cells and a higher percentage of polymorphonuclear cells than is found in older children [Nascimento-Carvalho and Moreno-Carvalho, 1998]. Older children have normal values similar to those of adults [Dyken and Mulina, 1973]. The normal values determined by these studies are shown in Table 10-2.
Table 10-2 Total White Blood Cell Counts in the Cerebrospinal Fluid of Children without Central Nervous System Disease
Age | N | WBC Count* |
---|---|---|
Neonate | ||
Premature (28–37 wk GA) | 38 | 5.1 ± 5.8 |
Term (38–42 wk GA) | 25 | 4.5 ± 3.6 |
Infant | ||
<6 weeks | 64 | 3.7 ± 3.4 |
6 weeks to 3 months | 67 | 2.9 ± 2.9 |
3–6 months | 84 | 1.9 ± 2.0 |
6–12 months | 75 | 2.6 ± 2.5 |
Child | 81 | 1.9 ± 2.7 |
Juvenile and adult* | 2.6 ± 1.7 |
The juvenile and adult level is provided for comparison.
* White blood cell counts (cells/mm3) are reported as means ± standard deviations.WBC, white blood cell; wk GA, gestational age in weeks.
Centrifugation and staining of the CSF is suitable for most clinical cytology, but filtration and sedimentation techniques better preserve cell morphology and are preferred for the precise identification of abnormal cells, particularly tumor cells [Herndon and Brumback, 1989]. The white blood cells found in normal CSF are typically 70 percent lymphocytes and 30 percent monocytes. An elevated white blood cell count or more than a few polymorphonuclear cells may indicate inflammation or infection within the CNS, but counts are not specific enough to allow ready differentiation, for example, of bacterial from viral meningitis. This point is examined in detail in Chapters 80–82 on infections of the nervous system. Finding an elevated polymorphonuclear cell count despite a normal white blood cell count is common among children with systemic infections without apparent CNS involvement [Naqvi et al., 1983]. Reports differ about whether seizures are associated with elevated white blood cell and polymorphonuclear cell counts [Woody et al., 1988]. Neoplastic cells, plasma cells, stem cells, and eosinophils within the CSF are always considered abnormal. However, because cytologic analysis has such a low sensitivity, CSF testing for leptomeningeal metastasis of a systemic malignancy should be repeated multiple times and should employ large collection volumes [DeAngelis and Cairncross, 2002].
Excessive red cells in CSF can be diagnostic of subarachnoid hemorrhage but can also occur with a traumatic LP. Clot formation within the tube of CSF is quite unusual after subarachnoid hemorrhage but is common after traumatic puncture. Analysis of the supernatant fluid of centrifuged CSF for xanthochromia, if sufficient time has passed to allow for red blood cell breakdown, can help in differentiating the two possibilities, with additional attention paid to the red blood cell concentration. There is some validity to comparing red blood cell counts in serial tubes, because the counts always decrease in cases of traumatic puncture. This analysis must be informed by a realization that traumatic puncture can and does occur in patients with true subarachnoid hemorrhage. The possibility of subarachnoid hemorrhage should not be considered excluded by this method unless the later tubes have a red blood cell count approaching zero [Shah and Edlow, 2002].
A formula for the correction of the white blood cell count in patients with suspected traumatic LP is widely used. The expected number of white blood cells is calculated based on the assumption that the red blood cell count is purely a reflection of peripheral blood contamination. In patients with a concurrently normal hemogram, this assumption predicts 1 white blood cell/mm3 (CSF) for every 700 red blood cell/mm3 (CSF). In patients with an abnormal hemogram, the direct calculation is corrected by the observed plasma ratio, predicting white blood cell (CSF) = red blood cell (CSF) × [white blood cell (plasma)/red blood cell (plasma)]. Although the rigid application of this method has been justifiably questioned [Novak, 1984], children with meningitis and a traumatic puncture are unlikely to be misdiagnosed through overcorrection as their white blood cell counts frequently are more than 10 times that predicted by this formula. Children with meningitis usually have other clinical or CSF findings that suggest the diagnosis [Bonadio et al., 1990].
Microorganisms
Additional studies that are available for the rapid identification of microorganisms include counterimmunoelectrophoresis, latex agglutination, and limulus lysate assays. Adjunctive bacterial antigen detection tests, especially the more accurate latex agglutination test, have had diagnostic value in patients who had been treated with antibiotics before LP for suspected meningitis [Bhisitkul et al., 1994]. Other studies have strongly questioned the value of such tests, finding high false-positive rates, low sensitivity, or no added benefit over Gram staining and culture [Nigrovic et al., 2004]. Tests to distinguish pyogenic from viral infections, including C-reactive protein [Putto et al., 1986] and lactate [Jordan et al., 1983], demonstrate some specificity but not enough for their clinical application.
Immunologic tests for antigens and antibodies and polymerase chain reaction tests for DNA and RNA can greatly aid in the identification of organisms that are difficult to culture [Greenlee, 1990]. These techniques can be applied to cases of bacterial meningitis but are particularly useful in the evaluation of immunocompromised hosts, encephalitis, and chronic meningitis, when viral, fungal, rickettsial, and other unusual organisms are more likely to be identified.
Glucose
Facilitated transporters on the membranes of capillary endothelial cells allow glucose to enter the interstitial fluid of the brain [Fishman, 1964]. With normal plasma glucose concentrations between 70 and 120 mg/dL, normal CSF glucose concentrations range between 45 and 80 mg/dL, or about two-thirds of the plasma glucose concentration. Glucose readily equilibrates between the CSF and interstitial fluid, and the decline from plasma levels is thought to be a sign of the high cerebral metabolic rate for glucose. A CSF glucose concentration below 40 mg/dL (i.e., hypoglycorrhachia) or a ratio of less than two-thirds of the plasma glucose level is considered abnormal.
Very low CSF glucose values, sometimes below the limits of laboratory detection, are most characteristic of acute bacterial meningitis, but mildly to moderately low values are commonly seen in fungal, parasitic, protozoal, spirochetal, and tuberculous meningitis. A low CSF glucose level is uncommon in viral meningitis but has been reported in association with mumps, herpes simplex, and herpes zoster infections [Donald et al., 1987; Mommeja-Marin et al., 2003]. Besides plasma hypoglycemia, there are other noninfectious causes of low CSF glucose, including meningeal carcinomatosis, sarcoidosis, chemical meningitis, and subarachnoid hemorrhage. In diverse causes of meningeal inflammation, a low CSF glucose level probably results from a combination of increased glucose use by the brain and spinal cord adjacent to the CSF; from glucose use by leukocytes within the CSF, especially polymorphonuclear cells; and from inhibition of glucose transport into the CSF.
In the setting of plasma hyperglycemia, the saturability of the endothelial glucose transporters allows for only a partial parallel increase in the CSF glucose concentration. Diabetic patients without signs of meningitis can have CSF to plasma glucose ratios of less than one-third [Powers, 1981]. Acute changes in the plasma glucose concentration are maximally reflected in the CSF concentration after 2 hours, with equilibration after a transient change requiring up to 4 hours. Optimal interpretation of the CSF glucose level therefore requires a simultaneous plasma glucose measurement, after the patient fasts for 4 hours.
Defective transport of glucose across the blood–brain barrier was initially proposed as a novel mechanism of disease in two infants with low CSF glucose concentrations, low CSF lactate levels, and epileptic encephalopathy [De Vivo et al., 1991]. This theory was borne out by discovery of mutations in the SLC2A1 gene (formerly designated GLUT1) for the cerebral endothelial glucose transporter [Seidner et al., 1998]. Defects in SLC2A1 have since been found in children and adults with a variety of neurologic symptoms [Brockmann, 2009]. In the classic phenotype, seizures begin within the first 4 months of life in previously healthy and normal infants. Other paroxysmal events may be observed over time, including apneic episodes, opsoclonus, ataxia, confusion, lethargy, alternating hemiparesis, total body paralysis, abnormal movements or postures, sleep disturbances, and headaches. Deceleration of head growth, with acquired microcephaly in most patients, and cognitive impairment ranging from learning disabilities to severe mental retardation have also been described. The paroxysmal events may be exacerbated by fasting and fatigue, and may respond poorly to conventional antiepileptic drugs. In vitro tests have revealed aggravation of the SLC2A1 transport defect by barbiturates and methylxanthines, suggesting that phenobarbital and caffeinated foods be avoided. The ketogenic diet is highly effective in controlling seizures but does not appear to improve the neurobehavioral outcome of this condition. Nonclassic phenotypes include patients without clinical seizures who have predominant symptoms of mental retardation, ataxia, spasticity, choreoathetosis, or dystonia. A low CSF glucose level (<40 mg/dL) and a low–normal or low CSF lactate concentration (<1.4 nM) in the absence of systemic hypoglycemia are virtually diagnostic. Positron emission tomography (PET) scans have demonstrated very characteristic patterns of global reductions in glucose uptake, with more severe hypometabolism found in the medial temporal lobes and thalamic nuclei. Measurement of the glucose uptake into erythrocytes, which depends on the same SLC2A1 transporter, provides further confirmatory testing. Genetic testing with fluorescence in situ hybridization for microdeletions has a low sensitivity and must be followed by full genomic analysis [Pascual et al., 2004].
Protein
The total protein content of the CSF varies in children with their age. It is highest at birth and falls over the first year of life before gradually climbing toward its adult value [Widell, 1958]. The protein concentrations obtained by LP in healthy subjects are displayed in Table 10-3 and Figure 10-7. Protein levels also vary by the anatomic area from which the CSF is sampled. In normal infants and adults, the CSF protein concentration is 6–15 mg/dL in the ventricles, 15–25 mg/dL in the cisterna magna, and 20–50 mg/dL in the lumbar sac [Merritt and Fremont-Smith, 1938]. This concentration gradient results from regional differences in the permeability of the blood–CSF barrier.
Table 10-3 Total Protein Content in the Cerebrospinal Fluid of Healthy Children
Age | N | Protein* |
---|---|---|
0–6 days | 11 | 80.9 ± 20.8 |
7–13 days | 7 | 70.4 ± 23.6 |
14–27 days | 13 | 53.9 ± 17.8 |
28–41 days | 10 | 46.5 ± 15.4 |
42–59 days | 11 | 45.6 ± 14.2 |
2–4 months | 9 | 34.8 ± 12 |
5–8 months | 7 | 20.4 ± 2.4 |
9–11 months | 5 | 16.6 ± 2.6 |
12–23 months | 8 | 16.8 ± 2.7 |
2–7 years | 21 | 17.7 ± 3.1 |
8–13 years | 19 | 20.8 ± 4.1 |
* Protein concentrations (mg/dL) are reported as means ± standard deviations.
A slight increase in lumbar CSF protein content, between 45 mg/dL and 75 mg/dL in older infants and adults, occurs with multiple neurologic conditions associated with blood–brain barrier breakdown, a process often visualized on neuroimaging as contrast enhancement and vasogenic cerebral edema. Higher CSF protein concentrations (>500 mg/dL) occur in patients with meningitis and subarachnoid hemorrhage, and these levels may decrease the rate of protein absorption from the CSF, leading to further elevation of protein levels [Prockop and Fishman, 1968]. With a spinal cord tumor, arachnoiditis, and other reasons for complete spinal CSF obstruction, very high CSF protein levels are caused by poor CSF outflow and local breakdown of the blood–brain barrier. A loculated CSF collection with sufficient fibrinogen content to allow clot formation, frequently seen when the total protein concentration rises above 1 g/dL, is known as Froin’s syndrome. Table 10-4 shows the elevations in CSF protein content found in adults with various neurologic disorders [Merritt and Fremont-Smith, 1938].
Electrophoresis separates plasma and CSF proteins into groups of similar molecular weight and electrical charge, classically defined as the prealbumin (transthyretin), albumin, and globulin (alpha1, alpha2, beta1, beta2, tau, and gamma) fractions. Such testing demonstrates that, compared with plasma, normal CSF has relatively higher transthyretin and tau globulin fractions and a lower gamma globulin fraction [Werner, 1969]. This CSF-specific electrophoresis pattern has been exploited for the identification of CSF otorrhea and rhinorrhea [Porter et al., 1992]. Transthyretin is made by the choroid plexus, accounting for its increased concentration in the CSF [Herbert et al., 1986].
Changes in the CSF concentrations of a number of specific proteins can accompany brain injury from inflammatory, traumatic, cerebrovascular, epileptic, and neurodegenerative disorders. Several such biomarkers have been investigated, including the 72-kDa heat shock protein (HSP70), aggregated beta amyloid, neuron-specific enolase, S-100 beta, tau protein, vascular endothelial growth factor, glial fibrillary acidic protein, brain-derived growth factor and nerve growth factor, apolipoprotein E, interleukin-6, soluble FAS receptor, and BCL2 [Ramaswamy et al., 2009]. Other chemicals altered in patients with significant brain injury include free fatty acids [Pilitsis et al., 2003], the lipid peroxidation marker F2-isoprostane [Varma et al., 2003], and the excitatory neurotransmitters glutamate and glycine [Zhang et al., 2001]. Such detailed CSF analysis has added to our understanding of the pathophysiology of brain injury and holds great potential for future clinical use in diagnosis and prognosis [Ramaswamy et al., 2009].
Immunologic Analysis
Testing for oligoclonal bands uses isoelectric focusing and immunolabeling to look for discrete separation of the CSF IgG. This separation occurs when clonal expansion of activated B cells leads to the production of IgG antibodies against a small set of antigens. In normal test subjects, IgG is homogeneously distributed, or it forms a monoclonal band [Wurster, 2004]. The presence of more than two clonal bands suggests an active humoral immune response. If oligoclonal bands are seen in the CSF but not in a simultaneously collected plasma sample, the immune response is considered to be of intrathecal origin [Siemes et al., 1981]. An example of a positive test result for intrathecal oligoclonal bands is provided in Figure 10-8.
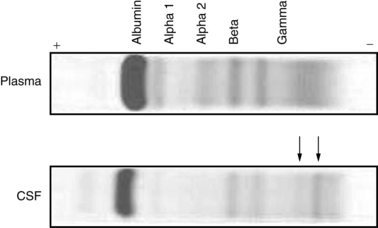
Fig. 10-8 CSF protein oligoclonal bands.
(Courtesy of Dr. Mark Braun, Indiana University School of Medicine, accessed at http://medsci.indiana.edu/c602web/602/start.htm.)
The IgG index compares simultaneous measurements of plasma and CSF IgG and albumin concentrations to estimate the amount of intrathecal IgG production [Tourtellotte et al., 1980]. The IgG index calculation is simply the ratio of the CSF and plasma IgG concentrations divided by the ratio of the CSF and plasma albumin concentrations:
Among patients with clinically definite multiple sclerosis, for example, up to 95 percent have intrathecal oligoclonal bands, and more than 70 percent have an elevated IgG index. Such tests are highly sensitive and highly predictive of multiple sclerosis when supported by appropriate clinical and radiologic findings. However, they are nonspecific in isolation. Positive intrathecal oligoclonal bands are demonstrated for patients with postinfectious encephalomyelitis, subacute sclerosing panencephalitis, progressive multifocal leukoencephalopathy, human immunodeficiency virus encephalitis, Lyme disease, and other disorders from which multiple sclerosis must sometimes be differentiated [Olsson, 1994]. Some reports suggest that more detailed analysis of CSF immunoglobulins, such as measuring the kappa to lambda ratio of immunoglobulin free light chains, may be useful in the diagnosis and prognosis of multiple sclerosis [Jenkins et al., 2001]. Specific antineuronal antibodies may be found in a wide range of neurologic conditions but most commonly are associated with paraneoplastic diseases [Vianello et al., 2004].
Neurometabolic Studies
The usual laboratory evaluation of a child with unexplained encephalopathy consists of plasma, urine, and radiologic studies. When CSF is collected as a part of the evaluation, metabolic tests can be expanded to include lactate, pyruvate, 5-methyltetrahydrofolate, and quantitative amino acid measurement [Gordon, 2009a]. Proper collection and handling of the CSF is even more important in this context to avoid spurious test results. Some syndromic presentations suggest additional CSF tests. For example, organic acid analysis of the CSF is indicated when a child has a presentation suggesting an organic aciduria but has had repeatedly normal plasma and urine test results. Suspected early-onset nonketotic hyperglycinemia is confirmed by an elevated CSF glycine concentration or CSF to plasma glycine ratio on a quantitative test for CSF amino acids [Applegarth and Toone, 2001]. Patients with neuroimaging features of the Aicardi–Goutières syndrome, a leukoencephalopathy of infantile onset with progressive basal ganglia calcification, can be tested for CSF interferon-alpha elevation and lymphocytosis [Lanzi et al., 2003].
Pediatric neurotransmitter diseases (see Chapter 39) may be suspected in patients with unexplained seizures, encephalopathy, dysautonomia, extrapyramidal symptoms, or intermittent ocular abnormalities, such as oculogyric crises or convergence spasms [Hyland, 2003]. Defects have been recognized in most of the enzymes involved in monoamine neurotransmitter synthesis, including tryptophan hydroxylase, which converts tryptophan to 5-hydroxytryptophan (5-HT); tyrosine hydroxylase, which converts tyrosine to l-hydroxyphenylalanine (l-dopa); and aromatic l-amino acid decarboxylase, which converts 5-HT to serotonin and l-dopa to dopamine. Tetrahydrobiopterin (BH4) is a crucial co-factor for the tryptophan and tyrosine hydroxylases, and neurotransmitter disorders may arise from defective BH4 synthesis from neopterin, as occurs in guanosine triphosphate-cyclohydrolase I deficiency (i.e., Segawa’s disease), or from defective recycling of dihydrobiopterin (BH2), as with dihydropteridine reductase deficiency. These disorders can usually be distinguished using clinically available CSF tests for BH4, neopterin, and the neurotransmitter metabolites 5-hydroxyindolacetic acid (5-HIAA, derived from serotonin), homovanillic acid (derived from dopamine), and ortho-methyldopa (derived from l-dopa). CSF samples for these tests must be carefully collected into specially treated tubes and snap-frozen at the bedside in dry ice or liquid nitrogen.
Disordered gamma-aminobutyric acid (GABA) synthesis may result from succinic semialdehyde dehydrogenase deficiency, which can be diagnosed through careful urine or CSF testing for GABA hydroxybutyrate. The diagnosis of less common GABA disorders, such as GABA transaminase deficiency and homocarnosinosis, primarily depends on CSF testing [Pearl and Gibson, 2004]. Details of the indications for and interpretation of these and other metabolic tests are discussed in later chapters of this textbook.
References
The complete list of references for this chapter is available online at www.expertconsult.com.
Abolnik I.Z., Eaton J.V., Sexton D.J. Propionibacterium acnes vertebral osteomyelitis following lumbar puncture: case report and review. Clin Infect Dis. 1995;21:694.
Adler M.D., Comi A.E., Walker A.R. Acute hemorrhagic complication of diagnostic lumbar puncture. Pediatr Emerg Care. 2001;17:184.
Ahmed S.V., Jayawarna C., Jude E. Post lumbar puncture headache: diagnosis and management. Postgrad Med J. 2006;82:713.
Applegarth D., Toone J. Nonketotic hyperglycinemia (glycine encephalopathy): Laboratory diagnosis. Mol Genet Metab. 2001;74:139.
Aronson P.L., Zonfrillo M.R. Epidural cerebrospinal fluid collection after lumbar puncture. Pediatr Emerg Care. 2009;25:467.
Bhisitkul D., Hogan A., Tanz R. The role of bacterial antigen detection tests in the diagnosis of bacterial meningitis. Pediatr Emerg Care. 1994;10:67.
Bonadio W., Smith D., Goddard S. Distinguishing cerebrospinal fluid abnormalities in children with bacterial meningitis and traumatic lumbar puncture. J Infect Dis. 1990;162:251.
Brockmann K. The expanding phenotype of GLUT1-deficiency syndrome. Brain Dev. 2009;31:545.
Carp H., Bailey S. The association between meningitis and dural puncture in bacteremic rats. Anesthesiology. 1992;76:739.
Clark R., Kochanek P., Adelson P. Increases in BCl-2 protein in cerebrospinal fluid and evidence for programmed cell death in infants and children after severe traumatic brain injury. J Pediatr. 2000;137:197.
Corbett J., Mehta M. Cerebrospinal fluid pressure in normal obese subjects and patients with pseudotumor cerebri. Neurology. 1983;33:1386.
Cousins M., Brazier D., Cook R. Intracranial hypotension caused by cervical cerebrospinal fluid leak: Treatment with epidural blood patch. Anesth Analg. 2004;98:1794.
Cowan F., Whitelaw A. Acute effects of acetazolamide on cerebral blood flow velocity and Pco2 in the newborn infant. Acta Pediatr Scand. 1991;80:22.
Cserr H., Cooper D., Milhorat T. Flow of cerebral interstitial fluid as indicated by the removal of extracellular markers from rat caudate nucleus. In: Bito L., Davson H., editors. The ocular and cerebrospinal fluids. New York: New York Academic Press, 1977.
Davson H., Welch K., Segal H. The physiology and pathophysiology of the cerebrospinal fluid. New York: Churchill Livingston; 1987.
De Vivo D., Triflletti R., Jacobson R. Defective glucose transport across the blood-brain barrier as a cause of persistent hypoglycorrhachia, seizures, and developmental delay. N Engl J Med. 1991;325:703.
DeAngelis L., Cairncross J. A better way to find tumor in the CSF? Neurology. 2002;58:339.
Donald P., Burger P., Becker W. Mumps meningo-encephalitis. S Afr Med J. 1987;71:283.
Dyken P., Mulina J. The normal cellular population of the cerebrospinal fluid by sedimentation-flocculation techniques at various ages. Proc Child Neurol Soc. 1973;2:26.
Ebinger F., Kosel C., Pietz J., et al. Headache and backache after lumbar puncture in children and adolescents: a prospective study. Pediatrics. 2004;113:1588.
Ebinger F., Kosel C., Pietz J., et al. Strict bed rest following lumbar puncture in children and adolescents is of no benefit. Neurology. 2004;62:1003.
Evans R. Complications of lumbar puncture. Neurol Clin. 1998;16:83.
Evans R.W., Armon C., Frohman E.M., et al. Assessment: prevention of post-lumbar puncture headaches: report of the therapeutics and technology assessment subcommittee of the american academy of neurology. Neurology. 2000;55:909.
Faraci F., Kinzenbaw D., Heistad D. Effect of endogenous vasopressin on blood flow to choroid plexus during hypoxia and intracranial hypertension. Am J Physiol. 1994;266:H393.
Ferrante E., Savino A., Sances G., et al. Spontaneous intracranial hypotension syndrome: Report of twelve cases. Headache. 2004;44:615.
Fishman R. Carrier transport of glucose between blood and the cerebrospinal fluid. Am J Physiol. 1964;206:836.
Fishman R. Cerebrospinal fluid in diseases of the nervous system, ed 2. Philadelphia: WB Saunders; 1992.
Go K. The normal and pathological physiology of brain water. Adv Tech Stand Neurosurg. 1997;23:47.
Gordon N. Cerebral folate deficiency. Dev Med Child Neurol. 2009;51:180.
Gordon N. Spontaneous intracranial hypotension. Dev Med Child Neurol. 2009;51:932.
Grant R., Condon B., Hart I. Changes in intracranial CSF volume after lumbar puncture and their relationship to post-LP headache. J Neurol Neurosurg Psychiatry. 1991;54:440.
Graves P., Sidman R. Xanthochromia is not pathognomonic for subarachnoid hemorrhage. Acad Emerg Med. 2004;11:131.
Greenlee J. Approach to diagnosis of meningitis: Cerebrospinal fluid evaluation. Infect Dis Clin North Am. 1990;4:583.
Hannerz J., Ericson K., Skejo H. MR imaging with gadolinium in patients with and without post-lumbar puncture headache. Acta Radiol. 1999;40:135.
Hasbun R., Abrahams J., Jekel J. Computed tomography of the head before lumbar puncture in adults with suspected meningitis. N Engl J Med. 2001;345:1727.
Herbert J., Wilcox J., Kim-Thu C. Transthyretin: A choroid plexus-specific transport protein in human brain. Neurology. 1986;36:900.
Herndon R., Brumback R. The cerebrospinal fluid. Boston: Kluwer Academic Publishers; 1989.
Hochwald G. Cerebrospinal fluid. In: Baker A., Baker L., editors. Clinical neurology. New York: Harper and Row, 1984.
Howard S.C., Gajjar A., Ribeiro R.C., et al. Safety of lumbar puncture for children with acute lymphoblastic leukemia and thrombocytopenia. JAMA. 2000;284:2222.
Hyland K. The lumbar puncture for diagnosis of pediatric neurotransmitter diseases. Ann Neurol. 2003;54:S13.
Jenkins M., Cheng L., Ratnaike S. Multiple sclerosis: Use of light-chain typing to assist diagnosis. Ann Clin Biochem. 2001;38:235.
Johanson C., Palm D., Dyas M. Microdialysis analysis of effects of loop diuretics and acetazolamide on chloride transport from blood to CSF. Brain Res. 1994;641:121.
Johnston M., Zakharov A., Papaiconomou C., et al. Evidence of connections between cerebrospinal fluid and nasal lymphatic vessels in humans, non-human primates and other mammalian species. Cerebrospinal Fluid Res. 2004;1:2.
Jordan G., Statland B., Halsted C. CSF lactate in diseases of the CNS. Arch Intern Med. 1983;143:85.
Kaur G., Gupta P., Kumar A. A randomized trial of eutectic mixture of local anesthetics during lumbar puncture in newborns. Arch Pediatr Adolesc Med. 2003;157:1065.
Kirkpatrick D., Goodman S. Combined sub-arachnoid and subdural spinal hematoma following spinal puncture. Surg Neurol. 1975;3:109.
Koh L., Zakharov A., Johnston M. Integration of the subarachnoid space and lymphatics: is it time to embrace a new concept of cerebrospinal fluid absorption? Cerebrospinal Fluid Res. 2005;2:6.
Kozlowski G. Ventricular route hypothesis and peptide-containing structures of the cerebroventricular system. Front Horm Res. 1982;9:105.
Lanzi G., D’Arrigo S., Drumbl G. Aicardi-Goutieres syndrome: Differential diagnosis and aetiopathogenesis. Funct Neurol. 2003;18:71.
Lee L.C., Sennett M., Erickson J.M. Prevention and management of post-lumbar puncture headache in pediatric oncology patients. J Pediatr Oncol Nurs. 2007;24:200.
Lindvall M., Owman C. Autonomic nerves in the mammalian choroid plexus and their influence on the formation of cerebrospinal fluid. J Cereb Blood Flow Metab. 1981;1:245.
Macaulay N., Zeuthen T. Water transport between CNS compartments: contributions of aquaporins and cotransporters. Neuroscience Jul 28. 2010;168(4):941-956. Epub Sep 15, 2009
Merritt H., Fremont-Smith F. The cerebrospinal fluid. Philadelphia: WB Saunders; 1938.
Miyazawa K., Shiga Y., Hasegawa T. CSF hypovolemia vs. intracranial hypertension in “spontaneous intracranial hypotension syndrome”. Neurology. 2003;60:941.
Mokri B., Maher C., Sencakova D. Spontaneous CSF leaks: Underlying disorder of connective tissue. Neurology. 2002;58:814.
Mommeja-Marin H., Lafaurie M., Scieux C. Herpes simplex virus type 2 as a cause of severe meningitis in immunocompromised adults. Clin Infect Dis. 2003;37:1527.
Naqvi S., Dunkle L., Naseer S. Significance of neutrophils in cerebrospinal fluid samples processed by cytocentrifugation. Clin Pediatr (Phila). 1983;22:608.
Nascimento-Carvalho C., Moreno-Carvalho O. Normal cerebrospinal fluid values in full-term gestation and premature neonates. Arq Neuropsiquiatr. 1998;56:375.
Nigrovic L., Kuppermann N., McAdam A., et al. Cerebrospinal latex agglutination fails to contribute to the microbiologic diagnosis of pretreated children with meningitis. Pediatr Infect Dis J. 2004;23:786.
Nigrovic L.E., Kuppermann N., Neuman M.I. Risk factors for traumatic or unsuccessful lumbar punctures in children. Ann Emerg Med. 2007;49:762.
Nilsson C., Lindvall-Axelsson M., Owman C. Neuroendocrine regulatory mechanisms in the choroid plexus-cerebrospinal fluid system. Brain Res Rev. 1992;17:109.
Nogradi A., Kelly C., Carter N. Localization of acetazolamide-resistant carbonic anhydrase III in human and rat choroid plexus by immunocytochemistry and in situ hybridization. Neurosci Lett. 1993;151:162.
Novak R. Lack of validity of standard corrections for white blood cell counts of blood-contaminated cerebrospinal fluid in infants. Am J Clin Pathol. 1984;82:95.
Oliver W., Shope T., Kuhns L. Fatal lumbar puncture: Fact versus fiction-an approach to a clinical dilemma. Pediatrics. 2003;112:e174.
Olsson T. Cerebrospinal fluid. Ann Neurol. 1994;36:S100.
Pascual J., Wang D., Lecumberri B. GLUT1 deficiency and other glucose transporter diseases. Eur J Endocrinol. 2004;150:627.
Pearl P., Gibson K. Clinical aspects of the disorders of GABA metabolism in children. Curr Opin Neurol. 2004;17:107.
Pilitsis J., Coplin W., O’Regan M. Free fatty acids in cerebrospinal fluids from patients with traumatic brain injury. Neurosci Lett. 2003;349:136.
Porter M., Brookes G., Zeman A., et al. Use of protein electrophoresis in the diagnosis of cerebrospinal fluid rhinorrhoea. J Laryngol Otol. 1992;106:504.
Powers W. Cerebrospinal fluid to plasma glucose ratios in diabetes mellitus and bacterial meningitis. Am J Med. 1981;71:217.
Prockop L., Fishman R. Experimental pneumococcal meningitis: Permeability changes influencing the concentration of sugars and macromolecules in cerebrospinal fluid. Arch Neurol. 1968;19:449.
Proescholdt M.G., Hutto B., Brady L.S., et al. Studies of cerebrospinal fluid flow and penetration into brain following lateral ventricle and cisterna magna injections of the tracer [14C]inulin in rat. Neuroscience. 2000;95:577.
Putto A., Ruuskanen O., Meurman O. C-reactive protein in the evaluation of febrile illness. Arch Dis Child. 1986;61:24.
Ramaswamy V., Horton J., Vandermeer B., et al. Systematic review of biomarkers of brain injury in term neonatal encephalopathy. Pediatr Neurol. 2009;40:215.
Roos K. Lumbar puncture. Semin Neurol. 2003;23:105.
Rosenberg G. Brain fluids and metabolism. New York: Oxford University Press; 1990.
Sadjadpour K. Hazards of anticoagulation therapy shortly after lumbar puncture. JAMA. 1977;237:1692.
Saifuddin A., Burnett S., White J. The variation of position of the conus medullaris in an adult population: A magnetic resonance imaging study. Spine. 1998;23:1452.
Saito Y., Wright E. Regulation of bicarbonate transport across the brush border membrane of the bull-frog choroid plexus. J Physiol. 1984;350:327.
Schievink W. Misdiagnosis of spontaneous intracranial hypotension. Arch Neurol. 2003;60:1713.
Schievink W.I. Spontaneous spinal cerebrospinal fluid leaks. Cephalalgia. 2008;28:1345.
Segal M. Extracellular and cerebrospinal fluid. J Inherit Metab Dis. 1993;16:617.
Seidner G., Alvarez M., Yeh J. GLUT-1 deficiency syndrome caused by haploinsufficiency of the blood-brain barrier. Nat Genet. 1998;18:188.
Shah K., Edlow J. Distinguishing traumatic lumbar puncture from true subarachnoid hemorrhage. J Emerg Med. 2002;23:67.
Shinefield H. Bacteremia, lumbar puncture and meningitis. Am J Dis Child. 1975;129:547.
Siemes H., Siegert M., Hanefeld F. Occurrence of oligoclonal gamma globulin in the CSF of children with prolonged and chronic CNS infections. Acta Paediatr Scand. 1981;70:91.
Silverman R., Kwiatkowski T., Bernstein S., et al. Safety of lumbar puncture in patients with hemophilia. Ann Emerg Med. 1993;22:1739.
Simon R., Abele J. Spinal-fluid pleocytosis estimated by the Tyndall effect. Ann Intern Med. 1978;89:75.
Sorensen P., Hammer M., Gjerris F. Cerebrospinal fluid vasopressin in benign intracranial hypertension. Neurology. 1982;32:1255.
Speake T., Whitwell C., Kajita H. Mechanisms of CSF secretion by the choroid plexus. Microsc Res Tech. 2001;52:49.
Spector R., Johanson C. The mammalian choroid plexus. Sci Am. 1989;261:68.
Strupp M., Brandt T., Muller A. Incidence of post-lumbar puncture syndrome reduced by reinserting the stylet: A randomized prospective study of 600 patients. J Neurol. 1998;245:589.
Strupp M., Schueler O., Straube A. “Atraumatic” Sprotte needle reduces the incidence of post-lumbar puncture headaches. Neurology. 2001;57:2310.
Szmydynger-Chodobska J., Chodobski A., Johanson C. Postnatal developmental changes in blood flow to choroid plexus and cerebral cortex of the rat. Am J Physiol. 1994;255:R1488.
Tait M.J., Saadoun S., Bell B.A., et al. Water movements in the brain: role of aquaporins. Trends Neurosci. 2008;31:37.
Tourtellotte W., Henderson W., Tucker R. A randomized double-blind clinical trial comparing the 22 versus 26 gauge needle in the production of the post-lumbar puncture syndrome in normal individual animals. Headache. 1972;12:73.
Tourtellotte W., Potvin A., Fleming J. Multiple sclerosis: Measurement and validation of central nervous system IgG synthesis rate. Neurology. 1980;30:240.
Tunkel A.R., Hartman B.J., Kaplan S.L., et al. Practice guidelines for the management of bacterial meningitis. Clin Infect Dis. 2004;39:1267.
van Kooten F., Oedit R., Bakker S.L., et al. Epidural blood patch in post dural puncture headache: a randomised, observer-blind, controlled clinical trial. J Neurol Neurosurg Psychiatry. 2008;79:553.
Vandam L. On the origins of intrathecal anesthesia. Int Anesthesiol Clin. 1989;27:2.
Varma S., Janesko K., Wisniewski S. F2-isoprostane and neuron-specific enolase in cerebrospinal fluid after severe traumatic brain injury in infants and children. J Neurotrauma. 2003;20:781.
Vates T.J., Bonting S., Oppelt W. Na-K activated adenosine triphosphatase formation of cerebrospinal fluid in the cat. Am J Physiol. 1964;206:1165.
Vianello M., Vitaliani R., Pezzani R. The spectrum of antineuronal autoantibodies in a series of neurological patients. J Neurol Sci. 2004;220:29.
Volpe J. Neurology of the newborn, ed 4. Philadelphia: WB Saunders; 2001.
Walton J. Subarachnoid hemorrhage. Edinburgh: Livingstone; 1956.
Welch K., Friedman V. The cerebrospinal fluid valves. Brain. 1960;83:454.
Werner M. A combined procedure for protein estimation and electrophoresis of cerebrospinal fluid. J Lab Clin Med. 1969;74:166.
Widell S. On the cerebrospinal fluid in normal children and in patients with acute abacterial meningoencephalitis. Acta Paediatr Scand. 1958;47(Suppl 115):1.
Woody R., Yamauchi T., Bolyard K. Cerebrospinal fluid cell counts in childhood idiopathic status epilepticus. Pediatr Infect Dis J. 1988;7:298.
Wurster U. The clinical significance of an intrathecal monoclonal immunoglobulin band: A follow-up study. Neurology. 2004;62:1237.
Yamada S., Miyazaki M., Kanazawa H., et al. Visualization of cerebrospinal fluid movement with spin labeling at MR imaging: preliminary results in normal and pathophysiologic conditions. Radiology. 2008;249:644.
Zhang H., Zhang X., Zhang T. Excitatory amino acids in cerebrospinal fluid of patients with acute head injuries. Clin Chem. 2001;47:1458.
Ziv E., McComb G., Krieger M. Iatrogenic intraspinal epidermoid tumor: Two cases and a review of the literature. Spine. 2004;29:E15.