Respiratory Failure and the Need for Ventilatory Support
After reading this chapter you will be able to:
Define acute respiratory failure.
Differentiate between hypoxemic respiratory failure (type I) and hypercapnic respiratory failure (type II).
Discuss the causes of acute respiratory failure.
Discuss the differences between chronic respiratory failure and acute-on-chronic respiratory failure.
Identify the complications of respiratory failure.
Discuss the indication for ventilatory support.
Discuss general management principles of hypoxemic and hypercapnic respiratory failure.
Respiratory failure is a clinical problem that all respiratory care practitioners must be skilled at identifying, assessing, and treating. A 1994 study of more than 1400 patients concluded that 44% of patients diagnosed with acute respiratory failure requiring intensive care unit (ICU) admission died in the hospital.1 A review of hospital discharge records from 2005 in six states in the United States showed only marginal improvement, with hospital mortality of 34.5%.2 The need for oxygen (O2) delivery, mechanical ventilation, and other modalities makes the respiratory therapist (RT) indispensable in the treatment of this life-threatening condition.
Respiratory failure is the “inability to maintain either the normal delivery of O2 to the tissues or the normal removal of carbon dioxide (CO2) from the tissues”3 and often results from an imbalance between respiratory workload and ventilatory strength or endurance. Criteria for respiratory failure based on arterial blood gases (ABGs) were established by Campbell4 and generally define failure as arterial partial pressure of oxygen (PaO2) less than 60 mm Hg or alveolar partial pressure of carbon dioxide (PaCO2) greater than 50 mm Hg (or both) in otherwise healthy individuals breathing room air at sea level. Respiratory failure can be an acute or a chronic process. Additionally and classically, it has also been separated into two other categories to reflect the type of physiologic impairment. Hypoxemic (type I) respiratory failure occurs when the primary problem is inadequate O2 delivery. Hypercapnic (type II) respiratory failure describes “bellows failure” of the lungs resulting in elevated CO2 levels. Hypercapnic respiratory failure is also known as ventilatory failure. Patients with baseline acid-base derangement (e.g., chronic obstructive pulmonary disease [COPD], restrictive lung disease) may be chronically hypercapnic and in chronic ventilatory failure based on the guidelines. These individuals develop acute failure when their chronic state deteriorates significantly; this is sometimes referred to as acute ventilatory failure superimposed on chronic ventilatory failure.
Hypoxemic Respiratory Failure (Type I)
The primary causes of hypoxemia are the following:
These entities are briefly discussed here and are discussed in more detail in Chapters 10 and 11.
Ventilation/Perfusion Mismatch
There are regions in healthy lungs where ventilation and perfusion are not evenly matched, so it seems logical that this is the most common cause of hypoxemia. RTs are familiar with this concept through the work of West,5 which described a high ratio at the apex of the lungs and a low ratio at the bases. This concept can be oversimplified and stated as there being more air than blood at the apices and more blood than air at the bases.
Pathologic mismatch occurs when disease disrupts this balance, and hypoxemia results (Figure 41-1, A). Most commonly, areas of low
ratio are seen in which ventilation is compromised despite adequate blood flow. Obstructive lung diseases are frequent causes. The bronchospasm, mucous plugging, inflammation, and premature airway closure that signal asthmatic or emphysematous exacerbations worsen ventilation and create
mismatch. Infection, heart failure, and inhalation injury may lead to partially collapsed or fluid-filled alveoli, also resulting in decreased ventilation and reduced blood O2 levels.
Clinical Presentation
Because patients present with hypoxemia, the initial goal is always to treat the low PaO2 or SpO2 (arterial O2 saturation by pulse oximeter). mismatch responds to supplemental O2 (see Figure 41-1, B). Hypoxemia commonly manifests with dyspnea, tachycardia, and tachypnea, but these are very nonspecific findings. However, patient observation is extremely valuable. The use of accessory muscles of respiration (scalene, pectoralis major, and sternomastoid) is an important sign that normal diaphragmatic inspiration is inadequate. In an elderly, cachectic, or barrel-chested individual who is leaning forward on his or her arms, COPD is the likely diagnosis. Nasal flaring may be present. Lower extremity edema is more indicative of cardiac failure as the cause of hypoxemia. Cyanosis may be peripheral and primarily due to decreased blood flow. Central cyanosis, seen most easily as a bluish tint around the lips, occurs when greater than 5 g/dl of unsaturated hemoglobin is present. This finding is more common in patients with polycythemia but may be subject to wide observer variability. More severe hypoxemia can lead to significant central nervous system (CNS) dysfunction, ranging from irritability to confusion to coma.
Shunt
Shunt is an extreme version of mismatch in which there is no ventilation to match perfusion (
= 0). About 2% to 3% of the blood supply is shunted via the bronchial and thebesian veins that feed the lungs and heart; this is normal anatomic shunt. Pathologic anatomic shunt occurs as a result of right-to-left blood flow through cardiac openings (e.g., atrial or ventricular septal defects) or in pulmonary arteriovenous malformations. Physiologic shunt leads to hypoxemia when alveoli collapse or are filled with fluid or exudate. Common etiologies of physiologic shunting include atelectasis, pulmonary edema, and pneumonia. In contrast to
mismatch, shunt does not respond to supplemental O2 because the gas exchange unit (the alveolus) is not open (Figure 41-2, A).
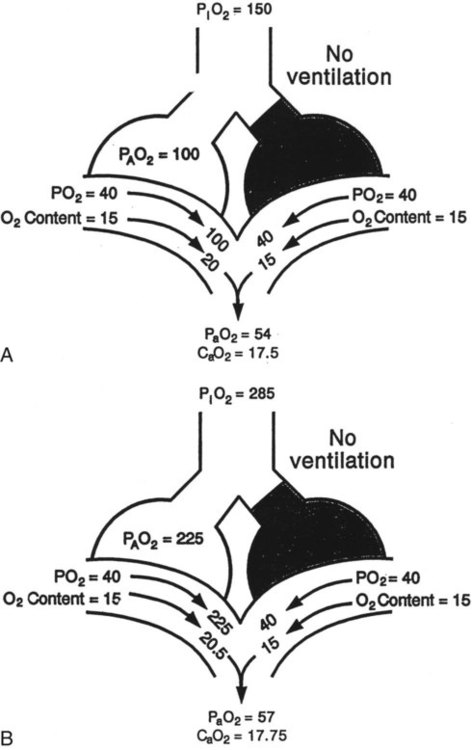

Clinical Presentation
The clinical presentation of shunt is very similar in many ways to the presentation of mismatch. Patient observations are similar, although chest excursion occasionally may be asymmetric in shunt. Bilateral or unilateral crackles are common owing to the alveolar filling process. Unilateral absence of breath sounds may indicate significant collapse, mass, or effusion; these conditions require treatment before oxygenation can improve. Shunt usually manifests with a “white” chest radiograph. The most advanced example of this is the diffuse, bilateral haziness in acute respiratory distress syndrome (ARDS). Shunting can be diagnosed by using 100% O2 breathing techniques, contrast-enhanced echocardiography, macroaggregated albumin scanning, or pulmonary angiography.6 Shunt is differentiated from
mismatch by the lack of increase in PO2 as fractional inspired oxygen (FiO2) is increased (see Figure 41-2, B).
Diffusion Impairment
Diffusion refers to movement of gas across the alveolar-capillary membrane secondary to a pressure gradient. Although diffusion is rarely a cause of significant hypoxemia at rest, its effects become more pronounced with exercise, which limits the time for gas exchange. This time limitation is normally not a problem, unless diffusion impairment is present, in which case hypoxemia results. Diffusion impairment in interstitial lung disease may contribute 20% to 30% of the widening in the alveolar-arterial O2 gradient during exercise.7 Diffusion impairment most commonly manifests in patients with interstitial lung disease (e.g., pulmonary fibrosis, asbestosis, sarcoidosis) in which the thickening and scarring of the interstitium undermine normal gas exchange. Emphysema, with its inherent alveolar destruction, also has subnormal transfer of O2 and CO2 between the alveolus and the capillary. The reduced ventilation in both diseases implies that mismatch also plays a role in the resulting hypoxemia.
Perfusion/Diffusion Impairment
Perfusion/diffusion impairment is a rare cause of hypoxemia found in individuals with liver disease complicated by hepatopulmonary syndrome.6 In this condition, right-to-left intracardiac shunt combines with dilated pulmonary capillaries resulting in impaired gas exchange. Specifically, the normal alveolar partial pressures of O2 may be insufficient to drive the O2 molecules to the center of the dilated pulmonary vasculature. Cirrhosis is the most common liver disease, and portal hypertension is usually present. Although shunt is a component of the syndrome, significant supplemental O2 can overcome the gas transfer reduction owing to the dilated vessels, so this is commonly called a perfusion/diffusion defect.
Decreased Inspired Oxygen
Also clinically uncommon, hypoxemia may develop when the inspired O2 is less than body requirements. The most common situation is at high altitude, where barometric pressure decreases, which results in a decrease in the partial pressure of inspired O2. Although airlines account for this decrease in barometric pressure by pressurizing their cabins, travelers with chronic hypoxemia may still need supplemental O2.8 Similarly, mountain climbers sometimes require O2 masks. Cases of patient-O2 disconnects and delivery of an incorrect gas source, which, it is hoped, occur rarely, are also included in this category.
Inspired O2 less than 21% can be used diagnostically and therapeutically. The Hypoxia Altitude Simulation Test seeks to replicate inspired partial pressure of O2 (PiO2) during air travel by asking the potential traveler to inhale a hypoxic mixture. Inhaling at FiO2 of 15% replicates the PiO2 found at an altitude of 8000 feet (108 mm Hg). For a lower altitude of 5400 feet, an equivalent FiO2 of 17% can be calculated.8 Infants with certain cyanotic congenital heart defects (e.g., hypoplastic left ventricle) may benefit from FiO2 below room air level. In the preoperative state, low FiO2 helps to prevent pulmonary dilation and the excessive pulmonary blood flow, which could flood the lungs.
Venous Admixture
A decrease in mixed venous O2 increases the gradient by which O2 needs to be stepped up as it passes through the lungs and can contribute to the development of hypoxemia. Congestive heart failure with low cardiac output is the most common cause of low mixed venous O2, owing to increased peripheral extraction of O2. Other causes include low hemoglobin concentration and increased O2 consumption. A low mixed venous O2 may have a significant effect on the ultimate arterial O2 tension in the presence of lung disease. There may be other, more important coexisting determinants of hypoxemia, such as mismatch and shunting.3
Differentiating the Causes of Acute Hypoxemic Respiratory Failure
It is important to recognize the physiologic basis of each of the three main causes of hypoxemic respiratory failure (hypoventilation, mismatch, and shunt). Hypoventilation differs from the other causes in manifesting with a normal alveolar-to-arterial PO2 difference [P(A − a)O2] indicating normal lung parenchyma (Table 41-1). A clinical determination of this difference is made by subtracting PaO2 from PAO2 (partial pressure of alveolar O2) derived from the alveolar air equation:
TABLE 41-1
Differentiating the Cause of Hypoxemia
Cause | P(a − a)O2 | Response to Increased FiO2 |
Hypoventilation | Normal | Marked |
Shunt | Increased | Minimal |
![]() |
Increased | Marked |
< ?xml:namespace prefix = "mml" />

A mismatch and shunt both result in elevated P(A − a)O2 levels, indicating that the resultant hypoxemia is due to an abnormality of lung tissue, requiring treatment to address that abnormality. When the RT encounters an increased P(A − a)O2, a
mismatch and shunt can be differentiated by means of O2 administration (see Figures 41-1 and 41-2). A significant response to applying even small amounts of O2 identifies
mismatch as the cause of hypoxemia because altered P(A − a)O2 has not been totally obliterated. True shunt shows little or no improvement in oxygenation even with 100% FiO2 (see Table 41-1). As a result, treatment of intrapulmonary shunt must be directed toward opening collapsed alveoli or clearing fluid or exudative material before O2 can be beneficial at below toxic levels. Testing to rule out anatomic shunt should be done in the right clinical setting (e.g., clear or black parenchyma on the chest radiograph).
Hypercapnic Respiratory Failure (Type II)


Similarly, this relationship shows that PaCO2 may increase as dead space (VD/VT) rises or as CO2 production () increases. Additionally, a change in the
distribution of the lung toward lower ratios not only causes hypoxemia, as shown in Figures 41-1 and 41-2, but also to a lesser extent can cause an elevation of PaCO2 by reducing the CO2 discharge from the pulmonary circulation to the alveoli. However, increased dead space, increased
, and shifts in the
distribution toward lower ratios all are usually matched by a corrective increase in ventilation because respiratory control mechanisms tend to maintain the PaCO2 constant. The following sections describe mechanisms of hypercarbia caused by an imbalance between CO2 exposure (external or internal) and CO2 clearance (central and respiratory effector mechanisms). Hypoxemia may often accompany pump failure simply because of the displacement of alveolar PO2 (PAO2) by the increased PaCO2. This situation is identified on a room air ABG assessment by a normal P(A − a)O2 as discussed previously. This situation identifies alveolar hypoventilation as a cause of hypoxemic respiratory failure, as mentioned earlier. The presence of an increased P(A − a)O2 indicates that concomitant hypoxemia is present, most likely as a result of
mismatch or shunt. The disorders responsible for hypercapnic respiratory failure (ventilatory failure) are discussed next.
Impairment in Respiratory Control
Inspiratory muscles are innervated by the phrenic and intercostal nerves via spinal cord transmission from the CNS. Both central (medullary) and peripheral (aortic and carotid bodies) chemoreceptors responding to CO2 tension and O2 tension stimulate the drive to breathe.9 This ventilatory drive can be diminished by various factors, such as drugs (overdose or sedation), bilateral carotid endarterectomy with incidental resection of the carotid bodies, brainstem lesions, diseases of the CNS such as multiple sclerosis or Parkinson disease, hypothyroidism, morbid obesity (e.g., obesity-hypoventilation), and sleep apnea. Other, less common potential causes include metabolic alkalosis, malnutrition, and sleep deprivation.10 Patients with metabolic encephalopathy or elevated intracranial pressure (ICP) may develop a reduced drive to breathe. Patients at risk of having a decreased ventilatory drive usually can be identified by their clinical situation (e.g., CNS insult, overdose of sedative medications). Significantly, many causes of central depression are easily reversible with treatment, so the clinician should be attentive to reversible causes.
Clinical Presentation
The hallmark of the clinical scenario of decreased ventilatory drive is bradypnea and perhaps ultimately apnea. A respiratory rate less than 12 breaths/min is abnormal in adults. Drug overdose or a brain disorder can manifest with an altered level of consciousness ranging from merely lethargic to obtunded and comatose, with decreased respirations. Evidence of drug use by history or toxicity screen confirms the diagnosis of drug overdose. Evidence of head trauma and brain computed tomography (CT) scan abnormalities are important in the diagnosis of a brain disorder. Although hypothyroidism classically manifests with fatigue, weight gain, hyporeflexia, and constipation, it can progress to significant hypoventilation and myxedema coma. Patients with obesity-hypoventilation may have a rapid, shallow breathing pattern, which results from decreased compliance and microatelectasis. Although these patients may also have nighttime sleep apnea, daytime PaCO2 is also elevated because of a decrease in the drive to breathe or an increase in the work of breathing.11
Impairment in Respiratory Effectors
Neurologic Diseases
The lung is basically a pump that inhales and exhales under the guidance of the CNS. In some patients, the CNS signal does not reach its goal, resulting in neuromuscular dysfunction. Examples include spinal trauma, motor neuron disease in which lesions of the anterior horn cells may gradually lead to progressive ventilatory failure (e.g., amyotrophic lateral sclerosis or poliomyelitis), motor nerve disorders (including Guillain-Barré syndrome and Charcot-Marie-Tooth disease), disorders of the neuromuscular junction (e.g., myasthenia gravis and botulism), and muscular diseases (including muscular dystrophy, myositis, critical care myopathy, and metabolic disorders).12 These diseases range from irreversible and usually terminal (e.g., amyotrophic lateral sclerosis) to reversible and usually self-limiting (e.g., myasthenia and Guillain-Barré syndrome).12
Clinical Presentation
Although hypercapnia may be a common end point, these diseases have varied clinical presentations. Patient observation is a key skill. Drooling, dysarthria, and weak cough are common signs in amyotrophic lateral sclerosis. As muscle wasting and weakness become more severe, diaphragmatic insufficiency develops, and supine paradoxical breathing is common.13 Guillain-Barré syndrome commonly manifests with lower extremity weakness progressing to the respiratory muscles in one-third of patients.14 Weak cough and gag may be seen, which can threaten airway patency and lead to microatelectasis, hypoxemia, and uncompensated respiratory acidosis. Although myasthenia gravis commonly manifests with ocular muscle weakness, it also can exhibit bulbar weakness on its path to respiratory muscle fatigue. Myasthenia gravis does not always result in respiratory failure.15 These diseases are quite different in clinical course, but there is much overlap in their presentations, and they commonly result in respiratory muscle fatigue and failure and elevated PaCO2.
Increased Work of Breathing
Despite normal respiratory drive, nerve transmission, and neuromuscular response, hypercapnic respiratory failure can still occur if the imposed workload cannot be overcome.3,16 Most commonly, this situation occurs when increased dead space accompanies COPD, or elevated airway resistance accompanies asthma. Both of these obstructive airway diseases may increase respiratory work requirements excessively secondary to the presence of intrinsic positive end-expiratory pressure (PEEP). Increased workload can also result from thoracic abnormalities such as pneumothorax, rib fractures with a flail chest, pleural effusions, and other conditions creating a restrictive burden on the lungs. Finally, requirements for increased minute ventilation can arise when increased CO2 production accompanies hypermetabolic states, such as in extensive burns.
Clinical Presentation
The RT must be alert to the possibility of respiratory failure when a heavy load is imposed on the respiratory system. Patients with asthma or COPD should present with hyperventilation in an exacerbation, but if breathing becomes more rapid but shallow, it may indicate impending failure. This increased VD/VT ratio leads to hypercapnia because the significant airway obstruction does not resolve with treatment. Diminished breath sounds in a young patient with asthma likewise can be an ominous sign. Irritability, confusion, and ultimately coma are possible signs in worsening hypercapnia, as they are in hypoxemic respiratory failure. More subtle findings include muscle tremor owing to catecholamine release and papilledema resulting from cerebral vasodilation in states of elevated arterial PCO2.17
In summary, hypercapnic (type II) respiratory failure, also known as ventilatory failure, develops when ventilation is impaired secondary to intrinsically or extrinsically increased CO2 exposure; impairment in respiratory control; or impairment in respiratory effector mechanisms, including neurologic disease or pulmonary and chest wall disorders associated with increased work of breathing (Table 41-2).
TABLE 41-2
Type I (Hypoxemic) | Type II (Hypercapnic) | |||
Increased Exposure | Impaired Respiratory Control | Neurologic Disease | Increased Work of Breathing | |
ARDS | Extrinsic | Drug overdose | Spinal cord trauma | Obstructive lung disease |
Pulmonary embolism | Defective CO2 scrubbers (anesthesia or life-support systems) | Bilateral endarterectomy with carotid body resection | Motor neuron | COPD |
Pulmonary edema | Occupational exposure (miners, spelunkers, dry-ice workers, firemen) | Central sleep apnea | Poliomyelitis | Asthma |
Septic shock | Intrinsic | Hypocapnia | Amyotrophic lateral sclerosis | Upper airway obstruction |
Pulmonary infection | Fever | Cheyne-Stokes | Motor nerve | Obesity-hypoventilation |
Viral | Shivering | Acromegaly | Phrenic nerve | Pneumothorax |
Bacterial | Hypermetabolism | Hypothyroid | Guillain-Barré | Severe burns |
Fungal | Agitation | Brainstem lesions | Charcot-Marie-Tooth | Chest wall disorders |
Inhalation | Excess caloric intake | Cerebrovascular accident | Neuromuscular junction | Kyphoscoliosis |
Smoke | Encephalitis | Myasthenia gravis | Ankylosing spondylitis | |
Chemical | Multiple sclerosis | Botulism | ||
Water | Parkinson disease | Muscular | ||
Pleural effusion | Metabolic alkalosis | Muscular dystrophy | ||
Interstitial lung disease | Primary alveolar hypoventilation | Myositis | ||
Obstructive lung disease | (Ondine’s curse) | Myopathy | ||
Aspiration | Congenital central hypoventilation | Acid maltase | ||
Primary pulmonary hypertension | Carotid body resection | Metabolic | ||
Obesity-hypoventilation |
Chronic Respiratory Failure (Type I and Type II)
Similarly, polycythemia may result from prolonged hypoxemic respiratory failure (e.g., sleep apnea) when O2 delivery to the tissues is compromised, and erythropoietin levels increase to elicit erythrocytosis. Hemoglobin also releases O2 more easily as the O2 dissociation curve shifts to the right in the face of acidosis. Finally, O2 delivery to the brain is enhanced when hypercapnia results in increased cerebral blood flow.17
Acute-on-Chronic Respiratory Failure
Chronic respiratory failure can be complicated by acute setbacks that create acute-on-chronic respiratory failure. Patients with chronic hypercapnic respiratory failure (chronic ventilatory failure) are at significant risk for this condition, as indicated by the fact that COPD is now the fourth leading cause of death in the United States.18 Acute-on-chronic respiratory failure can also be the presenting manifestation of neuromuscular disease in the setting of a concurrent pulmonary infection.19 Most common precipitating factors include bacterial or viral infections, congestive heart failure, pulmonary embolus, chest wall dysfunction, and medical noncompliance.19–21 In these patients, the presence of respiratory failure cannot be judged by the normal ABG criteria but by a significant change from the baseline PaCO2 to a level having the potential for morbidity and mortality.
Treatment goals include normalizing pH (avoiding mechanical ventilation if possible), elevating SaO2 to 90% (if hypoxemia is also present), improving airflow, treating infection, monitoring and maintaining fluid status, and preventing or treating complications as necessary.16,20,21 Optimally treated patients with acute-on-chronic respiratory failure have shown improving hospital mortality rates despite the overall stagnant rates for respiratory failure in general.22,23 Higher death rates are associated with factors such as significant baseline disease, a severe precipitating illness, severity of acidosis, and presence of complications.23 The use of advance directives regarding a patient’s desire for mechanical ventilation influences short-term mortality. Episodes of acute respiratory failure in these patients seem to have a significant long-term influence with mortality rates reaching 49% within 2 years of an acute exacerbation.22
Complications of Acute Respiratory Failure
Although respiratory failure is life-threatening by itself, complications frequently arise that can add significantly to morbidity and mortality. Especially in patients with ARDS, more deaths are due to complications (e.g., sepsis, multiorgan failure) than to the primary disease.24 Modern ICUs with sophisticated mechanical ventilation can prolong but may not preserve life. Pulmonary complications such as emboli, barotrauma, and infection may be secondary to treatment strategies such as catheters, mechanical ventilation, and endotracheal tubes. A wide array of nonpulmonary complications exists, ranging from cardiac disorders (e.g., arrhythmias, hypotension) to gastrointestinal ailments (e.g., hemorrhage, dysmotility) to renal disturbances (e.g., acute renal failure, positive fluid balance). Bacteremia, malnutrition, and psychosis secondary to prolonged ICU stays can also seriously complicate an episode of acute respiratory failure.24
Clinical Presentation
Clinically, a patient with respiratory muscle fatigue shows an initially increased respiratory rate followed by bradypnea (slowed respiratory rate) and apnea as fatigue ensues. Respiratory alternans, which is a phasic alteration between rib cage and abdominal breathing, may also occur. Opinions vary on the sensitivity and specificity of abdominal motion paradox in patients with respiratory muscle weakness, but at least some investigators suggest that respiratory muscle paradox is an early sign (see Chapter 15). When ventilatory failure is full-blown, ABG results show hypercapnia with acidosis. As mentioned earlier, the presence of hypercapnia with acidosis can also indicate that the respiratory center is not responding properly.25
Tachypnea is the cardinal sign of increased work of breathing. Tachypnea occurs when the respiratory center increases breathing frequency in an attempt to lessen respiratory excursion and reduce the amount of work performed by the respiratory muscles.26 Overall workload is reflected in the minute volume needed to maintain normocapnia.
Indications for Ventilatory Support
For each type of oxygenation and ventilatory failure, the goal of mechanical ventilation is either to support the patient until the underlying problem resolves or to maintain support of the patient with chronic ventilatory problems. These goals may be achieved by improving alveolar ventilation and arterial oxygenation, increasing lung volume, or reducing work of breathing.27 This section discusses the indications for mechanical ventilation for hypoxemic (type I) and hypercapnic (type II) respiratory failure. Hypoxemic respiratory failure is divided into processes that require short-term and long-term ventilatory support. Hypercapnic respiratory failure is broken down into unstable ventilatory drive, muscle fatigue, excessive work of breathing, and alveolar hypoventilation. The relationship between work of breathing and the need for mechanical ventilation is discussed. Numerical indicators of the need for ventilatory support are reviewed. Management that is specific for each type of respiratory failure is described, including the indications for less commonly used modes of ventilation. Finally, the specific management of patients with obstructive lung disease and patients with head injury is discussed.
Parameters Indicating Need for Ventilatory Support
Although various measurements have been proposed to help decide if a patient needs mechanical ventilation, the clinical status of the patient is the most important criterion. Table 41-3 and the discussion that follows review common physiologic indicators for initiating support by the underlying cause of respiratory failure.
TABLE 41-3
Mechanism | Normal Values | Support Indicated |
Inadequate Alveolar Ventilation | ||
PaCO2 (mm Hg) | 35-45 | >55 |
pH | 7.35-7.45 | <7.20 |
Inadequate Lung Expansion | ||
Tidal volume (VT) ml/kg | 5-8 | <5 |
Vital capacity (VC) ml/kg | 65-75 | <10 |
Respiratory rate | 12-20 | >35 |
Inadequate Muscle Strength | ||
Maximum inspiratory pressure (cm H2O) | −80-100 | ≥−20 |
Vital capacity (VC, ml/kg) | 65-75 | <10 |
Maximum voluntary ventilation (MVV, L/min) | 120-180 | <2× VE |
Increased Work of Breathing | ||
Minute ventilation (![]() |
5-6 | >10 |
VD/VT (%) | 0.25-0.40 | >0.6 |
Hypoxemia | ||
P(A − a)O2 on 100% O2 (mm Hg) | 25-65 | >350 |
PaO2/FiO2 | 350-450 | <200 |
Hypoxemic Respiratory Failure
Severe, refractory hypoxemia is a common indication for intubation and ventilator support. Table 41-3 lists different measures of hypoxemia that have been used to assess the need for ventilatory support. Most commonly, PaO2 is compared with FiO2 as with the PaO2/FiO2 ratio or the alveolar-arterial O2 difference [P(A − a)O2]. Indicators of profoundly impaired oxygenation suggesting the need for intubation, high inspired O2 administration, and PEEP include P(A − a)O2 value of 350 mm Hg on FiO2 of 1.0 or a PaO2/FiO2 value of less than 200. These values are useful for all causes of hypoxemic respiratory failure (type I) but cannot help distinguish if the process is a readily reversible one, such as pulmonary edema or atelectasis, or a process, such as acute lung injury, that resolves more slowly. Frequently, patients have a combination of hypoxemic and hypercapnic respiratory failure.
Hypercapnic Respiratory Failure (Ventilatory Failure)
As previously discussed, hypercapnic (type II) respiratory failure or ventilatory failure can be caused by increased ventilatory dead space, increased CO2 production, or decreased alveolar ventilation. All of these processes cause an increase in PaCO2.25 Assessment of the pH allows a determination of whether the problem is acute or chronic. Chronic hypoventilation is compensated by the kidneys’ retention of bicarbonate, although this response requires several days. The following example shows the importance of pH in interpreting the significance of elevated PaCO2.
Patient A | Patient B | |
PaCO2 | 60 mm Hg | 60 mm Hg |
Serum HCO3− | 25 mEq/L | 36 mEq/L |
pH | 7.25 | 7.38 |
Significance of Elevated Alveolar Partial Pressure of Carbon Dioxide
Because elevated PaCO2 increases ventilatory drive in healthy subjects, the existence of hypoventilation suggests other problems with the respiratory apparatus. Specifically, the presence of acute respiratory acidosis indicates one of three major problems: (1) The respiratory center is not responding normally to elevated PaCO2; (2) the respiratory center is responding normally, but the signal is not getting through to the respiratory muscles; or (3) despite normal neurologic response mechanisms, the lungs and chest bellows are incapable of providing adequate ventilation because of parenchymal lung disease or muscular weakness.28
Assessment of Respiratory Fatigue, Weakness, and Failure and Work of Breathing
Respiratory Muscle Weakness
Respiratory muscle weakness refers to the decreased capacity of a rested muscle to generate force and decreased endurance.29 Respiratory muscle weakness occurs most commonly in patients with neuromuscular disease. Other conditions that lead to muscle weakness by increasing demand include COPD, kyphoscoliosis, and obesity.
The most commonly used tests to assess respiratory muscle strength at the bedside are maximum inspiratory pressure (MIP) and maximum expiratory pressure (MEP),30 forced vital capacity, and maximum voluntary ventilation (MVV) (see Table 41-3). MIP of −30 cm H2O or less (more negative) usually indicates adequate respiratory muscle strength to continue spontaneous breathing, but the overall trend needs to be considered. This consideration is especially important in patients with myasthenic crisis or Guillain-Barré syndrome, where values of MIP that are becoming less negative may be the only clue to impending respiratory failure. The MVV maneuver can be performed at the bedside with a hand-held spirometer, but its use in the critical care setting is limited because substantial patient cooperation is required. The sniff nasal inspiratory pressure may also be used to assess inspiratory muscle strength. Advantages include ease of performance even in patients with advanced disease and its prognostic value.31
Respiratory Muscle Fatigue
Fatigue is usually defined as a condition in which there is loss of the capacity to develop force or velocity of a muscle resulting from muscle activity under load, which is reversible by rest.32 Fatigue can be assessed by measuring the loss of force in response to repeated stimulations. It can be caused by both specific demands placed on the muscle and reduced supply of necessary nutrients. The demand on a muscle is increased by increased work of breathing, increased strength of muscle contraction, and decreased muscle efficiency. Hypoxemia, decreased inspiratory muscle blood flow, poor nutrition, and inability of a muscle to extract energy from supplied substrates can lead to fatigue as well.29
There are three types of respiratory muscle fatigue. (1) Central muscle fatigue is an exertion-induced, reversible decrease in central respiratory drive; (2) transmission muscle fatigue is an exertion-induced, reversible impairment in the transmission of neural impulses; and (3) contractile muscle fatigue is a reversible impairment in the contractile response to a neural impulse in an overloaded muscle.28
Respiratory Failure
Respiratory failure is an unfavorable imbalance between a respiratory workload, on the one hand, and ventilatory muscle strength and endurance, on the other hand. The tension-time index takes into account the fact that respiratory muscle endurance depends both on the size of the respiratory load in relation to respiratory strength (Pdi/Pdimax) and on the duration of the inspiratory effort in relation to total breath time (the duty cycle, or Ti/Ttot). This index (Pdi/Pdimax) × (Ti/Ttot) determines whether a respiratory load can be tolerated without development of failure: Values less than 0.15 are generally tolerated for a long period, whereas indices greater than 0.18 usually result in fatigue and respiratory failure within 45 minutes.33 Comparing the spontaneous minute ventilation with MVV is also a helpful index because fatigue and failure are both likely to occur if the minute ventilation exceeds 60% of MVV.34
These closely related concepts of weakness, fatigue, and failure usually overlap and can result in acute or chronic respiratory failure. Respiratory muscle weakness can predispose to ventilatory muscle fatigue. Whether fatigue consistently leads to failure has historically been the subject of much debate.35 In one study, weaning failure was not accompanied by fatigue of the diaphragm despite the presence of diaphragm weakness.36 Alternatively, fatigue of the diaphragm lasting for 24 hours is reliably present after hyperventilation at 60% of MVV or greater until task failure.34,37
Work of Breathing
Work of breathing is the amount of pressure needed to move a given volume into the lung with a relaxed chest wall. Excessive work of breathing is the most common cause of respiratory muscle fatigue. Work of breathing is due to physiologic work and imposed work. Physiologic work involves overcoming the elastic forces during inspiration and overcoming the resistance of the airways and lung tissue. Normal work of breathing is 0.3 to 0.6 J/L. Airway and pulmonary parenchymal abnormalities can increase the physiologic work of breathing. In intubated patients, sources of imposed work of breathing include the endotracheal tube, ventilator circuit, and auto-PEEP secondary to dynamic hyperinflation with airflow obstruction, as is commonly seen in a patient with COPD.26 Increased work of breathing can also be an impediment to weaning.38 Measurement of work of breathing with an esophageal balloon catheter and a flow transducer has been used to determine work of breathing and break it down into physiologic and imposed components.38 Kirton and colleagues39 showed that 96% of patients with a physiologic work of breathing less than 0.8 J/L were successfully weaned and extubated from ventilatory support.
Choosing a Ventilatory Support Strategy for Different Causes of Respiratory Failure
The remainder of this chapter briefly discusses current ventilatory strategies for hypoxemic and hypercapnic respiratory failure. The clinical application of specific modes of mechanical ventilation is described in Chapters 42 and 44, and noninvasive ventilation (NIV) is reviewed in more detail in Chapter 45. When it has been determined that the patient needs ventilatory support, the initial decision is whether to intubate or to ventilate noninvasively. In the acute setting, this decision is sometimes based on the underlying process, the type of respiratory failure, and how rapidly the underlying process can be reversed.
Noninvasive Ventilation
A consensus report supports the use of noninvasive support of ventilation to reduce the morbidity and possibly the mortality of both hypoxemic and hypercarbic respiratory failure.40 In this context, noninvasive ventilation (NIV) can be defined as any mode of ventilatory support that is provided without endotracheal intubation, encompassing continuous positive airway pressure (CPAP) alone or in combination with any mode of pressure-limited or volume-limited ventilation.40 NIV can improve hypoxemia and hypercarbia via several mechanisms including but not limited to (1) compensating for the inspiratory threshold load imposed by intrinsic PEEP,41 (2) supplementing a reduced tidal volume,42 (3) partial or complete unloading of the respiratory muscles,42 (4) reducing venous return and left ventricular afterload,43,44 (5) alveolar recruitment,45 (6) preventing intermittent narrowing and collapse in patients with concomitant obstructive sleep apnea hypopnea syndrome by acting as a pneumatic splint during sleep,46 and (7) improving lung function (particularly functional residual capacity) and daytime gas exchange in obstructive sleep apnea hypopnea syndrome.47 NIV currently has indications in both acute48 and chronic49 respiratory failure.
Noninvasive Ventilation in Acute Conditions
Exacerbations of Chronic Obstructive Pulmonary Disease
NIV is considered to be a standard of care practice in patients with exacerbations of COPD.50 A meta-analysis of eight randomized controlled trials showed that NIV combined with usual care in exacerbations of COPD resulted in a lower relative risk (RR) of treatment failure (defined as mortality, need for intubation, or intolerance) of 0.51 in favor of NIV (number needed to treat [NNT] to prevent 1 treatment failure was 5), reduced risk of intubation (RR 0.43, NNT = 5), reduction in mortality (RR 0.41, NNT = 8), reduced risk of complications (RR 032, NNT = 3), and reduced hospital length of stay by about 3 days.50
Current recommendations are to initiate NIV before the development of severe acidosis in the course of a COPD exacerbation with PaCO2 greater than 45 mm Hg.50 Otherwise, in patients with mild COPD exacerbations (pH > 7.35), NIV was no more effective than standard medical therapy in preventing the occurrence of acute respiratory failure, improving mortality, or reducing length of hospitalization.51 Nearly 50% of the patients did not tolerate NIV.51
Cardiogenic Pulmonary Edema
NIV is a recommended option in the management of acute respiratory failure in the setting of cardiogenic pulmonary edema. In the largest randomized trial comparing CPAP or NIV with standard O2 therapy in acute pulmonary edema (N = 1156 patients), the combined noninvasive treatment arms (CPAP and NIV) significantly reduced dyspnea score, heart rate, acidosis, and hypercapnia within the first hour after the start of treatment.52 However, there was no significant treatment effect compared with standard therapy in the 7-day or 30-day mortality in the rates of intubation, rate of admission to the critical care unit, or mean length of hospital stay.52 The results of this large study are in contrast to smaller randomized trials and meta-analyses that showed decreased intubation and mortality rates with NIV.53 Factors that may account for those differences include the much smaller intubation rates in the study by Gray and colleagues (2.9% overall vs. 20% with conventional therapy in other trials),52 the higher mortality in the Gray study, and methodologic differences (patients failing standard therapy in the Gray study received rescue NIV). There was no difference in outcomes between CPAP and NIV devices in this setting.52,53
Acute Asthma
In a prospective study of episodes of asthma associated with acute respiratory failure, NIV progressively improved pH and PaCO2 over 12 to 24 hours and reduced the respiratory rate.54 In a subsequent controlled trial, NIV improved lung function, resolved the attack faster, and reduced the need for hospitalization.55 Despite those promising results, the use of NIV in status asthmaticus remains controversial, and large, prospective, randomized controlled trials are needed to confirm the role of NIV in that setting.56
Acute Lung Injury and Acute Respiratory Distress Syndrome
NIV in the settings of acute lung injury and ARDS has been disappointing; a meta-analysis of the topic concluded that such an approach was associated with a 50% failure rate.57 An earlier study that used CPAP in patients with acute respiratory failure predominantly caused by acute lung injury showed early physiologic improvements but no reduction in the need for intubation, no improvement in outcomes, and an increase in adverse events including cardiac arrest in patients randomly assigned to CPAP.58 A prospective study showed that NIV was successful in improving gas exchange and avoiding intubation in 54% of patients, with consequent reduction in ventilator-associated pneumonia and lower ICU mortality rate.59 Factors that may be associated with NIV failure in these settings include the presence of shock, metabolic acidosis, severe hypoxemia, a Simplified Acute Physiology Score (SAPS) II greater than 34, and a PaO2/FiO2 less than 175 after 1 hour of NIV.59,60
Noninvasive Ventilation in Chronic Conditions
Obesity-Hypoventilation Syndrome
Obesity-hypoventilation syndrome refers to the presence of daytime hypercapnia (PaCO2 > 45 mm Hg) in obese individuals when no other cause of hypoventilation is present. In a meta-analysis, factors associated with daytime hypercapnia included body mass index, the presence of nocturnal apnea hypopnea, mean overnight O2 saturation, and severity of restrictive pulmonary function.61 In a study of patients with obesity-hypoventilation syndrome who failed initial CPAP treatment, average volume-assured pressure support, a form of NIV in which pressure support is automatically adjusted to reach a set tidal volume, was found to lower PaCO2 compared with bilevel positive airway pressure alone but without improving oxygenation, sleep quality, or quality of life.62
Stable Chronic Obstructive Pulmonary Disease
A randomized controlled trial of NIV in patients with severe COPD and PaCO2 greater than 46 mm Hg showed a survival benefit in favor of NIV and O2 compared with O2 alone (hazard ratio 0.6).63 However, there was a low level of adherence to NIV, an apparent worsening in certain quality-of-life indices, and no reduction in hospitalization rates,63 perhaps owing to selection of inspiratory pressures that were insufficient to reduce hypercapnia. In a randomized trial, compared with low-intensity NIV (mean inspiratory positive airway pressure 14 cm H2O, backup rate 8/min), the use of settings that aimed to reduce PaCO2 maximally (mean inspiratory positive airway pressure 29 cm H2O with backup rate 17.5/min) increased the daily use of NIV by 3.6 hr/day and improved exercise-related dyspnea, daytime PaCO2, forced expiratory volume in 1 second, vital capacity and health-related quality of life.64
Neuromuscular Diseases and Thoracic Cage Abnormalities
This condition most commonly applies to chronic neuromuscular disease, with several studies showing that even in progressive neuromuscular disorders, NIV can prolong survival, improve quality of life, enhance cognitive function, and reduce pneumonia and hospitalization rates.12 Other NIV techniques using rocking beds, pneumobelts, and negative pressure ventilation are much less frequently used and are becoming less easily available.
Invasive Ventilatory Support
Acute Respiratory Distress Syndrome
Profound hypoxemic respiratory failure is often due to severe pneumonia and ARDS. Patients with these conditions have very noncompliant lungs. Volume-cycled ventilation in patients with ARDS frequently leads to high peak airway and plateau pressures. It has been established that ventilating these patients with small tidal volumes (about 6 ml/kg) reduces complications associated with mechanical ventilation and improves survival.65
Increased Intracranial Pressure
Hyperventilation applied acutely and for short periods may be used to reduce ICP. The goal is to lower PaCO2 to between 25 mm Hg and 30 mm Hg, which causes alkalosis, which in combination with hypocapnia helps reduce cerebral blood flow until ICP can be controlled by other measures. Ongoing ventilatory support should maintain PCO2 in the range of 30 to 40 mm Hg. By maintaining PCO2 in this range, sudden increases in ICP can be quickly controlled by short-term hyperventilation. Although reducing blood flow can reduce brain swelling and ICP, cerebral ischemia can also result. Another concern in ventilating patients with elevated ICP is using PEEP to manage hypoxemia. There is a concern that increased intrathoracic pressure secondary to PEEP would cause decreased cerebral venous return leading to increased ICP and that PEEP can decrease cerebral perfusion by limiting cardiac output. The use of PEEP in patients with elevated ICP may require invasive monitoring of ICP because the combination of decreased cerebral perfusion and elevated ICP can narrow cerebral perfusion pressure.66 Elevation of the head of the bed can offset the increased ICP associated with the application of PEEP.
Obstructive Lung Disease
Patients with obstructive lung disease have markedly increased airway resistance that leads to a decrease in the rate of expiratory flow with resulting hyperinflation. These patients frequently have problems with elevated airway pressure or dynamic hyperinflation (auto-PEEP), which can cause barotrauma and increased dyssynchrony between the patient and ventilator.67
The management goal for patients with COPD with respiratory failure is to oxygenate and ventilate the patient successfully, while avoiding dyssynchrony and dynamic hyperinflation. In these patients, lower tidal volumes (6 to 8 ml/kg), moderate respiratory rates, and high sustained (square wave) inspiratory flow rates (70 to 100 L/min) are recommended to avoid dynamic hyperinflation.68 These maneuvers reduce inspiratory time and prolong expiratory time, which allows a patient with obstructive lung disease to have a longer time to exhale.
Another consideration in patients with obstructive lung disease is the inspiratory threshold load imposed by auto-PEEP resulting in increased patient inspiratory work.41 In this case, applied (or extrinsic) PEEP can compensate for this threshold load and reduce the work of breathing for patient-triggered breaths in any assisted ventilatory mode.41