Placenta and Extraembryonic Membranes
The tissues that compose the fetal-maternal interface (placenta and chorion) are derivatives of the trophoblast, which separates from the inner cell mass and surrounds the cellular precursors of the embryo proper even as the cleaving zygote travels down the uterine tube on its way to implanting into the uterine wall (see Fig. 3.18). Other extraembryonic tissues are derived from the inner cell mass. These include the following: the amnion (an ectodermal derivative), which forms a protective fluid-filled capsule around the embryo; the yolk sac (an endodermal derivative), which in mammalian embryos no longer serves a primary nutritive function; the allantois (an endodermal derivative), which is associated with the removal of embryonic wastes; and much of the extraembryonic mesoderm, which forms the bulk of the umbilical cord, the connective tissue backing of the extraembryonic membranes, and the blood vessels that supply them.
Extraembryonic Tissues
Amnion
The origin of the amniotic cavity within the ectoderm of the inner cell mass in the implanting embryo is described in Chapter 5 (see Figs. 3.18 and 5.2). As the early embryo undergoes cephalocaudal and lateral folding, the amniotic membrane surrounds the body of the embryo like a fluid-filled balloon (Fig. 7.1), thus allowing the embryo to be suspended in a liquid environment for the duration of pregnancy. The amniotic fluid serves as a buffer against mechanical injury to the fetus; in addition, it accommodates growth, allows normal fetal movements, and protects the fetus from adhesions.
The thin amniotic membrane consists of a single layer of extraembryonic ectodermal cells lined by a nonvascularized layer of extraembryonic mesoderm. Keeping pace with fetal growth, the amniotic cavity steadily expands until its fluid content reaches a maximum of nearly 1 L by weeks 33 to 34 of pregnancy (Fig. 7.2).
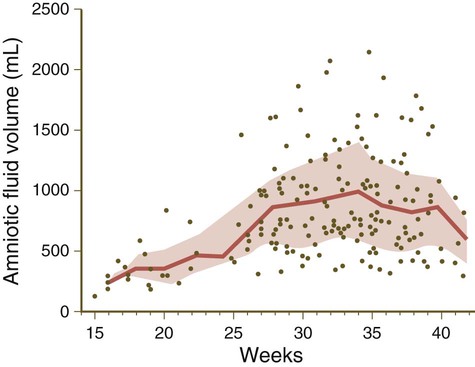
The lined and shaded area represents the mean ± standard deviation. Dots represent outlying values. (Data from Queenan JT and others: Am J Obstet Gynecol 114:34-38, 1972.)
In the third trimester of pregnancy, the amniotic fluid turns over completely every 3 hours, and at term, the fluid-exchange rate may approach 500 mL/hour. Although much of the amniotic fluid is exchanged across the amniotic membrane, fetal swallowing is an important mechanism in late pregnancy, with about 20 mL/hour of fluid swallowed by the fetus. Swallowed amniotic fluid ultimately enters the fetal bloodstream after absorption through the gut wall. The ingested water can leave the fetal circulation through the placenta. During the fetal period, excreted urine from the fetus contributes to amniotic fluid. Clinical Correlation 7.1 discusses conditions related to the amount of amniotic fluid or substance concentrations in the fluid.
Yolk Sac
The yolk sac, which is lined by extraembryonic endoderm, is formed ventral to the bilayered embryo when the amnion appears dorsal to the embryonic disk (see Fig. 5.2). In contrast to birds and reptiles, the yolk sac of mammals is small and devoid of yolk. Although vestigial in terms of its original function as a major source of nutrition, the yolk sac remains vital to the embryo because of other functions that have become associated with it. Some evidence indicates that before the placental circulation is established, nutrients, such as folic acid and vitamins A, B12, and E, are concentrated in the yolk sac and are absorbed by endocytosis. Because this form of histiotrophic nutrition occurs during the time of neurulation, it may play a role in the prevention of neural tube defects (see p. 138).
When it first appears, the yolk sac is in the form of a hemisphere bounded at the equatorial region by the dorsal wall of the primitive gut (see Fig. 7.1). As the embryo grows and undergoes lateral folding and curvature along the craniocaudal axis, the connection between the yolk sac and the forming gut becomes attenuated in the shape of a progressively narrowing stalk attached to a more spherical yolk sac proper at its distal end. In succeeding weeks, the yolk stalk becomes very long and attenuated as it is incorporated into the body of the umbilical cord. The yolk sac itself moves nearer the chorionic plate of the placenta (Fig. 7.3).
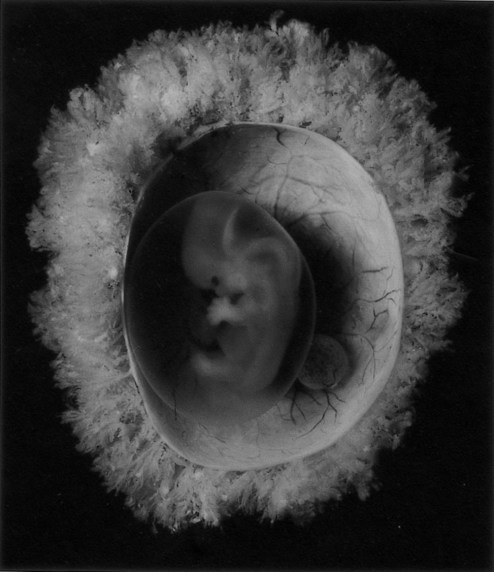
The embryo was exposed by cutting open the chorion. The small sphere to the right of the embryo is the yolk sac. (Carnegie embryo No. 8537A, Courtesy of Chester Reather, Baltimore.)
The endoderm of the yolk sac is lined on the outside by well-vascularized extraembryonic mesoderm. Cells found in each of these layers contribute vital components to the body of the embryo. During the third week, primordial germ cells, which arise in the extraembryonic mesoderm near the base of the allantois, become recognizable in the lining of the yolk sac (see Fig. 1.1). Soon these cells migrate into the wall of the gut and the dorsal mesentery as they make their way to the gonads, where they differentiate into oogonia or spermatogonia.
In the meantime, groups of extraembryonic mesodermal cells in the wall of the yolk sac become organized into blood islands (see Fig. 6.19), and many of the cells differentiate into primitive blood cells. Extraembryonic hematopoiesis continues in the yolk sac until about the sixth week, when blood-forming activity transfers to intraembryonic sites, especially the liver.
As the tubular gut forms, the attachment site of the yolk stalk becomes progressively less prominent, until by 6 weeks it has effectively lost contact with the gut. In a small percentage of adults, traces of the yolk duct persist as a fibrous cord or an outpouching of the small intestine known as Meckel’s diverticulum (see Fig. 15.15A). The yolk sac itself may persist throughout much of pregnancy, but it is not known to have a specific function in the fetal period. The proximal portions of the blood vessels of the yolk sac (the vitelline circulatory arc) persist as vessels that supply the midgut region.
Allantois
The allantois arises as an endodermally lined ventral outpocketing of the hindgut (see Fig. 7.1). In the human embryo, it is just a vestige of the large, saclike structure that is used by the embryos of many mammals, birds, and reptiles as a major respiratory organ and repository for urinary wastes. Similar to the yolk sac, the allantois in a human retains only a secondary function, in this case respiration. In humans, this function is served by the blood vessels that differentiate from the mesodermal wall of the allantois. These vessels form the umbilical circulatory arc, consisting of the arteries and veins that supply the placenta (see Fig. 6.26). (The postnatal fate of these vessels is discussed in Chapter 18.)
The allantois proper, which consists of little more than a cord of endodermal cells, is embedded in the umbilical cord. Later in development, the proximal part of the allantois (called the urachus) is continuous with the forming urinary bladder (see Fig. 16.2). After birth, it becomes transformed into a dense fibrous cord (median umbilical ligament), which runs from the urinary bladder to the umbilical region (see Fig. 18.19).
Chorion and Placenta
Formation of the placental complex represents a cooperative effort between the extraembryonic tissues of the embryo and the endometrial tissues of the mother. (Early stages of implantation of the embryo and the decidual reaction of the uterine lining are described in Chapter 3.) After implantation is complete, the original trophoblast surrounding the embryo has undergone differentiation into two layers: the inner cytotrophoblast and the outer syncytiotrophoblast (see Fig. 3.18D). Lacunae in the rapidly expanding trophoblast have filled with maternal blood, and the connective tissue cells of the endometrium have undergone the decidual reaction (containing increased amounts of glycogen and lipids) in response to the trophoblastic invasion.
Formation of Chorionic Villi
In the early implanting embryo, the trophoblastic tissues have no consistent gross morphological features; consequently, this is called the period of the previllous embryo. Late in the second week, defined cytotrophoblastic projections called primary villi begin to take shape (see Fig. 5.2). Shortly thereafter, a mesenchymal core appears within an expanding villus, at which point it is properly called a secondary villus (Fig. 7.4). Surrounding the mesenchymal core of the secondary villus is a complete layer of cytotrophoblastic cells, and outside of that is the syncytiotrophoblast. By definition, the secondary villus becomes a tertiary villus when blood vessels penetrate its mesenchymal core and newly formed branches. This event occurs toward the end of the third week of pregnancy.
The terminal portion of a villus remains trophoblastic, consisting of a solid mass of cytotrophoblast called a cytotrophoblastic cell column (see Fig. 7.4) and a relatively thin covering of syncytiotrophoblast over that. The villus is bathed in maternal blood. A further development of the tip of the villus occurs when, under the influence of the local hypoxic environment, the cytotrophoblastic cell column expands distally and penetrates the syncytiotrophoblastic layer (Fig. 7.5). These cytotrophoblastic cells abut directly on maternal decidual cells and spread over them to form a complete cellular layer known as the cytotrophoblastic shell, which surrounds the embryo complex. The villi that give off the cytotrophoblastic extensions are known as anchoring villi (see Fig. 7.4) because they represent the real attachment points between the embryo complex and the maternal tissues.
It is important to understand the overall relationships of the various embryonic and maternal tissues at this stage of development (see Fig. 7.5). The embryo, attached by the body stalk, or umbilical cord, is effectively suspended in the chorionic cavity. The chorionic cavity is bounded by the chorionic plate, which consists of extraembryonic mesoderm overlaid with trophoblast. The chorionic villi extend outward from the chorionic plate, and their trophoblastic covering is continuous with that of the chorionic plate. The villi and the outer surface of the chorionic plate are bathed in a sea of continually exchanging maternal blood. Because of this, the human placenta is designated the hemochorial type.*
Although chorionic villi are structurally very complicated, it is convenient to liken the basic structure of a villus complex to the root system of a plant. The anchoring villus is equivalent to the central tap root; by means of the cytotrophoblastic cell columns, it attaches the villus complex to the outer cytotrophoblastic shell. The unattached branches of the floating villi (see Fig. 7.12) dangle freely in the maternal blood that fills the space between the chorionic plate and the outer cytotrophoblastic shell. All surfaces of the villi, chorionic plate, and cytotrophoblastic shell that are in contact with maternal blood are lined with a continuous layer of syncytiotrophoblast.
Establishing the Uteroplacental Circulation
One of the critical features of the developing embryonic-maternal interface is the establishment of a uteroplacental circulation that serves as the medium for bringing food and oxygen to and removing wastes from the embryo. This is accomplished by erosion of the walls of the spiral arteries of the uterus and their modification so that, as the embryo grows, these arteries can provide an increasing flow of blood at low pressure to bathe the syncytiotrophoblastic surface of the placenta (see Fig. 7.10). Specialized invasive cytotrophoblastic cells, migrating out from the anchoring villi, invade the spiral arteries (but not the veins) and cause major modifications of their walls by secreting a specialized extracellular matrix and displacing many of the normal cellular elements of the spiral arteries. As a result, the arteries become wider, but the blood escaping from their open ends leaves at a much lower pressure than normal arterial pressure.
Hypoxia stimulates cytotrophoblastic cells to undergo mitosis. This may be one of the environmental conditions that underlies the rapid growth of the cytotrophoblast during the early embryonic period. After 12 weeks, when the maternal blood in the placental space contains large numbers of erythrocytes and is more highly oxygenated, the fetal erythrocytes, through an isoform switch, begin to produce fetal hemoglobin, which requires higher oxygen tension to bind oxygen efficiently. The maternal blood that leaves the spiral arteries freely percolates throughout the intervillous spaces and bathes the surfaces of the villi. The maternal blood is then picked up by the open ends of the uterine veins, which also penetrate the cytotrophoblastic shell (see Fig. 7.10).
Gross Relationships of Chorionic and Decidual Tissues
Within days after implantation of the embryo, the stromal cells of the endometrium undergo a striking transformation called the decidual* reaction. After the stromal cells swell as the result of the accumulation of glycogen and lipid in their cytoplasm, they are known as decidual cells (Fig. 7.6). The decidual reaction spreads throughout stromal cells in the superficial layers of the endometrium. The maternal decidua are given topographic names based on where they are located in relation to the embryo.
The decidual tissue that overlies the embryo and its chorionic vesicle is the decidua capsularis, whereas the decidua that lies between the chorionic vesicle and the uterine wall is the decidua basalis (Fig. 7.7). With continued growth of the embryo, the decidua basalis becomes incorporated into the maternal component of the definitive placenta. The remaining decidua, which consists of the decidualized endometrial tissue on the sides of the uterus not occupied by the embryo, is the decidua parietalis.
In human embryology, the chorion is defined as the layer consisting of the trophoblast and the underlying extraembryonic mesoderm (see Fig. 7.1). The chorion forms a complete covering (chorionic vesicle) that surrounds the embryo, amnion, yolk sac, and body stalk. During the early period after implantation, primary and secondary villi project almost uniformly from the entire outer surface of the chorionic vesicle. The formation of tertiary villi is asymmetric, however, and the invasion of the cytotrophoblastic core of the primary villi by mesenchyme and embryonic blood vessels occurs preferentially in the primary villi located nearest the decidua basalis. As these villi continue to grow and branch, the villi located on the opposite side (the abembryonic pole) of the chorionic vesicle fail to keep up and eventually atrophy as the growing embryo complex bulges into the uterine cavity (see Fig. 7.7). The region that contains the flourishing chorionic villi and that ultimately becomes the placenta is the chorion frondosum. The remainder of the chorion, which ultimately becomes smooth, is the chorion laeve (Fig. 7.8).
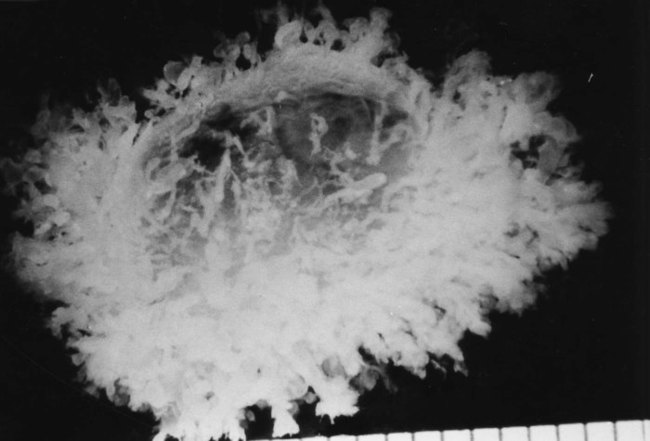
The small, bare area in this photograph of a human chorionic vesicle is a region where the chorionic villi have atrophied. This area will enlarge in succeeding weeks. (From Gilbert-Barness E, ed: Potter’s pathology of the fetus and infant, St Louis, 1997, Mosby.)
The overall growth of the chorionic vesicle (Fig. 7.9), with its bulging into the uterine lumen, pushes the decidua capsularis progressively farther from the endometrial blood vessels. By the end of the first trimester, the decidua capsularis itself undergoes pronounced atrophy. Within the next month, portions of the atrophic decidua capsularis begin to disappear and leave the chorion laeve in direct contact with the decidua parietalis on the opposite side of the uterus (see Fig. 7.7). By midpregnancy, the decidua capsularis has fused with the tissues of the decidua parietalis, thereby effectively obliterating the original uterine cavity. While the chorion laeve and decidua capsularis are undergoing progressive atrophy, the placenta takes shape in its definitive form and acts as the main site of exchange between the mother and embryo.
Formation and Structure of the Mature Placenta
As the distinction between the chorion frondosum and chorion laeve becomes more prominent, the limits of the placenta proper can be defined. The placenta consists of a fetal and a maternal component (Fig. 7.10). The fetal component is the part of the chorionic vesicle represented by the chorion frondosum. It consists of the wall of the chorion, called the chorionic plate, and the chorionic villi that arise from that region. The maternal component is represented by the decidua basalis, but covering the decidua basalis is the fetally derived outer cytotrophoblastic shell. The intervillous space between the fetal and maternal components of the placenta is occupied by freely circulating maternal blood. In keeping with its principal function as an organ-mediating exchange between the fetal and maternal circulatory systems, the overall structure of the placenta is organized to provide a very large surface area (>10 m2) for that exchange.
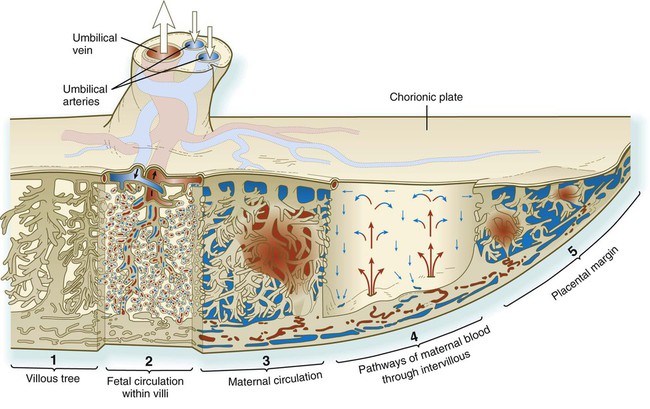
Blood enters the intervillous spaces from the open ends of the uterine spiral arteries. After bathing the villi, the blood (blue) is drained via endometrial veins. (From Bloom W, Fawcett DW: Textbook of histology, Philadelphia, 1986, Saunders.)
Structure of the Mature Placenta
The mature placenta is disklike in shape, 3 cm thick, and about 20 cm in diameter (Table 7.1). A typical placenta weighs about 500 g. The fetal side of the placenta is shiny because of the apposed amniotic membrane. From the fetal side, the attachment of the umbilical cord to the chorionic plate and the large placental branches of the umbilical arteries and vein radiating from it are evident.
Table 7.1
Age of Embryo (Weeks after Fertilization) | Placental Diameter (mm) | Placental Weight (g) | Placental Thickness (mm) | Length of Umbilical Cord (mm) | Embryo Weight (g)/ Placental Weight (g) | Villous Mass (g) | Total Villous Surface Area (cm2) | Diffusion Distance from Maternal to Fetal Circulation (µm) | Mean Trophoblastic Thickness on Villi (µm) |
6 | — | 6 | — | — | 0.18 | 5 | 830 | 55.9 | 15.4 |
10 | — | 26 | — | — | 0.65 | 18 | 3,020 | — | — |
14 | 70 | 65 | 12 | 180 | 0.92 | 28 | 5,440 | 40.2 | 9.6 |
18 | 95 | 115 | 15 | 300 | 2.17 | 63 | 14,800 | 27.7 | 9.9 |
22 | 120 | 185 | 18 | 350 | 3.03 | 102 | 28,100 | 21.6 | 7.4 |
26 | 145 | 250 | 20 | 400 | 4.00 | 135 | 42,200 | — | — |
30 | 170 | 315 | 22 | 450 | 4.92 | 191 | 72,200 | 20.6 | 6.9 |
34 | 195 | 390 | 24 | 490 | 5.90 | 234 | 101,000 | 11.7 | 5.2 |
38 | 220 | 470 | 25 | 520 | 7.23 | 273 | 125,000 | .8 | 4.1 |
Adapted from Kaufmann P, Scheffen I: In Polin R, Fox W, eds: Fetal and neonatal physiology, vol 1, Philadelphia, 1992, Saunders, p 48.
Umbilical Cord
The originally broad-based body stalk elongates and becomes narrower as pregnancy progresses. The umbilical cord becomes the conduit for the umbilical vessels, which traverse its length between the fetus and the placenta (see Fig. 7.10). The umbilical vessels are embedded in a mucoid connective tissue that is often called Wharton’s jelly.
Placental Circulation
Both the fetus and the mother contribute to the placental circulation (see Fig. 7.10). The fetal circulation is contained in the system of umbilical and placental vessels. Fetal blood reaches the placenta through the two umbilical arteries, which ramify throughout the chorionic plate. Smaller branches from these arteries enter the chorionic villi and break up into capillary networks in the terminal branches of the chorionic villi, where the exchange of materials with the maternal blood occurs (see Fig. 7.14). From the villous capillary beds, the blood vessels consolidate into successively larger venous branches. These retrace their way through the chorionic plate into the large single umbilical vein and to the fetus.
The maternal blood enters the intervillous space under reduced pressure because of the cytotrophoblastic plugs that partially occlude the lumens of the spiral arteries. Nevertheless, the maternal blood pressure is sufficient to force the oxygenated maternal arterial blood to the bases of the villous trees at the chorionic plate (see Fig. 7.10). The overall pressure of the maternal placental blood is about 10 mm Hg in the relaxed uterus. From the chorionic plate, the blood percolates over the terminal villi as it returns to venous outflow pathways located in the decidual (maternal) plate of the placenta. An adequate flow of maternal blood to the placenta is vital to the growth and development of the fetus, and a reduced maternal blood supply to the placenta leads to a small fetus.
In the terminal (floating) villi, the fetal capillaries are located next to the trophoblastic surface to facilitate exchange between the fetal and maternal blood (Fig. 7-11). The placental barrier of the mature placenta consists of the syncytiotrophoblast, its basal lamina, the basal lamina of the fetal capillary, and the capillary endothelium. Often the two basal laminae seem to be consolidated. In younger embryos, a layer of cytotrophoblast is present in the placental barrier, but by 4 months the cytotrophoblastic layer begins to break up, and by 5 months, it is essentially gone.
Structure of a Mature Chorionic Villus
Mature chorionic villi constitute a very complex mass of seemingly interwoven branches (Fig. 7.12). The core of a villus consists of blood vessels and mesenchyme that is similar in composition to the mesenchyme of the umbilical cord (see Fig. 7.11). Scattered among the mesenchymal cells are large Hofbauer cells, which function as fetal macrophages.
The villus core is covered by a continuous layer of syncytiotrophoblast, with minimum numbers of cytotrophoblastic cells beneath it. The surface of the syncytiotrophoblast is covered by immense numbers of microvilli (>1 billion/cm2 at term), which greatly increase the total surface area of the placenta (Fig. 7.13). The size and density of the microvilli are not constant, but they change with the increasing age of the placenta and differing environmental conditions. Under conditions of poor maternal nutrition or oxygen transport, the microvilli increase in prominence. Poor adaptation of the microvilli to adverse conditions can lead to newborns with low birth weight.
Placental Physiology
The transport of substances between the placenta and the maternal blood that bathes it is facilitated by the great surface area of the placenta, which expands from 5 m2 at 28 weeks to almost 11 m2 at term. Approximately 5% to 10% of the human placental surface consists of scattered areas where the barrier between fetal and maternal blood is extremely thin, measuring only a few micrometers. These areas, sometimes called epithelial plates, are apparently morphological adaptations designed to facilitate the diffusion of substances between the fetal and maternal circulations (Fig. 7.14).
The transfer of substances occurs both ways across the placenta. The bulk of the substances transferred from mother to fetus consists of oxygen and nutrients. The placenta represents the means for the final elimination of carbon dioxide and other fetal waste materials into the maternal circulation. Under some circumstances, other substances, some of them harmful, can be transferred across the placenta. Clinical Correlation 7.2 describes abnormal placental transfer.
Steroid hormones cross the placental barrier from the maternal blood. Newborn boys show evidence of the effects of exposure to maternal sex hormones. The prostatic utricle, the vestigial rudiment of the uterine primordium (fused paramesonephric ducts [see p. 394]), is slightly enlarged in newborn boys. Conversely, female fetuses exposed to testosterone or certain synthetic progestins (especially during the 1950s and 1960s, before the effects were recognized) undergo masculinization of the external genitalia. Protein hormones are, in general, poorly transported across the placenta, although symptoms of maternal diabetes may be reduced during late pregnancy because of insulin produced by the fetus. Maternal thyroid hormone gains slow access to the fetus.
Placental Immunology
The first possibility is that the fetal tissues, especially those of the placenta, which constitute the direct interface between fetus and mother, do not present foreign antigens to the mother’s immune system. To some extent, this hypothesis is true because neither the syncytiotrophoblast nor the nonvillous cytotrophoblast (cytotrophoblastic shell) expresses the two major classes of major histocompatibility antigens that trigger the immune response of the host in the rejection of typical foreign tissue grafts (e.g., a kidney transplant). These antigens are present, however, on cells of the fetus and in stromal tissues of the placenta. The expression of minor histocompatibility antigens (e.g., the HY antigen in male fetuses [see Chapter 16]) follows a similar pattern. Nevertheless, other minor antigens are expressed on trophoblastic tissues. In addition, because of breaks in the placental barrier, fetal red and white blood cells are frequently found circulating in the maternal blood. (In addition, maternal cells can colonize the fetus.) These cells should be capable of sensitizing the mother’s immune system.
Placenta After Birth
About 30 minutes after birth, the placenta, embryonic membranes, and remainder of the umbilical cord, along with much of the maternal decidua, are expelled from the uterus as the afterbirth. The fetal surface of the placenta is smooth, shiny, and grayish because of the amnion that covers the fetal side of the chorionic plate. The maternal surface is a dull red and may be punctuated with blood clots. The maternal surface of the placenta must be examined carefully because if a cotyledon is missing and is retained in the uterine wall, it could cause serious postpartum bleeding. Recognition of certain types of placental diseases can provide valuable clues to intrauterine factors that could affect the well-being of the newborn (Clinical Correlation 7.3).
Placenta and Membranes in Multiple Pregnancies
Several different configurations of the placenta and extraembryonic membranes are possible in multiple pregnancies. Dizygotic twins or monozygotic twins resulting from complete separation of blastomeres very early in cleavage can have completely separate placentas and membranes if the two embryos implant in distant sites on the uterine wall (Fig. 7.17A). In contrast, if the implantation sites are closer together, the placentas and chorions (which were initially separate at implantation) can fuse, although the vascular systems of the two embryos remain separate (Fig. 7.17B).
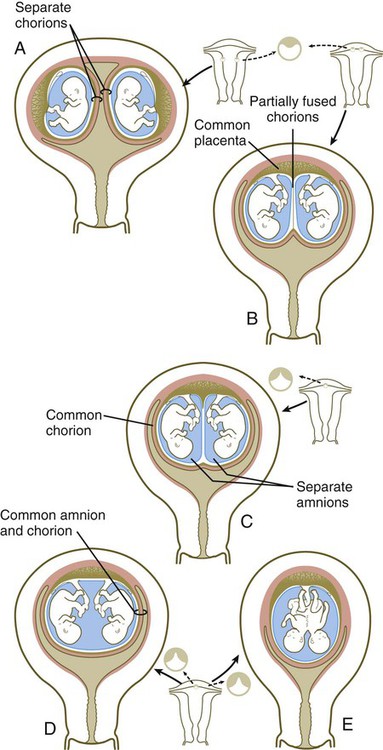
A, Completely separate membranes in dizygotic or completely separated monozygotic twins. B, Common fused placenta, separate amnions, and partially fused chorions. C, Common placenta with separate or common fused vessels and separate amnions enclosed in a common chorion. D and E, Common placenta and amniotic cavity in separate or conjoined twins.
When monozygotic twins form by splitting of the inner cell mass in the blastocyst, it is usual to have a common placenta and a common chorion, but inside the chorion the twin embryos each develop within separate amnions (Fig. 7.17C). In this case, there can be separate or fused vascular systems within the common placenta. When the vascular systems are fused, one twin may receive a greater proportion of the placental blood flow than the other (twin-to-twin transfusion syndrome). This situation may result in mild to severe stunting of growth of the embryo that receives the lesser amount of blood from the placenta. The twin from which the blood is siphoned is often highly misshapen and is commonly called an acardiac monster (Fig. 7.18).
In conjoined twins and, rarely, in monozygotic twins with minimal separation of the inner cell mass, the embryos develop within a single amnion and chorion and have a common placenta with a common blood supply (Fig. 7.17D and E). This and the previously described conditions can be determined by examination of the membranes of the afterbirth. It was previously thought that it could be determined whether twins were monozygotic or dizygotic by simple examination of the membranes. Although in most cases the correct inference can be made, this method is not foolproof. Other methods, ranging from simple observation of gender, eye color, and fingerprint patterns to determination of blood types or even DNA fingerprinting, should be used for a definitive determination. In the current age of organ and cell transplantation, it can be vital to know whether twins are monozygotic in the event that one develops a condition that can be treated by a transplant.
Summary
The extraembryonic membranes consist of the chorion (the combination of trophoblast plus underlying extraembryonic mesoderm), amnion, yolk sac, and allantois.
The amnion, a thin ectodermal membrane lined with mesoderm, grows to enclose the embryo like a balloon. It is filled with a clear fluid, which is generated from many sources, such as the fetal skin, the amnion itself, the fetal kidneys, and possibly the fetal vessels. At term, the volume of amniotic fluid approaches 1 L. Amniotic fluid is removed by exchange across the amniotic membrane and by fetal swallowing.
The yolk sac is a ventral, endodermally lined structure that does not serve a nutritive function in mammalian embryos. Mesodermal blood islands in the wall of the yolk sac form the first blood cells and vessels. Primordial germ cells are recognizable in the wall of the yolk sac, but they originate in extraembryonic mesoderm at the base of the allantois.
The allantois is a small, endodermally lined diverticulum off the ventral side of the hindgut. It does not serve a direct function of respiration or storage of wastes in humans. These functions are carried out through the placenta and the umbilical vessels that arise in conjunction with the allantois.
Chorionic villi form as outward projections from the trophoblast. Primary villi consist of projections of trophoblast alone. When a mesenchymal core forms within a villus, it is a secondary villus, and when the mesenchyme becomes vascularized, the villus is a tertiary villus. As villi mature, the cytotrophoblast in some villi grows through the syncytiotrophoblast as cytotrophoblastic cell columns and makes contact with the maternal endometrial tissue. Cytotrophoblast continues to grow around the blood-filled space surrounding the chorion to form a cytotrophoblastic shell, which is the direct interface between the fetal and maternal tissues. Villi that make direct contact with maternal tissues are anchoring villi; villi that do not make such contact are floating villi. Because chorionic villi float in a pool of maternal blood, the human placenta is designated a hemochorial placenta.
Stimulated by the implanting embryo, endometrial stromal cells undergo the decidual reaction. Maternal tissues that are lost at childbirth are, collectively, the decidua. The decidua basalis underlies the placenta; the decidua capsularis encircles the remainder of the chorion like a capsule; portions of the uterine wall not occupied by the fetal chorion are the decidua parietalis. As the fetal chorion matures, it becomes subdivided into a chorion laeve, in which the villi regress, and the chorion frondosum, which is the region of chorion nearest the basal tissues of the endometrium. The chorion frondosum ultimately develops into the placenta.
The mature placenta consists of the wall of the chorion (the chorionic plate) and numerous villi protruding from it. The fetal surface of the placenta is smooth and shiny because of the apposed amniotic membrane. The maternal surface is dull and lobulated, with cotyledons of numerous placental villi and their branches. The umbilical cord (formerly the body stalk) enters the middle of the placenta. Blood from the fetus reaches the placenta via the umbilical arteries. These arteries branch out into numerous small vessels that terminate into capillary loops in the ends of the placental villi. There, oxygen, nutrients, and wastes are exchanged between fetal and maternal blood, which bathes the villi. Fetal blood returns to the body of the mature fetus via a single umbilical vein. Maternal blood exiting from open-ended spiral arteries of the endometrium bathes the placental villi.
The transfer of substances from fetal to maternal blood must occur across the endothelium of the fetal capillaries, a basal lamina, and trophoblastic tissues before reaching the maternal blood. The transfer of substances is accomplished by passive and active mechanisms. In addition to normal substances, alcohol, certain drugs, and some infectious agents can pass from the maternal blood into the fetal circulation and interfere with normal development. If a fetus is Rh positive and the mother is Rh negative, maternal anti-Rh antibodies from a previous pregnancy can pass to the fetus to cause erythroblastosis fetalis.
The placenta produces a wide variety of hormones, many of which are normally synthesized in the hypothalamus and anterior pituitary gland. The first hormone released is HCG, which serves as the basis of many pregnancy tests. Other placental hormones are chorionic somatomammotropin (human placental lactogen), steroid hormones, human placental growth hormone, and chorionic thyrotropin and corticotropin.
The fetal and placental tissues are immunologically different from those of the mother, but the placenta and fetus are not immunologically rejected. The reason is still unclear, but some explanations involve reduced antigenicity of the trophoblastic tissues, paralysis of the mother’s immune system during pregnancy, and local immunological barriers between the fetus and mother.
The placenta is delivered about 30 minutes after the fetus as the afterbirth. Inspection of the placenta can reveal placental pathological conditions, missing cotyledons, or the arrangement of membranes in multiple pregnancies. The last finding can help to determine whether a multiple birth is monozygotic in origin. Placental pathological findings include abnormal gross shape, benign hydatidiform moles, and malignant choriocarcinomas.
*Other mammals have various arrangements of tissue layers through which materials must pass to be exchanged between mother and fetus. For example, in an epitheliochorial placenta, which is found in pigs, the fetal component of the placenta (chorion) rests on the uterine epithelium instead of being directly bathed in maternal blood.
*The term “deciduum” refers to tissues that are shed at birth. These include the extraembryonic tissues in addition to the superficial layers of the endometrial connective tissue and epithelium.