Getting Ready for Pregnancy
Gametogenesis
Phase 1: Origin and Migration of Germ Cells
Primordial germ cells, the earliest recognizable precursors of gametes, arise outside the gonads and migrate into the gonads during early embryonic development. Human primordial germ cells first become readily recognizable at 24 days after fertilization in the endodermal layer of the yolk sac (Fig. 1.1A) by their large size and high content of the enzyme alkaline phosphatase. In the mouse, their origin has been traced even earlier in development (see p. 390). Germ cells exit from the yolk sac into the hindgut epithelium and then migrate* through the dorsal mesentery until they reach the primordia of the gonads (Fig. 1.1B). In the mouse, an estimated 100 cells leave the yolk sac, and through mitotic multiplication (6 to 7 rounds of cell division), about 4000 primordial germ cells enter the primitive gonads.
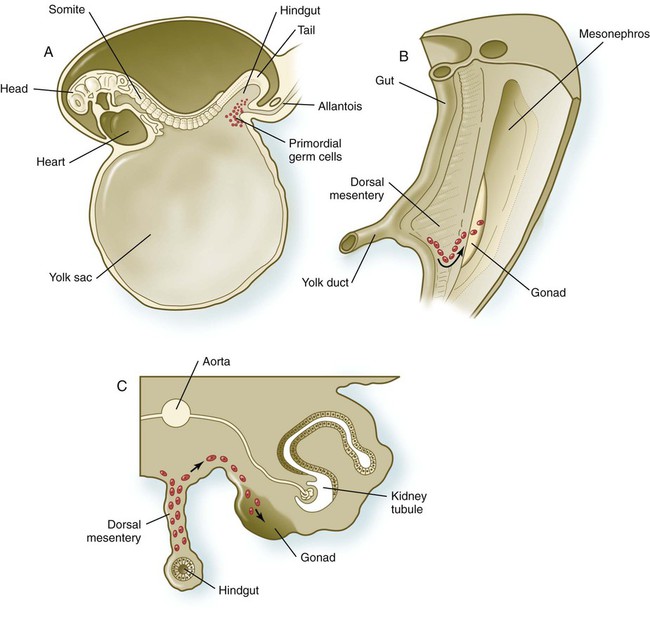
A, Location of primordial germ cells in the 16-somite human embryo (midsagittal view). B, Pathway of migration (arrow) through the dorsal mesentery. C, Cross section showing the pathway of migration (arrows) through the dorsal mesentery and into the gonad.
Misdirected primordial germ cells that lodge in extragonadal sites usually die, but if such cells survive, they may develop into teratomas. Teratomas are bizarre growths that contain scrambled mixtures of highly differentiated tissues, such as skin, hair, cartilage, and even teeth (Fig. 1.2). They are found in the mediastinum, the sacrococcygeal region, and the oral region.
Phase 2: Increase in the Number of Germ Cells by Mitosis
After they arrive in the gonads, the primordial germ cells begin a phase of rapid mitotic proliferation. In a mitotic division, each germ cell produces two diploid progeny that are genetically equal. Through several series of mitotic divisions, the number of primordial germ cells increases exponentially from hundreds to millions. The pattern of mitotic proliferation differs markedly between male and female germ cells. Oogonia, as mitotically active germ cells in the female are called, go through a period of intense mitotic activity in the embryonic ovary from the second through the fifth month of pregnancy in the human. During this period, the population of germ cells increases from only a few thousand to nearly 7 million (Fig. 1.3). This number represents the maximum number of germ cells that is ever found in the ovaries. Shortly thereafter, numerous oogonia undergo a natural degeneration called atresia. Atresia of germ cells is a continuing feature of the histological landscape of the human ovary until menopause.
Phase 3: Reduction in Chromosomal Number by Meiosis
Stages of Meiosis
Meiosis involves two sets of divisions (Fig. 1.4). Before the first meiotic division, deoxyribonucleic acid (DNA) replication has already occurred, so at the beginning of meiosis, the cell is 2n, 4c. (In this designation, n is the species number of chromosomes, and c is the amount of DNA in a single set [n] of chromosomes.) The cell contains the normal number (2n) of chromosomes, but as a result of replication, its DNA content (4c) is double the normal amount (2c).
In the first meiotic division, often called the reductional division, a prolonged prophase (see Fig. 1.4) leads to the pairing of homologous chromosomes and frequent crossing-over, resulting in the exchange of segments between members of the paired chromosomes. Crossing-over even occurs in the sex chromosomes. This takes place in a small region of homology between the X and Y chromosomes. Crossing-over is not a purely random process. Rather, it occurs at sites along the chromosomes known as hot spots. Their location is based on configurations of proteins that organize the chromosomes early in meiosis. One such protein is cohesin, which helps to hold sister chromatids together during division. Hypermethylation of histone proteins in the chromatin indicates specific sites where the DNA strands break and are later repaired after crossing-over is completed. Another protein, condensin, is important in compaction of the chromosomes, which is necessary for both mitotic and meiotic divisions to occur.
Meiosis in Females
Meiosis in the human female is a very leisurely process. As the primary oocytes enter the diplotene stage of the first meiotic division in the early months after birth, the first of two blocks in the meiotic process occurs (Fig. 1.5). The suspended diplotene phase of meiosis is the period when the primary oocyte prepares for the needs of the embryo. In oocytes of amphibians and other lower vertebrates, which must develop outside the mother’s body and often in a hostile environment, it is highly advantageous for the early stages of development to occur very rapidly so that the stage of independent locomotion and feeding is attained as soon as possible. These conditions necessitate a strategy of storing up the materials needed for early development well in advance of ovulation and fertilization because normal synthetic processes would not be rapid enough to produce the materials required for the rapidly cleaving embryo. In such species, yolk is accumulated, the genes for producing ribosomal ribonucleic acid (rRNA) are amplified, and many types of RNA molecules are synthesized and stored in an inactive form for later use.
RNA synthesis in the amphibian oocyte occurs on the lampbrush chromosomes, which are characterized by many prominent loops of spread-out DNA on which messenger RNA (mRNA) molecules are synthesized. The amplified genes for producing rRNA are manifested by the presence of 600 to 1000 nucleoli within the nucleus. Primary oocytes also prepare for fertilization by producing several thousand cortical granules, which are of great importance during the fertilization process (see Chapter 2).
Because cortical granules play an important role in preventing the entry of excess spermatozoa during fertilization in human eggs (see p. 31), the formation of cortical granules (mainly from the Golgi apparatus) continues to be one of the functions of the diplotene stage that is preserved in humans. Roughly 4500 cortical granules are produced in the mouse oocyte. A higher number is likely in the human oocyte.
With the completion of the first meiotic division shortly before ovulation, two unequal cellular progeny result. One is a large cell, called the secondary oocyte. The other is a small cell called the first polar body (see Fig. 1.5). The secondary oocytes begin the second meiotic division, but again the meiotic process is arrested, this time at metaphase. The stimulus for the release from this meiotic block is fertilization by a spermatozoon. Unfertilized secondary oocytes fail to complete the second meiotic division. The second meiotic division is also unequal; one of the daughter cells is relegated to becoming a second polar body. The first polar body may also divide during the second meiotic division. Formation of both the first and second polar bodies involves highly asymmetric cell divisions. To a large extent, this is accomplished by displacement of the mitotic spindle apparatus toward the periphery of the oocyte through the actions of the cytoskeletal protein actin (see Fig. 2.7).
Meiosis in Males
Meiosis in the male does not begin until after puberty. In contrast to the primary oocytes in the female, not all spermatogonia enter meiosis at the same time. Large numbers of spermatogonia remain in the mitotic cycle throughout much of the reproductive lifetime of males. When the progeny of a spermatogonium have entered the meiotic cycle as primary spermatocytes, they spend several weeks passing through the first meiotic division (Fig. 1.6). The result of the first meiotic division is the formation of two secondary spermatocytes, which immediately enter the second meiotic division. About 8 hours later, the second meiotic division is completed, and four haploid (1n, 1c) spermatids remain as progeny of the single primary spermatocyte. The total length of human spermatogenesis is 64 days.
Disturbances that can occur during meiosis and result in chromosomal aberrations are discussed in Clinical Correlation 1.1 and Figure 1.7.
Phase 4: Final Structural and Functional Maturation of Eggs and Sperm
Oogenesis
In the embryo, oogonia are naked, but after meiosis begins, cells from the ovary partially surround the primary oocytes to form primordial follicles (see Fig. 1.5). By birth, the primary oocytes are invested with a complete layer of follicular cells, and the complex of primary oocyte and the follicular (granulosa) cells is called a primary follicle (Fig. 1.8). Both the oocyte and the surrounding follicular cells develop prominent microvilli and gap junctions that connect the two cell types.
The meiotic arrest at the diplotene stage of the first meiotic division is the result of a complex set of interactions between the oocyte and its surrounding layer of follicular (granulosa) cells. The principal factor in maintaining meiotic arrest is a high concentration of cyclic adenosine monophosphate (cAMP) in the cytoplasm of the oocyte (Fig. 1.9). This is accomplished by both the intrinsic production of cAMP by the oocyte and the production of cAMP by the follicular cells and its transport into the oocyte through gap junctions connecting the follicular cells to the oocyte. In addition, the follicular cells produce and transport into the oocyte cyclic guanosine monophosphate (cGMP), which inactivates phosphodiesterase 3A (PDE3A), an enzyme that converts cAMP to 5′AMP. The high cAMP within the oocyte inactivates maturation promoting factor (MPF), which at a later time functions to lead the oocyte out of meiotic arrest and to complete the first meiotic division.
As the primary follicle takes shape, a prominent, translucent, noncellular membrane called the zona pellucida forms between the primary oocyte and its enveloping follicular cells (Fig. 1.10). The microvillous connections between the oocyte and follicular cells are maintained through the zona pellucida. In rodents, the components of the zona pellucida (four glycoproteins and glycosaminoglycans) are synthesized almost entirely by the egg, but in other mammals, follicular cells also contribute materials to the zona. The zona pellucida contains sperm receptors and other components that are important in fertilization and early postfertilization development. (The functions of these molecules are discussed more fully in Chapter 2.)
Early development of the follicle occurs without the significant influence of hormones, but as puberty approaches, continued follicular maturation requires the action of the pituitary gonadotropic hormone follicle-stimulating hormone (FSH) on the granulosa cells, which have by this time developed FSH receptors on their surfaces (see Fig. 1.10). In addition, the oocyte itself exerts a significant influence on follicular growth. After blood-borne FSH is bound to the FSH receptors, the stimulated granulosa cells produce small amounts of estrogens. The most obvious indication of the further development of some of the follicles is the formation of an antrum, a cavity filled with a fluid called liquor folliculi. Initially formed by secretions of the follicular cells, the antral fluid is later formed mostly as a transudate from the capillaries on the outer side of the membrana granulosa.
Formation of the antrum divides the follicular cells into two groups. The cells immediately surrounding the oocyte are called cumulus cells, and the cells between the antrum and the membrana granulosa become the mural granulosa cells. Factors secreted by the oocyte confer different properties on the cumulus cells from the mural granulosa cells. In the absence of a direct stimulus from the oocyte, the granulosa cells follow a default pathway and begin to assemble hormone receptors on their surface (see Fig. 1.10). In contrast, the cumulus cells do not express hormone receptors, but under the influence of the oocyte, they undergo changes that facilitate the release of the ovum at the time of ovulation.
Enlargement of the follicle results largely from the proliferation of granulosa cells. The direct stimulus for granulosa cell proliferation is a locally produced signaling protein, activin, a member of the transforming growth factor-β family of signaling molecules (see Table 4.1). The local action of activin is enhanced by the actions of FSH.
Responding to the stimulus of pituitary hormones, secondary follicles produce significant amounts of steroid hormones. The cells of the theca interna possess receptors for luteinizing hormone (LH), also secreted by the anterior pituitary (see Fig. 1.15). The theca interna cells produce androgens (e.g., testosterone), which pass through the membrana granulosa to the granulosa cells. The influence of FSH induces the granulosa cells to synthesize the enzyme (aromatase), which converts the theca-derived androgens into estrogens (mainly 17β-estradiol). Not only does the estradiol leave the follicle to exert important effects on other parts of the body, but also it stimulates the formation of LH receptors on the granulosa cells. Through this mechanism, the follicular cells are able to respond to the large LH surge that immediately precedes ovulation (see Fig. 1.16).
Under multiple hormonal influences, the follicle enlarges rapidly (Fig. 1.11; see Fig. 1.10) and presses against the surface of the ovary. At this point, it is called a tertiary (graafian) follicle. About 10 to 12 hours before ovulation, meiosis resumes.
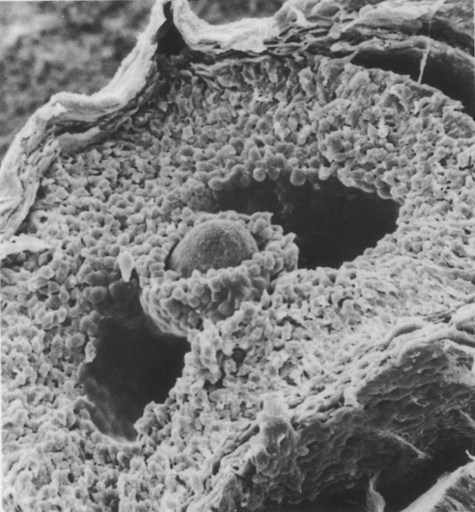
The spherical oocyte (center) is surrounded by smaller cells of the corona radiata, which projects into the antrum. (×840.) (Courtesy of P. Bagavandoss, Ann Arbor, Mich.)
The resumption of meiosis in response to the LH surge is initiated by the cumulus (granulosa) cells, because the oocyte itself does not possess LH receptors. Responding to LH, the cumulus cells shut down their gap junctions (see Fig. 1.9B). This reduces the transfer of both cAMP and cGMP from the cumulus cells into the oocyte. The resulting reduction of cGMP in the oocyte allows the activation of PDE3A. The activated PDE3A then breaks down the intra-oocytic cAMP into 5′AMP. The decline in the concentration of cAMP sets off a signaling pathway leading to the activation of MPF and the subsequent resumption of meiosis.
The tertiary follicle protrudes from the surface of the ovary like a blister. The granulosa cells contain numerous FSH and LH receptors, and LH receptors are abundant in the cells of the theca interna. The follicular cells secrete large amounts of estradiol (see Fig. 1.16), which prepares many other components of the female reproductive tract for gamete transport. Within the antrum, the follicular fluid contains the following: (1) a complement of proteins similar to that seen in serum, but in a lower concentration; (2) 20 enzymes; (3) dissolved hormones, including FSH, LH, and steroids; and (4) proteoglycans. The strong negative charge of the proteoglycans attracts water molecules, and with greater amounts of secreted proteoglycans, the volume of antral fluid increases correspondingly. The follicle is now poised for ovulation and awaits the stimulus of the preovulatory surge of FSH and LH released by the anterior pituitary gland.
The reason only one follicle normally matures to the point of ovulation is still not completely understood. Early in the cycle, as many as 50 follicles begin to develop, but only about 3 attain a diameter of as great as 8 mm. Initial follicular growth is gonadotropin independent, but continued growth depends on a minimum “tonic” level of gonadotropins, principally FSH. During the phase of gonadotropin-induced growth, a dominant enlarging follicle becomes independent of FSH and secretes large amounts of inhibin (see p. 19). Inhibin suppresses the secretion of FSH by the pituitary, and when the FSH levels fall below the tonic threshold, the other developing follicles, which are still dependent on FSH for maintenance, become atretic. The dominant follicle acquires its status about 7 days before ovulation. It may also secrete an inhibiting substance that acts directly on the other growing follicles.
Spermatogenesis
All spermatogonia are sequestered at the base of the seminiferous epithelium by interlocking processes of Sertoli cells, which are complex cells that are regularly distributed throughout the periphery of the seminiferous epithelium and that occupy about 30% of its volume (see Fig. 1.6). As the progeny of the type B spermatogonia (called primary spermatocytes) complete the leptotene stage of the first meiotic division, they pass through the Sertoli cell barrier to the interior of the seminiferous tubule. This translocation is accomplished by the formation of a new layer of Sertoli cell processes beneath these cells and, slightly later, the dissolution of the original layer that was between them and the interior of the seminiferous tubule. The Sertoli cell processes are very tightly joined and form an immunological barrier (blood-testis barrier [see Fig. 1.6]) between the forming sperm cells and the rest of the body, including the spermatogonia. When they have begun meiosis, developing sperm cells are immunologically different from the rest of the body. Autoimmune infertility can arise if the blood-testis barrier is broken down.
The progeny of the type B spermatogonia, which have entered the first meiotic division, are the primary spermatocytes (see Fig. 1.6). Located in a characteristic position just inside the layer of spermatogonia and still deeply embedded in Sertoli cell cytoplasm, primary spermatocytes spend 24 days passing through the first meiotic division. During this time, the developing sperm cells use a strategy similar to that of the egg—producing in advance molecules that are needed at later periods when changes occur very rapidly. Such preparation involves the production of mRNA molecules and their storage in an inactive form until they are needed to produce the necessary proteins.
Several major categories of change occur during spermiogenesis (Fig. 1.12). One is the progressive reduction in the size of the nucleus and tremendous condensation of the chromosomal material, which is associated with the replacement of histones by protamines. Along with the changes in the nucleus, a profound reorganization of the cytoplasm occurs. Cytoplasm streams away from the nucleus, but a condensation of the Golgi apparatus at the apical end of the nucleus ultimately gives rise to the acrosome. The acrosome is an enzyme-filled structure that plays a crucial role in the fertilization process. At the other end of the nucleus, a prominent flagellum grows out of the centriolar region. Mitochondria are arranged in a spiral around the proximal part of the flagellum. During spermiogenesis, the plasma membrane of the head of the sperm is partitioned into several antigenically distinct molecular domains. These domains undergo numerous changes as the sperm cells mature in the male and at a later point when the spermatozoa are traveling through the female reproductive tract. As spermiogenesis continues, the remainder of the cytoplasm (residual body [see G in Fig. 1.12]) moves away from the nucleus and is shed along the developing tail of the sperm cell. The residual bodies are phagocytized by Sertoli cells (Box 1.1 and Fig. 1.13).
On completion of spermiogenesis (approximately 64 days after the start of spermatogenesis), the spermatozoon is a highly specialized cell well adapted for motion and the delivery of its packet of DNA to the egg. The sperm cell consists of the following: a head (2 to 3 µm wide and 4 to 5 µm long) containing the nucleus and acrosome; a midpiece containing the centrioles, the proximal part of the flagellum, and the mitochondrial helix; and the tail (about 50 µm long), which consists of a highly specialized flagellum (see Fig. 1.12). (Specific functional properties of these components of the sperm cell are discussed in Chapter 2.)
Preparation of the Female Reproductive Tract for Pregnancy
Structure
Ovaries and Uterine Tubes
The almond-shaped ovaries, located on either side of the uterus, are positioned very near the open, funnel-shaped ends of the ampullary segments of the uterine tubes. Numerous fingerlike projections, called fimbriae (Fig. 1.14), project toward the ovary from the open infundibulum of the uterine tube and are involved in directing the ovulated egg into the tube. The uterine tube is characterized by a complex internal lining, with a high density of prominent longitudinal folds in the upper ampulla. These folds become progressively simpler in parts of the tube closer to the uterus. The lining epithelium of the uterine tubes contains a mixture of ciliated cells that assist in gamete transport and secretory cells that produce a fluid supporting the early development of the embryo. Layers of smooth muscle cells throughout the uterine tubes provide the basis for peristaltic contractions. The amount and function of many of these components are under cyclic hormonal control, and the overall effect of these changes is to facilitate the transport of gametes and the fertilized egg.
The two segments of the uterine tubes closest to the uterus play particularly important roles as pathways for sperm transport toward the ovulated egg. The intramural segment, which is embedded in the uterine wall, has a very thin lumen containing mucus, the composition of which varies with phases in the menstrual cycle. This segment serves as a gateway regulating the passage of spermatozoa into the uterine tube, but it also restricts the entry of bacteria into the tube. The middle isthmus segment serves as an important site of temporary sperm storage and participates in the final stages of functional maturation of sperm cells (see Chapter 2).
Uterus
The uterus is a pear-shaped organ with thick walls of smooth muscle (myometrium) and a complex mucosal lining (see Fig. 1.14). The mucosal lining, called the endometrium, has a structure that changes daily throughout the menstrual cycle. The endometrium can be subdivided into two layers: a functional layer, which is shed with each menstrual period or after parturition, and a basal layer, which remains intact. The general structure of the endometrium consists of (1) a columnar surface epithelium, (2) uterine glands, (3) a specialized connective tissue stroma, and (4) spiral arteries that coil from the basal layer toward the surface of the endometrium. All these structures participate in the implantation and nourishment of the embryo.
Hormonal Control of the Female Reproductive Cycle
Reproduction in women is governed by a complex series of interactions between hormones and the tissues that they influence. The hierarchy of cyclic control begins with input to the hypothalamus of the brain (Fig. 1.15). The hypothalamus influences hormone production by the anterior lobe of the pituitary gland. The pituitary hormones are spread via the blood throughout the entire body and act on the ovaries, which are stimulated to produce their own sex steroid hormones. During pregnancy, the placenta exerts a powerful effect on the mother by producing several hormones. The final level of hormonal control of female reproduction is exerted by the ovarian or placental hormones on other reproductive target organs (e.g., uterus, uterine tubes, vagina, breasts).
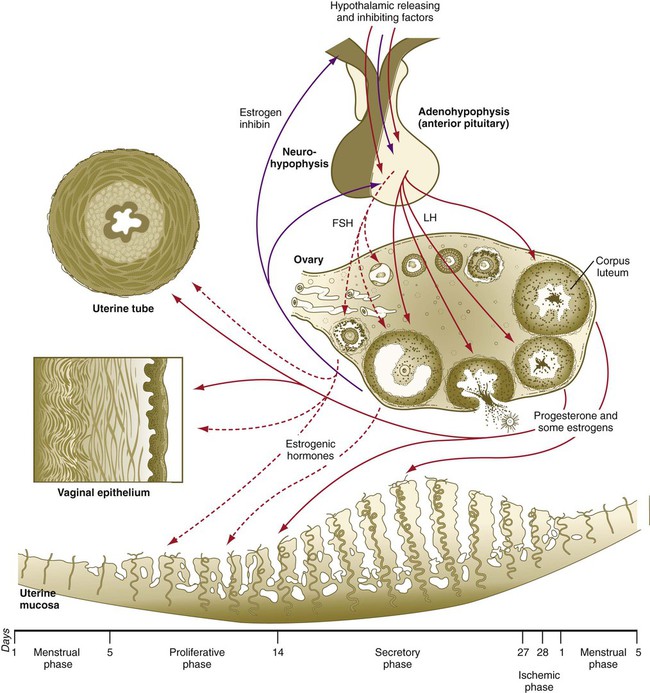
Inhibitory factors are represented by purple arrows. Stimulatory factors are represented by red arrows. Hormones involved principally in the proliferative phase of the menstrual cycle are represented by dashed arrows; those involved principally in the secretory phase are represented by solid arrows. FSH, follicle-stimulating hormone; LH, luteinizing hormone.
Hypothalamic Control
The first level of hormonal control of reproduction is in the hypothalamus. Various inputs stimulate neurosecretory cells in the hypothalamus to produce gonadotropin-releasing hormone (GnRH), along with releasing factors for other pituitary hormones. Releasing factors and an inhibiting factor are carried to the anterior lobe of the pituitary gland by blood vessels of the hypothalamohypophyseal portal system, where they stimulate the secretion of pituitary hormones (Table 1.1).
Table 1.1
Major Hormones Involved in Mammalian Reproduction
Hormone | Chemical Nature | Function |
HYPOTHALAMUS | ||
Gonadotropin-releasing hormone (GnRH, LHRH) | Decapeptide | Stimulates release of LH and FSH by anterior pituitary |
Prolactin-inhibiting factor | Dopamine | Inhibits release of prolactin by anterior pituitary |
ANTERIOR PITUITARY | ||
Follicle-stimulating hormone (FSH) | Glycoprotein (α and β subunits) (MW ≈35,000) | Male: Stimulates Sertoli cells to produce androgen-binding protein |
Female: Stimulates follicle cells to produce estrogen | ||
Luteinizing hormone (LH) | Glycoprotein (α and β subunits) (MW ≈28,000) | Male: Stimulates Leydig cells to secrete testosterone |
Female: Stimulates follicle cells and corpus luteum to produce progesterone | ||
Prolactin | Single-chain polypeptide (198 amino acids) | Promotes lactation |
POSTERIOR PITUITARY | ||
Oxytocin | Oligopeptide (MW ≈1100) | Stimulates ejection of milk by mammary gland |
Stimulates uterine contractions during labor | ||
OVARY | ||
Estrogens | Steroid | Has multiple effects on reproductive tract, breasts, body fat, and bone growth |
Progesterone | Steroid | Has multiple effects on reproductive tract and breast development |
Testosterone | Steroid | Is precursor for estrogen biosynthesis, induces follicular atresia |
Inhibin | Protein (MW ≈32,000) | Inhibits FSH secretion, has local effects on ovaries |
Activin | Protein (MW ≈28,000) | Stimulates granulosa cell proliferation |
TESTIS | ||
Testosterone | Steroid | Has multiple effects on male reproductive tract, hair growth, and other secondary sexual characteristics |
Inhibin | Protein (MW ≈32,000) | Inhibits FSH secretion, has local effects on testis |
PLACENTA | ||
Estrogens | Steroid | Has same functions as ovarian estrogens |
Progesterone | Steroid | Has same functions as ovarian progesterone |
Human chorionic gonadotropin (HCG) | Glycoprotein (MW ≈30,000) | Maintains activity of corpus luteum during pregnancy |
Human placental lactogen (somatomammotropin) | Polypeptide (MW ≈20,000) | Promotes development of breasts during pregnancy |
LHRH, luteinizing hormone–releasing hormone; MW, molecular weight.
Pituitary Gland (Hypophysis)
Under the influence of GnRH and direct feedback by steroid hormone levels in the blood, the anterior pituitary secretes two polypeptide gonadotropic hormones, FSH and LH, from the same cell type (see Table 1.1). In the absence of an inhibiting factor (dopamine) from the hypothalamus, the anterior pituitary also produces prolactin, which acts on the mammary glands.
Ovaries and Placenta
The ovaries and, during pregnancy, the placenta constitute a third level of hormonal control. Responding to blood levels of the anterior pituitary hormones, the granulosa cells of the ovarian follicles convert androgens (androstenedione and testosterone) synthesized by the theca interna into estrogens (mainly estrone and the 10-fold more powerful 17β-estradiol), which then pass into the bloodstream. After ovulation, progesterone is the principal secretory product of the follicle after its conversion into the corpus luteum (see Chapter 2). During later pregnancy, the placenta supplements the production of ovarian steroid hormone by synthesizing its own estrogens and progesterone. It also produces two polypeptide hormones (see Table 1.1). Human chorionic gonadotropin (HCG) acts on the ovary to maintain the activity of the corpus luteum during pregnancy. Human placental lactogen (somatomammotropin) acts on the corpus luteum; it also promotes breast development by enhancing the effects of estrogens and progesterone and stimulates the synthesis of milk constituents.
Hormonal Interactions with Tissues during Female Reproductive Cycles
During the preovulatory, or proliferative, phase (days 5 to 14) of the menstrual cycle, estrogens produced by the ovary act on the female reproductive tissues (see Fig. 1.15). The uterine lining becomes re-epithelialized from the just-completed menstrual period. Then, under the influence of estrogens, the endometrial stroma progressively thickens, the uterine glands elongate, and the spiral arteries begin to grow toward the surface of the endometrium. The mucous glands of the cervix secrete glycoprotein-rich but relatively watery mucus, which facilitates the passage of spermatozoa through the cervical canal. As the proliferative phase progresses, a higher percentage of the epithelial cells lining the uterine tubes becomes ciliated, and smooth muscle activity in the tubes increases. In the days preceding ovulation, the fimbriated ends of the uterine tubes move closer to the ovaries.
Toward the end of the proliferative period, a pronounced increase in the levels of estradiol secreted by the developing ovarian follicle acts on the hypothalamohypophyseal system, thus causing increased responsiveness of the anterior pituitary to GnRH and a surge in the hypothalamic secretion of GnRH. Approximately 24 hours after the level of 17β-estradiol reaches its peak in the blood, a preovulatory surge of LH and FSH is sent into the bloodstream by the pituitary gland (Fig. 1.16). The LH surge is not a steady increase in gonadotropin secretion; rather, it constitutes a series of sharp pulses of secretion that appear to be responding to a hypothalamic timing mechanism.
After the LH surge and with the increasing concentration of progesterone in the blood, the basal body temperature increases (see Fig. 1.16). Because of the link between an increase in basal body temperature and the time of ovulation, accurate temperature records are the basis of the rhythm method of birth control.
Hormonal Interactions Involved with Reproduction in Males
Along with the homologies of certain structures between the testis and ovary, some strong parallels exist between the hormonal interactions involved in reproduction in males and females. The most important homologies are between granulosa cells in the ovarian follicle and Sertoli cells in the seminiferous tubule of the testis and between theca cells of the ovary and Leydig cells in the testis (Table 1.2).
Table 1.2
Homologies between Hormone-Producing Cells in Male and Female Gonads
Parameter | Granulosa Cells (Female) | Sertoli Cells (Male) | Theca Cells (Female) | Leydig Cells (Male) |
Origin | Rete ovarii | Rete testis | Stromal mesenchyme | Stromal mesenchyme |
Major receptors | FSH | FSH | LH | LH |
Major secretory products | Estrogens, progesterone, inhibin | Estrogen, inhibin, androgen-binding protein, Leydig cell stimulatory factor | Androgens | Testosterone |
Sertoli cells are stimulated by pituitary FSH via surface FSH receptors and by testosterone from the Leydig cells via cytoplasmic receptors. After FSH stimulation, the Sertoli cells convert some of the testosterone to estrogens (as the granulosa cells in the ovary do). Some of the estrogen diffuses back to the Leydig cells along with a Leydig cell stimulatory factor, which is produced by the Sertoli cells and reaches the Leydig cells by a paracrine (non–blood-borne) mode of secretion (Fig. 1.17). The FSH-stimulated Sertoli cell produces androgen-binding protein, which binds testosterone and is carried into the fluid compartment of the seminiferous tubule, where it exerts a strong influence on the course of spermatogenesis. Similarly, their granulosa cell counterparts in the ovary, the hormone-stimulated Sertoli cells produce inhibin, which is carried by the blood to the anterior pituitary and possibly the hypothalamus. There inhibin acts by negative feedback to inhibit the secretion of FSH. In addition to inhibin and androgen-binding protein, the Sertoli cells have a wide variety of other functions, the most important of which are summarized in Box 1.2 and Clinical Correlation 1.2.
Summary
Gametogenesis is divided into four phases:
1. Extraembryonic origin of germ cells and their migration into the gonads
2. An increase in the number of germ cells by mitosis
Primordial germ cells are first readily recognizable in the yolk sac endoderm. They then migrate through the dorsal mesentery to the primordia of the gonads.
In the female, oogonia undergo intense mitotic activity in the embryo only. In the male, spermatogonia are capable of mitosis throughout life.
Meiosis involves a reduction in chromosome number from diploid to haploid, independent reassortment of paternal and maternal chromosomes, and further redistribution of genetic material through the process of crossing-over.
In the oocyte, there are two meiotic blocks—in diplotene of prophase I and in metaphase II. In the female, meiosis begins in the 5-month embryo; in the male, meiosis begins at puberty.
Failure of chromosomes to separate properly during meiosis results in nondisjunction, which is associated with multiple anomalies, depending on which chromosome is affected.
Developing oocytes are surrounded by layers of follicular cells and interact with them through gap junctions. When stimulated by pituitary hormones (e.g., FSH, LH), the follicular cells produce steroid hormones (estrogens and progesterone). The combination of oocyte and follicular (granulosa) cells is called a follicle. Under hormonal stimulation, certain follicles greatly increase in size, and each month, one of these follicles undergoes ovulation.
Spermatogenesis occurs in the testis and involves successive waves of mitosis of spermatogonia, meiosis of primary and secondary spermatocytes, and final maturation (spermiogenesis) of postmeiotic spermatids into spermatozoa. Functional maturation of spermatozoa occurs in the epididymis.
Female reproductive tissues undergo cyclic, hormonally induced preparatory changes for pregnancy. In the uterine tubes, this involves the degree of ciliation of the epithelium and smooth muscle activity of the wall. Under the influence of estrogens and then progesterone, the endometrium of the uterus builds up in preparation to receive the embryo. In the absence of fertilization and with the subsequent withdrawal of hormonal support, the endometrium breaks down and is shed (menstruation). Cyclic changes in the cervix involve thinning of the cervical mucus at the time of ovulation.
Hormonal control of the female reproductive cycle is hierarchical, with releasing or inhibiting factors from the hypothalamus acting on the adenohypophysis and causing the release of pituitary hormones (e.g., FSH, LH). The pituitary hormones sequentially stimulate the ovarian follicles to produce estrogens and progesterone, which act on the female reproductive tissues. In pregnancy, the remains of the follicle (corpus luteum) continue to produce progesterone, which maintains the early embryo until the placenta begins to produce sufficient hormones to maintain pregnancy.
In the male, LH stimulates the Leydig cells to produce testosterone, and FSH acts on the Sertoli cells, which support spermatogenesis. In males and females, feedback inhibition decreases the production of pituitary hormones.
*Considerable controversy surrounds the use of the term “migration” with respect to embryonic development. On the one hand, some believe that displacements of cells relative to other structural landmarks in the embryo are due to active migration (often through ameboid motion). On the other hand, others emphasize the importance of directed cell proliferation and growth forces in causing what is interpreted as apparent migration of cells. As is often true in scientific controversies, both active migration and displacement as a result of growth seem to operate in many cases where cells in the growing embryo appear to shift with respect to other structural landmarks.