Sense Organs
The major sense organs arise in large measure from the thickened ectodermal placodes that appear lateral to the neural plate in the early embryo (see Fig. 6.6). The following descriptions begin with the most cranial placodes and continue to the most caudal. The midline hypophyseal placode, located in the anterior neural ridge (see Fig. 6.6B), becomes the primordium of Rathke’s pouch (the precursor of the adenohypophysis). This structure arises adjacent to the neural tissue that ultimately forms the neurohypophysis. Arising also from the anterior neural region, bilateral olfactory placodes (see Fig. 6.6) are the precursors of the olfactory epithelium. They give rise to olfactory neurons and their supporting cells and to glial cells and neuroendocrine cells that migrate from the placode into the brain. Closely associated with the olfactory placodes is the preneural tissue that forms the functionally associated olfactory bulbs of the brain. The bilateral lens placodes, associated with the optic vesicles (future retina) extending outward from the diencephalic part of the brain, are the lens precursors.
Next in line are the paired trigeminal placodes (cranial nerve V), each of which arises from two placodal precursors—ophthalmic and maxillomandibular (see Fig. 6.6C). The otic placodes (precursors of the inner ear) in the human are the remaining representatives of the dorsolateral series of placodes, all of which produce vibration-detecting organs. In fishes and some amphibians, the other members of the dorsolateral series give rise to the lateral line organs, which serve as vibration and electroreceptors in aquatic vertebrates.
The caudalmost group constitutes the epibranchial placodes, which are located just dorsal to the region where the first through third pharyngeal pouches abut the cervical ectoderm (see Fig. 6.6A). Their specification depends on signals (fibroblast growth factor [FGF] and bone morphogenetic protein [BMP]) emanating from the pharyngeal pouch endoderm. These placodes produce sensory neurons that supply visceral structures. The first epibranchial placode produces neurons (geniculate ganglion of cranial nerve VII) (Fig. 13.1B) that innervate taste buds. Similarly, neurons arising from the second epibranchial placode (inferior [petrosal] ganglion of cranial nerve IX) innervate taste buds, as well as the heart and other visceral organs. The third epibranchial placode contributes to the inferior (nodose) ganglion of the vagus nerve (cranial nerve X), and its neurons innervate the heart, stomach, and other viscera. Of cranial nerves V, VII, IX, and X, the proximal sensory ganglia are derived largely from neural crest cells, and the distal ganglia are principally placodal in origin (see Fig. 13.1B). The placode-derived neurons (those in the distal ganglia) begin to establish peripheral and central connections before axons emerge in the neural crest–derived neuronal precursors in the proximal ganglia.
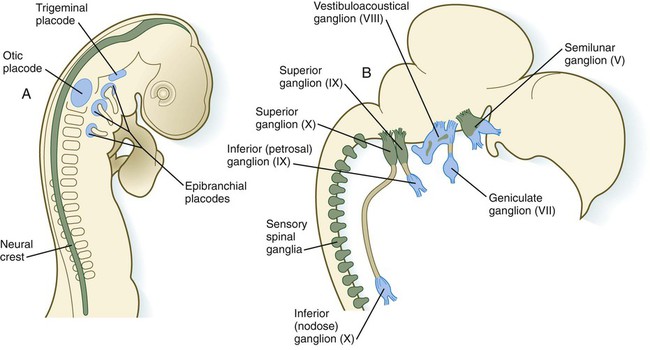
A, At 2 days. B, At 8 days. Neural crest is shown in green; placodes are shown in blue. (Adapted from LeDouarin N and others: Trends Neurosci 9:175-180, 1986.)
A major function of both the epibranchial and trigeminal placodes is to produce neurons. The conversion of epithelial cells to neuroblasts in the placodes is accomplished in much the same manner as that which occurs within the neural tube (see Fig. 11.4). Through the process called interkinetic nuclear migration, actual cell division occurs at the apical (in this case, outer) end of the tall epithelial placodal cells. Then the nuclei migrate toward the basal (inner) surface of the cells as they become committed to a neuronal fate as sensory neuroblasts. They then pass through breaches in the basal lamina and migrate internally. There they join other neuroblasts from the same origin to form precursors of the appropriate sensory ganglia.
All the placodes arise from a single preplacodal region, which encircles the cranial neural plate. The preplacodal region is induced by cranial mesoderm, with the neural tube playing a supporting role. The inductive process involves activating the FGF pathway, along with inhibiting Wnt and BMP by their natural antagonists. Levels of BMP must be lower to induce placodes rather than neural crest, and there is a gradient of BMP from highest in the neural plate, to medium for neural crest, and lowest for placodal induction. Characteristic of the induced preplacodal tissue is the expression of the transcription factors, Six and Eya, which promote a generic placodal fate to the cells within the preplacodal region. This is followed by specific secondary inductive signals from different sources that specify the formation of individual placodes (Table 13.1).
Table 13.1
Origins and Inducing Tissues for Cranial Placodes
Placode | Origin | Inducing Tissue | Inducers |
Adenohypophysis | Anterior neural ridge | Anterior neural ridge | Shh |
Non-neural ectoderm | Mesoderm | Nodal | |
Lens | Anterior neural ridge | Neural plate | FGFR |
Non-neural ectoderm | Mesoderm | BMP | |
Olfactory | Anterior neural ridge | Anterior neural ridge | FGF |
Non-neural ectoderm | Neural crest | ||
Otic | Neural folds | Hindbrain | FGF |
Non-neural ectoderm | Mesoderm | FGFR | |
Retinoic acid | |||
Trigeminal | Non-neural ectoderm | Dorsal neural tube | PDGF |
Wnt | |||
Epibranchial | Non-neural ectoderm | Hindbrain | FGF |
Mesoderm | FGFR |
Modified from McCabe KL, Bronner-Fraser M: Dev Biol 332:192, 2009.
This chapter concentrates on the development of the eyes and ears, the most complex and important sense organs in humans. The organs of smell and taste are discussed in Chapter 14 because their development is intimately associated with the development of the face and pharynx. The sensory components of the cranial nerves are discussed in Chapter 11.
Eye
Early Events in the Establishment of the Eye
A single continuous eye field begins to take shape around the area of the prechordal plate during late gastrulation. Cells in the eye field express RAX (retina and anterior neural fold homeobox). Mutations in RAX are the basis for anophthalmia, a rare condition characterized by the absence of any ocular structures in humans. Other prominent markers are Pax-6 and Lhx-2, which are heavily involved in patterning the eye fields (Fig. 13.2). With the secretion of sonic hedgehog (shh) by the prechordal plate and the ventral midline of the diencephalon, Pax-6 expression in the midline is repressed, and the single eye field splits into two separate eye fields, located on either side of the diencephalon. Rax and another important transcription factor, Six-3, protect the ability of the forebrain to secrete shh by suppressing Wnt activity. If Wnt is not suppressed, the anterior region of the developing brain becomes posteriorized and is unable to secrete shh. The absence of Six-3 activity results in the loss of shh secretion and prevents the splitting of the eye fields and leads to the condition of holoprosencephaly and the formation of only one eye (see p. 309 and Fig. 8.18).
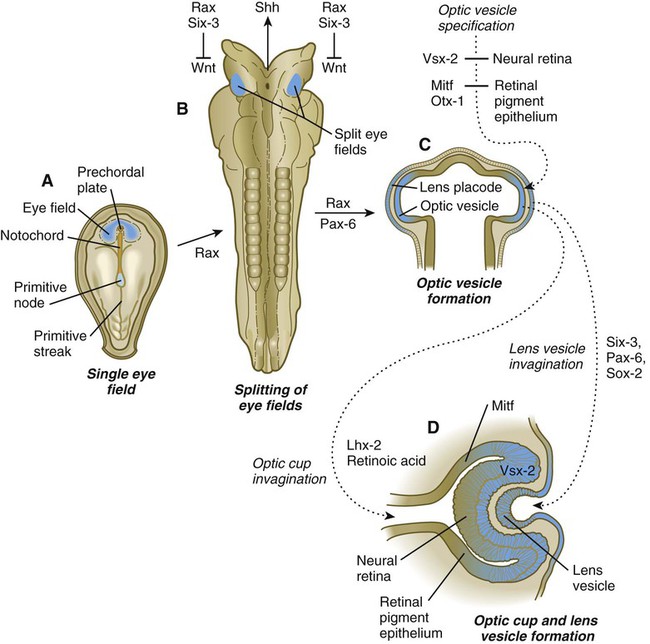
A, A single eye field forms during late gastrulation. B, Through the action of sonic hedgehog (Shh), the single eye field splits into bilateral fields. C, The formation of optic vesicles is heavily dependent on the expression of Pax-6 and Rax. The optic vesicles are patterned into future neural retina (through Vsx-2) and retinal pigment epithelium (through Mitf and Otx-2). D, Inductive interactions between the optic vesicle and the overlying lens placode result in invagination of both the optic cup and the lens vesicle.
Development of the eye is first evident at about 22 days’ gestation, when the lateral walls of the diencephalon begin to bulge out as optic grooves (Fig. 13.3). Within a few days, the optic grooves enlarge to form optic vesicles, which terminate very close to the overlying lens placode in the surface ectoderm. As the optic vesicle expands, the pattern for the future neural retina and retinal pigment epithelium is laid down. Initially, the transcription factor Mitf is expressed throughout the optic vesicle, but the subsequent expression of Vsx-2 in the distal optic vesicle (future neural retina) confines Mitf to more proximal regions, which will become the retinal pigment epithelium. Apposition of the outer wall of the optic vesicle to the surface ectoderm is essential for the transmission of an important inductive message that stimulates the cells of the lens placode to thicken and begin forming the lens (see Fig. 13.2; Fig. 13.4).
As the process of lens induction occurs, the surface ectoderm stimulates the outer face of the optic vesicle to flatten and ultimately to become concave. This results in the transformation of the optic vesicle to the optic cup (see Fig. 13.3F). The progression from optic vesicle to optic cup requires the expression of Lhx-2 and the action of retinoic acid. In their absence, eye development is arrested at the optic vesicle stage (see Fig. 13.2). Meanwhile, the induced lens ectoderm thickens and invaginates to form a lens vesicle, which detaches from the surface epithelium from which it originated (Fig. 13.5; see Fig. 13.3). Then the lens vesicle takes over and becomes the primary agent of a new inductive reaction by acting on the overlying surface ectoderm and causing it to begin corneal development (see Fig. 13.4).
Formation of the optic cup is an asymmetric process that occurs at the ventral margin of the optic vesicle, rather than at its center. This results in the formation of a gap called the choroid fissure, which is continuous with a groove in the optic stalk (Fig. 13.6). During much of early ocular development, the choroid fissure and optic groove form a channel through which the hyaloid artery passes into the posterior chamber of the eye. Differential expression of Pax genes determines which cells become optic cup (future retina), and which cells become optic stalk (future optic nerve). Through exposure to high concentrations of shh, the expression of Pax-6 is inhibited, and Pax-2 is induced in the optic stalk, whereas a lower concentration of shh more distally permits the expression of Pax-6 in the optic vesicle, thus paving the way toward formation of the retina.
Later in development, the choroid fissure closes, and no trace of it is seen in the normal iris. Nonclosure of the choroid fissure results in the anomaly of coloboma (see Fig. 13.19B). In certain forms of coloboma, especially forms that are associated with anomalies of the kidneys, mutations of Pax-2 genes are seen. In Pax-2–mutant mice, retinal axons do not cross the midline through the optic chiasm, but rather remain in the ipsilateral optic tract.
Formation of the Lens
The lens is derived from cells in the generic preplacodal region, as discussed earlier. From the earliest stages, formation of the lens depends on genetic instructions provided by Pax-6. Pax-6 expression is required for the surface ectoderm to respond to inductive signals (FGF and BMP) from the underlying optic vesicle by activating and combining with another transcription factor, Sox-2. This leads to the thickening of the surface ectoderm to form the lens placode (see Fig. 13.3D). At the same time, migrating neural crest cells, which do not penetrate the space between optic vesicle and prospective lens, give off signals that inhibit cells in other areas of the preplacodal region from forming lens. Inhibition of lens-forming capacity is signaled by the downregulation of Pax-6 by these cells. Pax-6 expression continues as the lens placode invaginates to form the lens vesicle, which ultimately breaks off from the surface ectoderm. At this point, Pax-6 plays a new role in regulating the activity of the genes governing the formation of the lens crystallin proteins.
When it is breaking off from the surface ectoderm, the lens vesicle is roughly spherical and has a large central cavity (see Fig. 13.3E). At the end of the sixth week, the cells at the inner pole of the lens vesicle begin to elongate in an early step toward their transformation into the long, transparent cells called lens fibers (Fig. 13.7A). The influence of the transcriptional regulator Foxe-3, which operates downstream of Pax-6, facilitates the breaking off of the lens vesicle from the surface ectoderm and the transformation of posterior cells into lens fibers.
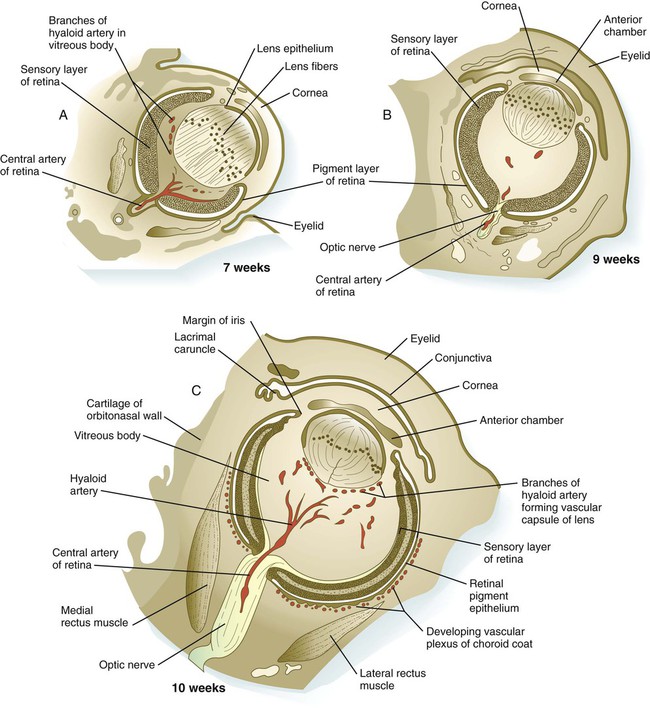
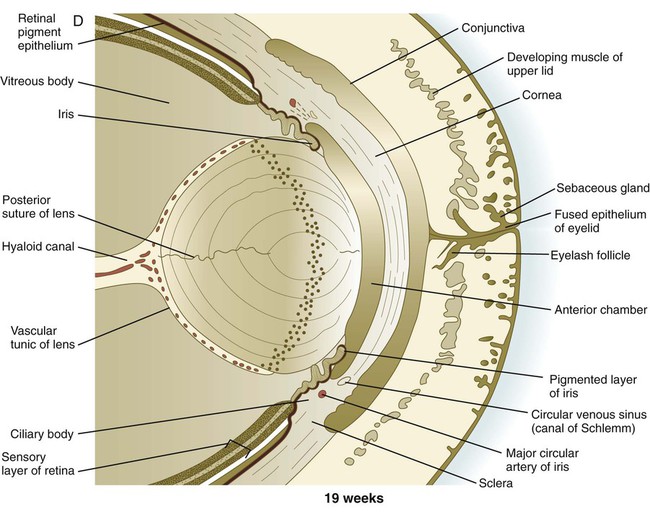
A, At 7 weeks. B, At 9 weeks. C, At 10 weeks. D, At 19 weeks. (Adapted from Carlson B: Patten’s foundations of embryology, ed 6, New York, 1996, McGraw-Hill.)
The formation of crystallin-containing lens fibers begins with the elongation of epithelial cells from the inner pole of the lens vesicle (see Fig. 13.3). These cells become the fibers of the lens nucleus (Fig. 13.8). The remaining lens fibers arise from the transformation of the cuboidal cells of the anterior lens epithelium. During embryonic life, mitotic activity is spread throughout the outer lens epithelial cells. Around the time of birth, mitotic activity ceases in the central region of this epithelium, thus leaving a germinative ring of mitotically active cells surrounding the central region. Daughter cells from the germinative region move into the equatorial region of cellular elongation, where they cease to divide and take on the cytological characteristics of RNA-producing cells and begin to form crystallin mRNAs. These cells soon elongate tremendously, fill up with crystallins, and transform into secondary lens fibers that form concentric layers around the primary fibers of the lens nucleus. The midline region where secondary lens fibers from opposite points on the equator join is recognized as the anterior and posterior lens suture (see Fig. 13.7D). With this arrangement, the lens fibers toward the periphery are successively younger. As long as the lens grows, new secondary fibers move in from the equator onto the outer cortex of the lens.
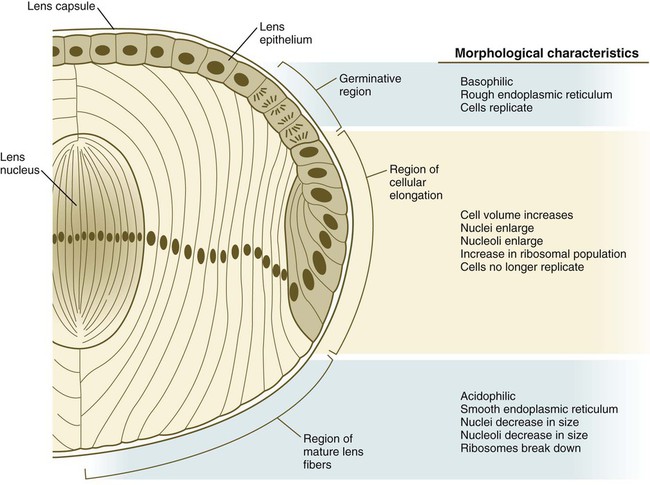
As the lens grows, epithelial cells from the germinative region stop dividing, elongate, and differentiate into lens fiber cells that produce lens crystallin proteins. (From Papaconstantinou J: Science 156:338-346, 1967.)
Throughout much of its life, the lens is under the influence of the retina. After induction of the lens, secretions of the retina, of which FGF is a major component, accumulate in the vitreous humor behind the lens and stimulate the formation of lens fibers. A striking example of the continued influence of the retina on lens morphology is seen after a developing lens is rotated so that its outer pole faces the retina. Very rapidly, under the influence of retinal secretions, the low epithelial cells of the former outer pole begin to elongate and form an additional set of lens fibers (Fig. 13.9). A new lens epithelium forms on the corneal side of the rotated lens. Such structural adaptations are striking evidence of a mechanism that ensures correct alignment between the lens and the rest of the visual system throughout development.
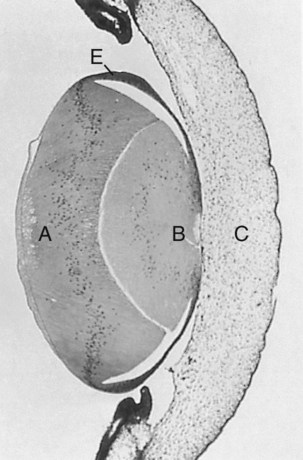
At 5 days, the lens was surgically reversed so that the anterior epithelial cells (E) faced the vitreous body and retina. The formerly low epithelial cells elongated to form new lens fibers (A). Because of the reversal of the polarity of the equatorial zone of the lens, new epithelial cells were added over the original mass of lens fibers (B) onto the corneal face of the reversed lens. C indicates cornea. (From Coulombre JL, Coulombre AJ: Science 142:1489-1490, 1963.)
Formation of the Cornea
Formation of the cornea is the result of the last of the series of major inductive events in eye formation (see Fig. 13.4), with the lens vesicle acting on the overlying surface ectoderm. This induction results in the transformation of a typical surface ectoderm, consisting of a basal layer of cuboidal cells and a superficial periderm, to a transparent, multilayered structure with a complex extracellular matrix and cellular contributions from several sources. In keeping with its multifaceted role at almost all stages of eye development, Pax-6 expression in the surface ectoderm is a requirement for corneal induction.
The inductive influence of the lens stimulates a change in the basal ectodermal cells. These cells increase in height, largely as a result of the elaboration of secretory organelles (e.g., Golgi apparatus) on the basal ends of the cells. As these changes are completed, the cells begin to secrete epithelially derived collagen types I, II, and IX to form the primary stroma of the cornea (Fig. 13.10).
After the corneal endothelium has formed a continuous layer, its cells synthesize large amounts of hyaluronic acid and secrete it into the primary stroma. Because of its pronounced water-binding capacities, hyaluronic acid causes the primary stroma to swell greatly. This provides a proper substrate for the second wave of cellular migration into the developing cornea (Fig. 13.11). These cells, also of neural crest origin, are fibroblastic. They migrate and proliferate in the hyaluronate-rich spaces between layers of collagen in the primary corneal stroma. The migratory phase of cellular seeding of the primary corneal stroma ceases when these cells begin to produce large amounts of hyaluronidase, which breaks down much of the hyaluronic acid in the primary stroma. In other parts of the embryo (e.g., limb bud), there is also a close correlation between high amounts of hyaluronic acid and cellular migration and a cessation of migration with its removal. With the removal of hyaluronic acid, the cornea decreases in thickness. When the migratory fibroblasts have settled, the primary corneal stroma is considered to have been transformed into the secondary stroma.
The fibroblasts of the secondary stroma contribute to its organization by secreting coarse collagen fibers to the stromal matrix. Nevertheless, prominent layers of acellular matrix continue to be secreted by epithelial and endothelial cells of the cornea. These secretions provide the remaining layers that constitute the mature cornea. Listed from outside in, they are (1) the outer epithelium, (2) Bowman’s membrane, (3) the secondary stroma, (4) Descemet’s membrane, and (5) the corneal endothelium (see Fig. 13.10).
Retina and Other Derivatives of the Optic Cup
While the lens and cornea are taking shape, profound changes are also occurring in the optic cup (see Fig. 13.3). The inner layer of the optic cup thickens, and the epithelial cells begin a long process of differentiation into neurons and light receptor cells of the neural retina. The outer layer of the optic cup remains thin and ultimately becomes transformed into the retinal pigment epithelium (see Fig. 13.7). Cells of the retinal pigment epithelium do not differentiate into neurons during normal embryogenesis, but in postnatal life some of these cells maintain stem cell properties and can differentiate into multiple cell types. At the same time, the outer lips of the optic cup undergo a quite different transformation into the iris and ciliary body, which are involved in controlling the amount of light that enters the eye (iris) and the curvature of the lens (ciliary body).
The neural retina is a multilayered structure; its embryonic development can be appreciated only after its adult organization is understood (Fig. 13.12). When seen in cross section under a microscope, the neural retina consists of alternating light-staining and dense-staining strips that correspond to layers rich in nuclei or cell processes. The direct sensory pathway in the neural retina is a chain of three neurons that traverse the thickness of the retina. The first element of the chain is the light receptor cell, either a rod or a cone. A light ray that enters the eye passes through the entire thickness of the neural retina until it impinges on the outer segment of a rod or cone cell (photoreceptor) in the extreme outer layer of the retina. The nucleus of the stimulated rod and cone cell is located in the outer nuclear layer. The photoreceptor cell sends a process toward the outer plexiform layer, where it synapses with a process from a bipolar cell located in the inner nuclear layer. The other process from the bipolar neuron leads into the internal plexiform layer and synapses with the third neuron in the chain, the ganglion cell. The bodies of the ganglion cells, which are located in the ganglion cell layer, send out long processes that course through the innermost nerve fiber layer toward their exit site from the eye, the optic nerve, through which they reach the brain.
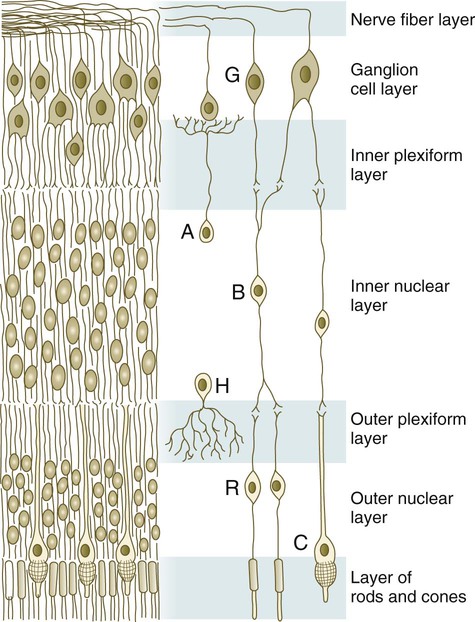
A, amacrine cell; B, bipolar cell; C, cone; G, ganglion cell; H, horizontal cell; R, rod.
If all light signals were processed only through the simple three-link series of neurons in the retina, visual acuity would be much less than it actually is. Many levels of integration have occurred by the time a visual pattern is stored in the visual cortex of the brain. The first is in the neural retina. At synaptic sites in the inner and the outer plexiform layers of the retina, other cells, such as horizontal and amacrine cells (see Fig. 13.12), are involved in the horizontal redistribution of the simple visual signal. This redistribution facilitates the integration of components of a visual pattern. Another prominent cell type in the retina is the Müller glial cell, which sends processes to almost all layers of the retina and seems to play a role similar to that of astrocytes in the central nervous system.
Neural Retina
From the original columnar epithelium of the inner sensory layer of the optic cup (see Fig. 13.7), the primordium of the neural retina takes on the form of a mitotically active, thickened pseudostratified columnar epithelium organized in a manner similar to that of the early neural tube. During the early stages of development of the retina, its polarity becomes fixed according to the same axial sequence as that seen in the limbs (see Chapter 10). The nasotemporal (anteroposterior) axis is fixed first; this is followed by fixation of the dorsoventral axis. Finally, radial polarity is established.
Dorsoventral patterning is initiated through the presence of BMP-4 dorsally and shh ventrally (Fig. 13.13A). The presence of shh ventrally stimulates the production of Otx-2 in the outer layer of the optic vesicle and differentiation of the retinal pigment epithelium. Within the inner layer of the optic vesicle, shh and a protein, ventroptin, both antagonists of BMP-4, stimulate the expression of the transcription factors Vax-2 and Pax-2 in the ventral retina. In the dorsal part of the future retina, BMP-4 signals the expression of Tbx-5, the transcription factor involved in the formation of the forelimb (see p. 193). Although many molecules are unequally distributed along the two axes of the retina, opposing gradients of specific ephrins and their receptors are heavily involved in the characterization of the retinal axes (see Fig. 13.13A).
As the number of cells in the early retina increases, the differentiation of cell types begins. There are two major gradients of differentiation in the retina. The first proceeds roughly vertically from the inner to the outer layers of the retina. The second moves horizontally from the center toward the periphery of the retina (Fig. 13.13B).
Evidence obtained on fish retinas suggests that the horizontal gradient of neurogenesis may be driven by an initial point source of shh in a manner similar to a better-understood process that occurs in Drosophila. Differentiation in the horizontal gradient begins with the appearance of ganglion cells and the early definition of the ganglion cell layer (Fig. 13.14). As the ganglion cells differentiate, the surrounding cells are prevented from premature differentiation by the activity of the Notch gene. A major function of Notch is to maintain populations of cells in the nondifferentiated state until the appropriate local cues for their further differentiation appear. With the later differentiation of the horizontal and amacrine cells, the inner and outer nuclear layers take shape. As the cells within the nuclear layers send out processes, the inner and outer plexiform layers become better defined. The bipolar neurons and cone cells differentiate last, thus completing the first gradient.
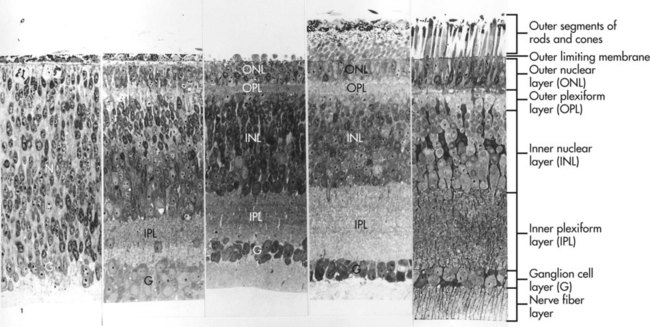
At the far left, the ganglion cell layer begins to take shape from the broad neuroblast layer (N). With time, further layers take shape until all layers of the retina are represented (far right). (From Sheffield JB, Fischman DA: Z Zellforsch Mikrosk Anat 104:405-418, 1970.)
Cell lineage experiments involving the use of retroviral or other tracers (e.g., horseradish peroxidase) introduced into neuronal precursors in the early retina have revealed two significant cellular features of retinal differentiation. First, progeny of a single labeled cell are distributed in a remarkably straight radial pattern following the vertical axis of retinal differentiation. There seems to be little lateral mixing among columns of retinal cells (Fig. 13.15). The second cellular feature of retinal differentiation is that a single labeled precursor cell can give rise to more than one type of differentiated retinal cell.
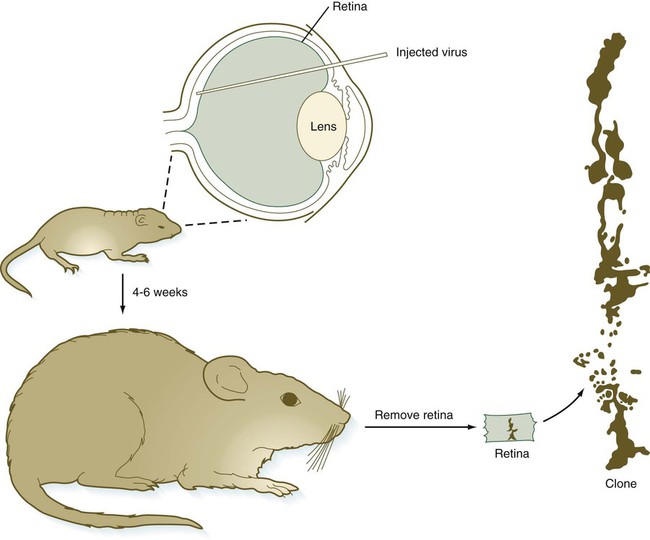
Top, Injection of a retroviral vector that includes the gene for β-galactosidase into the space between the neural and pigmented retinal layers. About 4 to 6 weeks later, the retinas were removed, fixed, and histochemically reacted for β-galactosidase activity. The drawing at the right illustrates a vertical clone of cells derived from a virally infected precursor cell. Several cell types (rods, a bipolar cell, and a Müller glial cell) constituted this clone. (Adapted from Turner DL, Cepko CL: Nature 328:131-136, 1987.)
Iris and Ciliary Body
Mediated by a still poorly defined influence of the lens, differentiation of the iris and ciliary body occurs at the lip of the optic cup, where the developing neural and pigmented retinal layers meet. Rather than being sensory in function, these structures are involved in modulating the amount and character of light that ultimately impinges on the retina. In addition, the ciliary body is the source of the aqueous humor that fills the anterior chamber of the eye. The iris partially encircles the outer part of the lens, and through contraction or relaxation, it controls the amount of light passing through the lens. The iris contains an inner unpigmented epithelial layer and an outer pigmented layer, which are continuous with the neural and pigmented layers of the retina (Fig. 13.16). The stroma of the iris, which is superficial to the outer pigmented layer of the iris, is of neural crest origin and secondarily migrates into the iris. Within the stroma of the iris lie the primordia of the sphincter pupillae and dilator pupillae muscles. These muscles are unusual because they are of neurectodermal origin; they arise from the anterior epithelial layer of the iris through the transdifferentiation of pigmented cells into smooth muscle.
The ciliary body of the eye secretes aqueous humor into the posterior chamber of the eye. The fluid passes in front of the lens through the pupil into the anterior chamber, where it maintains outward pressure on the cornea. It is then resorbed through a trabecular meshwork and into the canal of Schlemm in the angle of the eye (see Fig. 13.16). This outflow area arises from the organization of neural crest cells into a trabecular meshwork of lamellae covered by flat endotheliumlike cells, which abut onto an enlarged venous sinus, designated the canal of Schlemm. Development of this network in humans is not completed until around the time of birth.
Vitreous Body and Hyaloid Artery System
During much of embryonic development, the vitreous body is supplied by the hyaloid artery and its branches (Fig. 13.17). The hyaloid artery enters the eyeball through the choroid fissure of the optic stalk (see Fig. 13.6), passes through the retina and vitreous body, and terminates in branches to the posterior wall of the lens. As development of the retinal vasculature progresses, the portions of the hyaloid artery (and its branches supplying the lens) in the vitreous body regress through apoptosis of their endothelial cells and leave a hyaloid canal. The more proximal part of the hyaloid arterial system persists as the central artery of the retina and its branches.
Choroid Coat and Sclera
Outside the optic cup lies a layer of mesenchymal cells, largely of neural crest origin. Reacting to an inductive influence from the pigment epithelium of the retina, these cells differentiate into structures that provide vascular and mechanical support for the eye. The innermost cells of this layer differentiate into a highly vascular tunic called the choroid coat (see Fig. 13.7C), and the outermost cells form a white, densely collagenous covering known as the sclera. The opaque sclera, which serves as a tough outer coating of the eye, is continuous with the cornea. The extraocular muscles, which are derived from cranial mesoderm and provide gross movements to the eyeball, attach to the sclera.
Eyelids and Lacrimal Glands
The eyelids first become apparent during the seventh week as folds of skin that grow over the cornea (Fig. 13.18A; see Fig. 13.7). When their formation has begun, the eyelids rapidly grow over the eye until they meet and fuse with one another by the end of the ninth week (Fig. 13.18B; see Fig. 18.4). Many growth factors are involved in the migration of the epidermal cells across the cornea. Mutants of these result in lack of eyelid fusion in the embryo and impaired epidermal wound healing postnatally. The temporary fusion involves only the epithelial layers of the eyelids, thus resulting in a persistent epithelial lamina between them. Before the eyelids reopen, eyelashes and the small glands that lie along the margins of the lids begin to differentiate from the common epithelial lamina. Although signs of loosening of the epithelial union of the lids can be seen in the sixth month, BMP-mediated reopening of the eyelids normally does not occur until well into the seventh month of pregnancy.
The space between the front of the eyeball and the eyelids is known as the conjunctival sac. Multiple epithelial buds grow from the lateral surface ectoderm at about the time when the eyelids fuse. These buds differentiate into the lacrimal glands, which produce a watery secretion that bathes the outer surface of the cornea when mature. This secretion ultimately passes into the nasal chamber by way of the nasolacrimal duct (see Chapter 14). The lacrimal glands are not fully mature at birth, and newborns typically do not produce tears when crying. The glands begin to function in lacrimation at about 6 weeks.
Despite the many types of malformations of the eye and visual system, the incidence of most individual types of defects is uncommon. Mutations of almost any of the genes mentioned in this section result in a recognizable malformation of the eye. Among these, anophthalmia, microphthalmia, congenital cataracts, and colobomas are common phenotypes. Examples of a few of the many ocular malformations are given in Clinical Correlation 13.1.
Ear
Development of the Inner Ear
Development of the ear begins with preliminary inductions of the surface ectoderm, first by the notochord (chordamesoderm) and then by the paraxial mesoderm (Fig. 13.20). These inductions prepare the ectoderm for a third induction, in which FGF-3 signals from the rhombencephalon induce the adjacent surface ectoderm to express Pax-2. Then Wnt signals greater than a certain threshold stimulate Pax-2–positive cells to form both the otic and epibranchial placodes (Fig. 13.21). The cells exposed to a subthreshold concentration of Wnt are destined to become epidermal cells. Late in the fourth week, possibly under the influence of FGF-3 secreted by the adjacent rhombencephalon, the otic placode invaginates and then separates from the surface ectoderm to form the otic vesicle, or otocyst (see Fig. 13.21C). Even at its earliest stages, the development of the main components of the inner ear is under separate genetic control: Pax-2 and Sox-3 for the auditory portion (cochlea) and Nkx-5 for the vestibular portion (semicircular canals).
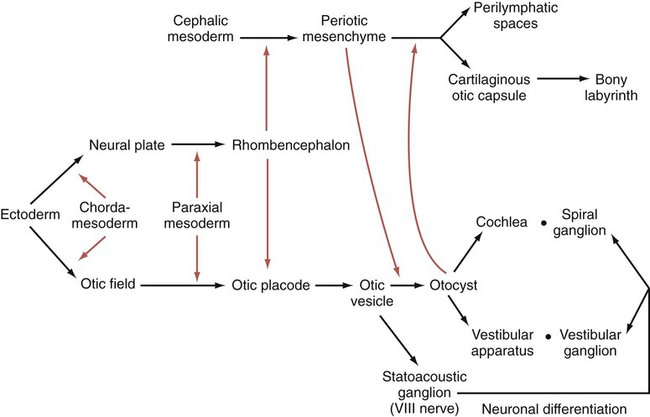
Colored arrows refer to inductive events. (Based on McPhee JR, van de Water TR. In Jahn AF, Santos-Sacchi J, eds: Physiology of the ear, New York, 1988, Raven, pp 221-242.)
The otic vesicle soon begins to elongate, thus forming a dorsal vestibular region and a ventral cochlear region (Fig. 13.22A). The paired box gene, Pax2, is heavily involved in early development of the otic vesicle. Expression of Pax-2 in the ventral otic vesicle is important for the continued development of the endolymphatic duct and the cochlear apparatus, whereas dorsal expression of Dlx-5 and Gbx-2 is important for development of the vestibular system. Quite early, the endolymphatic duct arises as a short, fingerlike projection from the dorsomedial surface of the otocyst (Fig. 13.22B). FGF-3, secreted by rhombomeres 5 and 6, is necessary for normal development of the endolymphatic duct. At about 5 weeks, the appearance of two ridges in the vestibular portion of the otocyst foreshadows the formation of two of the semicircular ducts (Fig. 13.22C).
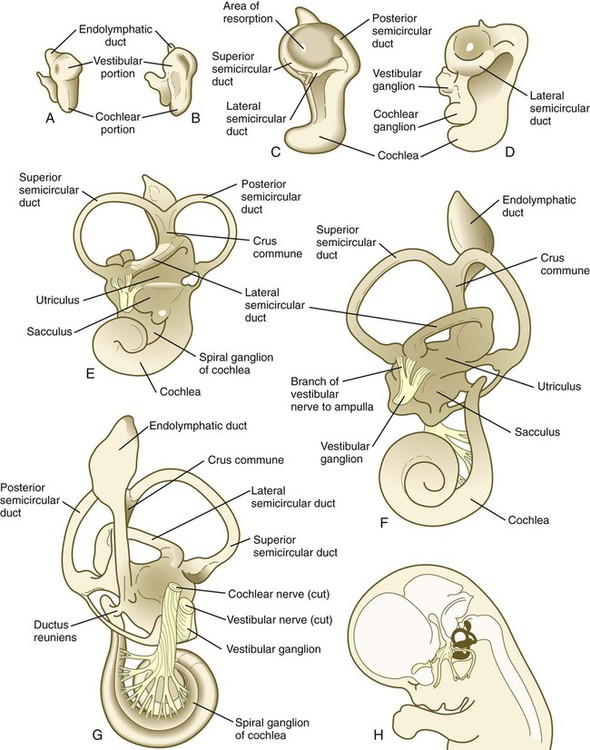
A, At 28 days. B, At 33 days. C, At 38 days. D, At 41 days. E, At 50 days. F, At 56 days, lateral view. G, At 56 days, medial view. H, Central reference drawing at 56 days. (From Carlson B: Patten’s foundations of embryology, ed 6, New York, 1996, McGraw-Hill.)
As the ridges expand laterally, their opposing epithelial walls approximate each other, to form a fusion plate. Programmed cell death in the central area of epithelial fusion or epithelial cell migration away from this area converts the flangelike structures to canals by setting up a zone of resorption (see Fig. 13.22C). The epithelial precursors of the semicircular canals express the homeobox transcription factor gene Nkx-5-1, which is important for the development of the dorsal vestibular portion of the inner ear. Other transcription factors play a role in the formation of individual semicircular canals. In the absence of Otx1, the lateral semicircular canal fails to form, and the homeobox transcription factor Dlx-5 must be expressed for the anterior and posterior canals to develop. The cochlear part of the otocyst begins to elongate in a spiral; it attains one complete revolution at 8 weeks and two revolutions by 10 weeks (see Fig. 13.22C through F). The last half turn of the cochlear spiral (a total of two and one-half turns) is not completed until 25 weeks.
The inner ear (membranous labyrinth) is encased in a capsule of skeletal tissue that begins as a condensation of mesenchyme around the developing otocyst at 6 weeks’ gestation. The process of encasement of the otocyst begins with an induction of the surrounding mesenchyme by the epithelium of the otocyst (see Fig. 13.20). This induction, involving BMP-4, stimulates the mesenchymal cells, mainly of mesodermal origin, to form a cartilaginous matrix (starting at about 8 weeks). The capsular cartilage serves as a template for the later formation of the true bony labyrinth. The conversion from the cartilaginous to the bony labyrinth occurs between 16 and 23 weeks’ gestation.
The sensory neurons that compose the eighth cranial nerve (specifically the statoacoustic ganglion) arise from cells that migrate out from a portion of the medial wall of the otocyst (see Fig. 13.21). How the axons originating from the statoacoustic ganglion make their way to precise locations within the inner ear is not well understood, but guidance by neural crest cells and channeling by Slit-Robo interactions are known to play prominent roles in directing growth cones to their proper targets. The cochlear part (spiral ganglion) of cranial nerve VIII fans out in close association with the sensory cells (collectively known as the organ of Corti) that develop within the cochlea. Neural crest cells invade the developing statoacoustic ganglion and ultimately form the satellite and supporting cells within it.
The sensory cells of the organ of Corti are also derived from the epithelium of the otocyst. They undergo a very complex pattern of differentiation (Fig. 13.23). The generation of sensory neuroblast precursors in the inner ear seems to use the Notch pathway to control the proportion of epithelial cells that differentiate into neuroblasts versus supporting cells in a manner similar to that described for the differentiation of ganglion cells in the early retina (see p. 280). As in other sensory systems, highly regulated developmental controls ensure precise matching between sensory cells designed to receive sound waves at different frequencies or gravitational information and the neurons that transmit the signals to the brain. Formation of the graded array of hair cells that respond to different frequencies of sound is to a great extent based on execution of the planar cell polarity pathway (see p. 87).
Development of the Middle Ear
Formation of the middle ear is intimately associated with developmental changes in the first and second pharyngeal arches (see Chapter 14). The middle ear cavity and the auditory tube arise from an expansion of the first pharyngeal pouch called the tubotympanic sulcus (Fig. 13.24). Such an origin ensures that the entire middle ear cavity and auditory tube are lined with an endodermally derived epithelium.
The middle ear ossicles themselves have a dual origin. According to comparative anatomical evidence, the malleus and incus arise from neural crest–derived mesenchyme of the first pharyngeal arch, whereas the stapes originates from second-arch mesenchyme (Fig. 13.25).
Development of the External Ear
The external ear (pinna) is derived from mesenchymal tissue of the first and second pharyngeal arches that flank the first (hyomandibular) pharyngeal cleft. During the second month, three nodular masses of mesenchyme (auricular hillocks) take shape along each side of the first pharyngeal cleft (Figs. 13.26 and 13.27). The auricular hillocks enlarge asymmetrically and ultimately coalesce to form a recognizable external ear. During its formation, the pinna shifts from the base of the neck to its normal adult location on the side of the head. Because of its intimate association with the pharyngeal arches and its complex origin, the external ear is a sensitive indicator of abnormal development in the pharyngeal region. Other anomalies of the first and second arches are often attended by misshapen or abnormally located external ears.
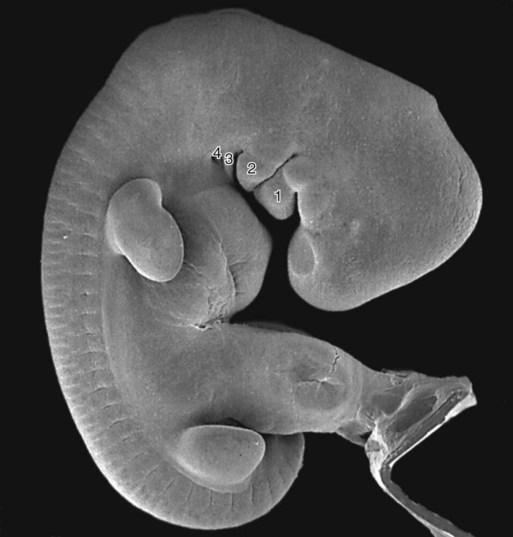
The external auditory meatus takes shape during the end of the second month by an inward expansion of the first pharyngeal cleft. Early in the third month, the ectodermal epithelium of the forming meatus proliferates and forms a solid mass of epithelial cells called the meatal plug (see Fig. 13.24C). Late during the fetal period (at 28 weeks), a channel within the meatal plug extends the existing external auditory meatus to the level of the tympanic membrane.
The external ear and external auditory meatus are very sensitive to drugs. Exposure to agents such as streptomycin, thalidomide, and salicylates during the first trimester can cause agenesis or atresia of both these structures. Congenital malformations of the ear are discussed in Clinical Correlation 13.2.
Summary
The eye begins as an outpocketing (optic groove) of the lateral wall of the diencephalon. The optic grooves enlarge to form the optic vesicles, which induce the overlying ectoderm to form the lens primordium. The optic stalk, which connects the optic cup to the diencephalon, forms a groove containing the hyaloid artery, which supplies the developing eye. The paired box gene Pax-6 acts as a master control gene for eye development.
Under the influence of Pax-6, the lens forms from an ectodermal thickening that invaginates to form a lens vesicle. The cells of the inner wall of the lens vesicle elongate and synthesize lens-specific crystallin proteins. In the growing lens, the inner epithelium forms a spherical mass of banana-shaped lens fibers (epithelial cells). The anterior lens epithelium consists of cuboidal epithelial cells. The overall polarity of the lens is under the influence of the retina.
The cornea is formed through induction of the surface ectoderm by the lens. After induction, the basal ectodermal cells secrete an extracellular matrix that serves as a substrate for the migration of neural crest cells forming the corneal endothelial layer. The corneal endothelial cells secrete large amounts of hyaluronic acid into the early cornea. This permits the migration of a second wave of neural crest cells into the cornea. These fibroblastlike cells secrete collagen fibers into the coarse corneal stroma matrix. Under the influence of thyroxine, water is removed from the corneal matrix, and it becomes transparent.
The neural retina differentiates from the inner layer of the optic cup. The outer layer forms the pigment layer of the retina. The neural retina is a complex multilayered structure with three layers of neurons connected by cellular processes. Cellular differentiation in the neural retina follows vertical and horizontal gradients. Under the influence of Pax-2, cell processes grow from retinal neurons through the optic stalk to make connections with optic centers within the brain.
The iris and ciliary body form from the outer edge of the optic cup. Sphincter and dilator pupillae muscles form within the iris. Eye color is related to the levels and distribution of pigmentation within the iris. Outside the optic cup, mesenchyme differentiates into a vascular choroid coat and a tough collagenous sclera. Eyelids begin as folds of skin that grow over the cornea and then fuse, closing off the eyes. The eyelids reopen late in the seventh month.
The developing eyes are sensitive to certain teratogens and intrauterine infections. Exposure to these can cause microphthalmia or congenital cataracts. Nonclosure of the choroid fissure results in coloboma.
The inner ear arises by an induction of the surface ectoderm by the developing hindbrain. Steps in its formation include ectodermal thickening (placode), invagination to form an otic vesicle, and, later, growth and morphogenesis into auditory (cochlea) and vestibular (semicircular canals) portions. Normal development of the cochlea depends on the proper expression of Pax-2, whereas Nkx-5 is required for the formation of the semicircular canals.
Development of the middle ear is associated with the first pharyngeal cleft and the arches on either side. Middle ear ossicles and associated muscles take shape within the middle ear cavity.
The external ear arises from six modular masses of mesenchyme that take shape in the pharyngeal arch tissue surrounding the first pharyngeal cleft.
Congenital deafness can occur after certain intrauterine disturbances, such as rubella infection. Structural anomalies of the external ear are common.