Chapter 6 Pharmacogenetics
The Promise of Personalized Medicine


Pharmacogenetics and Pharmacogenomics

One can consider pharmacogenetics to be the original term for this field, coined in the days when tools such as microarrays did not exist and the human genome had not been mapped. In those early days it seemed unlikely or impossible that an entire genome could be analyzed in an efficient enough manner to become a useful tool in everyday medicine. Now that we have the means and the knowledge to envision a genome-wide approach to everyday prescribing, the term pharmacogenomics is often used to refer to this field. Table 6-1 lists some of the terms commonly used in pharmacogenetics.
TABLE 6-1 Definitions of Some Common Terms Used in Pharmacogenetics
Gene | A sequence of nucleotides that corresponds to a sequence of amino acids in an entire protein or part of a protein. Genes are typically found at a specific location on a chromosome. |
Genome | The full genetic complement of an individual. |
Allele | Any of the alternative forms of a gene at a particular locus. These alternative forms may or may not result in different phenotypes. |
Null allele | A mutation in a gene that leads to a loss of function. Either the gene is not expressed at all (i.e., no protein or RNA) or the product is not functional. |
Polymorphism | Variation in DNA sequence present at a specific locus within a population. |
Genotype | The genetic makeup of an individual. |
Phenotype | The observable physical or biochemical characteristics of an organism. Determined by genotype and environment. |
Monogenic | Related to or controlled by a single gene |
Polygenic | Related to or controlled by multiple genes. A polygenic trait is a phenotype that is determined by multiple genes rather than a single gene (monogenic). |
Germ line | Cellular lineage; genetic information that is passed from one generation to the next. |
Haplotype | A group of alleles of different genes on a single chromosome that are so closely linked that they are inherited as a unit. |
Somatic cell | Any cell in the body, with the exception of those involved in reproduction. |
The most common basis for genetic variation, and thus the basis for a pharmacogenomic approach to drug therapy, is the single nucleotide polymorphism, or SNP (Figure 6-1). An SNP occurs when a single nucleotide is exchanged for another at a point in an individual’s genome. It is estimated that the human genome consists of approximately 3 billion nucleotides, which in specific combinations form 25,000 to 40,000 genes and encode approximately 100,000 proteins (at last count). SNPs that occur in coding regions of the genome have the potential to influence protein expression by altering an amino acid within the protein.


Pharmacokinetics



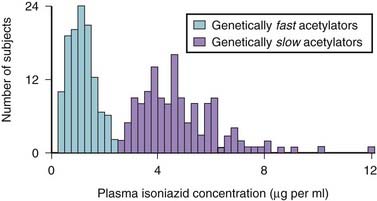
Figure 6-2 Influence of genetics on isoniazid metabolism.
(Modified from Meyer UA: Pharmacogenetics: five decades of therapeutic lessons from genetic diversity, Nat Rev Genet 2004 5:669, 2004. Reprinted by permission from Macmillan Publishers.)
Phase I Reactions, CYP450, and Genetic Polymorphisms


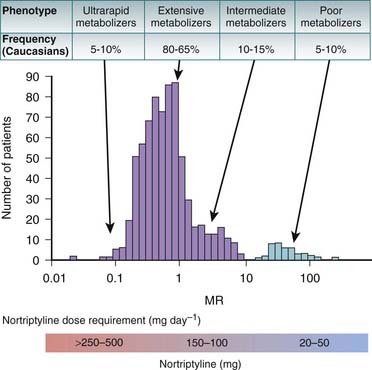
Figure 6-3 Influence of phenotype on dosing.
(Modified from Meyer UA: Pharmacogenetics: five decades of therapeutic lessons from genetic diversity, Nat Rev Genet 2004 5:669, 2004. Reprinted by permission from Macmillan Publishers.)
CYP2D6



CYP3A4
CYP2C9


CYP2C19

CYP1A2


Pharmacodynamics





The Next Step: from Genetics to Genomics




Detection of Genetic Polymorphisms
A microarray is a collection of immobilized single-stranded DNA fragments that contain a known nucleotide sequence that is used to identify and sequence DNA samples (Figure 6-4). Microarrays can be used in the analysis of gene expression. A microarray provides an automated means for identifying genes in a given sample. For example, cancerous and healthy cells may be analyzed from a single patient in order to determine the differences in gene expression between these two types of cells.





Limitations of Pharmacogenomics



Resources for Pharmacogenetic Information
The U.S. National Institutes of Health (NIH) funds a Pharmacogenetics and Pharmacogenomics Knowledge Base, which is available at www.pharmgkb.org. The site offers pharmacogenetic data categorized by genes, pathways (e.g., renin-angiotensin-aldosterone system), SNPs, and drugs.