Chapter 75 Non-accidental Head Trauma
Inflicted head injury is a form of traumatic brain injury resulting from assault of typically, but not exclusively, infants and young children of less than 3 years of age. Despite considerable prevention efforts, it remains a major cause of morbidity and mortality among children [Aldrich et al., 1992; Bonnier et al., 2003; Chiesa and Duhaime, 2009; Gilles and Nelson, 1998]. Infants with inflicted head injuries have the most severe illness, the highest mortality risk, and the worst outcome in comparison with other forms of childhood trauma [Vavilala et al., 2007; Irazuzta et al., 1997; Pfenninger and Santi, 2002; Rivara et al., 1996]. They also have the greatest utilization (and therefore cost) of health-care resources in children with traumatic brain injury [Libby et al., 2003].
The National Child Abuse and Neglect Data System reported 1760 child fatalities in 2007, a rate of 2.35 deaths/100,000 children. Of these, physical abuse or multiple forms of maltreatment constituted over 60 percent of fatalities. Infant boys less than 1 year of age had a death rate of 18.85 deaths per 100,000 same-aged infant boys, while infant girls had a rate of 15.39 deaths per 100,000 same-aged infant girls. Forty-two percent were infants younger than 1 year, while 75 percent were less than 4 years old [http://www.childwelfare.gov/pubs/factsheets/fatality.pdf; http://www.acf.hhs.gov/programs/cb/pubs/cm07/cm07.pdf]. In one study, the incidence of inflicted head injury among children younger than 2 years was 17 per 100,000 children [Keenan et al., 2003]. Other studies suggest that as many as 60 percent of deaths resulting from abuse or neglect are not recorded [Crume et al., 2002].
Historical Perspective
The acceptance of inflicted head injury as an age-related subtype of traumatic brain injury and a type of physical abuse is a relatively recent phenomenon, although descriptions of this disorder were made at least as early as the 19th century [Tardieu, 1860]. Tardieu was the first to document the range of injury patterns found in abused and neglected children, as distinct from those in adults, and to link them to caregiver actions. Starting in the 1920s, fractures, subdural hematomas, and retinal hemorrhages in infants were associated with trauma, but correlating these findings with caregiver actions was not done clearly until the late 1940s and 1950s [Caffey, 1946, 1972, 1974; Silverman, 1952; Guthkelch, 1971; Frauenberger and Lis, 1950; Kempe et al., 1962; Lindenberg and Freytag, 1969; Woolley and Evans, 1955].
It was not until the 1960s and 1970s that the terms “battered child syndrome” and “shaken-baby syndrome” were first employed, and additional correlation made with specific findings and etiology [Kempe et al., 1962; Lindenberg and Freytag, 1969; Guthkelch, 1971; Caffey, 1972; Caffey, 1974]. The term “battered child syndrome” was coined by John Kempe to describe severe physical abuse with fractures, bruises, failure to thrive, brain injury, and subdural hematomas [Kempe et al., 1962]. Subsequently, Guthkelch and Caffey independently hypothesized that head injury in the absence of obvious blunt force trauma was caused when a caregiver held the infant around the chest and “whiplashed” the head by shaking the baby (as some infants had associated rib fractures) [Guthkelch, 1971; Caffey, 1972, 1974]. Rotational movement of the head on the neck can, of course, be caused by a number of ways, not just shaking. Also in 1974, Caffey first used the term “shaken baby syndrome” for infants with severe head injuries but lacking signs of blunt force trauma on imaging studies. A number of pathologic studies have since confirmed that many infants without obvious signs of blunt force trauma antemortem frequently show evidence of impact trauma postmortem, and not infrequently with involvement at many impact sites [Duhaime et al., 1987; Hicks and Gaughan, 1995; Gill et al., 2009].
An important first step in accepting inflicted neurotrauma as a distinct form of trauma was recognizing that the history offered by the caregiver was biomechanically inconsistent with the severity of injury [Duhaime et al., 1987, 1992; Billmire and Myers, 1985; Goldstein et al., 1993; Weston, 1973]. Few authors have specifically addressed the discrepancies between initial historical data and subsequent caregiver admissions [Gilles and Nelson, 1998; Tardieu, 1860; Weston, 1973; Starling et al., 2004; Reece and Sege, 2000; Krugman, 1983].
Over the past three decades, our knowledge of inflicted childhood neurotrauma has increased significantly [Chiesa and Duhaime, 2009; Christian and Block, 2009]. It is important to realize that the majority of the relevant literature is observational and retrospective in nature. Many studies have failed to specify explicit inclusion or exclusion criteria, and have accepted, with minimal or no question, histories obtained from perpetrators. While prospective studies continue to be in the minority due to the inherent difficulties in defining abusive injury, both clinical and laboratory investigations increasingly are examining the unique nature of early life inflicted injury.
Terminology
It is recommended to use the terms inflicted, nonaccidental, or abusive head injury when referring to neurotrauma from assault in children [Christian and Block, 2009]. These are inclusive terms covering the range of craniospinal injuries sustained by infants and young children as a consequence of violent assault by parents or other caretakers. Unintentional, or accidental, head injury refers to injury sustained by chance and is unrelated to specific deliberate actions of caretakers.
There are a number of problems with using lay terminology, such as shaken baby syndrome and shaken-impact infant syndrome. These terms imply a uniform injury event sequence, mechanism of injury, and age at time of injury. The actual injury and postinjury event sequences are often much more complex [Gilles and Nelson, 1998; Duhaime et al., 1987; Alexander et al., 1990; Budenz et al., 1994; Pollanen et al., 2002].
Developmental Differences Predisposing the Immature Neuraxis to Injury
Structural and functional changes of central and peripheral nervous system organization and function are greatest during the first 2 years of life, when nonaccidental head injury occurs most frequently [Bauer and Fritz, 2004; Johnston, 1995; Robertson et al., 2006; Gilmore et al., 2007; Vexler et al., 2006; Law et al., 2003; Rice and Barone, 2000; Pouwels et al., 1999; Brody et al., 1987]. A number of biologic and mechanical factors predispose the infant and young child to injury of the craniospinal axis (Box 75-1). Of these, the most important are biomechanical factors and differences in postinjury response cascades.
Box 75-1 Biologic and Mechanical Features Predisposing the Infant to Head Injury
Mechanical Factors
The infant is more biomechanically vulnerable to significant applied forces than the older child and adult due to a larger head to body ratio and less developed neck and shoulder girdle musculature. The higher water content of the brain, coupled with incomplete myelination, contributes to distensibility and compliance of brain tissue during injury events and predisposes the infant to greater shear strains with angular and translational acceleration [Bauer and Fritz, 2004; Brody et al., 1987; Prange et al., 2003]. On the other hand, the relatively smooth inner cranial table and greater skull compliance decrease risk for contusion, as well as dispersing some force into the skull with impact [Lindenberg and Freytag, 1969; Carter and McCormick, 1983; Courville, 1965; Calder et al., 1984; Jaspan et al., 1992]. These features reinforce the observation that trivial trauma does not appear sufficient to cause the kinds of injuries seen in the context of abuse [Brody et al., 1987; Prange et al., 2003].
Biologic Factors
There are numerous age-related biochemical, microscopic, and macroscopic age-dependent differences in the immature versus mature central nervous system (CNS) [Bauer and Fritz, 2004; Pouwels et al., 1999]. Synaptic density in human infants is 50–60 percent above the adult mean [Novack et al., 1996; Teasdale and Graham, 1998]. Neuronal synapses and receptors differ in structure, number, and function [Azzarelli et al., 1996; Herlenius and Lagercrantz, 2004]. Among the many unique biochemical features of the developing brain are greater potential to excitability due to increased excitatory amino acids, and robust neuronal and synaptic networks [Herlenius and Lagercrantz, 2004; Johnston, 1996; Kubova and Moshe, 1994; McDonald et al., 1992; Wasterlain et al., 2002].
Responses to Injury
Injury and repair after a CNS insult are quite different in the immature versus the mature CNS. The biochemical and physiological responses to injury of the immature nervous system are unique and may render the infant more vulnerable to injury [Zupanc, 2009; Giza et al., 2007; Bauer and Fritz, 2004; Bittigau et al., 2003]. The immature CNS has decreased ischemic and seizure thresholds, especially after trauma and hypoxic-ischemic injury [Goldstein et al., 1993, 1998; Johnston, 1995; Kubova and Moshe, 1994; Adelson et al., 1997; Hagberg, 2004; Ruppel et al., 2002; Chiaretti et al., 2009; Lea and Faden, 2001; Satchell et al., 2005; Robertson et al., 2009; Duhaime et al., 2003; Raghupathi et al., 2004; Missios et al., 2009; Duhaime and Durham, 2007; Freeman et al., 2008; Wong et al., 2008; Pryds et al., 2005].
Other mediators of secondary injury include inflammatory and excitotoxic mechanisms, oxidative stress, and apoptosis [Ruppel et al., 2002; Chiaretti et al., 2009; Bittigau et al., 2003]. For instance, in the infant brain, neuronal apoptosis predominates as a cause of neuronal cell death, whereas in the adult brain, neuronal cell death is more associated with necrosis [Vexler et al., 2006; Lea and Faden, 2001]. Cytochrome C, a marker of apoptosis, has been shown to be increased in young children with inflicted head injury compared to a group of children with unintentional head injury [Satchell et al., 2005]. Mitochondrial dysfunction in the face of significant oxidative stress also plays a role [Robertson et al., 2009].
The immature gyrencephalic brain (having convolutions) is more resistant to direct cortical deformation and isolated subdural hematoma than the mature brain, but is more sensitive to white-matter strains [Duhaime et al., 2003; Raghupathi et al., 2004; Missios et al., 2009]. Specific vulnerability likely depends on maturational factors, as well as simultaneous physiologic stresses such as hypoventilation and seizures, and is the focus of on-going research [Duhaime and Durham, 2007].
On a more macroscopic level, age less than 4 years is a risk factor for development of impaired cerebral autoregulation, regardless of severity of traumatic brain injury in humans as autoregulation is not fully functional in premature infants and in animal studies [Freeman et al., 2008; Wong et al., 2008; Pryds et al., 2005; Tuor and Grewal, 1994; Zernikow et al., 1994]. Efficiency of the autoregulatory response improves with age [Tuor and Grewal, 1994]. Further, the more severe the abnormalities of cerebral autoregulation, the greater potential for reduced cerebral blood flow throughout the cortex and subcortical white matter in infants, and the worse the outcome, particularly with inflicted injury [Vavilala et al., 2004; Chaiwat et al., 2009].
Mechanisms of Injury
Basic principles of the mechanisms of head injury are the same for all ages and are reviewed in Chapter 74. As with other tissues, brain injuries occur when applied forces strain the tissue beyond its structural or functional tolerance. Differences exist in the specific patterns of response at different ages. When modeled biomechanically, significant forces are required to cause the type of injuries seen in children with severe inflicted trauma [Duhaime et al., 1987; Prange et al., 2003]. In general, greater applied forces result in more severe injuries, regardless of whether the injury was inflicted.
The exact type and the magnitude of applied forces determine the severity of injury. Most severe primary brain injuries in the clinical world result from head contact, because the contact, and the inertial forces generated when the head stops suddenly against a surface, are much higher than those produced by noncontact mechanisms, where the change in velocity occurs much more slowly and therefore the deceleration magnitude is much smaller. Rotational movement of the head from side to side (lateral movement) is associated with more brain damage than equivalent movements in the anterior–posterior direction, regardless of whether there is impact [Xiao-Sheng et al., 2000; Zhang et al., 2009].
Contribution of hypoxia-ischemia
Developmental differences alone fail to explain why children with inflicted head injury have more severe illness and a higher mortality risk than do children of the same age with unintentional head injury [Hymel et al., 2007; Case, 2008; Ichord et al., 2007; Vinchon et al., 2005]. There are numerous age-related differences in regional cerebral blood flow, autoregulatory mechanisms, and regional sensitivity to hypoxia, which all contribute to enhanced vulnerability to secondary ischemic brain damage initiated by mechanical brain injury.
Infant animals and human children less than 24 months of age seem particularly prone to secondary injury from hypoxia-ischemia after severe traumatic brain injury [Adelson et al., 1997; Ashwal et al., 2000]. In inflicted head injury, it appears to be the most significant factor contributing to the evolution of parenchymal injury and deleterious outcome [Aldrich et al., 1992; Ashwal et al., 1996; Biousse et al., 2002; Duhaime et al., 1993; Hymel et al., 2007; Duhaime and Durham, 2007; Pigula et al., 1993]. Immature medullary respiratory drive and lowered threshold to disruption of respiratory and cardiovascular drive may be the basis of the prolonged apnea and hypotension observed in severely injured young children [Aldrich et al., 1992; Goldstein et al., 1993; Bittigau et al., 2003; Adelson et al., 1997; Pigula et al., 1993; Goldstein et al., 1996].
Trauma-induced hypoxia and hypotension are strongly associated with adverse outcomes in adults and children and are an index of neurologic insult and shock [Gilles and Nelson, 1998; Hymel et al., 2007; Ichord et al., 2007; Kemp et al., 2003; Chesnut et al., 1993; Marton et al., 2007; Chiaretti et al., 2000]. Most infants with severe head injury, inflicted or not, experience apnea or altered respiration during or just after injury [Gilles and Nelson, 1998; Kemp et al., 2003; Johnson et al., 1995]. Apnea and hypotension can also be a response to smothering or to direct vessel compromise caused by strangulation [Shaikh et al., 2001; Ashwal et al., 1991].
Clinical Features
Acute Presentation
Regardless of age, there is a predictable pattern of brain response to mechanical trauma, whether blunt force, primarily angular acceleration-deceleration, or both. Studies have demonstrated repeatedly that severe primary brain injuries result in immediate decrease in the level of consciousness, apnea, and initial hypertension (from catecholamine surge), followed by hypotension [Atkinson, 2000; Denny-Brown and Russell, 1941; Ommaya and Gennarelli, 1974]. An apneustic response (abnormal breathing pattern with deep, gasping inspirations followed by brief insufficient expiration) is triggered, arising from disruption of the cerebral-brainstem reticular activating system and respiratory centers of the upper medulla/lower pons, or from loss of afferent vagal activity.
Symptoms of brain injury are nonspecific, and vary by the severity of injury. The classic presentation is that of a previously healthy infant who suddenly decompensates neurologically and develops severe respiratory distress and flaccidity, with or without seizures or posturing (usually extensor) in the absence of any trauma history [Talbert, 2008; Biron and Shelton, 2007; Arbogast et al., 2005; Gilles and Nelson, 1998; Kemp et al., 2003; Fujiwara et al., 2008]. Following initial loss of consciousness and apnea of variable duration, infants may remain apneic or develop other breathing patterns correlating with the severity of respiratory drive disruption. Less severely injured infants with concussive symptoms may exhibit a depressed level of consciousness, irritability, altered behavior, seizures, decreased feeding, vomiting, fever, and altered respiration. They may not appear sick enough for the perpetrator to seek medical attention. The accompanying caregiver may be unaware of the injury or may be the nondisclosing perpetrator.
Persistence of coma, apnea or respiratory distress, irritability, seizures, hypotonia, and vomiting reflects the severity of mechanical and physiologic disruptions and evolving secondary pathophysiologic cascades [Fujiwara et al., 2008; Gilles and Nelson, 1998; Goldstein et al., 1993; Johnson et al., 1995]. Infants who are severely injured cannot regulate behavior that requires higher cortical functions, such as coordinated action, eating, sitting, and walking. As Graham and colleagues noted, if an individual who sustains a head injury is able to talk, brain damage at the moment of injury cannot have been overwhelming [Graham et al., 1989].
Infants with increased intracranial pressure may have a bulging fontanel and diastatic sutures. Young children with inflicted brain injury develop the same patterns of brainstem abnormalities (pupils, eye movements, and breathing) as children and adults with severe unintentional brain injury [Gilles and Nelson, 1998; Johnson et al., 1995; Atkinson et al., 1998; Hahn et al., 1983].
Early Post-Traumatic Seizures
Early post-traumatic seizures (those occurring in the first 72–96 hours after trauma) are more common in infants and young children with inflicted head injury than in older children or adults with unintentional head injury or cerebral infarction from other etiologies [Chiaretti et al., 2000; Johnson et al., 1995; Barlow et al., 2000; Bechtel et al., 2004; Myhre et al., 2007]. The majority of early post-traumatic seizures start in the first 24 hours after trauma, and although seizures can accompany injury events, extensor posturing is more common after injury [Chiaretti et al., 2000; Johnson et al., 1995; Barlow et al., 2000; Bechtel et al., 2004; Myhre et al., 2007; Lewis et al., 1993]. Seizures are most commonly partial, partial complex, or partial, with or without secondary generalization. Clinical signs may be subtle or even absent. Although the onset of symptoms generally correlates with the timing of injury, clinically evident early post-traumatic seizures cannot and should not be used to time the injury events.
The frequency of seizures ranges from 30 to 80 percent in infants with nonaccidental injury and seems to vary in part as a function of the severity of injury, especially the severity of the degree of hypoxia-ischemia [Gilles and Nelson, 1998; Chiaretti et al., 2000; Johnson et al., 1995; Barlow et al., 2000; Bechtel et al., 2004; Myhre et al., 2007; Lewis et al., 1993]. Early post-traumatic seizures correlate with inflicted mechanism of injury, age younger than 18 months, loss of consciousness, low Glasgow Coma Scale score, and abnormal computed tomographic (CT) scan findings [Gilles and Nelson, 1998; Vinchon et al., 2005; Marton et al., 2007; Myhre et al., 2007; Lewis et al., 1993; Golden and Maliawan, 2005].
Early post-traumatic seizures potentiate postinjury ischemic cascades in the immature injured brain by compromising oxygen and substrate delivery to neurons and, if unrecognized, can be the source of severe secondary injury [Gilles and Nelson, 1998; Vinchon et al., 2005; Marton et al., 2007; Myhre et al., 2007; Lewis et al., 1993; Golden and Maliawan, 2005; Chiaretti et al., 2000; Diaz-Arrastia et al., 2009; Hossain, 2005]. The high incidence of early post-traumatic seizures and the difficulty encountered in their control support the early use of prophylactic antiepileptic medication in the child with severe inflicted closed head injury, particularly if a cerebral infarction syndrome is suspected. Only one study has examined this issue specifically, but as the majority did not sustain inflicted injury and did not have seizures, the efficacy of this intervention remains unproven [Young et al., 2004].
Post-traumatic epilepsy develops significantly more frequently in young children after inflicted head injury, compared with children with unintentional injury or adults after closed head injury [Gilles and Nelson, 1998; Diaz-Arrastia et al., 2009; Talvik et al., 2007; Ates et al., 2006]. The rate varies by study, but is generally in the 20–30 percent range, compared with less than 2 percent in adults [Gilles and Nelson, 1998; Barlow et al., 2000; Diaz-Arrastia et al., 2009; Talvik et al., 2007; Ates et al., 2006; Ghahreman et al., 2005; Singer, 2001]. Types of post-traumatic epilepsy syndromes are typically motor or partial complex. Of infants less than 6 months old at the time of injury who sustain severe hypoxic-ischemic injury, an occasional individual will develop infantile spasms 3–6 months after injury.
Sequelae of Inflicted Head Injury
Outcome data regarding inflicted childhood head injury have been accumulating since the 1950s. In contrast to the majority of children with severe unintentional head injury, who tend to be older and generally have better outcomes, infants have a higher mortality rate and significant sequelae [Bonnier et al., 2003; Gilles and Nelson, 1998; Duhaime et al., 1987; Goldstein et al., 1993; Kemp et al., 2003; Hymel et al., 2007; Keenan et al., 2006]. The majority of severely injured infants initially become macrocephalic, frequently with diastatic sutures and bulging fontanels. With time, cerebral atrophy and associated microcephaly develop, with enlargement of the cerebrospinal fluid spaces [Bonnier et al., 2003; Gilles and Nelson, 1998; Lo et al., 2003]. The development of posttraumatic hydrocephalus is less common.
Predictors of poor outcome are similar to those for other types of head injury. Clinically, these include low scores on the Glasgow Coma Scale or other infant coma indices, early hyperglycemia, extensor posturing, lack of brainstem reflexes on presentation, and coma duration of more than 7 days (Box 75-2) [Johnson et al., 1995; Chiaretti et al., 2002; Cochran et al., 2003; Durham et al., 2000; Prasad et al., 2002]. In inflicted head injury, age less than 4 years, type and repetitiveness of injury events, severity of brain swelling and subsequent brain atrophy, post-traumatic seizures, presence and number of focal lesions, and severe retinal hemorrhages are other important predictors. Cognitive and motor outcome correlates directly with severity of parenchymal injury [Bonnier et al., 2003; Prasad et al., 2002; Barlow and Minns, 1999].
There are an increasing number of markers of brain damage being studied as potential prognostic indicators after early-life traumatic brain injury. These include some that have been in use for a number of years, such as neuron-specific enolase and S100B [Chiaretti et al., 2009; Beers et al., 2007]. More recently, a number of other biomarkers, such as doublecortin, a neurotrophic factor, interleukin-6 (IL-6), IL-8, IL-10, soluble intracellular adhesion molecule (SICAM), L-selectin, and endothelin, have also been studied, with significant outcome predictability using paired biomarkers [Lo et al., 2009]. This was recently reviewed by Kochanek et al [Kochanek et al., 2008].
One of the most significant findings is the proportion of survivors developing microcephaly, a key indicator of the severity of brain injury in these young children. Up to 100 percent of severely injured children had evidence of cerebral atrophy on later imaging, typically evident within 1–2 months after admission [Gilles and Nelson, 1998; Lo et al., 2003; Perez-Arjona et al., 2003]. Visual impairment and behavioral, cognitive, and motor disabilities are common after inflicted head injury, and infants have an increased likelihood of developing post-traumatic epilepsy [Bonnier et al., 2003; Gilles and Nelson, 1998; Kemp et al., 2003; Duhaime et al., 1996; Ewing-Cobbs et al., 1999; Gilles et al., 2003; Haviland and Russell, 1997].
Cognitive and Executive Function
More than 50 percent of survivors have severe developmental disabilities (many vegetative and nonambulatory), with a sizeable proportion completely dependent for daily care [Hymel et al., 2007; Duhaime et al., 1996; Ewing-Cobbs et al., 1998, 1999; Barlow et al., 2005; Keenan et al., 2007]. Of these, 20–30 percent have post-traumatic epilepsy [Gilles and Nelson, 1998; Barlow et al., 2005]. Cognitive and executive functions are often severely impaired, with attention and emotional regulation frequently affected [Barlow et al., 2005; Keenan et al., 2007; Nadebaum et al., 2007]. Many survivors who are interactive develop pseudobulbar palsy.
Behavioral Sequelae
Similar to survivors of severe traumatic brain injury at other ages, behavioral problems are common, ranging from perseveration to oppositional behavior. Temper tantrums occur frequently and may begin to manifest as the child reaches a functional age of 2 years [Ewing-Cobbs et al., 1999; Barlow et al., 2005]. There are no systematic studies as to the most effective interventions for these behavioral disorders.
Visual Sequelae
Sequelae of ocular pathology are common, with 32–90 percent of children having visual impairments [Gilles et al., 2003; Barlow et al., 2005; Kivlin et al., 2008; McCabe and Donahue, 2000]. Cortical visual impairment contributes more to long-term visual deficits than do anterior visual defects, and is most frequently a homonymous hemianopia [Gilles et al., 2003; Kivlin et al., 2008]. Retinal hemorrhages generally resolve by 4 months after admission; those that are small resolve without compromising vision [McCabe and Donahue, 2000]. Larger hemorrhages, especially those near or obscuring the macula, may affect central vision permanently [McCabe and Donahue, 2000; Ehrenfest, 1922]. A few infants require vitrectomy for persistent vitreal hemorrhages.
Motor Sequelae
Motor deficits are exceedingly common and are found in over 50 percent of survivors of early-life abuse [Hymel et al., 2007; Barlow et al., 2005; Holloway et al., 1994]. Typical features are spastic hemiparesis, often superimposed on quadriparesis; spastic quadriparesis, often with truncal hypotonia; and, not infrequently, mixed tone, with elements of dystonia or choreoathetosis. Severe extensor posturing is not uncommon.
Neuroimaging
Findings of diffuse hypodensity and evidence of shear injury are predictive of worse outcome, regardless of imaging modality. T2-weighted and fluid-attenuated inversion-recovery imaging (FLAIR), diffusion abnormalities, and susceptibility images are most predictive of injury severity and outcome on magnetic resonance imaging (MRI) [Babikian et al., 2005; Foerster et al., 2009; Galloway et al., 2008; Sigmund et al., 2007; Suh et al., 2001; Tong et al., 2003]. Reduced N-acetylaspartate and elevated lactate levels on MR spectroscopy correlate with poor outcomes [Aaen et al., 2010; Ashwal et al., 2006; Babikian et al., 2006]. Long-term imaging anomalies include diffuse cortical atrophy, generalized white-matter attenuation, multicystic encephalomalacia, porencephaly, and ulegyria [Bonnier et al., 2003; Geddes et al., 2001; Marin-Padilla et al., 2002]. The presence and extent of parenchymal abnormalities on imaging at 3 months post injury is negatively predictive of neurodevelopmental outcome [Bonnier et al., 2003].
Pathologic Features
A broad range of physical findings is associated with inflicted head injury [Wygnanski-Jaffe et al., 2009; Tung et al., 2006]. Not all injuries have to be present to make the diagnosis, and no individual markers are pathognomonic of abusive injury. The major markers of injury are outlined in Box 75-3.
Extracranial Injuries
Scalp
The most common extracranial impact injuries in inflicted injury are scalp bruising, soft-tissue swelling, cephalohematoma, and subgaleal hematoma (Figure 75-1) [Duhaime et al., 1987; Case, 2008; Bechtel et al., 2004; Rubin et al., 2003]. Scalp bruising may be difficult to discern clinically [Atwal et al., 1998; Peters et al., 2008]. Skull fractures and subgaleal hematomas are more commonly associated with bruising than extremity fractures [Peters et al., 2008]. Atwal and associates found that facial and forehead bruising predominated in a series of fatal inflicted head injury, whereas limb and chest bruising were uncommon; this suggests that slapping or punching of the head, not gripping of the chest or arms and shaking, was more common in that series. Other authors have found a predominance of parieto-occipital sites for contact injuries. Subgaleal hematomas from abuse result from shearing injury to the scalp from impact or from a violent hair pulling [Anton et al., 1999; Hamlin, 1968; Greenes and Schutzman, 1998; Case et al., 2001; Ewing-Cobbs et al., 2000; Geddes et al., 2001; Shane and Fuchs, 1997; Kemp et al., 2008; Hobbs, 1984; Meservy et al., 1987; Arnholz et al., 1998; Banaszkiewicz et al., 2002; Jayakumar et al., 2010; Hiss and Kahana, 1995].
Skull Fractures
A variety of skull fractures have been reported after blunt trauma, regardless of etiology (see Figure 75-1). As such, they are not specific to either unintentional or inflicted trauma [Greenes and Schutzman, 1998]. Depending on the study, between 25 and 74 percent of infants and children with inflicted neurotrauma sustain skull fractures [Duhaime et al., 1992; Case et al., 2001; Ewing-Cobbs et al., 2000; Geddes et al., 2001; Shane and Fuchs, 1997; Kemp et al., 2008]. Simple linear fractures are the most common, and tend to be parietal or occipital in location [Rubin et al., 2003; Shane and Fuchs, 1997; Hobbs, 1984; Meservy et al., 1987].
In general, fractures that are complicated – i.e. bilateral, comminuted, multiple, or that cross suture lines – are more suggestive of being inflicted [Shane and Fuchs, 1997; Hobbs, 1984; Meservy et al., 1987; Arnholz et al., 1998; Banaszkiewicz et al., 2002; Jayakumar et al., 2010]. In one study of children with inflicted fractures, a skull fracture was most commonly associated with bruising or subgaleal hematoma at or near the fracture site [Peters et al., 2008]. Fractures in infants with open sutures generally do not usually cross suture lines. Bilateral or other multiple fractures usually indicate that there was more than one impact but have also been reported after simultaneous, severe head compression between two unyielding surfaces or from direct occipital impacts [Arnholz et al., 1998; Hiss and Kahana, 1995]. In rare cases, complicated fractures occur after falls.
Intracranial Injuries
In most severely injured children, neuroimaging reveals intracranial abnormalities, most often acute subdural hematoma, cerebral hypodensity, and intraparenchymal hemorrhage (Figure 75-2, 75-3, and 75-4; see also Figure 75-5A and 75-9) [Bonnier et al., 2003; Chiesa and Duhaime, 2009; Reece and Sege, 2000; Myhre et al., 2007; Datta et al., 2005].
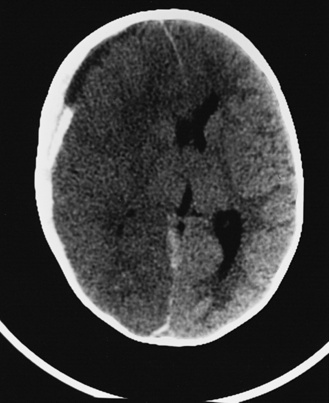
Fig. 75-4 Nonenhanced computed tomographic scan with an acute mixed-density right subdural hematoma.
Subdural Hematoma
Regardless of etiology, subdural hematomas are the most common intracranial pathological abnormality found in inflicted head injury. They are observed in up to 100 percent of infants and young children with inflicted neurotrauma [Foerster et al., 2009; Vinchon et al., 2005; Golden and Maliawan, 2005; Fernando et al., 2008; Vinchon et al., 2004; Kivlin, 2001; Duhaime et al., 1992; Feldman et al., 2001]. In fatal cases, some blood is almost always present in the subdural space.
Subdural hematomas associated with inflicted injuries in children are typically interhemispheric, unilateral, or bilateral over the cerebral convexities (see Figure 75-2, 75-3, 75-4, 75-5, and 75-9) [Biousse et al., 2002]. Interhemispheric or falcine subdural hematomas are particularly suspect for inflicted injury. Significant convexity or interhemispheric subdural hematomas with associated neurologic signs are extremely rare as a result of any etiology other than trauma. In adults, these are most often observed in the context of moderate to severe accidental traumatic head injury (see Figure 75-2 and 75-3) [Tzioumi and Oates, 1998].
Subdural hematomas develop from several mechanisms, alone or in combination, including the tearing of bridging veins extending from the cortex to the dural sinuses (particularly the superior sagittal sinus), injured surface vessels (about 10 percent), and dural lacerations [Case, 2007; Gennarelli et al., 1982; Matsuyama et al., 1997; Shenkin, 1982; Stålhammer, 1986]. Less commonly, they arise from the rupture of cortical arteries [Matsuyama et al., 1997]. Acute subdural hematomas typically liquefy and are resorbed, but may persist as chronic subdural effusions, and even become chronic and eventually calcify [Zouros et al., 2004; Tokmak et al., 2007].
Mixed-density or “hyperacute” subdural hematoma
Mixed-density subdural hematomas on CT scanning in an acutely injured individual are frequently misdiagnosed as “acute on chronic” subdural hematoma. Unlike the older individual with a chronic hematoma and a clearly delineated membrane, the mixed-density subdural collection in the acutely injured infant results from rapidly accumulating blood in the potential subdural space, and at surgery is typically found to be associated with fresh nonclotted blood with no evidence of an organized membrane [Zouros et al., 2004].
Other potential mechanisms for a mixed-density appearance are settling, washout, or compartmentalization of hemorrhage products (Figure 75-6; see also Figure 75-4 and 75-9) [Tung et al., 2006; Greenberg et al., 1985; Sargent et al., 1996]. Sometimes, cerebrospinal fluid mixed with blood in a subdural hematoma may also appear to be of mixed density (see Figure 75-4) [Zouros et al., 2004; Vinchon et al., 2009]. When seen in the context of an injured infant or young child, it is significantly more likely to be due to abuse [Tung et al., 2006]. Not infrequently, this finding is associated with abnormalities of coagulation [Greenberg et al., 1985].
Chronic subdural hematoma
Chronic subdural hematomas develop when an acute subdural hematoma fails to organize, either because of intrinsic abnormalities in coagulation within the clot, or more often because of rebleeding or exudation into the well-defined outer and inner membrane [Tokmak et al., 2007; Shim et al., 2007]. There is a predictable sequence in their growth, with the development of a membrane around the liquefying clot, and then neovascularization within, and finally ossification [Walter et al., 2009].
Chronic subdural effusions
Proteinaceous subdural collections frequently evolve from resolving acute subdural hematomas in young infants (Figure 75-7 and 75-8; see also Figure 75-6). In infants with traumatic subdural hematoma, the evolution to a chronic subdural collection (hygroma or effusion) may be associated with tears in the arachnoid and may not have the classic membrane structure [Zouros et al., 2004]. They may develop within days of the injury [Vinchon et al., 2009]. Sometimes referred to as subdural hygromas, they either resolve with time, or persist when there is diffuse brain injury with secondary atrophy [Hasegawa et al., 1992; Kaufman, 1993; Wetterling et al., 1988].
Subdural hygromas are defined as accumulations of fluid in the subdural space. The use of the term hygroma is controversial [Wetterling, 1988 #6429]. Like many terms, it came into use before more sophisticated imaging, such as MRI, was available to determine noninvasively the variability in the characteristics of extra-axial collections. Subdural hygromas are variably attributed to resolving subdural hematomas or to abnormal collections of cerebrospinal fluid, alone or mixed with blood, in the subdural space [Zouros et al., 2004]. Analysis of attenuation coefficients on CT scan is often adequate for making the diagnosis. If there is any question, MRI is the preferred technique, as it is accurate in determining whether extra-axial fluid is of cerebrospinal fluid intensity and whether fluid is in the subarachnoid or subdural space [Hasegawa et al., 1992; Wilms et al., 1993]. The majority of subdural collections resolve without intervention (see Figure 75-8).
Subarachnoid Hemorrhage
Subarachnoid hemorrhage develops after direct surface trauma to the brain, or from leakage of blood from injured cerebral vessels within the subarachnoid space. Subarachnoid hemorrhage is difficult to identify on CT scans and to differentiate from subdural hematoma, especially when located interhemispherically. In the setting of trauma, most patients with subdural hemorrhage also have subarachnoid hemorrhage, because of tearing of the arachnoid at the time of the injury. Abused infants and young children are no exception [Golden and Maliawan, 2005].
Epidural Hematoma
Arterial epidural hematoma is uncommon after trauma, especially after inflicted head injury [Gerlach et al., 2009; Schutzman et al., 1993; Shugerman et al., 1996]. It is discussed here to clarify its pathogenesis and clinical course. Both venous and arterial epidural hematomas can occur after relatively minor trauma [Schutzman et al., 1993]. The majority of arterial epidural hematomas develop adjacent to the middle meningeal artery or over the cerebral convexity, but they can also occur in the posterior fossa and are usually venous, rather than arterial, in this location. Venous epidural collections most often result from skull fractures, where the bone itself is the source of hemorrhage; these are most often low-pressure hemorrhages and usually are self-limited.
Arterial epidural hematomas typically accumulate more rapidly and result in larger and more clinically significant clots. Skull fracture is present in two-thirds of cases of arterial epidural hematomas. Epidural hematomas develop when the middle meningeal artery is torn as the result of temporal bone deformation. Fracture is not always a consequence, particularly in an infant, whose increased skull compliance allows increased skull deformation at the impact site [Shugerman et al., 1996]. In this situation, locally deforming forces do not usually injure brain parenchyma away from the impact site. Symptom progression varies from dramatic to more protracted, paralleling the rate of epidural blood accumulation.
Children with epidural hematomas are much more likely to have a lucid interval and do well clinically than are severely abused infants (see also “Timing” section) [Schutzman et al., 1993]. This finding is congruent with the biomechanics of injury because epidural hematomas result primarily from brief linear deceleration impacts, such as falls [Schutzman et al., 1993; Shugerman et al., 1996]. These children typically do well with prompt surgical intervention when needed and aggressive supportive care [Gerlach et al., 2009].
Brain Injuries
Brain swelling
Diffuse or focal brain hypodensity is the most common parenchymal pattern found on initial CT imaging [Duhaime and Durham, 2007; Kemp et al., 2003; Golden and Maliawan, 2005; Kazan et al., 1997; Rao et al., 1999]. Younger infants are more likely to present with diffuse hypodensity, with or without bilateral acute subdural hematomas, presumably reflecting the greater likelihood of diffuse hypoxic-ischemic insult (see Figure 75-5 and 75-8). Older infants and children more often present with focal or hemispheric brain swelling and ipsilateral acute subdural hematomas (Figure 75-9; see also Figure 75-4) [Gilles and Nelson, 1998; Duhaime et al., 1992; Kemp et al., 2003]. The particular pattern of severe bilateral hemispheric hypodensity is rare in adults, but not uncommon in infants after severe injury [Gilles and Nelson, 1998; Duhaime et al., 1992; Kemp et al., 2003]. The so-called “black brain” signifies profound parenchymal injury and secondary swelling [Duhaime and Durham, 2007]. Traumatic brainstem lesions are not common [Brennan et al., 2009; Eder et al., 2000].
Focal swelling has several patterns: hemispheric subjacent to an ipsilateral acute subdural hematoma, less often in a vascular distribution from an evolving infarction, or patchy hypodensity not in a vascular distribution. None of these patterns is specific for abuse [Lobato, 1993; Sarabia et al., 1988; Tomita et al., 1997]. Although there is a tendency to infer that focal brain swelling equates to localized brain injury, the finding of persistent coma in severely injured children in the absence of significant midline shift is supportive evidence that diffuse injury has also occurred.
Hemispheric swelling
Apnea or hypotension alone does not completely explain why one-third of abused infants and young children present with hypodensity affecting one hemisphere, in so far as these processes would be expected to be symmetric, so some synergy among pathophysiologic stressors appears to be at play [Gilles and Nelson, 1998; Duhaime and Durham, 2007; Duhaime et al., 1993]. In adults, asymmetric hemispheric swelling is associated with the highest mortality rate [Eisenberg et al., 1990; Lobato et al., 1988].
The etiology of hemispheric swelling and secondary necrosis subjacent to an acute subdural hematoma is not well understood. Putative mechanisms include the adverse effects of local pressure and midline shift (decreasing cerebral metabolic rate of oxygen), association with cortical ischemia, and decreased glucose metabolism [Miller et al., 1990; Valadka et al., 2000; Baechli et al., 2010; Schroder et al., 1994]. When hypoxia is added to the mix, it appears to result in further decreases in cerebral blood flow and severe brain swelling [Orlin et al., 1992; Sawauchi et al., 2004; Tomita et al., 1997].
Strangulation has been proposed as a causal mechanism of brain swelling, particularly hemispheric swelling, but corroborative clinical data are limited (Figure 75-10 and Figure 75-11) [Gilles and Nelson, 1998; Duhaime et al., 1996; Bird et al., 1987; Bonnier et al., 1995; Drack et al., 1999]. Circumferential strangulation in a young child can simultaneously compress carotid and vertebral arteries (the carotid arteries by anterior neck compression, and the vertebral arteries as they pass through the scalene muscles) [Rossen et al., 1943]. Traumatic vascular injury secondary to strangulation should be diagnosed only if there is unequivocal clinical, imaging, or postmortem examination evidence.
Cerebral infarction
Post-traumatic cerebral infarction develops after traumatic brain injury in both children and adults, predominantly but not exclusively as a result of vascular compression and herniation [Gilles and Nelson, 1998; Mirvis et al., 1990; Server et al., 2001; Steinborn et al., 2010]. Post-traumatic cerebral infarction after inflicted head injury has received relatively little attention [Gilles and Nelson, 1998; Bird et al., 1987; Ransom et al., 2003; Zimmerman et al., 1979]. Development of a post-traumatic infarction is more likely if the child is abused, but the actual frequency is unknown [Ransom et al., 2003]. In adult studies, the frequency ranges from 8 to 19 percent. Posterior cerebral artery and branch anterior cerebral artery distribution infarctions predominate (see Figure 75-7 and 75-9). Infarction in the lenticulostriate-thalamoperforator distribution leads to basal ganglia and thalamic injury. Hemispheric necrosis and border zone infarction are other prominent patterns (see Figure 75-9). The majority of infarctions in adults are also in vascular territories localizing to middle cerebral artery, lenticulostriate arteries, and posterior and anterior cerebral arteries, but border zone infarctions also occur [Marino et al., 2006]. In selected patients, evaluation for carotid arterial dissection needs to be considered.
The extent of infarction is correlated with poor outcome [Gilles and Nelson, 1998; Server et al., 2001]. Risk factors in adults include Glasgow Coma Scale score, hypotension, brain herniation and decompressive craniotomy, and blunt trauma to the neck [Steinborn et al., 2010; Marino et al., 2006; Tawil et al., 2008; Tian et al., 2008; Latronico and Marino, 2009]. Strangulation is not a common cause of cerebral infarction in abused infants [Gilles and Nelson, 1998; Drack et al., 1999].
Diffuse axonal injury
Diffuse axonal shear injury is an important determinant of clinical status and outcome from nonmissile head injury [Adams et al., 1982; Hammoud and Wasserman, 2002; Strich, 1961]. It is a common feature of severe traumatic brain injury in older children and adults; its frequency is not established in infants, and there is conflicting evidence about its importance in inflicted head injury [Tokutomi et al., 1997; Tong et al., 2004]. Diffuse axonal shear injury has been described in infants younger than 5 months of age, but it is not commonly diagnosed at necropsy in abused infants [Vowles et al., 1987].
Major angular deceleration forces are required. The most common accidental cause is a high-speed motor vehicle crash or a pedestrian struck by a high-velocity vehicle. It occurs at the moment of injury and, when severe, is associated with loss of consciousness at the moment of impact [Adams et al., 1977; Gennarelli, 1993]. Imaging findings of shear injuries include punctate hemorrhages, particularly at the gray–white matter, and tearing of the corpus callosum (see Figure 75-8) [Demaerel et al., 2002; Mendelsohn et al., 1992].
Cerebral contusions
An infant younger than 8 months is much less likely to develop cortical contusions in coup or contrecoup locations than are older children and adults [Lindenberg and Freytag, 1969]. The incidence of contrecoup injuries rises rapidly from later infancy to 3 years of age, when it begins to approximate that of the adults [Marin-Padilla et al., 2002; Digraham et al., 1989; McLaurine and Tutor, 1961].
White-Matter Contusional Tears
On occasion, young infants present with white-matter tears, also referred to as white-matter contusional tears or gliding contusions [Lindenberg and Freytag, 1969; Calder et al., 1984; Jaspan et al., 1992; Vowles et al., 1987]. Contusional tears occur where shear strain is greatest: namely, deep to the gray matter–white matter junction (Figure 75-12 and Figure 75-13) [Lindenberg and Freytag, 1969; Calder et al., 1984; Jaspan et al., 1992; Gentry et al., 1988]. From 1 to 3 cm in length, they are most often located in the frontal and temporal lobes, although they may be seen throughout the brain [Lindenberg and Freytag, 1969].
These tears are considerably larger than the tissue tear hemorrhages in the adult brain after closed head injury that are punctate and are found most often in the periventricular white matter, corpus callosum, and brainstem [Wilberger et al., 1990]. When white-matter tears are filled with fresh blood, they may be mistaken for parenchymal hemorrhages (rare in childhood inflicted or unintentional head injury). In an acutely swollen brain, white-matter contusional tears may be difficult to visualize on CT or MRI [Gentry et al., 1988]. They are most easily visualized on follow-up imaging studies, in which they appear as slit-like or oval lesions within the cerebral parenchyma (see Figure 75-12).
Ocular Pathology
Retinal Hemorrhages
A range of injuries to the globe, optic nerve, and surrounding tissues has been reported after inflicted injury, including retinoschisis, retinal folds, retinal detachment, periorbital edema and ecchymosis, subconjunctival hemorrhages, hyphema, and cataracts [Vinchon et al., 2009; Buys et al., 1992; Gaynon et al., 1988; Greenwald et al., 1986; Massicotte et al., 1991; Schloff et al., 2002]. Frequent associated findings include macular schisis, and peripapillary scleral hemorrhages [Emerson et al., 2007]. Periorbital bleeding can result from either direct impact to the eye or a basilar fracture.
Retinal and optic nerve sheath hemorrhages are the most common injuries. Their frequency varies from 47 to 100 percent of all children with inflicted head trauma, depending on study inclusion criteria [Fujiwara et al., 2008; Bechtel et al., 2004; Emerson et al., 2007; Togioka et al., 2009; Binenbaum et al., 2009].
Retinal hemorrhages have variable appearance and distribution within the retina, and are often asymmetric (Figure 75-14 and Figure 75-15) [Schloff et al., 2002; Emerson et al., 2007; Levin, 2002; Betz et al., 1996]. The terminology used to describe retinal hemorrhages is based on the location within the retinal layers (Table 75-1). They are most frequently found in the bipolar and nerve fiber layers, and can extend to the retinal edge [Riffenburgh and Sathyavagiswaran, 1991]. Controversy exists as to their terminology, pathogenesis, associations, and resolution. Younger age and greater hypoxic-ischemic injury are correlated with more severe retinal hemorrhages [Bonnier et al., 2003; Vinchon et al., 2005; Binenbaum et al., 2009; Riffenburgh and Sathyavagiswaran, 1991; Gilliland et al., 1994; Matthews and Das, 1996].
Name | Location |
---|---|
Vitreal | Vitreous |
Subhyaloid (sublaminar or boat) | Preretinal |
Intraretinal Flame or splinter Dot (dot and blot) |
Nerve fiber layer Inner retinal layers (including bipolar) |
A pattern of severe, bilateral, diffuse retinal hemorrhages; retinal folds or retinoschisis; or detachment in a child with severe brain injury is highly suggestive but not pathognomonic for inflicted injury (Figure 75-16) [Bechtel et al., 2004; Mills, 1998; Rao et al., 1988]. This pattern is particularly suspect in the setting of a trivial mechanism of injury in an otherwise healthy child [Buys et al., 1992; Betz et al., 1996]. Severe, diffuse retinal hemorrhages, especially with retinal folds or detachments, in the setting of a trivial mechanism of injury in an otherwise healthy child is strongly suggestive of an abusive mechanism [Buys et al., 1992; Betz et al., 1996]. In contrast, these patterns have not been reported in the setting of a low-height fall in an otherwise healthy child; therefore, in this context, some workers consider these findings clearly indicative of abuse [Binenbaum et al., 2009].
The resolution rates of retinal hemorrhages after inflicted injury have not been studied systematically. The only large study is that of Pierre-Kahn and co-workers, who prospectively monitored 231 children younger than 3 years who were admitted with subdural hematoma; they found that 88 percent of retinal hemorrhages resolved within 4 weeks [Pierre-Kahn et al., 2003]. Most birth-related retinal hemorrhages are small and disappear within the first few days to 2 weeks after birth [Ehrenfest, 1922; Emerson et al., 2007; Baum and Bulpitt, 1970; Suzuki and Awaya, 1998]. Macular hemorrhages may take longer to resolve [Suzuki and Awaya, 1998].
Theories of Retinal Hemorrhage Pathogenesis
The pathogenesis of retinal and vitreal hemorrhages in infants with inflicted trauma is multifactorial and not yet fully understood. Several hypotheses have been proposed, with varying amounts of supportive data. These hypotheses include increased intracranial pressure, hydraulic forces, traumatic retinoschisis from deceleration forces, increased optic nerve sheath pressure, and anoxic injury [Gilles and Nelson, 1998; Massicotte et al., 1991; Lyle et al., 1957]. Perhaps the strongest association is that with sudden intracranial hypertension, with or without intracranial hemorrhage, support for which dates back to the 1800s [Ehrenfest, 1922; de Schweinitz and Holoway, 1912; Hedges et al., 1964; Medele et al., 1998; Muller and Deck, 1974; Tureen, 1939; Arlotti et al., 2007].
Additional support for the relation between intracranial pressure and retinal hemorrhage pathogenesis comes from several other sources. Retinal hemorrhages have been correlated with hemispheric intracranial pathologic processes (e.g., ipsilateral to acute subdural hematoma and regional brain swelling) in a variety of settings [Budenz et al., 1994; Shaikh et al., 2001; Gilles et al., 2003; Drack et al., 1999; Arlotti et al., 2007; Christian et al., 1999; Giangiacomo et al., 1988; Keane, 1979; Paviglianiti and Donahue, 1999; Shaw et al., 1977; Forbes et al., 2008; Young et al., 1997]. This literature includes cases of unintentional and inflicted head injury in childhood and studies of adults after head injury, individuals with asymmetric rupture of vascular malformations or epidural hematomas, and infants on extracorporeal membrane oxygenation (ECMO).
Noninvasive measurement of optic nerve sheath diameter in adults with intracranial hemorrhage has been documented to detect changes in intracranial pressure accurately [Firsching et al., 2000; Moretti and Pizzi, 2009; Geeraerts et al., 2008]. Rapid increases in intracranial pressure are not necessarily associated with the development of papilledema, particularly when the sutures remain patent.
Levin has hypothesized that vitreoretinal traction is the main mechanism leading to retinal hemorrhage in inflicted head injury but this hypothesis has not yet been widely accepted [Levin, 2009; Galaznik, 2009]. There are several pieces of evidence that contradict this idea. First, it is quite common to see unilateral or predominantly unilateral retinal hemorrhages ipsilateral to a subdural hematoma. Second, there is an evolution of retinal hemorrhages over time, and the majority of dilated ophthalmologic examinations are performed more than a day after admission. One study had the potential to test this hypothesis but, unfortunately, timing of ophthalmologic examination was not related to timing of imaging and a specific cumulative hemispheric score was not compared to the retinal hemorrhage score per eye in the analysis [Morad et al., 2002].
Cardiac compressions during cardiopulmonary resuscitation do not seem to generate enough transmutative force to raise intracranial pressure sufficiently to result in retinal hemorrhages [Betz et al., 1996; Odom et al., 1997; Gilliland and Luckenbach, 1993; Fackler et al., 1992; Paradis et al., 1989]. Three studies documenting retinal examinations before or after death in more than 200 children who underwent either chest compressions or prolonged resuscitative efforts have failed to find an association between cardiopulmonary resuscitation and retinal hemorrhages [Budenz et al., 1994; Odom et al., 1997; Gilliland and Luckenbach, 1993]. Retinal hemorrhages are extremely unlikely to develop after seizures, Valsalva maneuvers, forceful vomiting, or persistent coughing, or in association with apparent life-threatening events [Togioka et al., 2009; Arlotti et al., 2007; Herr et al., 2004; Sandramouli et al., 1997].
Optic Nerve Sheath Hemorrhage
The optic nerve sheath has dural and arachnoid components, with only the subarachnoid space in continuity with the subarachnoid spaces of the brain. Hemorrhages are found in both the subdural and potential subarachnoid space, and tend to predominate in the distal optic nerve [Emerson et al., 2007]. The frequency of optic nerve sheath hemorrhages is unknown. They are found in 70–100 percent of children at postmortem examination [Budenz et al., 1994; Gilliland et al., 1994; Rao et al., 1988; Wygnanski-Jaffe et al., 2006; Elner et al., 1990]. MRI and ultrasound have successfully been used to measure optic nerve sheath diameter [Metzger et al., 2009; Tao et al., 2000].
Potential pathogenic mechanisms include local rupture of intradural vessels and bridging veins within the optic nerve sheath, injury to the optic nerve sheath with fracture of the base of the skull near the optic foramina, and spread of subarachnoid blood from the pericerebral subarachnoid space [Muller and Deck, 1974; Elner et al., 1990; Brinker et al., 1997; Munger et al., 1993].
Spinal Injuries
Spinal trauma has not been commonly reported as the result of inflicted injury [Feldman et al., 2008; Launay et al., 2005; Diamond et al., 1994; Ghatan and Ellenbogen, 2002; Ranjith et al., 2002; Rooks et al., 1998; Swischuk, 1969]. Autopsy series, however, show a high rate of injury to the upper cervical spinal cord in children who have died from inflicted injury [Geddes et al., 2001; Brennan et al., 2009]. The pathogenesis of cervical injury, whether from shaking, distraction forces, forced flexion during impact, or some other mechanism, remains unknown.
Differential Diagnosis
The scope of clinical and physical findings in inflicted neurotrauma is unique to this disorder. It is extremely uncommon for infants to suffer severe brain injuries from unintentional mechanisms. Falls and birth trauma are the most frequently offered explanations for injury, but these are uncommon causes of severe brain injury [Duhaime et al., 1992; Feldman et al., 2001]. Birth trauma is usually easily excluded as a reasonable explanation by the clinical course and imaging findings. Significant applied forces are required, such as those sustained in a vertical fall of greater than 10 feet or a high-speed motor vehicle accident.
Unintentional Injury
Falls
Fall data can be grouped into short falls (less than 4 feet), walker and stroller falls, falls down stairs, and falls from heights. These data overwhelmingly support the conclusion that severe traumatic brain injury and severe retinal hemorrhages are unlikely to result from household falls, although venous or arterial extra-axial hemorrhages may occur in both younger and older children through this mechanism [Gill et al., 2009; Duhaime et al., 1992; Bechtel et al., 2004; Betz et al., 1996; Elder et al., 1991; Nimityongskul and Anderson, 1987; Paret et al., 1999; Williams, 1991; Kuppermann, 2004].
Fall data should be interpreted cautiously. The majority of published data were collected retrospectively without consistent evaluation protocols. Routine neuroimaging has not been performed in children who were not clinically symptomatic; thus, intracranial injuries, such as small, insignificant subdural hematoma, would have been missed [Kuppermann et al., 2009]. An exception is the seminal study by Kupermann et al., who prospectively enrolled and analyzed 42,412 children presenting within 24 hours of head trauma with a Glasgow Coma Scale score of 14–15 [Kuppermann et al., 2009]. Children younger than 2 years of age were extremely unlikely to sustain clinically important traumatic brain injury.
Short falls
Short falls are generally low-impact, low-velocity injuries. The most common findings are concussions, scalp contusions, some fractures, an occasional epidural hematoma, and an occasional focal subarachnoid hemorrhage. In falls of less than 2 feet that involve fracture, clavicle fractures predominate; skull fractures are more common in falls of 2–4 feet [Musemeche et al., 1991; Roshkow et al., 1990]. Household free falls from furniture or other surfaces, with a head-to-ground distance of less than 3 feet, do not generally result in serious injury or in significant primary brain injuries, with the exception of epidural hematoma which can be a life-threatening injury if not treated promptly [Duhaime et al., 1992; Musemeche et al., 1991; Roshkow et al., 1990; Reiber, 1993]. Falls of 2–4 feet on to a hard surface, such as pavement, cement, linoleum, and wood, have, on rare occasions, resulted in complicated fractures in infants younger than 6 months [Nimityongskul and Anderson, 1987; Reiber, 1993; Lyons and Oates, 1993]. Studies suggesting that serious, life-threatening injury can occur in children allegedly falling short distances have typically relied on uncorroborated histories given by caretakers. Uncorroborated falls have a six times greater rate of serious injury, which suggests that the history was, in fact, suspect [Chadwick et al., 1991, 2008]. Chadwick et al. calculated that the risk of death from short falls in young children was less than 0.48 deaths per million per year [Chadwick et al., 2008].
Walker and stroller falls
Walkers continue to be widely used in the United States, despite the substantial amount of data detailing their risks [Injuries associated with infant walkers, 2001; Chiaviello et al., 1994; Emanuelson, 2003; Smith et al., 1997]. Mechanisms of injury include tipping over, but the majority of cases are due to falls down stairs [Ridenour, 1999]. The most common injuries are extremity trauma, bruises, skull and other fractures, and minor concussions. Rarely, infants sustain more significant intracranial injuries, including subdural hemorrhages and growing fractures.
Falls down stairs
Children falling down stairs (or infants dropped while being carried down stairs) most often have either no injury or fractures of the skull or extremities [Chiaviello et al., 1994; Docherty et al., 2010; Joffe and Ludwig, 1988]. Stairway falls usually are less serious than free falls of the same vertical distance [Joffe and Ludwig, 1988]. No correlation exists between the number of steps and the severity of injury. Most children who fall down stairs tumble and never develop significant acceleration, but exceptions to this general rule do exist, and the specific injuries depend on the exact mechanism and forces involved.
Falls from heights
Children falling from heights differ from children who have been maltreated or who have had minor falls [Williams, 1991; Musemeche et al., 1991; Roshkow et al., 1990; Reiber, 1993]. Extremity injuries again outnumber head injuries, and, overall, children recover better than adults. Rare injuries from falls include superior sagittal sinus rupture after impact to the vertex of the cranium (Figure 75-17).
The brain and skull accelerate at the same rate in a high free fall. The brain may escape major injury, and the skull may shatter with impact because the skull absorbs much of the translational energy and there is little rotational component [Lindenberg, 1973]. In infants and young children, inward deformity of the skull may cause significant surface trauma when falls are from major heights (such as out of windows). Comminuted skull fracture with surface brain contusion, subarachnoid hemorrhage, or both in older children is consistent with a history of a high free fall. Venous or arterial epidural hemorrhages may occur in both older and younger children through this mechanism.
Birth Trauma
Stresses applied to the cranial vault and its coverings during the birth process are an uncommon cause of a number of injuries [Shapiro and Smith, 1993]. Extracranial injuries predominate, including caput succedaneum, cephalohematomas, and subgaleal hematomas. Skull fractures, found after vaginal delivery, forceps use, or cesarean section, are generally either linear or depressed. Complicated skull fractures usually herald serious intracranial pathologic processes. Epidural and subdural hematomas, subarachnoid hemorrhage, and intracerebral hemorrhage have all been reported [Shapiro and Smith, 1993; Hayashi et al., 1987]. Subdural hematomas are usually associated with a difficult delivery or an infant large for gestational age. Approximately 70 percent of neonates with subdural hematoma have a good outcome, which is consistent with the absence of associated primary cerebral injury [Hayashi et al., 1987]. Rarely, injuries occur involving the vertebral column, particularly the cervical region. If cord transection results, it is uniformly fatal.
Between 11 and 50 percent of full-term newborns have retinal hemorrhages after vaginal delivery [Emerson et al., 2001; Govind et al., 1989; O’Leary et al., 1986]. These have been hypothesized to be a consequence of molding and compression of the cavernous sinus, but exactly how they develop is unknown [Ehrenfest, 1922]. Retinal hemorrhages are more frequent after complicated or traumatic births (such as those involving vacuum extraction), protracted labor, and prematurity [Emerson et al., 2001; O’Leary et al., 1986; Demissie et al., 2004; Egge et al., 1980, 1981; Sezen, 1971]. Types of hemorrhage are equivalent to those in infants with inflicted injuries (flame, dot and blot, subhyaloid), but are rarely severe and are not usually associated with visual loss [Ehrenfest, 1922; Baum and Bulpitt, 1970; Sezen, 1971; Zwaan et al., 1997].
Neurometabolic Disease
There are rare case reports of infants with metabolic disease who present with signs and symptoms that are misdiagnosed as abusive injury. Typically, children with metabolic disease do not present with the range of findings found in abused infants. Careful history taking, physical examination, and imaging characteristics usually suggest metabolic disease as a differential possibility. Infants with glutaric aciduria type 1 may present with macrocephaly, subdural effusions, and spasticity [Drigo et al., 1996; Kimura et al., 1994; Land et al., 1992; Osaka et al., 1993; Strauss et al., 2003; Nassogne et al., 2002; Gago et al., 2003]. These infants had other neuroimaging features of organic acidurias: namely, widened operculae and alterations of signal intensity in the basal ganglia [Brismar and Ozand, 1994]. Menkes’ disease may also manifest in infancy with subdural fluid collections and severe neurologic findings, such as seizures and developmental delay [Nassogne et al., 2002]. There are rare reports of type I osteogenesis imperfecta presenting with small subdural hematoma, with and without retinal hemorrhages after minor trauma [Ganesh et al., 2004; Sasaki-Adams et al., 2008]. These conditions are extremely rare causes of extra-axial fluid collections. Metabolic screening tests should be considered in infants presenting with somnolence, vomiting, seizures, dystonia, or dyskinesia and extra-axial fluid collections (either subdural effusion or subdural hematoma), particularly if ketoacidosis is present.
Differential of Specific Findings
Retinal and Optic Nerve Sheath Hemorrhages
Retinal hemorrhages are rarely found in children after accidental head injury, including low-height falls, but are usually small and scattered hemorrhages, often occurring unilaterally, in an otherwise well-appearing child [Buys et al., 1992; Christian et al., 1999]. In four prospective studies, investigators examining a total of 290 children after accidental head injury found 3 children with retinal hemorrhages [Duhaime et al., 1992; Buys et al., 1992; Elder et al., 1991; Johnson et al., 1993]. Two of the 3 children with retinal hemorrhages were involved in side-impact car accidents [Johnson et al., 1993].
Retinal and optic nerve sheath hemorrhages are found in numerous disorders. They have been reported most commonly in newborns but also with vascular malformations, coagulopathies, anemia, leukemia, meningitis, bacterial endocarditis, hypertension, infections, and papilledema [Schloff et al., 2002; Emerson et al., 2001; Carraro et al., 2001; Eisenbrey, 1979; Kessler and Siegel-Stein, 1984; Marshman et al., 1999; Sung et al., 2000; Weissgold et al., 1995]. Retinal hemorrhages have been reported in rare cases in children after unintentional head injury, such as high-speed motor vehicle accidents, in association with epidural hematoma, and after some falls [Buys et al., 1992; Christian et al., 1999; Elder et al., 1991; Vinchon et al., 2002]. When present in this setting, retinal hemorrhages are typically small and few in number [Betz et al., 1996]. They are not associated with seizures, coughing, or vomiting [Togioka et al., 2009; Sandramouli et al., 1997; Mei-Zahav et al., 2002]. Retinal hemorrhages in the presence of papilledema cannot be causally related to nonaccidental head injury. Finally, retinal hemorrhages are not usually found in the context of acute life-threatening events [Pitetti et al., 2002].
Optic nerve sheath hemorrhages are not specific to inflicted injury, having been reported secondary to sudden or prolonged increases in intracranial pressure (e.g., extensive subarachnoid hemorrhage) and, in rare cases, with unintentional head injury [de Schweinitz and Holoway, 1912; Muller and Deck, 1974; Elner et al., 1990; Munger et al., 1993; Weissgold et al., 1995].
Extensive Subarachnoid Hemorrhage
On occasion, lesions that appear to be acute subdural hematomas resolve rapidly, in 1–2 days [Duhaime et al., 1996]. These may represent predominantly subarachnoid collections. Other extra-axial collections that mimic subdural hematoma include venous epidural collections from contact events [Duhaime et al., 1996]. Among the conditions that can cause subarachnoid hemorrhages are vascular malformations, birth trauma, coagulopathy, meningitis, and unintentional trauma [Cohen et al., 1986; Govaert et al., 1990]. These are uncommon causes of subarachnoid hemorrhages, and, in the presence of extensive subarachnoid bleeding, vascular malformation is the most likely etiology. Vascular malformations, including arteriovenous malformations, dural fistulas, and aneurysms, however, are an exceedingly rare cause of subarachnoid hemorrhage in young children [Crisostomo et al., 1986; Hayashi et al., 1994; Matson, 1965; Matsuzaka et al., 1989; Perret and Nishioka, 1966; Sedzimir, 1955]. Three large series, involving more than 10,000 patients with subarachnoid hemorrhages, identified no children aged 1 year or younger [Matson, 1965; Perret and Nishioka, 1966; Sedzimir, 1955].
Enlarged subarachnoid spaces in infants (also known as benign external hydrocephalus) can be differentiated from subdural hygromas or subdural hematomas on MRI by fluid that matches the signal intensity of cerebrospinal fluid in the ventricles and subarachnoid spaces on all sequences, sulcal prominences, prominent basilar cisterns, and normal or minimally enlarged ventricles [Aoki, 1994; Hellbusch, 2007]. These infants, typically younger than 1 year, present with macrocrania, usually have normal growth and development, and typically have no history of trauma [Mori et al., 1980; Nishimura et al., 1996; Raul et al., 2008; Matsumura and Ito, 1996; Taff et al., 1996; Pascoe et al., 1979; Roberton et al., 1982; Dubowitz and Bross, 1992; Cirak et al., 2004; Chiolero and Berger, 1994; Reincke et al., 1993; Arita et al., 1993; Clifton et al., 1983; Koiv et al., 1997]. Presence of subarachnoid hemorrhages are not a risk factor for subdural bleeding [Raul et al., 2008].
Clinical Assessment
A detailed history of the circumstances of the injury and a timeline of the infant’s behavior for a minimum of the 72 hours preceding the injury is invaluable. This history should be documented in a legible summary, with quotations from caregivers where possible. Histories of minor injury should be closely examined, and any available caretakers should be questioned independently, if at all possible. The biomechanical history of the fall should include details such as the specific surface on to which the child fell, the location and type or types of injury, and the presence of corroborative witnesses [Duhaime et al., 1992]. It is also useful to ask about exactly what happened after the event – “What did the child look like?”, “What position was he or she in?”, “What did he or she do next?”, “What happened then?” – and so on, until the child arrived at a medical center. Also relevant are the age of the child and the baseline gross motor and language development level (e.g., “Can the child crawl, stand, walk, or run?”, “Can the child talk in words?”).
General Examination
After initial coma scale scoring and resuscitative efforts, an extensive physical examination should be completed. Although the Glasgow Coma Score scale is the one most often used, it actually has poor interobserver reliability in infants, even when the modified scoring system for infants is employed (see Chapter 73). The Infant Face Scale is a coma scale designed specifically in an attempt to identify correctly seriously injured infants who have sustained inflicted or other types of traumatic brain injuries, and it has improved interobserver variability in comparison with other impairment scales [Durham et al., 2000].
Serial vital signs, including temperature (rectal preferred), are very helpful in developing a post-injury timeline. Growth parameters, including head circumference, state of hydration, and any obvious signs of neglect, need to be documented. The examiner should palpate and inspect all skin surfaces, particularly the cranium, behind the ears, and the occiput. The presence of otorrhea and rhinorrhea can be easily confirmed as cerebrospinal fluid with a Clinitest® that reveals increased glucose concentration. Battle’s sign (bruising over the mastoid) and “raccoon eyes” (periorbital ecchymoses) are suggestive of a basilar skull fracture. Hemotympanum can result either from direct impact over the ear or from a basilar fracture. The examiner should inspect the frenula and the palate for tears and petechiae; forced feeding, smothering, and gagging can cause these injuries. Any unusual pattern of injuries should be documented (see Figures 75-10 and 75-11).
Classic features of asphyxia are facial cyanosis, petechial hemorrhages of the eyes and face, foam in the nose and mouth, and prominent bulging eyes [Matsumura and Ito, 1996; Taff et al., 1996]. All or some of these features may be present, depending on the amount of force applied to the neck, its rate and duration, and the surface area involved. Decreased carotid pulsations ipsilateral to neck bruising are suggestive of carotid injury. The neck should also be inspected closely, particularly along the mandible, to assess for subtle signs of strangulation.
The examiner should note the location and extent of any skin lesions, including bruises and bite marks. Bruises involving skin surfaces that are fairly protected, such as inner arms and other flexor surfaces, buttocks, and lower back, are of particular concern [Pascoe et al., 1979; Roberton et al., 1982]. A history of a simple fall is incongruous when there are multiple bruises of the same or different age, or if the bruises are on multiple and different body planes. Documentation includes both a complete written record of the examiner’s involvement with the child, and appropriate photographic documentation [Dubowitz and Bross, 1992]. Serial photographs are also very helpful. These should be taken with a color bar and a ruler in the photograph.
Neurologic Examination
Neurologic examinations, including level of coma, should be documented sequentially (see Chapter 73). Serial funduscopic examinations are useful for documenting the final extent of retinal hemorrhage development. Particular attention needs to be given to identifying and documenting asymmetries on the examination. Children younger than 3 years are particularly at risk for spinal cord injury without radiographic findings and if indicated should also be carefully examined for possible spinal cord or vertebral injuries with MRI [Cirak et al., 2004]. The presence of a neurogenic bladder or bowel, or other findings that suggest a spinal level, should raise suspicion of injury.
Autonomic and Neuroendocrine Responses
Traumatic brain injury and other critical illnesses are potent activators of the hypothalamic-pituitary-adrenal axis and the sympathetic efferent pathways [Chiolero and Berger, 1994; Reincke et al., 1993]. As injury severity increases, loss of adaptive negative feedback control mechanisms results in sustained activity of either or both of these pathways [Arita et al., 1993; Clifton et al., 1983; Koiv et al., 1997]. Autonomic cardiac control in children with traumatic brain injury is disrupted in proportion to the severity of injury, with autonomic uncoupling potentiated in young children [Goldstein et al., 1993, 1996; Biswas et al., 2000]. When efferent sympathetic pathways are completely interrupted, heart rate variability is lost and low-frequency heart-rate power decreases; these findings are highly correlated with brain death in both children and adults [Goldstein et al., 1996; Baillard et al., 2002].
After acute severe traumatic brain injury, the majority of children develop elevations of cortisol and adrenocorticotropic hormone (ACTH) that appear to return towards normal over the acute hospital course. A proportion of these evidence disproportionately low levels during this time [Srinivas et al., 2009]. Young infants may develop evidence of hypopituitarism or adrenal insufficiency during their post-injury course. In survivors, abnormalities of the neuroendocrine axis are not uncommon, being reported in upwards of 17 percent, of which a significant number will have multiple abnormalities of pituitary hormones [Poomthavorn et al., 2008]. In adults, Agha and colleagues [2005] followed 50 patients with moderate or severe traumatic brain injury for a year after their injury. Again noted were a significant proportion (16 percent) who showed a subnormal cortisol response acutely, with 4 patients recovering but 5 patients having new endocrine abnormalities discovered at 6 months. Gonadotropins were decreased in the majority (80 percent) acutely, with most of these changes (85 percent) recovered by 12 months. Growth hormone deficiency was found in the acute phase in 18 percent, with 55 percent having growth hormone deficiency at 12 months.
Clinical Laboratory Evaluation
Infants who have sustained inflicted head injuries are frequently anemic and acidotic on admission. Leukocytosis is not uncommon. As in adults, hyperglycemia has been associated with a more severe head injury and a poor prognosis and it is also suggestive of more recent injury [Paret et al., 1999; Melo et al., 2009; Atkinson et al., 1998; Michaud et al., 1991]. Some data suggest that the serum glucose level should be optimally maintained between 100 and 200 mg/dL, which may require an insulin infusion. As in other head-injured populations, secondary consumptive coagulopathy (disseminated intravascular coagulation) is associated with a higher mortality rate and more severe brain injury [Carrick et al., 2005; Hulka et al., 1996]. More commonly, mild elevations of the prothrombin time or evidence of activated coagulation may be found [Hymel et al., 1997]. These abnormalities do not appear to reflect pre-existing coagulation abnormalities. In situations in which a clinical index of suspicion arises or an alternative diagnosis has been proposed, such as an inherited metabolic disease, additional studies or consultation with a metabolic geneticist may be useful.
Biochemical Markers
There is a remarkable body of literature evolving that is studying the acute-phase changes of numerous “biomarkers,” including protein degradation products (spectrin breakdown product, c-tau, amyloid-beta), S-100B, and neuron specific enolase, as well as inflammatory mediators, such as IL-6, IL-8, IL-10, soluble intracellular adhesion molecule, L-selectin, and endothelin, among others [Lo et al., 2009; Kochanek et al., 2008].
The more classic markers (e.g., lactate, S-100B protein, neuron-specific enolase) have been proposed as potential biomarkers of severity of injury in several disorders, including brain injury, stroke, and neonatal encephalopathy [Herrmann et al., 2000; Raabe et al., 1998; O’Regan and Brown, 1998; McKeating et al., 1998]. S-100B (at this point) appears to predict injury and outcome in adults most consistently, whereas neuron specific enolase is also valuable in injury and outcome prediction in children [Beers et al., 2007; Berger et al., 2007; Kovesdi et al., 2010; Fridriksson et al., 2000]. Neuron-specific enolase is a nonspecific indicator of neuronal injury and ischemia, and has been correlated with reversible and irreversible damage after traumatic brain injury, stroke, cardiorespiratory arrest, and perinatal hypoxic-ischemic injury [Herrmann et al., 2000; Raabe et al., 1998; O’Regan and Brown, 1998; McKeating et al., 1998; Fridriksson et al., 2000; Blennow et al., 2001; Correale et al., 1998; Martens et al., 1998]. Transient increases have been documented just before elevations in intracranial pressure, after cardiac surgery, after seizures, and after both convulsive and nonconvulsive status epilepticus [O’Regan and Brown, 1998; Correale et al., 1998; Schmitt et al., 1998; Woertgen et al., 1997].
As with other types of brain injury, numerous post-injury cascades evolve acutely after inflicted head injury. Patterns of inflammatory excitotoxic and oxidative responses after injury are being defined [Shore et al., 2007; Haqqani et al., 2007; Berger et al., 2005, 2006; Vexler and Yenari, 2009; Buttram et al., 2007]. Their utility in discriminating inflicted from unintentional head injury and other brain injuries is not yet clear. What is known is that the immature brain has a more robust inflammatory response compared with adults, and a less robust response to oxidative stress [Robertson et al., 2009; Potts et al., 2006]. In addition, markers of apoptosis are also increased in infants with inflicted brain injury [Satchell et al., 2005].
Radiographic Evaluation
A noncontrast CT scan to include bone windows is the initial imaging study of choice [Bernardi et al., 1993]. A CT scan may miss skull fractures within the plane of the section, so skull films should be considered as part of the skeletal survey. Repeat or serial imaging is often helpful in establishing the extent of injury [Demaerel et al., 2002; Dias et al., 1998; Feldman et al., 1995]. MRI and diffusion-weighted imaging are more sensitive than CT scanning in defining the extent and age of extra-axial collections, hemorrhagic and nonhemorrhagic injury, and evolution of ischemic changes, and in demonstrating infarctions [Ichord et al., 2007; Prasad et al., 2002; Kemp et al., 2009; Biousse et al., 2002; Suh et al., 2001; Ashwal et al., 2010].
MRI is also helpful in delineating intracranial sequelae of abuse. Specific MRI sequences include diffusion-weighted imaging, T2-weighted gradient echo, T2-weighted spin echo, and susceptibility-weighted imaging [Biousse et al., 2002; Suh et al., 2001; Tong et al., 2004; Gerber et al., 2004; Huisman et al., 2003]. If carotid or vertebral injury is suspected, cerebral angiography are useful. Tong and associates have described a high-resolution MRI susceptibility-weighted technique sensitive for detecting hemorrhagic diffuse axonal injury in children [Tong et al., 2004].
Diffusion tensor imaging and (1)H-MR spectroscopy are additional imaging techniques that hold great promise in defining the extent of injury and predicting outcome [Aaen et al., 2010; Ashwal et al., 2006; Babikian et al., 2006; Suskauer and Huisman, 2009]. Aaen et al. studied 90 children with nonaccidental head injury with MR spectroscopy 5 days after injury and found significantly decreased N-acetylaspartate/creatine and N-acetylaspartate/choline ratios; these patients subsequently had poor outcome at follow-up [Aaen et al., 2010]. They developed a logistic regression model using age, initial Glasgow Coma Scale score, presence of retinal hemorrhages, lactate on MR spectroscopy, and mean total N-acetylaspartate/creatine ratios. This model was accurate in predicting outcome 100 percent of the time. More recent studies have also shown that the presence of intraparenchymal brain microhemorrhages, as detected on susceptibility weighted images, correlated with significantly poorer long-term neurologic outcome, and was most predictive when combined with presence of ischemic injury [Colbert et al., 2010].
There is considerable variability in the radiologic interpretation of neuroimaging data [Hymel et al., 1997]. Limited clinicopathologic studies have been published to date, and caution should be exercised in relying only on imaging to establish timing of injury. It is strongly recommended that clinicians review the neuroimaging themselves, in addition to obtaining a radiologist’s interpretation. At this point, there is limited clinical utility in more advanced imaging techniques, such as diffusion tensor imaging.
A full skeletal survey should be obtained in any child younger than 2 years, or in any older child with multiple traumatic injuries suspected to be nonaccidental in origin [Demaerel et al., 2002]. A bone scan may be indicated in equivocal or negative cases with a high level of suspicion; follow-up skeletal survey may also be useful [Howard et al., 1990; Kleinman, 1990; Mandelstam et al., 2003].
Postmortem Examination
A full postmortem examination, including examination of the eyes and testing for metabolic disease, is mandatory for any child with an unexplained death, as these findings frequently assist in establishing an unintentional or inflicted etiology [Case et al., 2001; Bass et al., 1986; Gilliland and Folberg, 1992]. This is particularly true in infants younger than 1 year, where the incidence of sudden infant death syndrome is higher than that of inflicted head injury.
At postmortem examination, evaluation of the reflected scalp allows for identification of galeal hemorrhage and impact sites (periosteal hemorrhages). The temporalis muscle and posterior cervical muscles are additional sites where internal soft tissue injury is found. Small patchy areas of subarachnoid hemorrhages are almost universally found at postmortem examination in infants who die from inflicted injury [Case et al., 2001].
Aside from greatly assisting in documenting the full extent of injuries, the postmortem examination provides additional information about the pathologic timing of injury events. The presence of intra-alveolar siderophages, for instance, is suggestive of previously imposed suffocation [Becroft and Lockett, 1997]. Confirmation of specific findings, such as subdural neomembranes and positive retinal staining for hemosiderin and iron, can further assist in the timing of injuries (Figure 75-18 and 75-19, and see Figure 75-14). In instances in which the infant has been kept alive while brain death and resultant necrosis evolve, however, clinical timing of injury is typically more precise than is timing based on pathologic findings.
Making the Diagnosis of Inflicted Head Injury
General Considerations
Child abuse is rarely premeditated. It occurs when caregivers lose control, often when trying to stop certain behavior (such as crying) or when punishing perceived transgressions (such as toileting accidents). Although many inflicted injuries are one-time events, infants and children may have been subjected to repeated and complex injury scenarios occurring during a single day, or as a series of events over days, weeks, or months [Gilles and Nelson, 1998; Weston, 1973; Alexander et al., 1990]. The sequence of injury events is highly variable. Injury events can include shaking, throwing, hitting, slapping, gagging, strangulation, and smothering [Gilles and Nelson, 1998; Duhaime et al., 1987; Bonnier et al., 1995].
Only children with injuries severe enough to frighten the caregiver are likely to be brought to medical attention. Resuscitation delay is also a potential factor, although not a uniform finding or unique to abuse [Adelson et al., 1997]. Presumably, resuscitation delay accentuates neuronal and biochemical ischemic cascades, which contribute to the severity of secondary neuronal injury [Gilles and Nelson, 1998; Goldstein et al., 1993; Johnson et al., 1995].
Establishing the diagnosis of inflicted trauma is frequently problematic and requires a high index of suspicion. The fewer markers of injury and the more nonspecific the history, the more difficult it is to distinguish inflicted from unintentional injury [Morris et al., 2000]. The less severe the injury, the less pronounced the postconcussive syndrome.
Children interface with the medical system at all points along their response curve after brain injury. It is unrealistic to expect findings in all children to be similar. Certain key questions should be asked and answered (Box 75-4). The physical findings, in conjunction with inconsistent historical features, usually point to trauma as the most likely etiology. Obvious cases are those with clear and convincing findings in the absence of an injury mechanism that accounts for the findings. The combination of acute serious brain injury, retinal hemorrhages, and posterior interhemispheric subdural hematomas appear to have greater specificity for an inflicted etiology, but are not unique to inflicted injury.
Evaluating the History
Histories provided to clinicians typically minimize actual injury events. Most often, there is either a history of minor trauma (such as a short fall with no neurologic symptoms) or no history at all [Weston, 1973; Shugerman et al., 1996; Chadwick et al., 1991; Dykes, 1986]. Changing histories, either by the same caregiver or by multiple caregivers, should also raise concerns. Typically, the actual injury and timeline of those events are understated, whereas descriptions of the clinical behaviors of the infant are accurate [Gilles and Nelson, 1998; Hettler and Greenes, 2003]. There are a number of clues that suggest an inflicted etiology (Box 75-5) [Goldstein et al., 1993; Bechtel et al., 2004; Tzioumi and Oates, 1998; Hettler and Greenes, 2003; Duhaime et al., 1998].
Timing
The timing of injury events in the still-living child can usually be estimated by using a combination of clinical course, physical findings, and imaging data, although best estimates are often insufficiently precise to eliminate all possible perpetrators. Children who sustain moderate to severe traumatic brain injury are almost always immediately symptomatic, often demonstrating an immediate apneustic response to mechanical events, whether abused or not [Gilles and Nelson, 1998; Nashelsky and Dix, 1995; Willman et al., 1997; Zuccarello et al., 1985]. Persistence of coma, apnea or respiratory distress, irritability, seizures, hypotonia, and vomiting reflects the severity of injury and evolving secondary pathophysiologic cascades [Aldrich et al., 1992; Gilles and Nelson, 1998; Goldstein et al., 1993; Johnson et al., 1995; Zuccarello et al., 1985].
Lucid Interval
The lucid interval occurs after an initial loss of consciousness. It consists of a period of clinical improvement, followed by deterioration within minutes to hours. Lucid intervals occur in less than 3 percent of children after moderate to severe traumatic brain injury of all etiologies, and generally imply a nonlethal injury if prompt care can be delivered [Arbogast et al., 2005; Willman et al., 1997; Bruce and Zimmerman, 1989; Hahn et al., 1988]. In Hahn et al., only 2.2 percent of 738 children aged 0–16 years with head injuries (318 less than 36 months) had a lucid interval [Hahn et al., 1988]. Bruce et al. detailed the temporal course of the lucid interval in 23 patients [Bruce and Zimmerman, 1989]. Eight children with Glasgow Coma Scale scores above 8 had a variable period of consciousness and speech, following the progressive loss of speech and decreased motor activity, but none progressed to coma. All 15 children with initial Glasgow Coma Scale scores of less than 8 were unconscious following trauma, then had a period of improved consciousness, followed by rapid secondary deterioration within minutes to hours. In a study by Willman et al. of 95 accidental childhood head injury fatalities, all but one child was obviously seriously injured from the time of injury until death [Willman et al., 1997]. Only one child (who had an epidural hematoma) had a lucid interval. Typically, these children do well with aggressive supportive care.
Dating by Neuroimaging
Establishing the timing of an injury on neuroimaging findings alone is difficult. Dias and associates found that the timing of injury could be determined by imaging findings to a reasonable certainty in only 50 percent of infants in a series of 33 infants with inflicted head injury [Dias et al., 1998].
Brain swelling may develop quite rapidly, even within 20–30 minutes, or more slowly [Dias et al., 1998; Gean, 1994; Kobrine et al., 1977; Waga et al., 1979]. Well-developed parenchymal abnormalities were already present on admission CT scans in nine infants whose injury had occurred within the previous 3 hours, which supports findings in the literature that evolution of cerebral parenchymal abnormalities occurs rapidly after injury [Duhaime et al., 1993; Dias et al., 1998; Steinbok et al., 2007; Lobato et al., 1988]. In children dying from accidental head injury, Willman and associates found that head CT scans revealed cerebral hypodensity as early as 1 hour 17 minutes after injury [Willman et al., 1997].
Imaging criteria for the dating of subdural hematoma are discussed in detail elsewhere [Gean, 1994]. In general, hyperdense subdural collections on noncontrast CT imaging are less than 7 days old; isointense collections, 7–14 days old; and hypodense collections, more than 14 days old. Subdural neomembranes, visualized with contrast CT scans, begin to develop between 7 and 10 days after injury. Subdural hematomas may appear hypodense in the very anemic infant or if there is a mixture of cerebrospinal fluid with subdural blood. The degree to which the subdural membrane has consolidated at postmortem examination and the evolution of the subdural membrane are helpful in pinpointing the age of the collection. MRI, like CT scanning, cannot date injuries precisely, and the finding of blood of different intensities does not always mean that there has been repeated trauma, in as much as blood in various compartments may have different signal characteristics.
Medicolegal Issues
Child abuse and neglect were defined by the Federal Child Abuse Prevention and Treatment Act (CAPTA) 42 U.S.C.A. §5106g, and subsequently amended by the Keeping Children and Families Safe Act of 2003 [http://www.acf.hhs.gov/programs/cb/pubs/cm07/cm07.pdf].
Mandated reporting laws require physicians and other health-care professionals to report patterns of injuries and behavior when reasonable suspicion of abuse or neglect arises. Reasonable suspicion does not mean that the clinician must be certain of the diagnosis; it means only that the clinical picture warrants full investigation and protection of the injured child and any siblings. Health-care providers actually fail to diagnose or misdiagnose about one-third of inflicted head injuries in infants and children during initial examinations, especially when injuries are not severe [Rubin et al., 2003; Jenny et al., 1999].
The law protects the physician if suspicion should prove erroneous but was made in good faith. Conversely, failure to report both is actionable and places children at significant risk for further harm if they are returned to the setting in which they were injured [Rubin et al., 2003]. Although there is a cross-reporting mandate between social service and law enforcement officials, it may not happen immediately. When serious physical injury is suspected to have resulted from assault, notification of both law enforcement and social services is recommended.
Translating and interpreting complex information from the medical to the legal arena is a critical component of the evaluation of suspected inflicted head injury for the protection of the child, other siblings, and the alleged perpetrator [Chadwick and Krous, 1997]. This process begins with adequate written, photographic, and schematic documentation, and extends through communication with Child Protective Services, law enforcement agencies, legal counsel, and the judicial system. Investigating agencies want treating clinicians to know whether the injuries were inflicted or not, but the clinician is sometimes unable to say more than that the injuries are clearly traumatic in origin. If there is uncertainty about the significance or timing of particular findings, an expert opinion, usually from a child abuse team physician, neurosurgeon, child neurologist, or forensic neuropathologist with expertise in this area is often helpful. Ultimately, the diagnosis of inflicted injury relies on the constellation of physical findings, a history of injury that is biomechanically feasible, and the timing of injury. It is imperative to have either the children’s services worker or law enforcement complete a scene investigation, obtaining photographs, again with tape measures, as soon as a fall history is given. Optimally, these data will be reviewed by the clinicians who are determining if the clinical and radiological findings are consistent or inconsistent with the given history.
References
The complete list of references for this chapter is available online at www.expertconsult.com.
Aaen G.S., Holshouser B.A., Sheridan C., et al. Magnetic resonance spectroscopy predicts outcomes for children with nonaccidental trauma. Pediatrics. 2010;125:295-303.
Adams J., Graham D., Murray L., et al. Diffuse axonal injury due to nonmissile head injury in humans: an analysis of 45 cases. Ann Neurol. 1982;12:557.
Adams J., Mitchell D., Graham D. Diffuse brain damage of immediate impact type. Brain. 1977;100:489-502.
Adelson P.D., Clyde B., Kochanek P.M., et al. Cerebrovascular response in infants and young children following severe traumatic brain injury: a preliminary report. Pediatr Neurosurg. 1997;26:200-207.
Agha A., Phillips J., O’Kelly P., et al. The natural history of post-traumatic hypopituitarism: implications for assessment and treatment. Am J Med. 2005;118(12):1416.
Aldrich E., Eisenberg H., Saydjari C., et al. Diffuse brain swelling in severely head-injured children. A report from the NIH Traumatic Coma Data Bank. J Neurosurg. 1992;76:450-454.
Alexander R., Crabbe L., Sato Y., et al. Serial abuse in children who are shaken. Am J Dis Child. 1990;144:58-60.
Anton J., Pineda V., Martin C., et al. Posttraumatic subgaleal hematoma: a case report and review of the literature. Pediatr Emerg Care. 1999;15:347-349.
Aoki N. Accidental head trauma and retinal hemorrhage. Neurosurgery. 1994;34:771.
Arbogast K.B., Margulies S.S., Christian C.W. Initial neurologic presentation in young children sustaining inflicted and unintentional fatal head injuries. Pediatrics. 2005;116:180-184.
Arita K., Uozumi T., Oki S., et al. The function of the hypothalamic-pituitary-adrenal axis in brain dead patients. Acta Neurochir (Wien). 1993;123:64-75.
Arlotti S.A., Forbes B.J., Dias M.S., et al. Unilateral retinal hemorrhages in shaken baby syndrome. J AAPOS. 2007;11:175-178.
Arnholz D., Hymel K.P., Hay T.C., et al. Bilateral pediatric skull fractures: accident or abuse? J Trauma. 1998;45:172-174.
Ashwal S., Holshouser B.A., Hinshaw D.B.Jr, et al. Proton magnetic resonance spectroscopy in the evaluation of children with congenital heart disease and acute central nervous system injury. J Thorac Cardiovasc Surg. 1996;112:403-414.
Ashwal S., Holshouser B.A., Shu S.K., et al. Predictive value of proton magnetic resonance spectroscopy in pediatric closed head injury. Pediatr Neurol. 2000;23:114-125.
Ashwal S., Holshouser B.A., Tong K.A. Use of advanced neuroimaging techniques in the evaluation of pediatric traumatic brain injury. Dev Neurosci. 2006;28:309-326.
Ashwal S., Perkin R.M., Thompson J.R., et al. CBF and CBF/PCO2 reactivity in childhood strangulation. Pediatr Neurol. 1991;7:369-374.
Ashwal S., Wycliffe N.D., Holshouser B.A. Advanced neuroimaging in children with nonaccidental trauma. Dev Neurosci. 2010;32(5–6):343-346.
Ates O., Ondul S., Onal C., et al. Post-traumatic early epilepsy in pediatric age group with emphasis on influential factors. Childs Nerv Syst. 2006;22:279-284.
Atkinson J., Anderson R.E., Murray M.J. The early critical phase of severe head injury: importance of apnea and dysfunctional respiration. J Trauma. 1998;45:941-945.
Atkinson J.L. The neglected prehospital phase of head injury: apnea and catecholamine surge. Mayo Clin Proc. 2000;75:37-47.
Atwal G.S., Rutty G.N., Carter N., et al. Bruising in non-accidental head injured children; a retrospective study of the prevalence, distribution and pathological associations in 24 cases. Forensic Sci Int. 1998;96:215-230.
Azzarelli B., Caldemeyer K.S., Phillips J.P., et al. Hypoxic-ischemic encephalopathy in areas of primary myelination: a neuroimaging and PET study. Pediatr Neurol. 1996;14:108-116.
Babikian T., Freier M.C., Ashwal S., et al. MR spectroscopy: predicting long-term neuropsychological outcome following pediatric TBI. J Magn Reson Imaging. 2006;24:801-811.
Babikian T., Freier M.C., Tong K.A., et al. Susceptibility weighted imaging: neuropsychologic outcome and pediatric head injury. Pediatr Neurol. 2005;33:184-194.
Baechli H., Behzad M., Schreckenberger M., et al. Blood constituents trigger brain swelling, tissue death, and reduction of glucose metabolism early after acute subdural hematoma in rats. J Cereb Blood Flow Metab. 2010;30:576-585.
Baillard C., Vivien B., Mansier P., et al. Brain death assessment using instant spectral analysis of heart rate variability. Crit Care Med. 2002;30:306-310.
Banaszkiewicz P.A., Scotland T.R., Myerscough E.J. Fractures in children younger than age 1 year: importance of collaboration with child protection services. J Pediatr Orthop. 2002;22:740-744.
Barlow K.M., Minns R.A. The relation between intracranial pressure and outcome in non-accidental head injury. Dev Med Child Neurol. 1999;41:220-225.
Barlow K.M., Spowart J.J., Minns R.A. Early posttraumatic seizures in non-accidental head injury: relation to outcome. Dev Med Child Neurol. 2000;42:591-594.
Barlow K.M., Thomson E., Johnson D., et al. Late neurologic and cognitive sequelae of inflicted traumatic brain injury in infancy. Pediatrics. 2005;116:e174-e185.
Bass M., Kravath R.E., Glass L. Death-scene investigation in sudden infant death. N Engl J Med. 1986;315:100-105.
Bauer R., Fritz H. Pathophysiology of traumatic injury in the developing brain: an introduction and short update. Exp Toxicol Pathol. 2004;56:65-73.
Baum J.D., Bulpitt C.J. Retinal and conjunctival haemorrhage in the newborn. Arch Dis Child. 1970;45:344-349.
Bechtel K., Stoessel K., Leventhal J.M., et al. Characteristics that distinguish accidental from abusive injury in hospitalized young children with head trauma. Pediatrics. 2004;114:165-168.
Becroft D., Lockett B. Intra-alveolar pulmonary siderophages in sudden infant death: a marker for previous imposed suffocation. Pathology. 1997;29:60.
Beers S.R., Berger R.P., Adelson P.D. Neurocognitive outcome and serum biomarkers in inflicted versus non-inflicted traumatic brain injury in young children. J Neurotrauma. 2007;24:97-105.
Berger R.P., Adelson P.D., Pierce M.C., et al. Serum neuron-specific enolase, S100B, and myelin basic protein concentrations after inflicted and noninflicted traumatic brain injury in children. J Neurosurg. 2005;103:61-68.
Berger R.P., Adelson P.D., Richichi R., et al. Serum biomarkers after traumatic and hypoxemic brain injuries: insight into the biochemical response of the pediatric brain to inflicted brain injury. Dev Neurosci. 2006;28:327-335.
Berger R.P., Beers S.R., Richichi R., et al. Serum biomarker concentrations and outcome after pediatric traumatic brain injury. J Neurotrauma. 2007;24:1793-1801.
Bernardi B., Zimmerman R.A., Bilaniuk L.T. Neuroradiologic evaluation of pediatric craniocerebral trauma. Top Magn Reson Imaging. 1993;5:161-173.
Betz P., Puschel K., Miltner E., et al. Morphometrical analysis of retinal hemorrhages in the shaken baby syndrome. Forensic Sci Int. 1996;78:71-80.
Billmire M.E., Myers P.A. Serious head injury in infants: accident or abuse? Pediatrics. 1985;75:340-342.
Binenbaum G., Mirza-George N., Christian C.W., et al. Odds of abuse associated with retinal hemorrhages in children suspected of child abuse. J AAPOS. 2009;13:268-272.
Biousse V., Suh D.Y., Newman N.J., et al. Diffusion-weighted magnetic resonance imaging in Shaken Baby Syndrome. Am J Ophthalmol. 2002;133:249-255.
Bird R., McMahan J.R., Gilles F.H., et al. Strangulation in child abuse: CT diagnosis? Radiology. 1987;175:373-375.
Biron D.L., Shelton D. Functional time limit and onset of symptoms in infant abusive head trauma. J Paediatr Child Health. 2007;43:60-65.
Biswas A.K., Scott W.A., Sommerauer J.F., et al. Heart rate variability after acute traumatic brain injury in children. Crit Care Med. 2000;28:3907-3912.
Bittigau P., Sifringer M., Felderhoff-Mueser U., et al. Neuropathological and biochemical features of traumatic injury in the developing brain. Neurotox Res. 2003;5:475-490.
Blennow M., Savman K., Ilves P., et al. Brain-specific proteins in the cerebrospinal fluid of severely asphyxiated newborn infants. Acta Paediatr. 2001;90:1171-1175.
Bonnier C., Nassogne M.-C., Evrard P. Outcome and prognosis of whiplash shaken infant syndrome; late consequences after a symptom free interval. Dev Med Child Neurol. 1995;37:943-956.
Bonnier C., Nassogne M.C., Saint-Martin C., et al. Neuroimaging of intraparenchymal lesions predicts outcome in shaken baby syndrome. Pediatrics. 2003;112:808-814.
Brennan L.K., Rubin D., Christian C.W., et al. Neck injuries in young pediatric homicide victims. J Neurosurg Pediatr. 2009;3:232-239.
Brinker T., Ludemann W., Berens von Rautenfeld D., et al. Breakdown of the meningeal barrier surrounding the intraorbital optic nerve after experimental subarachnoid hemorrhage. Am J Ophthalmol. 1997;124:373-380.
Brismar J., Ozand P.T.. CT and MR of the brain in the diagnosis of organic acidemias. Experiences from 107 patients. Brain Dev. 1994(16 Suppl):104-124.
Brody B., Kinney H., Kloman A., et al. Sequence of central nervous system myelination in human infancy. I. An autopsy study of myelination. J Neuropathol Exp Neurol. 1987;46:282.
Bruce D.A., Zimmerman R.A. Shaken impact syndrome. Pediatr Ann. 1989;18:482-484. 486–489, 492–494
Budenz D.L., Farber M.G., Mirchandani H.G., et al. Ocular and optic nerve hemorrhages in abused infants with intracranial injuries. Ophthalmology. 1994;101:559-565.
Buttram S.D., Wisniewski S.R., Jackson E.K., et al. Multiplex assessment of cytokine and chemokine levels in cerebrospinal fluid following severe pediatric traumatic brain injury: effects of moderate hypothermia. J Neurotrauma. 2007;24:1707-1717.
Buys Y.M., Levin A.V., Enzenauer R.W., et al. Retinal findings after head trauma in infants and young children. Ophthalmology. 1992;99:1718-1723.
Caffey J. Multiple fractures in long bones of infants suffering from chronic subdural hematoma. Am J Roentgenol. 1946;56(2):163-173.
Caffey J. On the theory and practice of shaking infants. Its potential residual effects of permanent brain damage and mental retardation. Am J Dis Child. 1972;124:161-169.
Caffey J. The whiplash shaken infant syndrome: manual shaking by the extremities with whiplash-induced intracranial and intraocular bleedings, linked with residual permanent brain damage and mental retardation. Pediatrics. 1974;54:396-403.
Calder I.M., Hill I., Scholtz C.L. Primary brain trauma in non-accidental injury. J Clin Pathol. 1984;37:1095-1100.
Carraro M.C., Rossetti L., Gerli G.C. The irreplaceable image: An unexpected manifestation of anemia. Haematologica. 2001;86:672.
Carrick M.M., Tyroch A.H., Youens C.A., et al. Subsequent development of thrombocytopenia and coagulopathy in moderate and severe head injury: support for serial laboratory examination. J Trauma. 2005;58:725-729. discussion 729–730
Carter J., McCormick A. Whiplash shaking syndrome: retinal hemorrhages and computerized axial tomography of the brain. Child Abuse Negl. 1983;7:279-286.
Case M.E. Abusive head injuries in infants and young children. Leg Med (Tokyo). 2007;9:83-87.
Case M.E. Accidental traumatic head injury in infants and young children. Brain Pathol. 2008;18:583-589.
Case M.E. Inflicted traumatic brain injury in infants and young children. Brain Pathol. 2008;18:571-582.
Case M.E., Graham M.A., Handy T.C., et al. Position paper on fatal abusive head injuries in infants and young children. Am J Forensic Med Pathol. 2001;22:112-122.
Chadwick D., Chin S., Salerno C., et al. Deaths from falls in children: how far is fatal. J Trauma. 1991;31:1353.
Chadwick D., Krous H. Irresponsible testimony by medical experts in cases involving the physical abuse and neglect of children. Child Maltreat. 1997;2:313.
Chadwick D.L., Bertocci G., Castillo E., et al. Annual risk of death resulting from short falls among young children: less than 1 in 1 million. Pediatrics. 2008;121:1213-1224.
Chadwick D.L., Chin S., Salerno C., et al. Deaths from falls in children: how far is fatal? J Trauma. 1991;31:1353-1355.
Chaiwat O., Sharma D., Udomphorn Y., et al. Cerebral hemodynamic predictors of poor 6-month Glasgow Outcome Score in severe pediatric traumatic brain injury. J Neurotrauma. 2009;26:657-675.
Chesnut R.M., Marshall L.F., Klauber M.R., et al. The role of secondary brain injury in determining outcome from severe head injury. J Trauma. 1993;34:216-222.
Chiaretti A., Barone G., Riccardi R., et al. NGF, DCX, and NSE upregulation correlates with severity and outcome of head trauma in children. Neurology. 2009;72:609-616.
Chiaretti A., De Benedictis R., Polidori G., et al. Early post-traumatic seizures in children with head injury. Childs Nerv Syst. 2000;16:862-866.
Chiaretti A., Piastra M., Pulitano S., et al. Prognostic factors and outcome of children with severe head injury: an 8-year experience. Childs Nerv Syst. 2002;18:129-136.
Chiaviello C.T., Christoph R.A., Bond G.R. Infant walker-related injuries: a prospective study of severity and incidence. Pediatrics. 1994;93:974-976.
Chiesa A., Duhaime A.C. Abusive head trauma. Pediatr Clin North Am. 2009;56:317-331.
Chiolero R., Berger M. Endocrine response to brain injury. New Horiz. 1994;2:432-442.
Christian C.W., Block R. Abusive head trauma in infants and children. Pediatrics. 2009;123:1409-1411.
Christian C.W., Taylor A.A., Hertle R.W., et al. Retinal hemorrhages caused by accidental household trauma. J Pediatr. 1999;135:125-127.
Cirak B., Ziegfeld S., Knight V.M., et al. Spinal injuries in children. J Pediatr Surg. 2004;39:607-612.
Clifton G., Robertson C., Kyper K., et al. Cardiovascular response to severe head injury. J Neurosurg. 1983;59:447-454.
Cochran A., Scaife E.R., Hansen K.W., et al. Hyperglycemia and outcomes from pediatric traumatic brain injury. J Trauma. 2003;55:1035-1038.
Cohen R.A., Kaufman R.A., Myers P.A., et al. Cranial computed tomography in the abused child with head injury. Am J Roentgenol. 1986;146:97-102.
Colbert C.A., Holshouser B.A., Aaen G.S., et al. Value of Cerebral Microhemorrhages Detected with Susceptibility-weighted MR Imaging for Prediction of Long-term Outcome in Children with Nonaccidental Trauma. Radiology. 2010;256(3):898-905.
Correale J., Rabinowicz A., Heck C., et al. Status epilepticus increases CSF levels of neuron-specific enolase and alters the blood-brain barrier. Neurology. 1998;50:1388-1391.
Courville C. Contrecoup injuries of the brain in infancy: remarks on the mechanism of fatal traumatic lesions of early life. Arch Surg. 1965;90:157.
Crisostomo E.A., Leaton E., Rosenblum E.L. Features of intracranial aneurysms in infants and report of a case. Dev Med Child Neurol. 1986;28:68-72.
Crume T.L., DiGuiseppi C., Byers T., et al. Underascertainment of child maltreatment fatalities by death certificates, 1990-1998. Pediatrics. 2002;110:e18.
Datta S., Stoodley N., Jayawant S., et al. Neuroradiological aspects of subdural haemorrhages. Arch Dis Child. 2005;90:947-951.
Demaerel P., Casteels I., Wilms G. Cranial imaging in child abuse. Eur Radiol. 2002;12:849-857.
Demissie K., Rhoads G.G., Smulian J.C., et al. Operative vaginal delivery and neonatal and infant adverse outcomes: population based retrospective analysis. BMJ. 2004;329:24-29.
Denny-Brown D., Russell W. Experimental cerebral concussion. Brain. 1941;64:93.
de Schweinitz G., Holoway T. Fracture of the skull with hemorrhage into the optic nerve-sheaths and retinas: microscopic examination of the eyeballs. Trans Am Ophthal Soc. 1912;13:120-128.
Diamond P., Hansen C.M., Christofersen M.R. Child abuse presenting as a thoracolumbar spinal fracture dislocation: a case report. Pediatr Emerg Care. 1994;10:83-86.
Dias M.S., Backstrom J., Falk M., et al. Serial radiography in the infant shaken impact syndrome. Pediatr Neurosurg. 1998;29:77-85.
Diaz-Arrastia R., Agostini M.A., Madden C.J., et al. Posttraumatic epilepsy: the endophenotypes of a human model of epileptogenesis. Epilepsia. 2009;50(Suppl 2):14-20.
Digraham D., Ford I., Adams J., et al. Fatal head injury in children. J Clin Pathol. 1989;42:18.
Docherty E., Hassan A., Burke D. Things that go bump … bump … bump: an analysis of injuries from falling down stairs in children based at Sheffield Children’s Hospital. Emerg Med J. 2010;27:207-208.
Drack A.V., Petronio J., Capone A. Unilateral retinal hemorrhages in documented cases of child abuse. Am J Ophthalmol. 1999;128:340-344.
Drigo P., Piovan S., Battistella P.A., et al. Macrocephaly, subarachnoid fluid collection, and glutaric aciduria type I. J Child Neurol. 1996;11:414-417.
Dubowitz H., Bross D.C. The pediatrician’s documentation of child maltreatment. Am J Dis Child. 1992;146:596-599.
Duhaime A., Balaniuk L., Zimmerman R. The “big black brain”: radiographic changes after severe inflicted head injury in infancy. J Neurotrauma. 1993;10(Suppl 1):S59.
Duhaime A.C., Alario A.J., Lewander W.J., et al. Head injury in very young children: mechanisms, injury types, and ophthalmologic findings in 100 hospitalized patients younger than 2 years of age. Pediatrics. 1992;90:179-185.
Duhaime A.C., Christian C., Armonda R., et al. Disappearing subdural hematomas in children. Pediatr Neurosurg. 1996;25:116-122.
Duhaime A.C., Christian C., Moss E., et al. Long-term outcome in infants with the shaking-impact syndrome. Pediatr Neurosurg. 1996;24:292-298.
Duhaime A.C., Christian C.W., Rorke L.B., et al. Nonaccidental head injury in infants – the “shaken-baby syndrome”. N Engl J Med. 1998;338:1822-1829.
Duhaime A.C., Durham S. Traumatic brain injury in infants: the phenomenon of subdural hemorrhage with hemispheric hypodensity (“Big Black Brain”). Prog Brain Res. 2007;161:293-302.
Duhaime A.C., Gennarelli T.A., Thibault L.E., et al. The shaken baby syndrome. A clinical, pathological, and biomechanical study. J Neurosurg. 1987;66:409-415.
Duhaime A.-C., Hunter J., Grate L., et al. Magnetic resonance imaging studies of age-dependent responses to scaled focal brain injury in the piglet. J Neurosurg. 2003;99:542-548.
Durham S.R., Clancy R.R., Leuthardt E., et al. CHOP Infant Coma Scale (“Infant Face Scale”): a novel coma scale for children less than two years of age. J Neurotrauma. 2000;17:729-737.
Dykes L.J. The whiplash shaken infant syndrome: what has been learned? Child Abuse Negl. 1986;10:211-221.
Eder H.G., Legat J.A., Gruber W. Traumatic brain stem lesions in children. Childs Nerv Syst. 2000;16:21-24.
Egge K., Lyng G., Maltau J.M. Retinal haemorrhages in the newborn. Acta Ophthalmol (Copenh). 1980;58:231-236.
Egge K., Lyng G., Maltau J.M. Effect of instrumental delivery on the frequency and severity of retinal hemorrhages in the newborn. Acta Obstet Gynecol Scand. 1981;60:153-155.
Ehrenfest H. Birth Injuries of the Child. New York: Appleton and Co, 1922.
Eisenberg H.M., Gary H.E.Jr, Aldrich E.F., et al. Initial CT findings in 753 patients with severe head injury. A report from the NIH Traumatic Coma Data Bank. J Neurosurg. 1990;73:688-698.
Eisenbrey A.B. Retinal hemorrhage in the battered child. Childs Brain. 1979;5:40-44.
Elder J.E., Taylor R.G., Klug G.L. Retinal haemorrhage in accidental head trauma in childhood. J Paediatr Child Health. 1991;27:286-289.
Elner S.G., Elner V.M., Arnall M., et al. Ocular and associated systemic findings in suspected child abuse. A necropsy study. Arch Ophthalmol. 1990;108:1094-1101.
Emanuelson I. How safe are childcare products, toys and playground equipment? A Swedish analysis of mild brain injuries at home and during leisure time 1998–1999. Inj Control Saf Promot. 2003;10:139-144.
Emerson M.V., Jakobs E., Green W.R. Ocular autopsy and histopathologic features of child abuse. Ophthalmology. 2007;114:1384-1394.
Emerson M.V., Pieramici D.J., Stoessel K.M., et al. Incidence and rate of disappearance of retinal hemorrhage in newborns. Ophthalmology. 2001;108:36-39.
Ewing-Cobbs L., Kramer L., Prasad M., et al. Neuroimaging, physical, and developmental findings after inflicted and noninflicted traumatic brain injury in young children. Pediatrics. 1998;102:300-307.
Ewing-Cobbs L., Prasad M., Kramer L., et al. Inflicted traumatic brain injury: relationship of developmental outcome to severity of injury. Pediatr Neurosurg. 1999;31:251-258.
Ewing-Cobbs L., Prasad M., Kramer L., et al. Acute neuroradiologic findings in young children with inflicted or noninflicted traumatic brain injury. Childs Nerv Syst. 2000;16:25-33. discussion 34
Fackler J.C., Berkowitz I.D., Green W.R. Retinal hemorrhages in newborn piglets following cardiopulmonary resuscitation. Am J Dis Child. 1992;146:1294-1296.
Feldman K.W., Avellino A.M., Sugar N.F., et al. Cervical spinal cord injury in abused children. Pediatr Emerg Care. 2008;24:222-227.
Feldman K.W., Bethel R., Shugerman R.P., et al. The cause of infant and toddler subdural hemorrhage: a prospective study. Pediatrics. 2001;108:756-846.
Feldman K.W., Brewer D.K., Shaw D.W. Evolution of the cranial computed tomography scan in child abuse. Child Abuse Negl. 1995;19:307-314.
Fernando S., Obaldo R.E., Walsh I.R., et al. Neuroimaging of nonaccidental head trauma: pitfalls and controversies. Pediatr Radiol. 2008;38:827-838.
Firsching R., Schutze M., Motschmann M., et al. Venous opthalmodynamometry: a noninvasive method for assessment of intracranial pressure. J Neurosurg. 2000;93:33-36.
Foerster B.R., Petrou M., Lin D., et al. Neuroimaging evaluation of non-accidental head trauma with correlation to clinical outcomes: a review of 57 cases. J Pediatr. 2009;154:573-577.
Forbes B.J., Cox M., Christian C.W. Retinal hemorrhages in patients with epidural hematomas. J AAPOS. 2008;12:177-180.
Frauenberger G., Lis E. Multiple fractures associated with subdural hematomas in infancy. Pediatrics. 1950;6:890.
Freeman S.S., Udomphorn Y., Armstead W.M., et al. Young age as a risk factor for impaired cerebral autoregulation after moderate to severe pediatric traumatic brain injury. Anesthesiology. 2008;108:588-595.
Fridriksson T., Kini N., Walsh-Kelly C., et al. Serum neuron-specific enolase as a predictor of intracranial lesions in children with head trauma: a pilot study. Acad Emerg Med. 2000;7:816-820.
Fujiwara T., Okuyama M., Miyasaka M. Characteristics that distinguish abusive from nonabusive head trauma among young children who underwent head computed tomography in Japan. Pediatrics. 2008;122:e841-e847.
Gago L.C., Wegner R.K., Capone A.Jr, et al. Intraretinal hemorrhages and chronic subdural effusions: glutaric aciduria type 1 can be mistaken for shaken baby syndrome. Retina. 2003;23:724-726.
Galaznik J.G. Optic nerve sheath hemorrhages, increased intracranial pressure, and retinal hemorrhages in central nervous system trauma. Arch Ophthalmol. 2009;127:346-347.
Galloway N.R., Tong K.A., Ashwal S., et al. Diffusion-weighted imaging improves outcome prediction in pediatric traumatic brain injury. J Neurotrauma. 2008;25:1153-1162.
Ganesh A., Jenny C., Geyer J., et al. Retinal hemorrhages in type I osteogenesis imperfecta after minor trauma. Ophthalmology. 2004;111:1428-1431.
Gaynon M.W., Koh K., Marmor M.F., et al. Retinal folds in the shaken baby syndrome. Am J Ophthalmol. 1988;106:423-425.
Gean A. Imaging of Head Trauma. New York: Raven Press, 1994.
Geddes J.F., Hackshaw A.K., Vowles G.H., et al. Neuropathology of inflicted head injury in children. I. Patterns of brain damage. Brain. 2001;124:1290-1298.
Geddes J.F., Vowles G.H., Hackshaw A.K., et al. Neuropathology of inflicted head injury in children. II. Microscopic brain injury in infants. Brain. 2001;124:1299-1306.
Geeraerts T., Merceron S., Benhamou D., et al. Non-invasive assessment of intracranial pressure using ocular sonography in neurocritical care patients. Intensive Care Med. 2008;34:2062-2067.
Gennarelli T.A. Cerebral concussion and diffuse brain injuries. In: Cooper P.R., editor. Head Injury. Baltimore: Williams & Wilkins, 1993.
Gennarelli T., Thibault L., Adams J., et al. Diffuse axonal injury and traumatic coma in the primate. Ann Neurol. 1982;12:564-574.
Gentry L.R., Godersky J.C., Thompson B. MR imaging of head trauma: review of the distribution and radiopathologic features of traumatic lesions. Am J Roentgenol. 1988;150:675-772.
Gerber D.J., Weintraub A.H., Cusick C.P., et al. Magnetic resonance imaging of traumatic brain injury: relationship of T2 SE and T2GE to clinical severity and outcome. Brain Inj. 2004;18:1083-1097.
Gerlach R., Dittrich S., Schneider W., et al. Traumatic epidural hematomas in children and adolescents: outcome analysis in 39 consecutive unselected cases. Pediatr Emerg Care. 2009;25:164-169.
Ghahreman A., Bhasin V., Chaseling R., et al. Nonaccidental head injuries in children: a Sydney experience. J Neurosurg. 2005;103:213-218.
Ghatan S., Ellenbogen R.G. Pediatric spine and spinal cord injury after inflicted trauma. Neurosurg Clin N Am. 2002;13:227-233.
Giangiacomo J., Khan J.A., Levine C., et al. Sequential cranial computed tomography in infants with retinal hemorrhages. Ophthalmology. 1988;95:295-299.
Gill J.R., Goldfeder L.B., Armbrustmacher V., et al. Fatal head injury in children younger than 2 years in New York City and an overview of the shaken baby syndrome. Arch Pathol Lab Med. 2009;133:619-627.
Gilles E.E., McGregor M.L., Levy-Clarke G. Retinal hemorrhage asymmetry in inflicted head injury: a clue to pathogenesis? J Pediatr. 2003;143:494-499.
Gilles E.E., Nelson M.D.Jr. Cerebral complications of nonaccidental head injury in childhood. Pediatr Neurol. 1998;19:119-128.
Gilliland M.G., Folberg R. Retinal hemorrhages: replicating the clinician’s view of the eye. Forensic Sci Int. 1992;56:77-80.
Gilliland M.G., Luckenbach M.W. Are retinal hemorrhages found after resuscitation attempts? A study of the eyes of 169 children. Am J Forensic Med Pathol. 1993;14:187-192.
Gilliland M.G., Luckenbach M.W., Chenier T.C. Systemic and ocular findings in 169 prospectively studied child deaths: retinal hemorrhages usually mean child abuse. Forensic Sci Int. 1994;68:117-132.
Gilliland M., Luckenbach M.W. Are retinal hemorrhages found after resuscitation attempts. A study of the eyes of 169 children. Am J Forensic Med Pathol. 1993;14:187-192.
Gilmore J.H., Lin W., Corouge I., et al. Early postnatal development of corpus callosum and corticospinal white matter assessed with quantitative tractography. Am J Neuroradiol. 2007;28:1789-1795.
Giza C.C., Mink R.B., Madikians A. Pediatric traumatic brain injury: not just little adults. Curr Opin Crit Care. 2007;13:143-152.
Golden N., Maliawan S. Clinical analysis of non-accidental head injury in infants. J Clin Neurosci. 2005;12:235-239.
Goldstein B., DeKing D., DeLong D.J., et al. Autonomic cardiovascular state after severe brain injury and brain death in children. Crit Care Med. 1993;21:228-233.
Goldstein B., Fiser D., Kelly M., et al. Decomplexification in critical illness and injury: relationship between heart rate variability, severity of illness, and outcome. Crit Care Med. 1998;26:352-357.
Goldstein B., Kelly M.M., Bruton D., et al. Inflicted versus accidental head injury in critically injured children. Crit Care Med. 1993;21:1328-1332.
Goldstein B., Kempski M.H., DeKing D., et al. Autonomic control of heart rate after brain injury in children. Crit Care Med. 1996;24:234-240.
Govaert P., Van De Velde E., Vanhaesebrouck P., et al. CT diagnosis of neonatal subarachnoid hemorrhage. Pediatr Radiol. 1990;20:139-142.
Govind A., Kumari S., Lath N.K. Retinal hemorrhages in newborn. Indian Pediatr. 1989;26:150-152.
Graham D.I., Ford I., Adams J.H., et al. Fatal head injury in children. J Clin Pathol. 1989;42:18-22.
Greenberg J., Cohen W.A., Cooper P.R. The “hyperacute” extraaxial intracranial hematoma: computed tomographic findings and clinical significance. Neurosurgery. 1985;17:48-56.
Greenes D.S., Schutzman S.A. Occult intracranial injury in infants. Ann Emerg Med. 1998;32:680-686.
Greenwald M.J., Weiss A., Oesterle C.S., et al. Traumatic retinoschisis in battered babies. Ophthalmology. 1986;93:618-625.
Guthkelch A. Infantile subdural hematoma and its relationship to whiplash injuries. Br Med J. 1971;2:430.
Hagberg H. Mitochondrial impairment in the developing brain after hypoxia-ischemia. J Bioenerg Biomembr. 2004;36:369-373.
Hahn Y.S., Chyung C., Barthel M.J., et al. Head injuries in children under 36 months of age. Demography and outcome. Childs Nerv Syst. 1988;4:34-40.
Hahn Y.S., Raimondi A.J., McLone D.G., et al. Traumatic mechanisms of head injury in child abuse. Childs Brain. 1983;10:229-241.
Hamlin H. Subgaleal hematoma caused by hair pull. JAMA. 1968;204:339.
Hammoud D.A., Wasserman B.A. Diffuse axonal injuries: pathophysiology and imaging. Neuroimaging Clin N Am. 2002;12:205-216.
Haqqani A.S., Hutchison J.S., Ward R., et al. Biomarkers and diagnosis; protein biomarkers in serum of pediatric patients with severe traumatic brain injury identified by ICAT-LC-MS/MS. J Neurotrauma. 2007;24:54-74.
Hasegawa M., Yamashima T., Yamashima J., et al. Traumatic subdural hygroma: pathology and meningeal enhancement on magnetic resonance imaging. Neurosurg. 1992;31:580.
Haviland J., Russell R.I. Outcome after severe non-accidental head injury. Arch Dis Child. 1997;77:504-507.
Hayashi N., Endo S., Oka N., et al. Intracranial hemorrhage due to rupture of an arteriovenous malformation in a full-term neonate. Childs Nerv Syst. 1994;10:344-346.
Hayashi T., Hashimoto T., Fukuda S., et al. Neonatal subdural hematoma secondary to birth injury. Clinical analysis of 48 survivors. Childs Nerv Syst. 1987;3:23-29.
Hedges T.R., Weinstein J.D., Crystle C.D. Orbital vascular response to acutely increased intracranial pressure in the rhesus monkey. Arch Ophthalmol. 1964;71:226-237.
Hellbusch L.C. Benign extracerebral fluid collections in infancy: clinical presentation and long-term follow-up. J Neurosurg. 2007;107:119-125.
Herlenius E., Lagercrantz H. Development of neurotransmitter systems during critical periods. Exp Neurol. 2004;190(Suppl 1):8-21.
Herr S., Pierce M.C., Berger R.P., et al. Does valsalva retinopathy occur in infants? An initial investigation in infants with vomiting caused by pyloric stenosis. Pediatrics. 2004;113:1658-1661.
Herrmann M., Jost S., Kutz S., et al. Temporal profile of release of neurobiochemical markers of brain damage after traumatic brain injury is associated with intracranial pathology as demonstrated in cranial computerized tomography. J Neurotrauma. 2000;17:113-122.
Hettler J., Greenes D.S. Can the initial history predict whether a child with a head injury has been abused? Pediatrics. 2003;111:602-607.
Hicks R.A., Gaughan D.C. Understanding fatal child abuse. Child Abuse Negl. 1995;19:855-875.
Hiss J., Kahana T. The medicolegal implications of bilateral cranial fractures in infants. J Trauma. 1995;38:32-34.
Hobbs C. Skull fracture and the diagnosis of abuse. Arch Dis Child. 1984;59:246-252.
Holloway M., Bye A.M., Moran K. Non-accidental head injury in children. Med J Aust. 1994;160:786-789.
Hossain M.A. Molecular mediators of hypoxic-ischemic injury and implications for epilepsy in the developing brain. Epilepsy Behav. 2005;7:204-213.
Howard J.L., Barron B.J., Smith G.G. Bone scintigraphy in the evaluation of extraskeletal injuries from child abuse. Radiographics. 1990;10:67-81.
Huisman T.A., Sorensen A.G., Hergan K., et al. Diffusion-weighted imaging for the evaluation of diffuse axonal injury in closed head injury. J Comput Assist Tomogr. 2003;27:5-11.
Hulka F., Mullins R.J., Frank E.H. Blunt brain injury activates the coagulation process. Arch Surg. 1996;131:923-927. discussion 927–928
Hymel K.P., Abshire T.C., Luckey D.W., et al. Coagulopathy in pediatric abusive head trauma. Pediatrics. 1997;99:371-375.
Hymel K.P., Makoroff K.L., Laskey A.L., et al. Mechanisms, clinical presentations, injuries, and outcomes from inflicted versus noninflicted head trauma during infancy: results of a prospective, multicentered, comparative study. Pediatrics. 2007;119:922-929.
Hymel K.P., Rumack C.M., Hay T.C., et al. Comparison of intracranial computed tomographic (CT) findings in pediatric abusive and accidental head trauma. Pediatr Radiol. 1997;27:743-747.
Ichord R.N., Naim M., Pollock A.N., et al. Hypoxic-ischemic injury complicates inflicted and accidental traumatic brain injury in young children: the role of diffusion-weighted imaging. J Neurotrauma. 2007;24:106-118.
Irazuzta J., McJunkin J., Danadian K., et al. Outcome and cost of child abuse. Child Abuse Negl. 1997;21:751-757.
Jaspan T., Narborough G., Punt J.A., et al. Cerebral contusional tears as a marker of child abuse – detection by cranial sonography. Pediatr Radiol. 1992;22:237-245.
Jayakumar P., Barry M., Ramachandran M. Orthopaedic aspects of paediatric non-accidental injury. J Bone Joint Surg Br. 2010;92:189-195.
Jenny C., Hymel K.P., Ritzen A., et al. Analysis of missed cases of abusive head trauma. JAMA. 1999;281:621-626.
Joffe M., Ludwig S. Stairway injuries in children. Pediatrics. 1988;82:457-459.
Johnson D.L., Boal D., Baule R. Role of apnea in nonaccidental head injury. Pediatr Neurosurg. 1995;23:305-310.
Johnson D.L., Braun D., Friendly D. Accidental head trauma and retinal hemorrhage. Neurosurgery. 1993;33:231-234. discussion 234–235
Johnston M. Developmental aspects of epileptogenesis. Epilepsia. 1996;37(Suppl 1):S2-S9.
Johnston M.V. Neurotransmitters and vulnerability of the developing brain. Brain Dev. 1995;17:301-306.
Kaufman H.H. Traumatic subdural hygroma: pathology and meningeal enhancement on magnetic resonance imaging. Neurosurgery. 1993;32:149.
Kazan S., Tuncer R., Karasoy M., et al. Post-traumatic bilateral diffuse cerebral swelling. Acta Neurochir (Wien). 1997;139:295-301. discussion 302
Keane J.R. Retinal hemorrhages. Its significance in 100 patients with acute encephalopathy of unknown cause. Arch Neurol. 1979;36:691-694.
Keenan H.T., Hooper S.R., Wetherington C.E., et al. Neurodevelopmental consequences of early traumatic brain injury in 3-year-old children. Pediatrics. 2007;119:e616-e623.
Keenan H.T., Runyan D.K., Marshall S.W., et al. A population-based study of inflicted traumatic brain injury in young children. JAMA. 2003;290:621-626.
Keenan H.T., Runyan D.K., Nocera M. Child outcomes and family characteristics 1 year after severe inflicted or noninflicted traumatic brain injury. Pediatrics. 2006;117:317-324.
Kemp A.M., Dunstan F., Harrison S., et al. Patterns of skeletal fractures in child abuse: systematic review. BMJ. 2008;337:a1518.
Kemp A.M., Rajaram S., Mann M., et al. What neuroimaging should be performed in children in whom inflicted brain injury (iBI) is suspected? A systematic review. Clin Radiol. 2009;64:473-483.
Kemp A.M., Stoodley N., Cobley C., et al. Apnoea and brain swelling in non-accidental head injury. Arch Dis Child. 2003;88:472-476. discussion 476
Kempe C., Silverman F., Steele B., et al. The battered child syndrome. JAMA. 1962;181:17.
Kessler D.B., Siegel-Stein F. Retinal hemorrhage, meningitis, and child abuse. N Y State J Med. 1984;84:59-60.
Kimura S., Hara M., Nezu A., et al. Two cases of glutaric aciduria type 1: clinical and neuropathological findings. J Neurol Sci. 1994;123:38-43.
Kivlin J.D. Manifestations of the shaken baby syndrome. Curr Opin Ophthalmol. 2001;12:158-175.
Kivlin J.D., Currie M.L., Greenbaum V.J., et al. Retinal hemorrhages in children following fatal motor vehicle crashes: a case series. Arch Ophthalmol. 2008;126:800-804.
Kleinman P. Diagnostic imaging in infant abuse. Am J Radiology. 1990;155:703-712.
Kobrine A.I., Timmins E., Rajjoub R.K., et al. Demonstration of massive traumatic brain swelling within 20 minutes after injury. Case report. J Neurosurg. 1977;46:256-258.
Kochanek P.M., Berger R.P., Bayir H., et al. Biomarkers of primary and evolving damage in traumatic and ischemic brain injury: diagnosis, prognosis, probing mechanisms, and therapeutic decision making. Curr Opin Crit Care. 2008;14:135-141.
Koiv L., Merisalu E., Zilmer K., et al. Changes in sympatho-adrenal and hypothalamic-pituitary-adrenocortical system in patients with head injury. Acta Neurol Scand. 1997;96:52-58.
Kovesdi E., Luckl J., Bukovics P., et al. Update on protein biomarkers in traumatic brain injury with emphasis on clinical use in adults and pediatrics. Acta Neurochir (Wien). 2010;152:1-17.
Krugman R.D. Fatal child abuse: analysis of 24 cases. Pediatrician. 1983;12:68-72.
Kubova H., Moshe S.L. Experimental models of epilepsy in young animals. J Child Neurol. 1994;9(Suppl 1):S3-S11.
Kuppermann N. Intracranial injury in minor head trauma. Arch Dis Child. 2004;89:593-594.
Kuppermann N., Holmes J.F., Dayan P.S., et al. Identification of children at very low risk of clinically-important brain injuries after head trauma: a prospective cohort study. Lancet. 2009;374:1160-1170.
Land J., Goulder P., Johnson A., et al. Glutaric aciduria type 1: an atypical presentation together with some observations upon treatment and the possible cause of cerebral damage. Neuropediatrics. 1992;23:322.
Laraque D. Injuries associated with infant walkers. Pediatrics. 2001;108:790-792.
Latronico N., Marino R. Posttraumatic cerebral infarction (PTCI) in patients with severe head trauma. J Trauma. 2009;66:1745-1746.
Launay F., Leet A.I., Sponseller P.D. Pediatric spinal cord injury without radiographic abnormality: a meta-analysis. Clin Orthop Relat Res. 2005:166-170.
Law A.J., Weickert C.S., Webster M.J., et al. Expression of NMDA receptor NR1, NR2A and NR2B subunit mRNAs during development of the human hippocampal formation. Eur J Neurosci. 2003;18:1197-1205.
Lea PMt, Faden A.I. Traumatic brain injury: developmental differences in glutamate receptor response and the impact on treatment. Ment Retard Dev Disabil Res Rev. 2001;7:235-248.
Levin A.V. Ophthalmology of shaken baby syndrome. Neurosurg Clin N Am. 2002;13:201-211. vi
Levin A.V. Retinal hemorrhages: advances in understanding. Pediatr Clin North Am. 2009;56:333-344.
Lewis R.J., Yee L., Inkelis S.H., et al. Clinical predictors of post-traumatic seizures in children with head trauma. Ann Emerg Med. 1993;22:1114-1118.
Libby A.M., Sills M.R., Thurston N.K., et al. Costs of childhood physical abuse: comparing inflicted and unintentional traumatic brain injuries. Pediatrics. 2003;112:58-65.
Lindenberg R. Mechanical injuries of brain and meninges. In: Spitz W.U., Fisher R.S., editors. Medicolegal investigation of death: Guidelines for the application of pathology to crime investigation. Springfield, Illinois: Charles C Thomas; 1973:420-469.
Lindenberg R., Freytag E. Morphology of brain lesions from blunt trauma in early infancy. Arch Pathol. 1969;87:298.
Lobato R. Post-traumatic brain swelling. Adv Tech Stand Neurosurg. 1993;20:3-38.
Lobato R.D., Sarabia R., Cordobes F., et al. Posttraumatic cerebral hemispheric swelling. Analysis of 55 cases studied with computerized tomography. J Neurosurg. 1988;68:417-423.
Lo T.Y., Jones P.A., Minns R.A. Pediatric brain trauma outcome prediction using paired serum levels of inflammatory mediators and brain-specific proteins. J Neurotrauma. 2009;26:1479-1487.
Lo T.Y., McPhillips M., Minns R.A., et al. Cerebral atrophy following shaken impact syndrome and other non-accidental head injury (NAHI). Pediatr Rehabil. 2003;6:47-55.
Lyle D.J., Stapp J.P., Button R.R. Ophthalmologic hydrostatic pressure syndrome. Am J Ophthalmol. 1957;44:652-657.
Lyons T.J., Oates R.K. Falling out of bed: a relatively benign occurrence. Pediatrics. 1993;92:125-127.
Mandelstam S.A., Cook D., Fitzgerald M., et al. Complementary use of radiological skeletal survey and bone scintigraphy in detection of bony injuries in suspected child abuse. Arch Dis Child. 2003;88:387-390. discussion 390
Marino R., Gasparotti R., Pinelli L., et al. Posttraumatic cerebral infarction in patients with moderate or severe head trauma. Neurology. 2006;67:1165-1171.
Marin-Padilla M., Parisi J.E., Armstrong D.L., et al. Shaken infant syndrome: developmental neuropathology, progressive cortical dysplasia, and epilepsy. Acta Neuropathol (Berl). 2002;103:321-332.
Marshman W.E., Adams G.G., Ohri R. Bilateral vitreous hemorrhages in an infant with low fibrinogen levels. J AAPOS. 1999;3:255-256.
Martens P., Raabe A., Johnsson P. Serum S-100 and neuron-specific enolase for prediction of regaining consciousness after global cerebral ischemia. Stroke. 1998;29:2375-2376.
Marton E., Mazzucco M., Nascimben E., et al. Severe head injury in early infancy: analysis of causes and possible predictive factors for outcome. Childs Nerv Syst. 2007;23:873-880.
Massicotte S.J., Folberg R., Torczynski E., et al. Vitreoretinal traction and perimacular retinal folds in the eyes of deliberately traumatized children. Ophthalmology. 1991;98:1124-1127.
Matson D. Intracranial arterial aneurysms in children. J Neurosurg. 1965;23:578.
Matsumura F., Ito Y. Petechial hemorrhage of the conjunctiva and histological findings of the lung and pancreas in infantile asphyxia – evaluation of 85 cases. Kurume Med J. 1996;43:259-266.
Matsuyama T., Shimomura T., Okumura Y., et al. Acute subdural hematomas due to rupture of cortical arteries: a study of the points of rupture in 19 cases. Surg Neurol. 1997;47:423-427.
Matsuzaka T., Yoshinaga M., Tsuji Y., et al. Incidence and causes of intracranial hemorrhage in infancy: a prospective surveillance study after vitamin K prophylaxis. Brain Dev. 1989;11:384-388.
Matthews G.P., Das A. Dense vitreous hemorrhages predict poor visual and neurological prognosis in infants with shaken baby syndrome. J Pediatr Ophthalmol Strabismus. 1996;33:260-265.
McCabe C.F., Donahue S.P. Prognostic indicators for vision and mortality in shaken baby syndrome. Arch Ophthalmol. 2000;118:373-377.
McDonald J.W., Trescher W.H., Johnston M.V. Susceptibility of brain to AMPA induced excitotoxicity transiently peaks during early postnatal development. Brain Res. 1992;583:54-70.
McKeating E.G., Andrews P.J., Mascia L. Relationship of neuron specific enolase and protein S-100 concentrations in systemic and jugular venous serum to injury severity and outcome after traumatic brain injury. Acta Neurochir Suppl (Wien). 1998;71:117-119.
McLaurine R., Tutor F. Acute subdural hematoma. A review of ninety cases. J Neurosurg. 1961;18:61.
Medele R.J., Stummer W., Mueller A.J., et al. Terson’s syndrome in subarachnoid hemorrhage and severe brain injury accompanied by acutely raised intracranial pressure. J Neurosurg. 1998;88:851-854.
Mei-Zahav M., Uziel Y., Raz J., et al. Convulsions and retinal haemorrhage: should we look further? Arch Dis Child. 2002;86:334-335.
Melo J.R., Di Rocco F., Lemos-Junior L.P., et al. Defenestration in children younger than 6 years old: mortality predictors in severe head trauma. Childs Nerv Syst. 2009;25:1077-1083.
Mendelsohn D.B., Levin H.S., Harward H., et al. Corpus callosum lesions after closed head injury in children: MRI, clinical features and outcome. Neuroradiology. 1992;34:384-388.
Meservy C.J., Towbin R., McLaurin R.L., et al. Radiographic characteristics of skull fractures resulting from child abuse. Am J Roentgenol. 1987;149:173-175.
Metzger J.C., Eastman A.L., Pepe P.E. Year in review 2008: Critical Care – trauma. Crit Care. 2009;13:226.
Michaud L.J., Rivara F.P., Longstreth W.T.Jr, et al. Elevated initial blood glucose levels and poor outcome following severe brain injuries in children. J Trauma. 1991;31:1356-1362.
Miller J.D., Bullock R., Graham D.I., et al. Ischemic brain damage in a model of acute subdural hematoma. Neurosurgery. 1990;27:433-439.
Mills M. Funduscopic lesions associated with mortality in shaken baby syndrome. J AAPOS. 1998;2:67-71.
Mirvis S., Wolf A., Numaguchi Y., et al. Posttraumatic cerebral infarction diagnosed by CT: prevalence, origin, and outcome. Am J Neuroradiol. 1990;11:355.
Missios S., Harris B.T., Dodge C.P., et al. Scaled cortical impact in immature swine: effect of age and gender on lesion volume. J Neurotrauma. 2009;26:1943-1951.
Morad Y., Kim Y.M., Armstrong D.C., et al. Correlation between retinal abnormalities and intracranial abnormalities in the shaken baby syndrome. Am J Ophthalmol. 2002;134:354-359.
Moretti R., Pizzi B. Optic nerve ultrasound for detection of intracranial hypertension in intracranial hemorrhage patients: confirmation of previous findings in a different patient population. J Neurosurg Anesthesiol. 2009;21:16-20.
Mori K., Handa H., Itoh M., et al. Benign subdural effusion in infants. J Comput Assist Tomogr. 1980;4:466-471.
Morris M.W., Smith S., Cressman J., et al. Evaluation of infants with subdural hematoma who lack external evidence of abuse. Pediatrics. 2000;105:549-553.
Muller P.J., Deck J.H.N. Intraocular and optic nerve sheath hemorrhage in cases of sudden intracranial hypertension. J Neurosurg. 1974;41:160-166.
Munger C.E., Peiffer R.L., Bouldin T.W., et al. Ocular and associated neuropathologic observations in suspected whiplash shaken infant syndrome. A retrospective study of 12 cases. Am J Forensic Med Pathol. 1993;14:193-200.
Musemeche C.A., Barthel M., Cosentino C., et al. Pediatric falls from heights. J Trauma. 1991;31:1347-1349.
Myhre M.C., Grogaard J.B., Dyb G.A., et al. Traumatic head injury in infants and toddlers. Acta Paediatr. 2007;96:1159-1175.
Nadebaum C., Anderson V., Catroppa C. Executive function outcomes following traumatic brain injury in young children: a five year follow-up. Dev Neuropsychol. 2007;32:703-728.
Nashelsky M.B., Dix J.D. The time interval between lethal infant shaking and onset of symptoms. A review of the shaken baby syndrome literature. Am J Forensic Med Pathol. 1995;16:154-157.
Nassogne M.C., Sharrard M., Hertz-Pannier L., et al. Massive subdural haematomas in Menkes disease mimicking shaken baby syndrome. Childs Nerv Syst. 2002;18:729-731.
Nimityongskul P., Anderson L.D. The likelihood of injuries when children fall out of bed. J Pediatr Orthop. 1987;7:184-186.
Nishimura K., Mori K., Sakamoto T., et al. Management of subarachnoid fluid collection in infants based on a long-term follow-up study. Acta Neurochir (Wien). 1996;138:179-184.
Novack T.A., Dillon M.C., Jackson W.T. Neurochemical mechanisms in brain injury and treatment: a review. J Clin Exp Neuropsychol. 1996;18:685-706.
Odom A., Christ E., Kerr N., et al. Prevalence of retinal hemorrhages in pediatric patients after in-hospital cardiopulmonary resuscitation: a prospective study. Pediatrics. 1997;99:E3.
O’Leary J.A., Ferrell R.E., Randolph C.R. Retinal hemorrhage and vacuum extraction delivery. J Perinat Med. 1986;14:197-199.
Ommaya A., Gennarelli T. Cerebral concussion and traumatic unconsciousness. Correlation of experimental and clinical observations on blunt head injuries. Brain. 1974;97:753-754.
O’Regan M.E., Brown J.K. Serum neuron specific enolase: a marker for neuronal dysfunction in children with continuous EEG epileptiform activity. Eur J Paediatr Neurol. 1998;2:193-197.
Orlin J.R., Zwetnow N.N., Bjorneboe A. Changes in CSF pressures during experimental acute arterial subdural bleeding in pig. Acta Neurochir (Wien). 1992;118:146-158.
Osaka H., Kimura S., Nezu A., et al. Chronic subdural hematoma, as an initial manifestation of glutaric aciduria type-1. Brain Dev. 1993;15:125-127.
Paradis N., Martin G., Goetting M., et al. Simultaneous aortic, jugular bulb, and right atrial pressures during cardiopulmonary resuscitation in humans. Insights into mechanisms. Circulation. 1989;80:361.
Paret G., Tirosh R., Lotan D., et al. Early prediction of neurological outcome after falls in children: metabolic and clinical markers. J Accid Emerg Med. 1999;16:186-188.
Pascoe J.M., Hildebrandt H.M., Tarrier A., et al. Patterns of skin injury in nonaccidental and accidental injury. Pediatrics. 1979;64:245-247.
Paviglianiti J.C., Donahue S.P. Unilateral retinal hemorrhages and ipsilateral intracranial bleeds in nonaccidental trauma. J AAPOS. 1999;3:383-384.
Perez-Arjona E., Dujovny M., DelProposto Z., et al. Late outcome following central nervous system injury in child abuse. Childs Nerv Syst. 2003;19:69-81.
Perret G., Nishioka H. Report on the cooperative study of intracranial aneurysms and subarachnoid hemorrhage. IV. Cerebral angiography. An analysis of the diagnostic value and complications of carotid and vertebral angiography in 5,484 patients. J Neurosurg. 1966;25:98.
Peters M.L., Starling S.P., Barnes-Eley M.L., et al. The presence of bruising associated with fractures. Arch Pediatr Adolesc Med. 2008;162:877-881.
Pfenninger J., Santi A. Severe traumatic brain injury in children – are the results improving? Swiss Med Wkly. 2002;132:116-120.
Pierre-Kahn V., Roche O., Dureau P., et al. Ophthalmologic findings in suspected child abuse victims with subdural hematomas. Ophthalmology. 2003;110:1718-1723.
Pigula F., Wald S., Shackford S., et al. The effect of hypotension and hypoxia on children with severe head injuries. J Pediatr Surg. 1993;28:310-316.
Pitetti R.D., Maffei F., Chang K., et al. Prevalence of retinal hemorrhages and child abuse in children who present with an apparent life-threatening event. Pediatrics. 2002;110:557-562.
Pollanen M.S., Smith C.R., Chiasson D.A., et al. Fatal child abuse-maltreatment syndrome. A retrospective study in Ontario, Canada, 1990-1995. Forensic Sci Int. 2002;126:101-104.
Poomthavorn P., Maixner W., Zacharin M. Pituitary function in paediatric survivors of severe traumatic brain injury. Arch Dis Child. 2008;93:133-137.
Potts M.B., Koh S.E., Whetstone W.D., et al. Traumatic injury to the immature brain: inflammation, oxidative injury, and iron-mediated damage as potential therapeutic targets. NeuroRx. 2006;3:143-153.
Pouwels P.J., Brockmann K., Kruse B., et al. Regional age dependence of human brain metabolites from infancy to adulthood as detected by quantitative localized proton MRS. Pediatr Res. 1999;46:474-485.
Prange M.T., Coats B., Duhaime A.C., et al. Anthropomorphic simulations of falls, shakes, and inflicted impacts in infants. J Neurosurg. 2003;99:143-150.
Prasad M.R., Ewing-Cobbs L., Swank P.R., et al. Predictors of outcome following traumatic brain injury in young children. Pediatr Neurosurg. 2002;36:64-74.
Pryds A., Tonnesen J., Pryds O., et al. Cerebral pressure autoregulation and vasoreactivity in the newborn rat. Pediatr Res. 2005;57:294-298.
Raabe A., Grolms C., Keller M., et al. Correlation of computed tomography findings and serum brain damage markers following severe head injury. Acta Neurochir (Wien). 1998;140:787-791. discussion 791–792
Raghupathi R., Mehr M.F., Helfaer M.A., et al. Traumatic axonal injury is exacerbated following repetitive closed head injury in the neonatal pig. J Neurotrauma. 2004;21:307-316.
Ranjith R.K., Mullett J.H., Burke T.E. Hangman’s fracture caused by suspected child abuse. A case report. J Pediatr Orthop B. 2002;11:329-332.
Ransom G.H., Mann F.A., Vavilala M.S., et al. Cerebral infarct in head injury: relationship to child abuse. Child Abuse Negl. 2003;27:381-392.
Rao N., Smith R.E., Choi J.H., et al. Autopsy findings in the eyes of fourteen fatally abused children. Forensic Sci Int. 1988;39:293-299.
Rao P., Carty H., Pierce A. The acute reversal sign: comparison of medical and non-accidental injury patients. Clin Radiol. 1999;54:495-501.
Raul J.S., Roth S., Ludes B., et al. Influence of the benign enlargement of the subarachnoid space on the bridging veins strain during a shaking event: a finite element study. Int J Legal Med. 2008;122:337-340.
Reece R.M., Sege R. Childhood head injuries: accidental or inflicted? Arch Pediatr Adolesc Med. 2000;154:11-15.
Reiber G.D. Fatal falls in childhood. How far must children fall to sustain fatal head injury? Report of cases and review of the literature. Am J Forensic Med Pathol. 1993;14:201-207.
Reincke M., Allolio B., Wurth G., et al. The hypothalamic-pituitary-adrenal axis in critical illness: response to dexamethasone and corticotropin-releasing hormone. J Clin Endocrinol Metab. 1993;77:151-156.
Rice D., Barone S.Jr. Critical periods of vulnerability for the developing nervous system: evidence from humans and animal models. Environ Health Perspect. 2000;108(Suppl 3):511-533.
Ridenour M.V. Ages of young children who fall down stairs. Percept Mot Skills. 1999;88:669-675.
Riffenburgh R.S., Sathyavagiswaran L. Ocular findings at autopsy of child abuse victims. Ophthalmology. 1991;98:1519-1524.
Rivara J.M., Jaffe K.M., Polissar N.L., et al. Predictors of family functioning and change 3 years after traumatic brain injury in children. Arch Phys Med Rehabil. 1996;77:754-764.
Roberton D.M., Barbor P., Hull D. Unusual injury? Recent injury in normal children and children with suspected non-accidental injury. Br Med J (Clin Res Ed). 1982;285:1399-1401.
Robertson C.L., Scafidi S., McKenna M.C., et al. Mitochondrial mechanisms of cell death and neuroprotection in pediatric ischemic and traumatic brain injury. Exp Neurol. 2009;218:371-380.
Robertson C.L., Soane L., Siegel Z.T., et al. The potential role of mitochondria in pediatric traumatic brain injury. Dev Neurosci. 2006;28:432-446.
Rooks V.J., Sisler C., Burton B. Cervical spine injury in child abuse: report of two cases. Pediatr Radiol. 1998;28:193-195.
Roshkow J.E., Haller J.O., Hotson G.C., et al. Imaging evaluation of children after falls from a height: review of 45 cases. Radiology. 1990;175:359-375.
Rossen R., Kabat H., Anderson J. Acute arrest of cerebral circulation in man. Arch Neurol Psych. 1943;50:510.
Rubin D.M., Christian C.W., Bilaniuk L.T., et al. Occult head injury in high-risk abused children. Pediatrics. 2003;111:1382-1386.
Ruppel R.A., Clark R.S., Bayir H., et al. Critical mechanisms of secondary damage after inflicted head injury in infants and children. Neurosurg Clin N Am. 2002;13:169-182. v
Sandramouli S., Robinson R., Tsaloumas M., et al. Retinal haemorrhages and convulsions. Arch Dis Child. 1997;76:449-451.
Sarabia R., Lobato R., Rivas J., et al. Cerebral hemisphere swelling in severe head injury patients. Acta Neurochir. 1988;42(Suppl):40.
Sargent S., Kennedy J.G., Kaplan J.A. “Hyperacute” subdural hematoma: CT mimic of recurrent episodes of bleeding in the setting of child abuse. J Forensic Sci. 1996;41:314-316.
Sasaki-Adams D., Kulkarni A., Rutka J., et al. Neurosurgical implications of osteogenesis imperfecta in children. Report of 4 cases. J Neurosurg Pediatr. 2008;1:229-236.
Satchell M.A., Lai Y., Kochanek P.M., et al. Cytochrome c, a biomarker of apoptosis, is increased in cerebrospinal fluid from infants with inflicted brain injury from child abuse. J Cereb Blood Flow Metab. 2005;25:919-927.
Sawauchi S., Marmarou A., Beaumont A., et al. Acute subdural hematoma associated with diffuse brain injury and hypoxemia in the rat: effect of surgical evacuation of the hematoma. J Neurotrauma. 2004;21:575-673.
Schloff S., Mullaney P.B., Armstrong D.C., et al. Retinal findings in children with intracranial hemorrhage. Ophthalmology. 2002;109:1472-1476.
Schmitt B., Bauersfeld U., Schmid E.R., et al. Serum and CSF levels of neuron-specific enolase (NSE) in cardiac surgery with cardiopulmonary bypass: a marker of brain injury? Brain Dev. 1998;20:536-539.
Schroder M.L., Muizelaar J.P., Kuta A.J. Documented reversal of global ischemia immediately after removal of an acute subdural hematoma. Report of two cases. J Neurosurg. 1994;80:324-327.
Schutzman S., Barnes P., Mantello M., et al. Epidural hematomas in children. Ann Emerg Med. 1993;22:535.
Sedzimir C. Head injury as a cause of internal carotid thrombosis. J Neurol Neurosurg Psychiatry. 1955;18:293.
Server A., Dullerud R., Haakonsen M., et al. Post-traumatic cerebral infarction. Neuroimaging findings, etiology and outcome. Acta Radiol. 2001;42:254-260.
Sezen F. Retinal haemorrhages in newborn infants. Br J Ophthalmol. 1971;55:248-253.
Shaikh S., Fishman M.L., Gaynon M., et al. Diffuse unilateral hemorrhagic retinopathy associated with accidental perinatal strangulation. A clinicopathologic report. Retina. 2001;21:252-255.
Shane S.A., Fuchs S.M. Skull fractures in infants and predictors of associated intracranial injury. Pediatr Emerg Care. 1997;13:198-203.
Shapiro K., Smith L. Special considerations for the pediatric age group. In Cooper P., editor: Head Injury, ed 3, Baltimore: Williams & Wilkins, 1993.
Shaw H.E.Jr, Landers M.B., Sydnor C.F. The significance of intraocular hemorrhages due to subarachnoid hemorrhage. Ann Ophthalmol. 1977;9:1403-1405.
Shenkin H. Acute subdural hematoma. Review of 39 consecutive cases with high incidence of cortical artery rupture. J Neurosurg. 1982;57:254.
Shim Y.S., Park C.O., Hyun D.K., et al. What are the causative factors for a slow, progressive enlargement of a chronic subdural hematoma? Yonsei Med J. 2007;48:210-217.
Shore P.M., Berger R.P., Varma S., et al. Cerebrospinal fluid biomarkers versus glasgow coma scale and glasgow outcome scale in pediatric traumatic brain injury: the role of young age and inflicted injury. J Neurotrauma. 2007;24:75-86.
Shugerman R.P., Paez A., Grossman D.C., et al. Epidural hemorrhage: is it abuse? Pediatrics. 1996;97:664-668.
Sigmund G.A., Tong K.A., Nickerson J.P., et al. Multimodality comparison of neuroimaging in pediatric traumatic brain injury. Pediatr Neurol. 2007;36:217-226.
Silverman F. The roentgen manifestation of unrecognized skeletal trauma in infants. Am J Roentgenol Radium Ther Nucl Med. 1952;69:413.
Singer R.B. Incidence of seizures after traumatic brain injury – a 50-year population survey. J Insur Med. 2001;33:42-45.
Smith G.A., Bowman M.J., Luria J.W., et al. Babywalker-related injuries continue despite warning labels and public education. Pediatrics. 1997;100:E1.
Srinivas R., Brown S.D., Chang Y.F., et al. Endocrine function in children acutely following severe traumatic brain injury. Childs Nerv Syst. 2009.
Stålhammer D.A.. Experimental models of head injury. Neurochirurgica. 1986(Suppl 36):33-46.
Starling S.P., Patel S., Burke B.L., et al. Analysis of perpetrator admissions to inflicted traumatic brain injury in children. Arch Pediatr Adolesc Med. 2004;158:454-458.
Steinbok P., Singhal A., Poskitt K., et al. Early hypodensity on computed tomographic scan of the brain in an accidental pediatric head injury. Neurosurgery. 2007;60:689-694. discussion 694–695
Steinborn M., Schaffeler C., Kabs C., et al. CT and MR imaging of primary cerebrovascular complications in pediatric head trauma. Emerg Radiol. 2010;17(4):309-315.
Strauss K.A., Puffenberger E.G., Robinson D.L., et al. Type I glutaric aciduria, part 1: natural history of 77 patients. Am J Med Genet C Semin Med Genet. 2003;121C:38-52.
Strich S. Shearing of nerve fibers as a cause of brain damage due to head injury. Lancet. 1961;2:443.
Suh D.Y., Davis P.C., Hopkins K.L., et al. Nonaccidental pediatric head injury: diffusion-weighted imaging findings. Neurosurgery. 2001;49:309-318. discussion 318–320
Sung V.C., Murray D.C., Price N.J. Subhyaloid or subinternal limiting membrane haemorrhage in meningococcal meningitis. Br J Ophthalmol. 2000;84:1206-1207.
Suskauer S.J., Huisman T.A. Neuroimaging in pediatric traumatic brain injury: current and future predictors of functional outcome. Dev Disabil Res Rev. 2009;15:117-123.
Suzuki Y., Awaya S. Long-term observation of infants with macular hemorrhage in the neonatal period. Jpn J Ophthalmol. 1998;42:124-128.
Swischuk L. Spine and spinal cord trauma in the battered child syndrome. Radiology. 1969;92:733.
Taff M.L., Boglioli L.R., DeFelice J.F. Controversies in shaken baby syndrome. J Forensic Sci. 1996;41:729-730.
Talbert D. The nature of shaken baby syndrome injuries and the significance of a “Lucid Interval”. Med Hypotheses. 2008;71:117-121.
Talvik I., Mannamaa M., Juri P., et al. Outcome of infants with inflicted traumatic brain injury (shaken baby syndrome) in Estonia. Acta Paediatr. 2007;96:1164-1168.
Tao H., Ma Z., Dai P., et al. Computer-aided 3-D reconstruction and measurement of the optic canal and intracanalicular structures. Chin Med J (Engl). 2000;113:140-143.
Tardieu A. Etude medico-legale sur les services et mauvais traitments exerceses sur des enfants. Hygiene Publication Medical Legale. 1860;361:13.
Tawil I., Stein D.M., Mirvis S.E., et al. Posttraumatic cerebral infarction: incidence, outcome, and risk factors. J Trauma. 2008;64:849-853.
Teasdale G.M., Graham D.I. Craniocerebral trauma: protection and retrieval of the neuronal population after injury. Neurosurgery. 1998;43:723-737. discussion 737–738
Tian H.L., Geng Z., Cui Y.H., et al. Risk factors for posttraumatic cerebral infarction in patients with moderate or severe head trauma. Neurosurg Rev. 2008;31:431-436. discussion 436–437
Togioka B.M., Arnold M.A., Bathurst M.A., et al. Retinal hemorrhages and shaken baby syndrome: an evidence-based review. J Emerg Med. 2009;37:98-106.
Tokmak M., Iplikcioglu A.C., Bek S., et al. The role of exudation in chronic subdural hematomas. J Neurosurg. 2007;107:290-295.
Tokutomi T., Hirohata M., Miyagi T., et al. Posttraumatic edema in the corpus callosum shown by MRI. Acta Neurochir Suppl. 1997;70:80-83.
Tomita H., Tone O., Ito U. Hemispheric cerebral atrophy after traumatic extra-axial hematoma in adults. Neurol Med Chir (Tokyo). 1997;37:819-824.
Tong K.A., Ashwal S., Holshouser B.A., et al. Hemorrhagic shearing lesions in children and adolescents with posttraumatic diffuse axonal injury: improved detection and initial results. Radiology. 2003;227:332-339.
Tong K.A., Ashwal S., Holshouser B.A., et al. Diffuse axonal injury in children: clinical correlation with hemorrhagic lesions. Ann Neurol. 2004;56:36-50.
Tung G.A., Kumar M., Richardson R.C., et al. Comparison of accidental and nonaccidental traumatic head injury in children on noncontrast computed tomography. Pediatrics. 2006;118:626-633.
Tuor U.I., Grewal D. Autoregulation of cerebral blood flow: influence of local brain development and postnatal age. Am J Physiol. 1994;267:H2220-H2228.
Tureen L. Lesions of the fundus associated with brain hemorrhage. Arch Neurol Psych. 1939;42:664.
Tzioumi D., Oates R.K. Subdural hematomas in children under 2 years. Accidental or inflicted? A 10-year experience. Child Abuse Negl. 1998;22:1105-1112.
Valadka A.B., Gopinath S.P., Robertson C.S. Midline shift after severe head injury: pathophysiologic implications. J Trauma. 2000;49:1-8. discussion 10
Vavilala M.S., Lee L.A., Boddu K., et al. Cerebral autoregulation in pediatric traumatic brain injury. Pediatr Crit Care Med. 2004;5:257-275.
Vavilala M.S., Muangman S., Waitayawinyu P., et al. Neurointensive care; impaired cerebral autoregulation in infants and young children early after inflicted traumatic brain injury: a preliminary report. J Neurotrauma. 2007;24:87-96.
Vexler Z.S., Sharp F.R., Feuerstein G.Z., et al. Translational stroke research in the developing brain. Pediatr Neurol. 2006;34:459-475.
Vexler Z.S., Yenari M.A. Does inflammation after stroke affect the developing brain differently than adult brain? Dev Neurosci. 2009;31:378-393.
Vinchon M., Defoort-Dhellemmes S., Desurmont M., et al. Accidental and nonaccidental head injuries in infants: a prospective study. J Neurosurg. 2005;102:380-384.
Vinchon M., de Foort-Dhellemmes S., Desurmont M., et al. Confessed abuse versus witnessed accidents in infants: comparison of clinical, radiological, and ophthalmological data in corroborated cases. Childs Nerv Syst. 2009.
Vinchon M., Noizet O., Defoort-Dhellemmes S., et al. Infantile subdural hematomas due to traffic accidents. Pediatr Neurosurg. 2002;37:245-253.
Vinchon M., Noule N., Tchofo P.J., et al. Imaging of head injuries in infants: temporal correlates and forensic implications for the diagnosis of child abuse. J Neurosurg. 2004;101:44-52.
Vowles G.H., Scholtz C.L., Cameron J.M. Diffuse axonal injury in early infancy. J Clin Pathol. 1987;40:185-189.
Waga S., Tochio H., Sakakura M. Traumatic cerebral swelling developing within 30 minutes after injury. Surg Neurol. 1979;11:191-193.
Walter T., Meissner C., Oehmichen M. Pathomorphological staging of subdural hemorrhages: statistical analysis of posttraumatic histomorphological alterations. Leg Med (Tokyo). 2009;11(Suppl 1):S56-S62.
Wasterlain C.G., Niquet J., Thompson K.W., et al. Seizure-induced neuronal death in the immature brain. Prog Brain Res. 2002;135:335-353.
Weissgold D.J., Budenz D.L., Hood I., et al. Ruptured vascular malformation masquerading as battered/shaken baby syndrome: a nearly tragic mistake. Surv Ophthalmol. 1995;39:509-512.
Weston J. Guidelines for the application of pathology to crime investigation. In: Spitz W., Fisher R., editors. Medicolegal Investigation of Death. Springfield, Ill: Charles C. Thomas, 1973.
Wetterling T., Demierre B., Rama B., et al. Protein analysis of subdural hygroma fluid. Acta Neurochirurgica (Wien). 1988;91:79.
Wilberger J.E.Jr, Rothfus W.E., Tabas J., et al. Acute tissue tear hemorrhages of the brain: computed tomography and clinicopathological correlations. Neurosurgery. 1990;27:208-213.
Williams R. Injuries in infants and small children resulting from witnessed and corroborated free falls. J Trauma. 1991;31:1350.
Willman K.Y., Bank D.E., Senac M., et al. Restricting the time of injury in fatal inflicted head injuries. Child Abuse Negl. 1997;21:929-940.
Wilms G., Vanderschueren G., Demaerel P.H., et al. CT and MR in infants with pericerebral collections and macrocephaly: benign enlargement of the subarachnoid spaces versus subdural collections. Am J Neuroradiol. 1993;14:855-860.
Woertgen C., Rothoerl R., Holzschuh M., et al. Comparison of serial S-100 or NSE serum measurements after severe head injury. Acta Neurochirurgica (Wien). 1997;139:1161-1165.
Wong F.Y., Leung T.S., Austin T., et al. Impaired autoregulation in preterm infants identified by using spatially resolved spectroscopy. Pediatrics. 2008;121:e604-e611.
Woolley P., Evans W. Significance of skeletal lesions in infancy resembling those of traumatic origin. JAMA. 1955;158:539.
Wygnanski-Jaffe T., Levin A.V., Shafiq A., et al. Postmortem orbital findings in shaken baby syndrome. Am J Ophthalmol. 2006;142:233-240.
Wygnanski-Jaffe T., Morad Y., Levin A.V. Pathology of retinal hemorrhage in abusive head trauma. Forensic Sci Med Pathol. 2009;5:291-297.
Xiao-Sheng H., Sheng-Yu Y., Xiang Z., et al. Diffuse axonal injury due to lateral head rotation in a rat model. J Neurosurg. 2000;93:626-633.
Young K.D., Okada P.J., Sokolove P.E., et al. A randomized, double-blinded, placebo-controlled trial of phenytoin for the prevention of early posttraumatic seizures in children with moderate to severe blunt head injury. Ann Emerg Med. 2004;43:435-446.
Young T.L., Quinn G.E., Baumgart S., et al. Extracorporeal membrane oxygenation causing asymmetric vasculopathy in neonatal infants. J AAPOS. 1997;1:235-240.
Zernikow B., Michel E., Kohlmann G., et al. Cerebral autoregulation of preterm neonates – a non-linear control system? Arch Dis Child Fetal Neonatal Ed. 1994;70:F166-F173.
Zhang J., Yoganandan N., Pintar F.A. Dynamic biomechanics of the human head in lateral impacts. Annu Proc Assoc Adv Automot Med. 2009;53:249-256.
Zimmerman R.A., Bilaniuk L.T., Bruce D., et al. Computed tomography of craniocerebral injury in the abused child. Radiology. 1979;130:687-690.
Zouros A., Bhargava R., Hoskinson M., et al. Further characterization of traumatic subdural collections of infancy. Report of five cases. J Neurosurg. 2004;100:512-518.
Zuccarello M., Facco E., Zampieri P., et al. Severe head injury in children: early prognosis and outcome. Childs Nerv Syst. 1985;1:158-162.
Zupanc M.L. Clinical evaluation and diagnosis of severe epilepsy syndromes of early childhood. J Child Neurol. 2009;24:6S-14S.
Zwaan J., Cardenas R., O’Connor P.S. Long-term outcome of neonatal macular hemorrhage. J Pediatr Ophthalmol Strabismus. 1997;34:286-288.