Neural Crest
The neural crest, whose existence has been recognized for more than a century, forms an exceptionally wide range of cell types and structures, including several types of nerves and glia, connective tissue, bones, and pigment cells. Its importance and prominence are such that the neural crest has often been called the fourth germ layer of the body. Not until adequate methods of marking neural crest cells became available—first with isotopic labels and subsequently with stable biological markers, monoclonal antibodies, intracellular dyes, and genetic markers—did the neural crest become one of the most widely studied components of the vertebrate embryo. Most studies on the neural crest have been conducted on the avian embryo because of its accessibility and the availability of specific markers (see Fig. 9.31). More recently, emphasis has shifted to studies on the mouse, especially for dissecting molecular controls, but it appears that most of the information on the biology of the neural crest derived from birds can be applied to mammalian embryos. Some important syndromes and malformations are based on abnormalities of the neural crest. Some of these syndromes are presented in Clinical Correlation 12.1, at the end of the chapter.
Developmental History of the Neural Crest
Origin, Induction, and Specification
According to the most recent data, the earliest stages of neural crest induction may occur as early as gastrulation, but according to the classical model, the neural crest arises as the result of inductive actions by the adjacent non-neural ectoderm and possibly nearby mesoderm on the neural plate (Fig. 12.1). The ectodermal inductive signals are bone morphogenetic proteins (BMPs) and Wnts. Fibroblast growth factor-8 (FGF-8) from mesoderm plays a role in neural crest induction in amphibians, and it seems to be involved in mammals as well. The role of BMPs is complex and relates to a concentration gradient along the ectodermal layer as neurulation proceeds. The highest concentrations of BMP are seen in the lateral ectoderm, and cells exposed to these concentrations remain ectodermal. Cells within the neural plate are exposed to the lowest concentrations of BMP because of the local inhibitory actions of noggin and chordin (see Fig. 5.8C), and, by default, they remain neural. Cells at the border of the neural plate are exposed to intermediate levels of BMP, and, in this environment, they are induced to form neural crest precursor cells.
Epitheliomesenchymal Transformation and Emigration from the Neural Tube
Within the neural tube, neural crest precursor cells are epithelial and are tightly adherent to other neuroepithelial cells through a variety of intercellular connections. Prominent among them are the cadherins. Among the new transcription factors upregulated in induced neural crest precursor cells are snail-1 and snail-2 (formerly called slug) and Foxd-3, which are instrumental in allowing the neural crest cells to break free from the neural epithelium and then migrate away as mesenchymal cells.* Under the influence of snail-1 and snail-2, the profile of cadherins produced by the neural crest precursors changes from type I cadherins (e.g., N-cadherin and E-cadherin), which are strongly adhesive, to type II cadherins, which are less adhesive.
Neural Crest Cell Migration
After leaving the neuroepithelium, the neural crest cells first encounter a relatively cell-free environment rich in extracellular matrix molecules (Fig. 12.2). In this environment, the cells undergo extensive migrations along several well-defined pathways. These migrations are determined by intrinsic properties of the neural crest cells and features of the external environment encountered by the migrating cells.
Neural crest migration is influenced by a variety of molecules residing in the extracellular matrix. Although the presence of a basal lamina can inhibit their emigration from the neural tube, neural crest cells often prefer to migrate along basal laminae, such as those of the surface ectoderm or neural tube, after they have left the neural tube. Components of the extracellular matrix permissive for migration include molecules found in basal laminae, such as fibronectin, laminin, and type IV collagen (Fig. 12.3). Attachment to and migration over these substrate molecules are mediated by the family of attachment proteins called integrins. Other molecules, such as chondroitin sulfate proteoglycans, are not good substrates for neural crest cells and inhibit their migration.
Neural crest cells emigrate from the neural tube or neural folds in streams, with each cell in contact with neighbors through filopodial contacts. During their migratory phase, neural crest cells are exquisitely sensitive to guidance molecules, most of which are inhibitory. Among the most important of these guidance molecules are the ligand/receptor pairs Robo/Slit, Neuropilin/Semaphorin and Ephrin/Eph (see Table 11.1). Much less is known about attractive influences on neural crest cell migration. During migration, neural crest cells extend protrusions that both test the environment and are part of the propulsive mechanism. If an inhibitory influence is encountered, the protrusions collapse through signals derived from a planar cell polarity pathway (see p. 87). This mechanism acts as a brake when the cells encounter an inhibitory environment, but it is also involved in their forward propulsion. In a migrating stream of neural crest cells, contact with the cells behind also results in the pulling of protrusions at the trailing edge of the cells, thus resulting in a net forward motion of the leading cells. Specific examples of the environmental control of neural crest cell migrations are given later in this chapter. Much remains to be learned about what causes neural crest cells to stop migrating, but often they stop migrating in areas where repulsive signals are low.
Differentiation of Neural Crest Cells
Neural crest cells ultimately differentiate into an astonishing array of adult structures (Table 12.1). What controls their differentiation is one of the principal questions of neural crest biology. Two opposing hypotheses have been proposed. According to one, all neural crest cells are equal in developmental potential, and their ultimate differentiation is entirely determined by the environment through which they migrate and into which they finally settle. The other hypothesis suggests that premigratory crest cells are already programmed for different developmental fates, and that certain stem cells are favored, whereas others are inhibited from further development during migration. More recent research indicates that the real answer can be found somewhere between these two positions. Increasing evidence suggests that among migrating neural crest cells is a mix of cells whose fate has been predetermined within the neural tube and cells whose ultimate phenotype depends on environmental influences.
Table 12.1
Major Derivatives of the Neural Crest
Trunk Crest | Cranial and Circumpharyngeal Crests | |
NERVOUS SYSTEM | ||
Sensory nervous system | Spinal ganglia | Ganglia of trigeminal nerve (V), facial nerve (VII), glossopharyngeal nerve (superior ganglion) (IX), vagus nerve (jugular ganglion) (X) |
Satellite cells of sensory ganglia | Satellite cells of sensory ganglia | |
Schwann cells of all peripheral nerves, enteric glial cells | Schwann cells of peripheral nerves | |
Merkel cells | ||
Autonomic nervous system | Sympathetic chain ganglia, collateral ganglia: celiac and mesenteric | Parasympathetic ganglia: ciliary, ethmoidal, sphenopalatine, submandibular, visceral |
Parasympathetic ganglia: pelvic and visceral plexuses | ||
Meninges | None | Leptomeninges of prosencephalon and part of mesencephalon |
Pigment cells | Melanocytes | Melanocytes |
Endocrine and paraendocrine cells | Adrenal medulla, neurosecretory cells of heart and lungs | Carotid body (type I cells), parafollicular cells (thyroid) |
MESECTODERMAL CELLS | ||
Skeleton | None | Cranial vault (squamosal and part of frontal), nasal and orbital, otic capsule (part), palate and maxillary, mandible, sphenoid (small contribution), trabeculae (part), visceral cartilages, external ear cartilage (part) |
Connective tissue | None | Dermis and fat of skin; cornea of eye (fibroblasts of stroma and corneal endothelium); dental papilla (odontoblasts); connective tissue stroma of glands: thyroid, parathyroid, thymus, salivary, lacrimal; outflow tract (truncoconal region) of heart; cardiac semilunar valves; walls of aorta and aortic arch–derived arteries; adipocytes |
Muscle | None | Ciliary muscles, dermal smooth muscles, vascular smooth muscle, minor skeletal muscle elements (?) |
A more striking example is the conversion of cells of the periocular neural crest mesenchyme, which in birds would normally form cartilage, into neurons if they are associated with embryonic hindgut tissue in vitro. Many of the regional influences on the differentiation of local populations of neural crest cells are now recognized to be interactions between the migrating neural crest cells and specific tissues that they encounter during migration. Examples of tissue interactions that promote the differentiation of specific neural crest derivatives are given in Table 12.2.
Table 12.2
Environmental Factors Promoting Differentiation of Neural Crest Cells
Neural Crest Derivative | Interacting Structure |
Bones of cranial vault | Brain |
Bones of base of skull | Notochord, brain |
Pharyngeal arch cartilages | Pharyngeal endoderm |
Meckel’s cartilage | Cranial ectoderm |
Maxillary bone | Maxillary ectoderm |
Mandible | Mandibular ectoderm |
Palate | Palatal ectoderm |
Otic capsule | Otic vesicle |
Dentin of teeth | Oral ectoderm |
Glandular stroma: thyroid, parathyroid, thymus, salivary | Local epithelium |
Adrenal medullary chromaffin cells | Glucocorticoids secreted by adrenal cortex |
Enteric neurons | Gut wall |
Sympathetic neurons | Spinal cord, notochord, somites |
Sensory neurons | Peripheral target tissue |
Pigment cells | Extracellular matrix along pathway of migration |
Many neural crest cells are bipotential, depending on signals from their local environment for cues to their final differentiation. Cultured heart cells secrete a protein that converts postmitotic sympathetic neurons from an adrenergic (norepinephrine transmitter) phenotype to a cholinergic (acetylcholine-secreting) phenotype (see Fig. 11.22). During normal development, the sympathetic neurons that innervate sweat glands are catecholaminergic until their axons actually contact the sweat glands. At that point, they become cholinergic.
Major Divisions of the Neural Crest
Trunk Neural Crest
The neural crest of the trunk extends from the level of the sixth somite to the most caudal somites. Three pathways of migration are commonly described (Fig. 12.4). These pathways occur in different sequences and are subject to different controls. The first neural crest cells to leave the neural tube migrate around and between the somites, which are still in an epithelial configuration. Their migratory path follows the intersomitic blood vessels, and the cells rapidly reach the region of the dorsal aorta (see Fig. 12.4, pathway 1). It may be that at this early stage no other pathway is available to these migrating cells. These cells constitute the sympathoadrenal lineage.
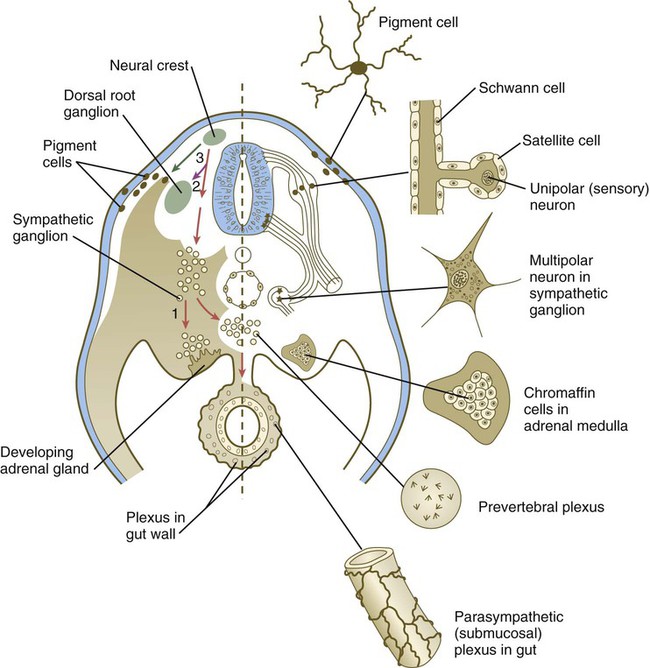
Left, Pathways in the early embryo. The first emigrating cells (pathway 1) follow the ventral (sympathoadrenal) pathway (red arrows). The second wave of emigrating cells (pathway 2) follows the ventrolateral pathway indicated by the purple arrow. The last cells to leave the neural tube (pathway 3) follow the dorsolateral pathway (green arrow) as they go on to differentiate into pigment cells.
Slightly later in development, the somites have become dissociated into sclerotomal and dermomyotomal compartments. At this stage, the neural crest cells preferentially enter the anterior compartment of the sclerotome. They are kept from entering the posterior compartment mainly through the repulsive action of semaphorinA3F (SEMA3F) in the posterior sclerotome, by acting through its receptor Neuropilin-2 (Nrp-2) on the neural crest cells. Other molecular repulsion mechanisms are also involved, but in mammals, this mechanism is the most influential. Passage through the anterior sclerotome is facilitated by extracellular matrix molecules, in particular thrombospondin. These cells constitute the ventrolateral pathway, and they ultimately form the dorsal root ganglia (see Fig. 12.4, pathway 2). These cells form the ganglia in concert with the outgrowth of the motor axons from the spinal cord, which follow similar environmental cues.
The last pathway (see Fig. 12.4, pathway 3) is the dorsolateral pathway, and the cells that follow it appear to be determined even before emigration from the neural tube to become pigment cells. Other neural crest cells are not able to use this pathway. In mammals, cells that follow this pathway depend on the Steel factor, produced by the dermomyotome, to be able to use this pathway. The cells that take this pathway migrate just beneath the ectoderm and ultimately enter the ectoderm as pigment cells (melanocytes).
Sympathoadrenal Lineage
The entire length of the gut is populated by neural crest–derived parasympathetic neurons and associated cells, the enteric glia. These arise from neural crest cells in the cervical (vagal) and sacral levels and, under the influence of glial-derived neurotrophic factor, undertake extensive migrations along the developing gut. Sacral neural crest cells colonize the hindgut, but even there they form only a few enteric neurons. The rest are derived from the vagal crest. The autonomic innervation of the gut is covered in greater detail in the discussion of the vagal crest (see p. 264).
Cranial Neural Crest
In the mammalian head, neural crest cells leave the future brain well before closure of the neural folds (Fig. 12.5). In the area of the forebrain, no neural crest arises rostral to the anterior diencephalon (anterior neural ridge [see Fig. 6.4B]), but from the region marked by prosomeres 1 to 3, a continuous sheet of neural crest cells migrates over much of the head (Fig. 12.6). Neural crest is inhibited from forming in the anterior neural ridge by the signaling molecule Dickkopf 1, a Wnt inhibitor that is secreted by the nearby prechordal mesoderm. Specific streams of neural crest cells emanating from the hindbrain populate the first three pharyngeal arches. Although the streams of migrating cranial neural crest appear at first glance to be not very discrete, there is an overall very specific spatiotemporal order in their pathways to their final destinations in the head and neck.
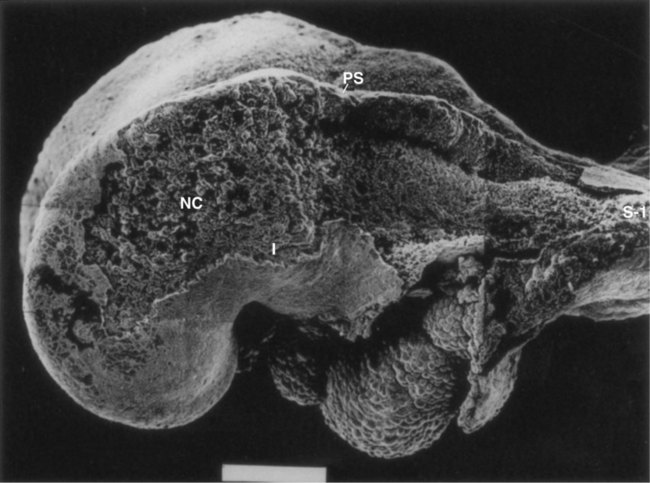
In this scanning electron micrograph, the ectoderm was removed from a large part of the side of the head, thus exposing migrating neural crest (NC) cells cranial (to the left) to the preotic sulcus (PS). Many of the cells are migrating toward the first pharyngeal arch (I). The area between the preotic sulcus and the first somite (S-1) is devoid of neural crest cells because in this region they have not begun to migrate from the closing neural folds. The white bar at the bottom represents 100 µm. (From Tan SS, Morriss-Kay G: Cell Tissue Res 204:403-416, 1985.)
A major functional subdivision of cranial neural crest occurs at the boundary between rhombomeres 2 (r2) and 3 (r3). Neural crest cells emerging from the diencephalon posteriorly through r3 do not express any Hox genes, whereas the cells emerging from the hindbrain region from r4 and posteriorly express a well-ordered sequence of Hox genes (see Fig. 12.8).
There is remarkable specificity in the relationship among the origins of the neural crest in the hindbrain, its ultimate destination within the pharyngeal arches, and the expression of certain gene products (Figs. 12.7 and 12.8). Neural crest cells associated with r1 and r2 migrate into and form the bulk of the first pharyngeal arch; those of r4, into the second arch; and those of r6 and r7, into the third arch, as three separate streams of cells.
A close correlation exists between the pattern of migration of the rhombomeric neural crest cells and the expression of products of the Hoxb gene complex. Hoxb-2, Hoxb-3, and Hoxb-4 products are expressed in a regular sequence in the neural tube and the neural crest–derived mesenchyme of the second, third, and fourth pharyngeal arches. Hoxb is not expressed in r1 and r2 or in the first pharyngeal arch mesenchyme. Only after the pharyngeal arches become populated with neural crest cells does the ectoderm overlying the arches express a similar pattern of Hoxb gene products (see Fig. 12.8). These Hoxb genes may play a role in positionally specifying the neural crest cells with which they are associated. Interactions between the neural crest cells and the surface ectoderm of the pharyngeal arches may specify the ectoderm of the arches.
Emigrating cranial neural crest cells consist of a mix of cells whose fate has already been fixed and those whose fate is largely determined by their environment. As they move away from the brain, cranial crest cells migrate as sheets rostrally or streams (in the pharyngeal area) in the dorsolateral pathway directly beneath the ectoderm. This is in strong contrast to migratory patterns in trunk neural crest, where the first two waves of migration head directly ventrally or ventrolaterally (see Fig. 12.4, pathways 1 and 2). As they approach the pharyngeal arches, especially the second arch, the lead cells in the streams of neural crest are attracted by vascular endothelial growth factor (VEGF), a chemoattractant produced by the distal ectoderm. The trailing cells in the stream are interconnected by long filopodia and follow the lead cells as they disperse into the pharyngeal arches themselves.
Cranial neural crest cells differentiate into a wide variety of cell and tissue types (see Table 12.1), including connective tissue and skeletal tissues. These tissues constitute much of the soft and hard tissues of the face (Fig. 12.9). (Specific details of morphogenesis of the head are presented in Chapter 14.)
Circumpharyngeal Neural Crest
The circumpharyngeal neural crest arises in the posterior rhombencephalic region at the levels of somites 1 to 7 (Fig. 12.10). This region of neural crest represents a transition between cranial and trunk neural crest. Cells arising at the levels of the first four somites behave more like cranial crest, whereas those emigrating at the levels of somites 5 to 7 follow pathways more characteristic of trunk crest. A prominent landmark in this area is the circumpharyngeal ridge, an arc-shaped aggregation of cells that passes behind the sixth pharyngeal arch (Fig. 12.11). Ventral to the pharynx, this ridge sweeps cranially and provides the pathway through which the hypoglossal nerve (XII) and its associated skeletal muscle precursors pass. Most neural crest cells from the somite 1 to 3 level pass into either the outflow tract of the heart or into the fourth and sixth pharyngeal arches (see Fig. 12.10). These cells are considered to constitute the cardiac crest. Other cells from this level, as well as those arising from the level of somites 4 to 7, are called the vagal crest. These cells migrate into the gut as precursors of the parasympathetic innervation of the digestive tract. They also form sensory neurons and glia, as well as making some contribution to sympathetic ganglia. Like the cranial crest cells, most cells of the cardiac crest migrate along the dorsolateral pathway between the somites and the ectoderm (see Fig. 12.10), whereas those of the vagal crest, like those of the trunk, initially migrate along the ventral pathways between the neural tube and the dermomyotome.
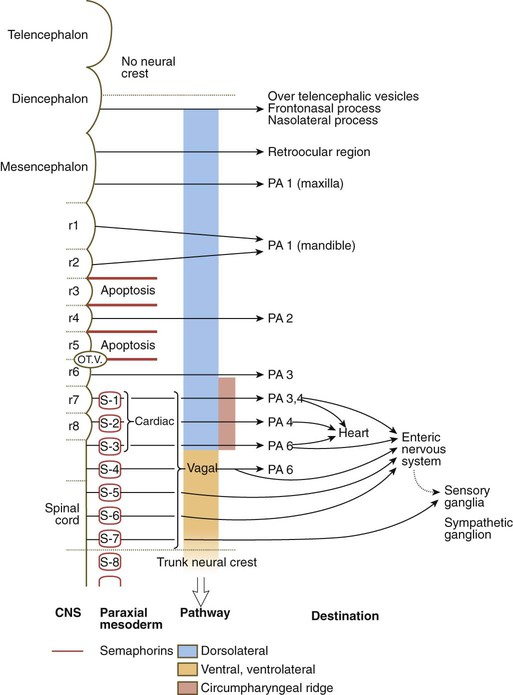
The arrows indicate migratory pathways, starting with their origin in the central nervous system. The circumpharyngeal crest arises from the level of somites 1 to 7. Note the shift in migratory pathway from one characteristic of cranial crest (blue) to one like that of trunk crest (pale orange). OT.V., otic vesicle; PA, pharyngeal arch; r, rhombomere; S, somite.
Cardiac Crest
The cardiac crest, arising at the level of somites 1 to 3, surrounds the endothelial precursors of the third, fourth, and sixth aortic arches, and it contributes massively to the truncoconal ridges that separate the outflow tract of the heart into aortic and pulmonary segments (see Chapter 17). Under the strong influence of semaphorins, cardiac crest cells migrate toward the heart and contribute to the leaflets of the semilunar valves at the base of the outflow tract, and in birds, at least, they may penetrate the interventricular septum. The cardiac neural crest may interact with pharyngeal endoderm to modify the signals leading to the normal differentiation of myocardial cells.
Summary
The neural crest arises from neuroepithelial cells along the lateral border of the neural plate. Having left the neural tube, neural crest cells migrate to peripheral locations throughout the body. Some substrates, such as those containing chondroitin sulfate molecules, are not favorable for neural crest cell migration.
Neural crest cells differentiate into many types of adult cells, such as sensory and autonomic neurons, Schwann cells, pigment cells, and adrenal medullary cells. Cells from the cranial and circumpharyngeal neural crest also differentiate into bone, cartilage, dentin, dermal fibroblasts, selected smooth muscle, the connective tissue stroma of pharyngeal glands, and several regions of the heart and great vessels.
The control of differentiation of neural crest cells is diverse; some cells are determined before they begin to migrate, and others respond to environmental cues along their paths of migration. Trunk neural crest cells cannot differentiate into skeletal elements.
Neural crest cells in the trunk follow three main paths of migration: (1) a ventral path for cells of the sympathoadrenal lineage, (2) a ventrolateral pathway leading through the anterior halves of the somites for sensory ganglion-forming cells, and (3) a dorsolateral pathway for pigment cells.
Cells of the cranial neural crest form many tissues of the facial region. In the pharyngeal region, the pathways of crest cell migration are closely correlated with regions of expression of products of the Hoxb gene complex. Cells of the cranial crest may be patterned with level-specific instructions, whereas cells of the trunk crest are not.
Several genetic diseases and syndromes are associated with disturbances of the neural crest. Neurofibromatosis is often characterized by multiple tumors and pigment disturbances. Disturbances of the cardiac neural crest can result in septation defects in the heart and outflow tract.