Medical Gas Therapy
After reading this chapter you will be able to:
Describe when oxygen (O2) therapy is needed.
Assess the need for O2 therapy.
Describe what precautions and complications are associated with O2 therapy.
Select an O2 delivery system appropriate for the respiratory care plan.
Describe how to administer O2 to adults, children, and infants.
Describe how to check for proper function and to identify and correct malfunctions of O2 delivery systems.
Describe how to evaluate and monitor a patient’s response to O2 therapy.
Describe how to modify or recommend modification of O2 therapy on the basis of patient response.
Describe how to implement protocol-based O2 therapy.
Identify what indications, complications, and hazards apply to hyperbaric O2 therapy.
Oxygen Therapy
Consensus exists among clinicians about the proper use of O2 therapy.1–4 As the primary member of the health care team responsible for O2 administration, the RT must be well versed in the goals and objectives of this therapy and its use in clinical practice.
General Goals and Clinical Objectives
• Correct documented or suspected acute hypoxemia
• Decrease symptoms associated with chronic hypoxemia
• Decrease the workload hypoxemia imposes on the cardiopulmonary system
Decreasing Symptoms of Hypoxemia
In addition to relieving hypoxemia, O2 therapy can help relieve the symptoms associated with certain lung disorders. Specifically, patients with chronic obstructive pulmonary disease (COPD) and some forms of interstitial lung disease report less dyspnea when receiving supplemental O2.5 O2 therapy also may improve mental function among patients with chronic hypoxemia.6
Minimizing Cardiopulmonary Workload
Hypoxemia causes pulmonary vasoconstriction and pulmonary hypertension. Pulmonary vasoconstriction and hypertension increase workload on the right side of the heart. For patients with chronic hypoxemia, this increased workload over the long-term can lead to right ventricular failure (cor pulmonale). O2 therapy can reverse pulmonary vasoconstriction and decrease right ventricular workload.7
Clinical Practice Guideline
To guide practitioners in safe and effective patient care, the American Association for Respiratory Care (AARC) has developed and published clinical practice guidelines for O2 therapy. Excerpts from the AARC guideline on O2 therapy in acute care hospitals appear in Clinical Practice Guideline 38-1.2 Additional AARC guidelines for O2 therapy in the home or an extended care facility3 and for selection of O2 delivery devices for neonatal and pediatric patients4 are provided in Chapters 48 and 51.
Assessing the Need for Oxygen Therapy
Laboratory measures for documenting hypoxemia include hemoglobin saturation and partial pressure of oxygen (PO2), as determined by either invasive or noninvasive means (see Chapter 18). Threshold criteria defining hypoxemia with these measures are described in the AARC clinical practice guideline (see Clinical Practice Guideline 38-1).2
O2 therapy is needed for patients with disorders associated with hypoxemia. Examples are postoperative patients; patients with carbon monoxide or cyanide poisoning, shock, trauma, or acute myocardial infarction, and some premature infants.1,2,8
Careful bedside physical assessment can disclose a patient’s need for O2 therapy. Table 38-1 summarizes the common respiratory, cardiovascular, and neurologic signs used in the detection of hypoxia. The RT combines this information with more quantitative measures such as arterial blood gas results to confirm inadequate oxygenation. The patient care team often relies on the RT to recommend administration of supplemental O2 based on quantitative and qualitative information.
TABLE 38-1
Finding | Mild to Moderate | Severe |
Respiratory | Tachypnea | Tachypnea |
Dyspnea | Dyspnea | |
Paleness | Cyanosis | |
Cardiovascular | Tachycardia | Tachycardia, eventual bradycardia, arrhythmia |
Mild hypertension, peripheral vasoconstriction | Hypertension and eventual hypotension | |
Neurologic | Restlessness | Somnolence |
Disorientation | Confusion | |
Headaches | Distressed appearance | |
Lassitude | Blurred vision | |
Tunnel vision | ||
Loss of coordination | ||
Impaired judgment | ||
Slow reaction time | ||
Manic-depressive activity | ||
Coma |
Precautions and Hazards of Supplemental Oxygen
Excerpts from the relevant AARC clinical practice guidelines (see Clinical Practice Guideline 38-1) outline the major precautions and hazards associated with administration of supplemental O2.2 Five of these hazards are common enough to warrant additional discussion.
Oxygen Toxicity
O2 toxicity primarily affects the lungs and the central nervous system (CNS).9–11 Two primary factors determine the harmful effects of O2: PO2 and exposure time (Figure 38-1). The higher the PO2 and the longer the exposure, the greater the likelihood of damage. Effects on the CNS, including tremors, twitching, and convulsions, tend to occur only when a patient is breathing O2 at pressures greater than 1 atm (hyperbaric pressure). Pulmonary effects can also occur with enriched O2 environments at normal atmospheric pressures.
Table 38-2 summarizes the physiologic response to breathing 100% O2 at sea level. A patient exposed to a high PO2 for a prolonged period has signs similar to bronchopneumonia. Patchy infiltrates appear on chest radiographs and usually are most prominent in the lower lung fields.
TABLE 38-2
Physiologic Responses of Healthy Individuals to Exposure to 100% Inspired Oxygen
Exposure Time (hr) | Physiologic Response |
0-12 | Normal pulmonary function |
Tracheobronchitis | |
Substernal chest pain | |
12-24 | Decreasing vital capacity |
25-30 | Decreasing lung compliance |
Increasing P(A-a)O2 | |
Decreasing exercise PO2 | |
30-72 | Decreasing diffusing capacity |
As the lung injury worsens, blood oxygenation deteriorates. If this progressive hypoxemia is managed with additional O2, the toxic effects worsen (Figure 38-2). However, if the patient can be kept alive while fractional inspired oxygen concentration (FiO2) is decreased, the pulmonary damage sometimes resolves.
The toxicity of O2 is caused by overproduction of O2 free radicals. O2 free radicals are by-products of cellular metabolism. If unchecked, these radicals can severely damage or kill cells.9 Normally, however, special enzymes such as superoxide dismutase inactivate the O2 free radicals before they can do serious damage. Antioxidants such as vitamin E, vitamin C, and beta-carotene also can defend against O2 free radicals.
Exactly how much O2 is safe is the subject of debate. Results of most studies indicate that adults can breathe up to 50% for extended periods without major lung damage.12 Rather than applying strict cutoffs, one can weigh both FiO2 and exposure time in assessing the risks of high PO2 (see the accompanying Rule of Thumb).13 The goal always should be to use the lowest possible FiO2 compatible with adequate tissue oxygenation.
Depression of Ventilation
When breathing moderate to high O2 concentrations, a very small percentage of patients with COPD and chronic hypercapnia tend to ventilate less.14 Decreases in ventilation of nearly 20% have been observed in these patients with accompanying elevations in arterial partial pressure of carbon dioxide (PaCO2) of 20 to 23 mm Hg.15 However, this hypoventilation is not typical of patients with COPD, and although these patients should always be carefully monitored, appropriate management of hypoxemia should never be avoided because of concern for O2-induced hypoventilation.
The primary reason some patients with COPD hypoventilate when given O2 is most likely suppression of the hypoxic drive. In these patients, the normal response to high partial pressure of carbon dioxide (PCO2) is blunted, the primary stimulus to breathe being lack of O2 as sensed by the peripheral chemoreceptors. The increase in the blood O2 level in these patients suppresses peripheral chemoreceptors, depresses ventilatory drive, and elevates the PCO2.16,17 High blood O2 levels may disrupt the normal ventilation/perfusion balance and cause an increase in dead space-to-tidal volume ratio (VD/VT) and in PaCO2.18
Retinopathy of Prematurity
Retinopathy of prematurity (ROP), also called retrolental fibroplasia, is an abnormal eye condition that occurs in some premature or low-birth-weight infants who receive supplemental O2. An excessive blood O2 level causes retinal vasoconstriction, which leads to necrosis of the blood vessels. In response, new vessels form and increase in number. Hemorrhage of these delicate new vessels causes scarring behind the retina. Scarring often leads to retinal detachment and blindness.19 ROP most often affects neonates up to approximately 1 month of age, by which time the retinal arteries have sufficiently matured. Excessive O2 is not the only factor associated with ROP; other factors associated with ROP include hypercapnia, hypocapnia, intraventricular hemorrhage, infection, lactic acidosis, anemia, hypocalcemia, and hypothermia.
Because premature infants often need supplemental O2, the risk of ROP poses a serious management problem. The American Academy of Pediatrics recommends keeping arterial PO2 in an infant less than 80 mm Hg as the best way to minimize the risk of ROP.8
Absorption Atelectasis
FiO2 greater than 0.50 presents a significant risk of absorption atelectasis.20 Nitrogen normally is the most plentiful gas in both the alveoli and the blood. Breathing high levels of O2 quickly depletes body nitrogen levels. As blood nitrogen levels decrease, the total pressure of venous gases rapidly decreases. Under these conditions, gases that exist at atmospheric pressure within any body cavity rapidly diffuse into the venous blood. This principle is used for removing trapped air from body cavities. Giving patients high levels of O2 can help clear trapped air from the abdomen or thorax.
This same phenomenon can cause lung collapse, especially if the alveolar region becomes obstructed (Figure 38-3). Under these conditions, O2 rapidly diffuses into the blood (see Figure 38-3, A). With no source for repletion, the total gas pressure in the alveolus progressively decreases until the alveolus collapses. Because collapsed alveoli are perfused but not ventilated, absorption atelectasis increases the physiologic shunt and worsens blood oxygenation.20
The risk of absorption atelectasis is greatest in patients breathing at low tidal volumes as a result of sedation, surgical pain, or CNS dysfunction. In these cases, poorly ventilated alveoli may become unstable when they lose O2 faster than it can be replaced. The result is a more gradual shrinking of the alveoli that may lead to complete collapse, even when the patient is not breathing supplemental O2 (see Figure 38-3, B). For an alert patient, this is not a great risk because the natural sigh mechanism periodically hyperinflates the lung.
Fire Hazard
Despite numerous preventive measures, fires involving enriched O2 environments continue to occur in health care facilities. Fires seem to pose the greatest risk in operating rooms and in association with selected respiratory procedures. During surgery and procedures such as tracheotomies, electronic scalpels and similar devices are often used while the patient is receiving supplemental O2. To complicate matters, even higher O2 concentrations may exist under surgical drapes.21 Other situations associated with increased fire risk involve home care patients smoking while receiving low-flow O2 and the use of aluminum O2 regulators. Additionally, hyperbaric oxygen (HBO) therapy or therapy at increased atmospheric pressures (discussed later in this chapter) often involves the administration of supplemental O2 and greatly increases fire risk.
Some simple strategies can be used to reduce the fire risk in health care facilities. Effectively managing the fire triangle of O2, heat, and fuel is key. An essential component is always using the lowest effective FiO2 for a given clinical situation. In addition, using scavenging systems to minimize O2 buildup beneath sterile drapes during surgery or while performing tracheostomies can help reduce fire risk. Avoiding the use of inappropriate or outdated equipment such as aluminum gas regulators and educating clinicians, patients and caregivers on safe O2 use are also important measures. Additionally, fire prevention protocols for HBO therapy should be strictly followed.21
Oxygen Delivery Systems: Design and Performance
RTs can select from an array of systems for administering O2 and other therapeutic gases. Proper device selection requires in-depth knowledge of both the general performance characteristics of these systems and the individual capabilities.22 O2 delivery devices traditionally are categorized by design. Three basic designs exist: low-flow systems, reservoir systems, and high-flow systems. Enclosures, commonly identified as a fourth category, are reservoirs that surround the head or body. The design categories share functional characteristics, capabilities, and limitations.
Although design plays an important role in the selection of these devices, clinical performance ultimately determines how the device is used. The user judges the performance of an O2 delivery system by answering two key questions: (1) How much O2 can the system deliver (FiO2 or FiO2 range)? (2) Does the delivered FiO2 remain fixed or vary under changing patient demands?22
Whether a device delivers a fixed or variable FiO2 depends on how much of the patient’s inspired gas it supplies. If the system provides all of the patient’s inspired gas, FiO2 remains stable, even under changing demands. If the device provides only some of the inspired gas, the patient must draw the remainder from the surrounding air. In this case, the more the patient breathes, the more air dilutes the delivered O2, and FiO2 is lower. If the patient breathes less with this type of device, less air dilutes the O2, and FiO2 increases. A system that supplies only a portion of the inspired gas always provides a variable FiO2.23 FiO2 supplied with such systems can vary widely from minute to minute and even from breath to breath.
Figure 38-4 shows these concepts as applied to low-flow, reservoir, and high-flow systems. With the low-flow system (see Figure 38-4, A) the patient’s inspiratory flow often exceeds the flow delivered by the device; the result is air dilution (shaded areas). The greater the patient’s inspiratory flow, the more air is breathed, and FiO2 is lower. The high-flow system (see Figure 38-4, B) always exceeds the patient’s flow and provides a fixed FiO2. A fixed FiO2 can be achieved with a reservoir system (see Figure 38-4, C), which stores a reserve volume (flow × time) that equals or exceeds the patient’s tidal volume. For a reservoir system to provide a fixed FiO2, the reservoir volume must always exceed the patient’s tidal volume, and there cannot be any air leaks in the system. Table 38-3 outlines the general specifications for the common O2 therapy systems in current use.
TABLE 38-3
Overview of Oxygen Therapy Systems
Category | Device | Flow | FiO2 Range | FiO2 Stability | Advantages | Disadvantages | Best Use |
Low flow | Nasal cannula | ![]() |
22%-40% | Variable | Use on adults, children, infants; easy to use; disposable; low cost; well tolerated | Unstable, easily dislodged; high flow uncomfortable; can cause dryness, bleeding; polyps; deviated septum and mouth breathing may reduce FiO2 | Patient in stable condition who needs low FiO2; home care patient who needs long-term therapy, low to moderate FiO2 while eating |
Nasal catheter | ![]() |
22%-45% | Variable | Use on adults, children, infants; good stability; disposable; low cost | Difficult to insert; high flow increases back pressure; needs regular changing; polyps, deviated septum may block insertion; may provoke gagging, air swallowing, aspiration | Procedures in which cannula is difficult to use (bronchoscopy); long-term care of infants | |
Transtracheal catheter | ![]() |
22%-35% | Variable | Lower O2 use and cost; eliminates nasal and skin irritation; improved compliance; increased exercise tolerance; increased mobility; enhanced image | High cost; surgical complications; infection; mucous plugging; lost tract | Home care or ambulatory patients who need increased mobility or do not accept nasal O2 | |
Reservoir cannula | ![]() |
22%-35% | Variable | Lower O2 use and cost; increased mobility; less discomfort because of lower flow | Unattractive, cumbersome; poor compliance; must be regularly replaced; breathing pattern affects performance | Home care or ambulatory patients who need increased mobility | |
Simple mask | 5-10 L/min | 35%-50% | Variable | Use on adults, children, infants; quick, easy to apply; disposable; inexpensive | Uncomfortable; must be removed for eating; prevents radiant heat loss; blocks vomitus in unconscious patients | Emergencies; short-term therapy requiring moderate FiO2; mouth breathing patients requiring moderate FiO2 | |
Partial rebreathing mask | Minimum of 10 L/min (prevent bag collapse on inspiration) | 40%-70% | Variable | Same as simple mask; moderate to high FiO2 | Same as simple mask; potential suffocation hazard | Emergencies; short-term therapy requiring moderate to high FiO2 | |
Nonrebreathing mask | Minimum of 10 L/min (prevent bag collapse on inspiration) | 60%-80% | Variable | Same as simple mask; high FiO2 | Same as simple mask; potential suffocation hazard | Emergencies; short-term therapy requiring high FiO2 | |
Nonrebreathing circuit (closed) | 3 × VE (prevent bag collapse on inspiration) | 21%-100% | Fixed | Full range of FiO2 | Potential suffocation hazard; requires 50 psi air/O2; blender failure common | Patients who need precise FiO2 at any level (21%-100%) | |
High flow | AEM | Varies; should provide output flow >60 L/min | 24%-50% | Fixed | Easy to apply; disposable, inexpensive; stable, precise FiO2 | Limited to adult use; uncomfortable, noisy; must be removed for eating; FiO2 >0.40 not ensured; FiO2 varies with back pressure | Patients in unstable condition who need precise low FiO2 |
Air-entrainment nebulizer | 10-15 L/min input; should provide output flow of at least 60 L/min | 28%-100% | Fixed | Provides temperature control and extra humidification | FiO2 < 0.28 or >0.40 not ensured; FiO2 varies with back pressure; high infection risk | Patients with artificial airways who need low to moderate FiO2 | |
Blending system (open) | Should provide output flow of at least 60 L/min | 21%-100% | Fixed | Full range of FiO2 | Requires 50 psi air/O2; blender failure or inaccuracy common | Patients with high Ve who need high FiO2 | |
High-flow nasal cannula system | Up to 40 L/min (depending on system) | 35%-90% | Variable or fixed depending on system and input flow | Wide range of FiO2 and relative/absolute humidity; use on adults, children, infants | FiO2 not ensured depending on input flow and patient breathing pattern; infection risk | Patients of all ages with high or variable Ve who need supplemental O2, positive pressure, or humidity | |
Enclosure | Oxyhood | ≥7 L/min | 21%-100% | Fixed | Full range of FiO2 | Difficult to clean, disinfect | Infants who need supplemental O2 |
Isolette | 8-15 L/min | 40%-50% | Variable | Provides temperature control | Expensive, cumbersome, unstable FiO2 (leaks); difficult to clean, disinfect; limits patient mobility; fire hazard | Infants who need supplemental O2 and precise thermal regulation | |
Tent | 12-15 L/min | 40%-50% | Variable | Provides concurrent aerosol therapy | Expensive, cumbersome, unstable FiO2 (leaks); requires cooling; difficult to clean, disinfect; limits patient mobility; fire hazard | Toddlers or small children who need low to moderate FiO2 and aerosol |
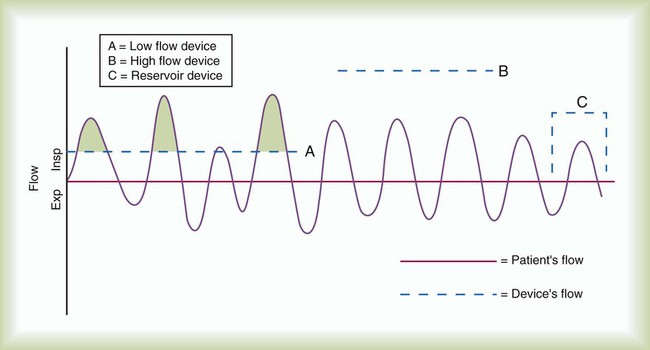
Low-Flow Systems
Nasal Cannula
A nasal cannula is a disposable plastic device consisting of two tips or prongs approximately 1 cm long that are connected to several feet of small-bore O2 supply tubing (Figure 38-5). The user inserts the prongs directly into the vestibule of the nose while attaching the supply tubing either directly to a flowmeter or to a bubble humidifier. In most cases, a humidifier is used only when the input flow is greater than 4 L/min.2 Even with extra humidity, flow greater than 6 to 8 L/min can cause patient discomfort, including nasal dryness and bleeding.22 Cannulas should not be used in newborns and infants if their nasal passages are obstructed, and flows generally should be limited to 2 L/min unless a specialized high-flow cannula system is being used.2 A high-flow nasal cannula, which is a variation of a standard nasal cannula, is discussed in more detail later in this chapter. Table 38-3 lists the FiO2 range, FiO2 stability, advantages, disadvantages, and best use of a nasal cannula.
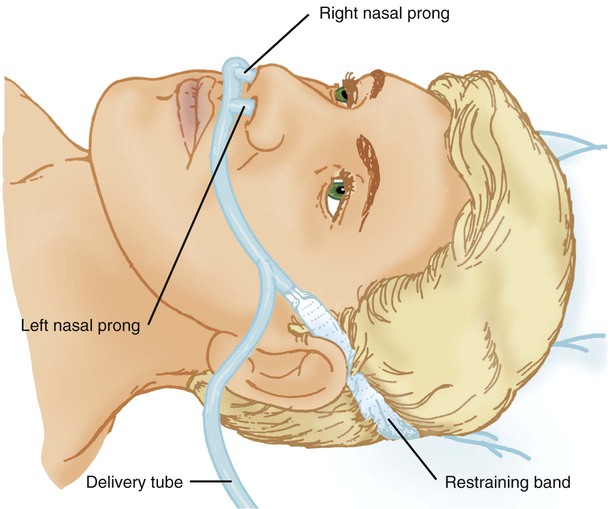
Nasal Catheter
Although use is generally limited to short-term O2 administration during specialized procedures such as a bronchoscopy, a nasal catheter is another low-flow O2 delivery device. A nasal catheter is a soft plastic tube with several small holes at the tip that is inserted by gently advancing it along the floor of either nasal passage and visualizing it just behind and above the uvula (Figure 38-6). Once in position, the catheter is taped to the bridge of the nose. If direct visualization is impossible, the catheter may be blindly inserted to a depth equal to the distance from the nose to the earlobe.
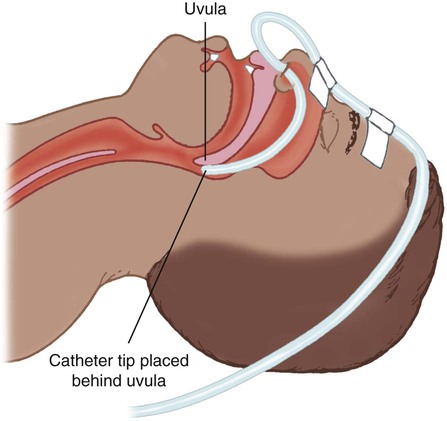
When placed too deep, the catheter can provoke gagging or swallowing of gas, which increases the likelihood of aspiration. Nasal catheters also affect the production of secretions; for this reason, a nasal catheter should be replaced with a new one (placed in the opposite naris) at least every 8 hours. Nasal catheters should be avoided in most patients with maxillofacial trauma, basal skull fracture, nasal obstruction, and coagulation problems. It has also been determined that nasal catheters are inappropriate for neonatal patients. As a result of these notable limitations, nasal catheters are rarely used today.4
Transtracheal Catheter
A transtracheal O2 catheter was first described by Heimlich in 1982.24 A physician surgically inserts this thin polytetrafluoroethylene (Teflon) catheter with a guidewire directly into the trachea between the second and third tracheal rings (Figure 38-7). A custom-sized chain necklace secures the catheter in position. Standard tubing connected directly to a flowmeter provides the O2 source flow.25 Because flow is so low, no humidifier is needed.
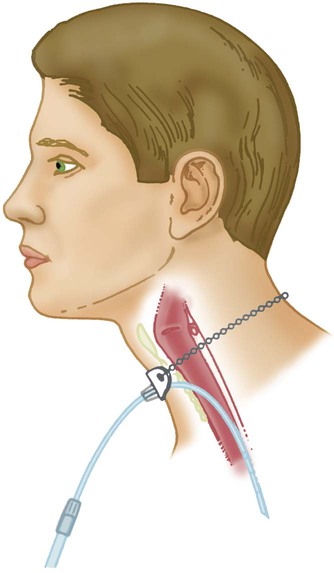
Because the transtracheal catheter resides directly in the trachea, O2 builds up both there and in the upper airway during expiration. This process effectively expands the anatomic reservoir and increases the FiO2 at any given flow. Compared with a nasal cannula, a transtracheal catheter needs about half of the O2 flow to achieve a given arterial partial pressure of oxygen (PaO2).25 Some patients need a flow of only 0.25 L/min to achieve adequate oxygenation. This reduced flow can be of great economic and practical benefit to patients needing continuous long-term O2 therapy because it can greatly increase the duration of flow of portable O2 systems. Transtracheal O2 therapy can pose problems and risks, however, and these devices have not received widespread acceptance. Careful patient selection, rigorous patient education, and ongoing self-care with professional follow-up evaluation can help minimize these risks. Chapter 51 provides details on these aspects of transtracheal O2 therapy. Table 38-3 lists the FiO2 range, FiO2 stability, advantages, disadvantages, and best use of a transtracheal catheter.
Performance Characteristics of Low-Flow Systems
Research studies on nasal low-flow systems show O2 concentration ranging from 22% at 1 L/min to 60% at 15 L/min.2,3,22 The range of 22% to 45% cited in Table 38-3 is based on 8 L/min as the upper limit of comfortable flow. These wide FiO2 ranges occur because the O2 concentration delivered by a low-flow system varies with the amount of air dilution. The amount of air dilution depends on several patient and equipment variables. Table 38-4 summarizes these key variables and how they affect FiO2 provided by low-flow systems.
TABLE 38-4
Variables Affecting FiO2 of Low-Flow Oxygen Systems
Increases FiO2 | Decreases FiO2 |
Higher O2 input | Lower O2 input |
Mouth-closed breathing* | Mouth-open breathing* |
Low inspiratory flow | High inspiratory flow |
Low tidal volume | High tidal volume |
Slow rate of breathing | Fast rate of breathing |
Small minute ventilation | Large minute ventilation |
Long inspiratory time | Short inspiratory time |
High I : E ratio | Low I : E ratio |
Troubleshooting Low-Flow Systems
Common problems with low-flow O2 delivery systems include inaccurate flow, system leaks and obstructions, device displacement, and skin irritation. The problem of inaccurate flow is greatest when low-flow flowmeters (≤3 L/min) are used. Given the trend toward assessment of outcome of O2 therapy (with either blood gases or pulse oximetry), ensuring the absolute accuracy of O2 input flow generally is not essential. Nonetheless, similar to all respiratory care equipment, flowmeters should be subjected to regular preventive maintenance and testing for accuracy. Equipment that fails preventive maintenance standards should be removed from service and repaired or replaced. Table 38-5 provides guidance on troubleshooting the most common clinical problems with nasal cannulas. Details on troubleshooting transtracheal catheters are provided in Chapter 51.
TABLE 38-5
Troubleshooting Common Problems With a Nasal Oxygen Cannula
Problem or Clue | Cause | Solution |
No gas flow can be felt coming from the cannula | Flowmeter not on System leak |
Adjust flowmeter Check connections |
Humidifier pop-off is sounding | Obstruction distal to humidifier | Find and correct the obstruction |
Flow is set too high | Use alternative device | |
Obstructed naris | Use alternative device | |
Patient reports soreness over lip or ears | Irritation or inflammation caused by appliance straps | Loosen straps |
Place cotton balls at pressure points | ||
Use a different device | ||
Mouth breathing | Habitual mouth breathing, blocked nasal passages | Switch to simple mask or Venturi mask |
Reservoir Systems
Reservoir Cannula
Reservoir cannulas are designed to conserve O2 and are an alternative to the pulse-dose or demand-flow O2 systems described in Chapter 51. There are two types of reservoir cannula: nasal reservoir and pendant reservoir. Table 38-3 lists the FiO2 range, FiO2 stability, advantages, disadvantages, and best use of a reservoir cannula.
A nasal reservoir cannula operates by storing approximately 20 ml of O2 in a small membrane reservoir during exhalation (Figure 38-8). The patient draws on this stored O2 during early inspiration. The amount of O2 available increases with each breath and decreases the flow needed for a given FiO2. Although the device is comfortable to wear, many patients object to its appearance and may not always comply with prescribed therapy.
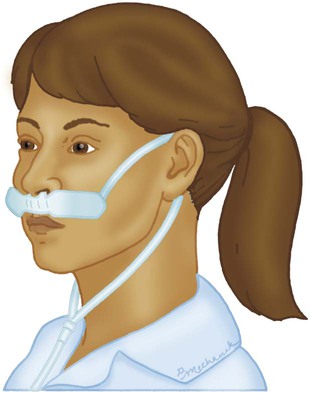
The pendant reservoir system helps overcome esthetic concerns by hiding the reservoir under the patient’s clothing on the anterior chest wall (Figure 38-9). Although the device is less visible, the extra weight of the pendant can cause ear and facial discomfort.
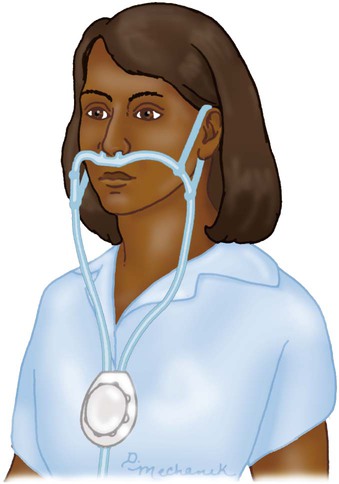
At low flow, reservoir cannulas can reduce O2 use 50% to 75%. A patient at rest who needs 2 L/min through a standard cannula to achieve an arterial oxygen saturation (SaO2) greater than 90% may need only 0.5 L/min through a reservoir cannula to achieve the same blood oxygenation. During exercise, reservoir cannulas can reduce flow needs approximately 66%; the savings is approximately 50% at high flow.26
Although flow savings is predictable, factors such as nasal anatomy and breathing pattern can affect the performance of the device. For these devices to function properly at low flow, patients must exhale through the nose (this reopens or resets the reservoir membrane). In addition, exhalation through pursed lips may impair performance, especially during exercise. For these reasons, prescribed flow settings should be individually determined by means of clinical assessment, including SaO2 monitoring, during rest and exercise.26
The low flow at which the reservoir cannula operates makes humidification unnecessary. Excess moisture can hinder proper action of the reservoir membrane.26 Even regular use can cause membrane wear. For this reason, patients should replace the reservoir cannula approximately every 3 weeks. Replacement needs partially offset the O2 cost savings afforded by these devices.
Reservoir Masks
Masks are the most commonly used reservoir systems. There are three types of reservoir masks: (1) simple mask, (2) partial rebreathing mask, and (3) nonrebreathing mask. Table 38-3 lists the FiO2 range, FiO2 stability, advantages, disadvantages, and best use of each of these devices.
A simple mask is a disposable plastic unit designed to cover both the mouth and the nose (Figure 38-10). The body of the mask itself gathers and stores O2 between patient breaths. The patient exhales directly through open holes or ports in the mask body. If O2 input flow ceases, the patient can draw in air through these holes and around the mask edge.
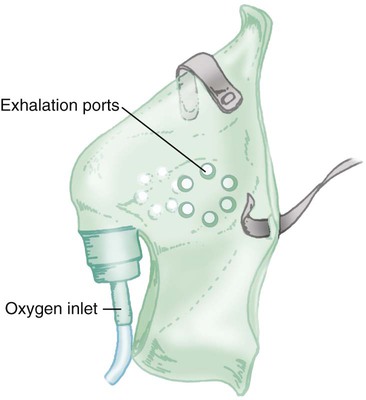
The input flow range for an adult simple mask is 5 to 10 L/min. Generally, if flow greater than 10 L/min is needed for satisfactory oxygenation, use of a device capable of a higher FiO2 should be considered. At a flow less than 5 L/min, the mask volume acts as dead space and causes carbon dioxide (CO2) rebreathing.27
Because air dilution easily occurs during inspiration through its ports and around its body, a simple mask provides a variable FiO2. How much FiO2 varies depends on the O2 input flow, the mask volume, the extent of air leakage, and the patient’s breathing pattern.28
As shown in Figure 38-11, a partial rebreathing mask and a nonrebreathing mask have a similar design. Each has a 1-L flexible reservoir bag attached to the O2 inlet. Because the bag increases the reservoir volume, both masks provide higher FiO2 capabilities than a simple mask. The key difference between these designs is the use of valves. A partial rebreathing mask has no valves (see Figure 38-11, A). During inspiration, source O2 flows into the mask and passes directly to the patient. During exhalation, source O2 enters the bag. However, because no valves separate the mask and the bag, some of the patient’s exhaled gas also enters the bag (approximately the first third). Because it comes from the anatomic dead space, the early portion of exhaled gas contains mostly O2 and little CO2. As the bag fills with both O2 and dead space gas, the last two-thirds of exhalation (high in CO2) escapes out the exhalation ports of the mask. As long as the O2 input flow keeps the bag from collapsing more than about one-third during inhalation, CO2 rebreathing is negligible.
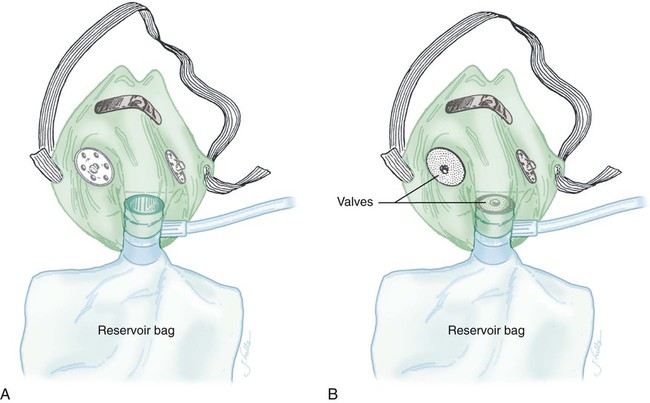
Although it can provide a higher FiO2 than a simple mask (see Table 38-3), a standard disposable partial rebreathing mask is subject to considerable air dilution. The result is delivery of a moderate but variable FiO2 dependent on the same factors as with a simple mask.
A nonrebreathing mask prevents rebreathing with one-way valves (see Figure 38-11, B). An inspiratory valve sits on top of the bag, and expiratory valves cover the exhalation ports on the mask body. During inspiration, slight negative mask pressure closes the expiratory valves, preventing air dilution. At the same time, the inspiratory valve on top of the bag opens, providing O2 to the patient. During exhalation, valve action reverses the direction of flow. Slight positive pressure closes the inspiratory valve, which prevents exhaled gas from entering the bag. Concurrently, the one-way expiratory valves open and divert exhaled gas out to the atmosphere.
Because it is a closed system, a leak-free nonrebreathing mask with competent valves and enough flow to prevent more than one-third bag collapse during inspiration can deliver 100% source gas. As indicated in Table 38-3, however, modern disposable nonrebreathing masks normally do not provide much more than approximately 70% O2.22
Nonrebreathing Reservoir Circuit
A nonrebreathing circuit operates with the same design principles as a nonrebreathing mask. Although the nonrebreathing circuit requires an elaborate combination of equipment and supplies, it can be more versatile than a nonrebreathing mask because it provides a full range of FiO2 (21% to 100%) and can be used for both intubated and nonintubated patients.22 As shown in Figure 38-12, a typical nonrebreathing circuit incorporates a blending system to premix air and O2. The gas mixture is warmed and humidified, ideally with a servo-controlled heated humidifier. Gas flows through large-bore tubing into an inspiratory volume reservoir, which includes a fail-safe inlet valve. The patient breathes through a closed airway appliance, in this case, a mask with one-way valves. A valved T tube also can be used in the care of a patient with an endotracheal or a tracheostomy tube.
Troubleshooting Reservoir Systems
Common problems with reservoir masks include device displacement, system leaks and obstructions, improper flow adjustment, and skin irritation. Table 38-6 provides guidance on troubleshooting the most common clinical problems with reservoir masks.
TABLE 38-6
Troubleshooting Common Problems With Reservoir Masks
Problem or Clue | Cause | Solution |
Patient constantly removes mask | Claustrophobia | Use alternative device |
Confusion | Restrain patient | |
No gas flow can be detected | Flowmeter not on | Adjust flowmeter |
System leak | Check connections | |
Humidifier pop-off is sounding | Obstruction distal to humidifier | Find and correct obstruction |
High input flow | Omit humidifier if therapy is short-term | |
Jammed inspiratory valve | Fix or replace valve | |
Reservoir bag collapses when the patient inhales | Flow is inadequate | Increase flow |
Reservoir bag remains inflated throughout inhalation | Large mask leak | Correct leak |
Inspiratory valve jammed or reversed | Repair or replace mask | |
Erythema develops over face or ears | Irritation or inflammation owing to appliance or straps | Reposition mask or straps |
Place cotton balls over ear pressure points | ||
Provide skin care |
High-Flow Systems
Principles of Gas Mixing
< ?xml:namespace prefix = "mml" />

Box 38-1 shows how to apply variations of this equation to compute (1) the final concentration of a mixture of air and O2, (2) the air-to-O2 ratio needed to obtain a given FiO2, (3) the total output flow from an air-entrainment device, and (4) the amount of O2 that must be added to a volume of air to obtain a given FiO2. Clinical examples of these computations are provided in the accompanying Mini Clini boxes.
Air-Entrainment Systems
Air-entrainment systems direct a high-pressure O2 source through a small nozzle or jet surrounded by air-entrainment ports (Figure 38-13). The amount of air entrained at these ports varies directly with the size of the port and the velocity of O2 at the jet. The larger the intake ports and the higher the gas velocity at the jet, the more air is entrained.
FiO2 provided by air-entrainment devices depends on two key variables: the air-to-O2 ratio and the amount of flow resistance downstream from the mixing site. Changing the input flow of an air-entrainment device alters the total output flow but has little effect on delivered FiO2. Generally, FiO2 remains within 1% to 2% of that specified by the manufacturer, regardless of input flow.29
Rather than using Equation 38-2 in Box 38-1 to compute air-to-O2 ratio, many RTs derive quick estimates by using a simple mathematical aid called the magic box (Figure 38-14). To use the magic box, one draws a square and places 20 in the top left corner and 100 in the bottom left corner. One places the desired O2 percentage in the center of the box (in this case, 70%). One subtracts diagonally from lower left to the upper right (disregard the sign). One subtracts diagonally again from upper left to lower right (disregard the sign). The resulting numerator (30) is the value for air, and the denominator (50) is the value for O2.
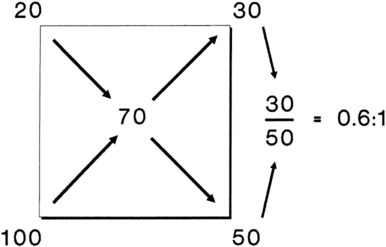
By convention, the air-to-O2 ratio is expressed with the denominator (liters of O2) set to 1. An air-entrainment device with a 7 : 1 ratio mixes 7 L of air with 1 L of O2. To reduce any ratio to a ratio of x:1, one divides both the numerator and the denominator by the denominator. In the magic box example (also see Figure 38-14):
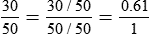
The magic box can be used only for estimation of air-to-O2 ratio. For absolute accuracy, Equation 38-2 always should be used. Based on Equation 38-2, Table 38-7 lists the approximate air-to-O2 ratios for several common O2 percentages.
TABLE 38-7
Approximate Air-to-Oxygen Ratios for Common Oxygen Concentrations*
Percentage O2 | Approximate Air-to-O2 Ratio | Total Ratio Parts |
100 | 0 : 1 | 1 |
80 | 0.3 : 1 | 1.3 |
70 | 0.6 : 1 | 1.6 |
60 | 1 : 1 | 2 |
50 | 1.7 : 1 | 2.7 |
45 | 2 : 1 | 3 |
40 | 3 : 1 | 4 |
35 | 5 : 1 | 6 |
30 | 8 : 1 | 9 |
29 | 10 : 1 | 11 |
24 | 25 : 1 | 26 |
*Total output flow (air + O2) in L/min can be calculated by multiplying the total ratio parts by the O2 input flow (L/min).
Although the delivered O2 concentration increases, the actual FiO2 received by the patient may decrease, especially on devices set to deliver 30% to 50% O2.29 This phenomenon is caused mainly by the decrease in total output flow. As the total output flow decreases below the flow needed to meet the patient’s inspiratory needs, room air is inhaled. A similar event occurs if the air intake ports surrounding the jet are blocked. Under both conditions, these high-flow systems begin to behave as low-flow devices. The two most common O2 delivery systems in which air entrainment is used are the air-entrainment mask (AEM) and the air-entrainment nebulizer.
Air-Entrainment (Venturi) Mask
The use of an O2 mask for provision of controlled FiO2 by means of air entrainment was first reported in 1941 by Barach and Eckman.30 The system provided relatively high FiO2 (>40%) through the use of adjustable air-entrainment ports that controlled the amount of air mixed with O2. Almost 20 years later, Campbell31 developed an entrainment mask that provided controlled, low FiO2 and called the device a Venturi mask or venti-mask.
As the name venti-mask suggests, the operating principle behind these devices has often been attributed to the Venturi principle (see Chapter 6). This assumption is incorrect.32 Rather than having an actual Venturi tube that entrains air, these devices have a simple restricted orifice or jet through which O2 flows at high velocity. Air is entrained by shear forces at the boundary of jet flow, not by low lateral pressures. The smaller the orifice, the greater the velocity of O2, and more air is entrained.
Figure 38-15 depicts a typical AEM, designed to deliver a range of low to moderate FiO2 (0.24 to 0.40). The mask consists of a jet orifice or nozzle around which is an air-entrainment port (top drawing). The body of the mask has several large ports, which allow escape of both excess flow from the device and exhaled gas from the patient. In this design, FiO2 is regulated by selection and changing of the jet adapter. The smallest jet provides the highest O2 velocity, the most air entrainment, and the lowest FiO2 (0.24). The largest jet provides the lowest O2 velocity, the least air entrainment, and the highest FiO2 (0.40). Other AEM designs may vary both jet and entrainment port size to provide an even broader range up to 50% FiO2. The aerosol entrainment collar fits over the air-entrainment ports (see later).
For controlled FiO2 at flow high enough to prevent air dilution, the total output flow of an AEM must exceed the patient’s peak inspiratory flow.29 With an entrainment ratio exceeding 5 : 1, an AEM set to deliver less than 35% O2 has little trouble meeting or exceeding the 60 L/min high-flow criterion (see previous Rule of Thumb). At settings greater than 35%, total AEM flow decreases significantly, and FiO2 becomes variable. For example, when set to deliver 50% O2, some AEMs provide 0.39 FiO2.32–34
Air-Entrainment Nebulizer
Because of added humidification and heat control, air-entrainment nebulizers have been the traditional device of choice for delivering O2 to patients with artificial tracheal airways. O2 typically is delivered with a T tube or a tracheostomy mask. An alternative is to use an aerosol mask or a face tent to deliver an O2 mixture via aerosol to patients with intact upper airways (Figure 38-16).35
AEMs can vary both jet and entrainment port size to obtain a given FiO2; however, gas-powered nebulizers have a fixed orifice. Air-to-O2 ratios can be altered only by varying entrainment port size. Disposable nebulizers usually have a continuous range of settings from 28% to 100%. Less commonly used nondisposable nebulizers have fixed entrainment settings, such as 100%, 70%, and 40%.22
Similar to AEMs, air-entrainment nebulizers perform as fixed-performance devices only when output flow meets or exceeds the patient’s inspiratory demand. In contrast to AEMs, air-entrainment nebulizers do not allow easy increases in nebulizer output flow by means of an increase in O2 input. With most nebulizer systems, the extremely small size of the jet needed for aerosol production limits the maximum O2 input flow to 12 to 15 L/min at 50 psig. For example, the total output flow of an air-entrainment nebulizer set to deliver 40% O2 ranges from 48 to 60 L/min. Although this amount may be adequate for most patients, it is insufficient for patients with very high inspiratory flow or minute volume.35
The actual FiO2 received by patients may be affected by the choice of airway appliance. The FiO2 delivered by face tent is consistently less than the set nebulizer concentration, especially at higher levels.36
Air-entrainment nebulizers should be treated as fixed-performance devices only when set to deliver low O2 concentration (≤35%).33 When a nebulizer is used to deliver a higher concentration of O2, the RT must determine whether the flow is sufficient to meet patient needs. There are two ways to assess whether the flow of an air-entrainment nebulizer meets the patient’s needs. The first method is simple visual inspection. With this approach (generally used only with a T tube), the RT sets up the device to deliver the highest possible flow at the prescribed FiO2. After connecting the system to the patient, the RT observes the mist output at the expiratory side of the T tube. As long as mist can be seen escaping throughout inspiration, flow is adequate to meet the patient’s needs, and the delivered FiO2 is ensured.
The second way to assess the adequacy of nebulizer flow is to compare it with the patient’s peak inspiratory flow. A patient’s peak inspiratory flow during tidal breathing is approximately three times minute volume. As long as the nebulizer flow exceeds this value, the delivered FiO2 is ensured. If the patient’s peak flow exceeds that provided by the nebulizer, the device functions as a low-flow system with variable FiO2 (see the accompanying Mini Clini for an example).35
Providing Moderate to High FiO2 at High Flow
This solution is impossible with most air-entrainment nebulizers. Because the small jets in many of these devices limit O2 flow to 12 to 15 L/min, the input flow cannot be increased beyond these levels. A few nebulizer models, such as the Thera-Mist Barrel Nebulizer (Smiths Medical, London, England), supposedly can provide moderately high output flows of 54 L/min at FiO2 80%. However, most air-entrainment nebulizers cannot because of the total flow-to-FiO2 tradeoff. The five alternatives for boosting the FiO2 capabilities in these situations are presented in Box 38-2.
The simplest approach to achieving higher FiO2 with these devices is to add a 50- to 150-ml aerosol tubing reservoir to the expiratory side of the T tube (Figure 38-17). Given its simplicity, adding an open volume reservoir to the expiratory side of T tubes is standard procedure in most clinical settings. This approach can be used only in the treatment of intubated patients. Even then, the small reservoir size limits the ability of this system to ensure stable FiO2, especially greater than 40%, and larger reservoirs can cause rebreathing.
Rather than a simple open reservoir, a closed reservoir or nonrebreathing system similar to that shown in Figure 38-12 can be used. These systems combine an inspiratory volume reservoir (usually a compliant 3- to 5-L anesthesia bag) with a one-way expiratory valve. Whenever patient flow exceeds nebulizer flow, the expiratory valve closes, and the patient draws additional gas from the reservoir. Although they can ensure delivery of the set O2 concentration, these systems pose considerable hazards. If source flow stops for any reason, the patient can suffocate. For this reason, these systems must be equipped with an emergency inlet valve that allows room air breathing in the event of source gas failure.
The third and most common approach to higher FiO2 with air-entrainment nebulizers is to connect two or more devices together with a “wye” adapter (Figure 38-18).22 Although a single air-entrainment nebulizer set at 60% (1 : 1 ratio) with a maximum input flow of 15 L/min has a total output flow of only 30 L/min, connecting two of these devices together doubles the total output flow to 60 L/min (the minimum needed for a high-flow device). This approach works well only for delivery of a concentration of 60% or less to patients with a minute volume less than 10 L/min.35,37
Commercial dual-flow systems entail a similar approach. One flow source powers the jet, while another flow source provides supplemental O2. The Misty Ox (Vital Signs, Inc, Totowa, New Jersey) gas injection nebulizer is an example. This system is not an air-entrainment system because it does not depend on entrainment ports to increase total flow or O2 concentration to the patient. Rather, it uses two flowmeters: one that operates the jet and one that feeds into the side of the jet manifold. The Misty Ox system can provide FiO2 of 0.96 at a flow of 42 L/min and offers O2 concentrations ranging from 0.21 to nearly 1.00.35
If aerosol is not needed, a simple dual-flow device such as the Downs flow generator (Figure 38-19) can be used. This device is attached to a 50-psig O2 source and provides O2 concentrations of 30% to 100% at a flow up to 100 L/min.38
Problems With Downstream Flow Resistance
Any increase in flow resistance downstream from (distal to) the point of air entrainment alters the performance of all air-entrainment systems. Increased downstream flow resistance causes back pressure. The back pressure decreases both the volume of entrained air and the total flow output of these devices. With less air entrained, the delivered O2 concentration increases; however, because total flow output also decreases, the effect on FiO2 varies. High downstream flow resistance usually turns air-entrainment systems from high-flow (fixed) O2 delivery systems into low-flow (variable) O2 delivery systems incapable of delivering a precise and constant FiO2.29
Blending Systems
Oxygen Blenders
Rather than manually mixing air and O2, the RT can use an O2 blender. Figure 38-20 shows the major components of a typical O2 blender. Air and O2 enter the blender and pass through dual pressure regulators that exactly match the two pressures. Gas flows to a precision proportioning valve. Because the two gas pressures at this point are equal, varying the size of the air and O2 inlets provides precise control over the relative concentration.
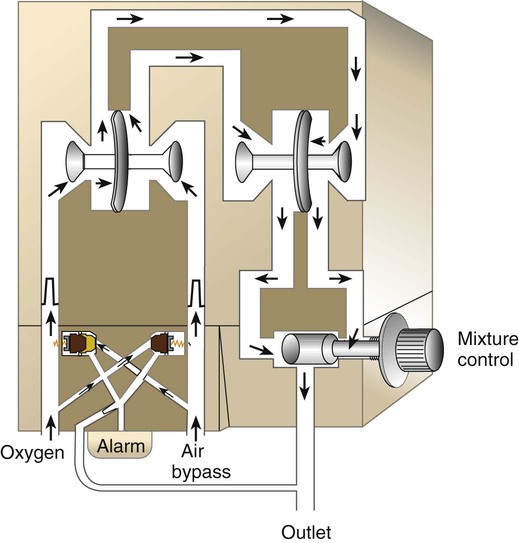
Although they allow ideal control over both FiO2 and flow, blenders are especially prone to inaccuracy and failure.39,40 To avoid these problems, the RT always should conduct an operational check of any blender before using it on a patient (Box 38-3). FiO2 should be checked and confirmed with a calibrated O2 analyzer at least once per shift.2 As always, a device that does not perform according to expectations should be replaced immediately. When a blender is used in the care of a neonate, an O2 analyzer should be kept in-line at all times. In the use of a nonrebreathing or closed delivery system, (1) all breathing valves should be inspected and tested before application to a patient, and (2) a fail-safe inspiratory valve should be included in the delivery system.
Enclosures
Oxygen Tents
O2 tents previously were the most common method of O2 therapy in the treatment of both adults and children. Use of O2 tents in both adults and children is rare at the present time. However, when they are used, it is common for tents to be air-conditioned or cooled with ice to provide a comfortable temperature within a plastic sheet canopy (Figure 38-21).
Hoods
An O2 hood, also known as an oxyhood, is often the best method for administration of controlled O2 therapy to infants. As shown in Figure 38-22, an O2 hood covers only the head, leaving the infant’s body free for nursing care. O2 is delivered to the hood through either a heated air-entrainment nebulizer or a blending system with a heated humidifier. A minimum flow of 7 L/min should be set to prevent accumulation of CO2.41 Depending on the size of the hood, flow of 10 to 15 L/min may be needed to maintain stable high O2 concentration. Higher flow generally is not needed and may produce a harmful noise level and additional stress on neonatal patients.42
In the care of premature infants, it is especially important to ensure that the gas mixture is properly warmed and humidified and not directed toward the patient’s face or head. Low temperatures or convection cooling produced by high flow over the head can cause heat loss and cold stress. In premature infants, cold stress can increase O2 consumption and cause apnea.43
The temperature of gases provided to an infant in an O2 hood should be precisely set to maintain a neutral thermal environment (NTE). The NTE temperature varies according to an infant’s age and weight. The NTE temperature for newborns weighing less than 1200 g is 95° F (35° C). For older infants weighing 2500 g or more, the NTE is lower, approximately 86° F (30° C).43 The importance of temperature regulation in infants is discussed in more detail in Chapter 48.
Incubators
Incubators, also known by the trade name Isolette (Dräger Medical AG & Co, Lübeck, Germany) are polymethyl methacrylate (Plexiglas) enclosures that combine servo-controlled convection heating with supplemental O2 (Figure 38-23). In older models, humidification was provided with a blow-over water reservoir located under the patient platform. Because of the high infection risk associated with this design, these systems are no longer in common use. When it is needed, supplemental humidity usually is provided with an external heated humidifier or nebulizer.
Supplemental O2 can be administered with a direct connection between the incubator and a flowmeter that has a heated humidifier. In some units, a filtered air-entrainment device limits the delivered concentration to approximately 0.40. However, leaks and frequent opening of the isolette dilute the O2 levels to much less than 40%. Blockage of the inlet filter can cause less air entrainment and a higher O2 concentration.43
Given the highly variable O2 concentration provided by these devices, the best way to control O2 delivery to infants in an isolette is with an oxyhood. The oxyhood is placed over the infant’s head inside the isolette. The O2 concentration and gas temperature within the oxyhood, not in the isolette, must be assessed. It is ideal to monitor isolette or oxyhood O2 concentration continuously (see later).2,4
Because hoods allow better FiO2 control, and because servo-controlled radiant heating warmers are generally more convenient, Plexiglas isolettes are not as popular as they used to be. However, these devices are still the best choice for providing O2 to infants in stable condition with a NTE.43
Other Oxygen Delivery Devices
High-Flow Nasal Cannula
A variation of the standard nasal cannula discussed earlier in this chapter is a high-flow nasal cannula. Various systems are available, including the system introduced by Salter Labs (Arivan, California), which provides nonheated and humidified O2 flows up to 15 L/min to achieve a maximum FiO2 greater than 60% to 70%. Another is the Vapotherm 2000i system (Annapolis, Maryland), which can deliver both FiO2 and relative humidity greater than 90% by using heated, humidified O2 flows up to 40 L/min. These systems have been shown to improve oxygenation by providing high FiO2 and positive pressure to the airway. Such high-flow systems have proven to be effective in treating patients of all ages in acute respiratory failure and have become a common alternative to traditional continuous positive airway pressure devices for preterm infants with disorders including apnea of prematurity and bronchopulmonary dysplasia. However, a major limitation of these devices is that it is generally impossible to determine precisely and monitor the level of positive pressure actually applied.35
Bag-Mask Devices
There are other ways to administer O2 in selected settings in addition to the previously described systems. Bag-mask devices use a self-inflating bag and nonrebreathing valve features to provide up to 100% O2. Bag-mask devices are often used in emergency life support and in critical care and are more completely discussed in Chapter 34.
Demand-Flow and Pulse-Dose Systems
Finally, specialized O2 delivery systems are common in non–acute care settings. These include demand-flow or pulse-dose systems that use a flow sensor and valve to synchronize gas delivery with inspiration. These devices can substantially extend duration of flow of a liquid or gaseous O2 tank and are popular in home care. Demand-flow systems and stand-alone O2 sources such as O2 concentrators and liquid O2 systems that are common in alternative settings are described in Chapter 51.
Selecting a Delivery Approach
With the various techniques available for giving O2, there is no one best delivery method. Although the decision to give O2 is a medical one, it is not always easy for the physician to know exactly which approach is the best for a given patient. For this reason, the RT should be involved in the initial selection of an appropriate delivery system. The RT also should be responsible for ongoing oversight of the prescribed therapy. This responsibility should include making recommendations—on the basis of sound patient assessment—to change or discontinue the treatment regimen (see later section on Protocol-Based Oxygen Therapy).
Patient
Key patient considerations in selecting O2 therapy equipment for use in acute care are summarized in Box 38-4. Knowledge of these factors helps guide the RT in selecting the appropriate equipment. For example, a simple mask at 5 to 6 L/min is probably more suitable than a nasal cannula at 4 L/min for a mouth breathing, mildly hypoxemic patient. An infant with moderate hypoxia and a normal airway usually needs an O2 enclosure (hood or enclosed incubator).
Performance
O2 systems vary according to actual FiO2 delivered and stability of FiO2 under changing patient demands. Generally, the more critically ill the patient, the greater the need for a stable, high FiO2. Less acutely ill patients generally need a lower, less exact FiO2. Table 38-8 lists guidelines for selecting an O2 delivery system on the basis of the level and stability of the FiO2 needed.
TABLE 38-8
Selection of an Oxygen Delivery System Based on Desired FiO2 Level and Stability
Desired FiO2 Level | Desired FiO2 Stability | |
Fixed | Variable | |
Low (<35%) | AEM | Nasal cannula |
Air-entrainment nebulizer | Nasal catheter | |
Blending system | Transtracheal catheter | |
Isolette, incubator (infant) | ||
Moderate (35%-60%) | Air-entrainment nebulizer | Simple mask |
Blending system | Air-entrainment nebulizer | |
Oxyhood (infant) | Tent (child) | |
High (>60%) | Blending system | Partial rebreather |
Oxyhood (infant) | Nonrebreather |
General Goals and Patient Categories
Adult patients with chronic lung disease and accompanying acute-on-chronic hypoxemia present a special case. In the care of these patients, the goal is to ensure adequate arterial oxygenation without depressing ventilation. Adequate oxygenation of these patients generally means SaO2 of 85% to 92% with PaO2 of 50 to 70 mm Hg.31,44 These values usually are achieved with either low-flow nasal O2 or a low-concentration (24% to 28%) AEM. The less stable the patient’s condition, the greater the need for a high-flow AEM.22
Because of size, discomfort, and appearance, AEMs are less well tolerated than nasal cannulas for long-term therapy. In contrast to a cannula, an AEM must be removed for eating and drinking. Because even a short break in O2 therapy can cause a rapid decrease in PaO2 in some patients, these patients should be taught to switch to a nasal cannula whenever they must remove the mask.22
Protocol-Based Oxygen Therapy
O2 therapy is ideally suited for a protocol. Bedside assessment of oxygenation by RTs and other clinicians has progressed to where it is more cost-effective and clinically appropriate to use a protocol rather than obtaining a new physician order for each change in FiO2. An order for “O2 therapy via protocol” permits O2 therapy to be initiated, modified, or discontinued by the RT, provided that an assessment reveals that the patient meets previously approved clinical criteria. A well-designed O2 protocol ensures the patient (1) undergoes initial assessment, (2) is evaluated for protocol criteria, (3) receives a treatment plan that is modified according to need, and (4) stops receiving therapy as soon as it is no longer needed.45
Figure 38-24 shows the decision algorithm underlying an O2 therapy titration protocol developed at the Cleveland Clinic Foundation. In the algorithm, a pulse oximetry saturation (SpO2) of 92% is the threshold value that indicates the need for therapy. As indicated in the algorithm, the titration is bypassed if a patient already has signs of hypoxemia, in which case the patient immediately receives supplemental O2. Otherwise, the level of supplemental O2 is adjusted, and the patient is reassessed on each shift for determination of continuing need. When the SpO2 is 92% or greater on room air, therapy is discontinued.
Hyperbaric Oxygen Therapy
Hyperbaric oxygen (HBO) therapy is the therapeutic use of O2 at pressures greater than 1 atm.46–48 Pressures during HBO therapy usually are expressed in multiples of atmospheric pressure absolute (ATA): 1 ATA equals 760 mm Hg (101.32 kPa). Most HBO therapy is conducted at pressures between 2 ATA and 3 ATA, although other pressures may be used, often based on U.S. Navy diving treatment tables.47–49
Physiologic Effects
The known physiologic effects of HBO therapy are summarized in Box 38-5.46 These effects are mainly due to either high pressure or high O2 tension in body fluids and tissues. In conditions such as air embolism and decompression sickness, high pressure exerts a physical effect on air or nitrogen bubbles trapped in the blood or tissues. According to Boyle’s law, high pressure decreases the size of these bubbles and minimizes potential harm. Because pressure is crucial in these cases, HBO treatments may be conducted at 6 ATA or more.47,49
The second beneficial effect of HBO is hyperoxia. When a patient is breathing room air, only a small amount of O2 dissolves in the plasma (approximately 0.3 ml/dl). At 3 ATA, plasma contains nearly 7 ml/dl dissolved O2, a level exceeding average resting tissue uptake.47
Hyperoxia also helps form new capillary beds, a process called neovascularization. Although the exact mechanism is unknown, neovascularization is an essential component of tissue repair, especially in radiation-induced injuries.47,48
Study results suggest that HBO may be useful in many other conditions, including the management of stroke, wound healing, and treating stubborn soft tissue infections. Because HBO has emerged as a highly effective therapy for various conditions, government and many private health insurance providers have expanded coverage for HBO. Likewise, many hospitals now offer HBO therapy, and opportunities in this area have increased for RTs.50,51
Methods of Administration
HBO is administered in either a multiplace or a monoplace chamber. A multiplace chamber is a large tank capable of holding a dozen or more people (Figure 38-25, A). Because patients are directly cared for by medical staff inside the tank, multiplace chambers have air locks that allow entry and exit without altering the pressure. The multiplace chamber is generally filled with air. If indicated, only the patient breathes supplemental O2 (through a mask or another device). Because they can achieve pressures of 6 ATA or more, multiplace chambers are ideal for the management of decompression sickness and air embolism.47–49
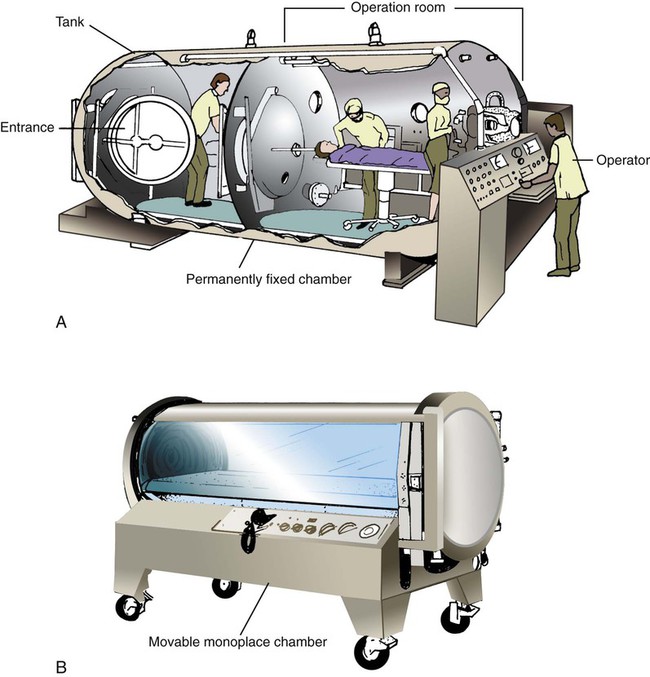
A typical monoplace chamber consists of a transparent Plexiglas cylinder large enough only for a single patient (see Figure 38-25, B). During therapy, the cylinder O2 concentration is kept at 100%. The patient need not wear a mask. Because of the high O2 concentration, most electronic equipment cannot be used in a monoplace chamber. In addition, many ventilators do not function properly under these conditions. However, monitoring systems and ventilators can be adapted to allow treatment of a critically ill patient with hyperbaric pressure. Additionally, artificial airways suited to function properly under hyperbaric conditions should be used.46
Indications
HBO has long been accepted as the primary treatment of divers with decompression sickness. Several other of the most common indications for HBO therapy are listed in Box 38-6.46,48 The two most common acute conditions for which RTs administer HBO are air embolism and carbon monoxide poisoning.46,49,52
Air Embolism
Air embolism is a complication that can occur with certain cardiovascular procedures, lung biopsy, hemodialysis, and central line placement. Air bubbles that reach the cerebral or cardiac circulation can cause severe neurologic symptoms or sudden death. HBO decreases the volume of air bubbles and helps oxygenate local tissues. Typical therapy for air embolism involves immediate pressurization in air to 6 ATA for 15 to 30 minutes. This step is followed by decompression to 2.8 ATA with prolonged O2 treatment.47–49
Carbon Monoxide Poisoning
Carbon monoxide poisoning accounts for about half of all poisoning deaths in the United States. The condition of a patient with carbon monoxide poisoning improves quickly with HBO treatment because this treatment is the fastest way to remove carbon monoxide from the blood.52 If a patient breathes air, it takes more than 5 hours to remove only one half of the carboxyhemoglobin in the blood. Breathing 100% O2 reduces this “half-life” to 80 minutes. The half-life of carboxyhemoglobin under HBO at 3 ATA is only 23 minutes. Box 38-7 lists the major criteria for selecting patients with acute carbon monoxide poisoning for treatment with HBO.52
Complications and Hazards
Although the benefits of HBO are significant, this type of therapy also has significant risks. As a result, the benefits should be compared with the hazards before therapy is initiated. Common complications of HBO are listed in Box 38-8.47 These complications are generally caused by high pressure, O2 toxicity, fire, or worsening of certain existing conditions. The most frequent problems involve barotrauma to closed body cavities, such as the middle ear or sinuses. Pneumothorax and air embolism also are possible during HBO treatment but are rare in patients with normal lungs.
O2 at high pressure can be neurotoxic. Early signs of impending CNS toxicity include twitching, sweating, pallor, and restlessness. These signs usually are followed by seizures and convulsions. However, CNS toxicity rarely occurs with the pressures and treatment times commonly used for clinical HBO therapy.48
In terms of pulmonary O2 toxicity, HBO treatments do not normally expose patients to high PO2 long enough to cause damage. However, HBO may have an additive effect on critically ill patients who receive high FiO2 between HBO treatments.47,48
There are numerous relative contraindications for HBO, many of which relate to the potential complications and hazards noted earlier. For patients with inner ear infections and seizure disorders, which represent relative contraindications, the risks of HBO should be carefully weighed against the benefits. Absolute contradictions include an untreated pneumothorax and congenital heart defects resulting in dependency on a patent ductus arteriosus for survival. Such patients generally should not receive HBO therapy.48
Troubleshooting
Although fire hazards restrict the use of certain electronic equipment, some state-of-the-art monitors and ventilators with solid-state circuitry can be used within the chamber. This equipment allows intensive care of critically ill patients.46
In regard to ventilator use, reductions in delivered tidal volume should be expected and corrected. Additionally, tracheostomy or endotracheal tubes with foam or fluid-filled cuffs should generally be used to preserve cuff integrity under pressure. If not accounted for, reduced tidal volumes and leaks can lead to respiratory hypercapnia and acidosis. Hypercapnia can result in respiratory acidosis and can worsen CNS toxicity owing to cerebral vasodilation.48 Generally, pressure-regulating and flow-regulating equipment used in a hyperbaric chamber must be specifically designed for operation at chamber pressure or appropriately modified to the additional barometric pressure exerted.
Other Medical Gas Therapies
Nitric Oxide Therapy
Mode of Action
NO gas is a colorless, odorless, highly diffusible, and lipid-soluble free radical that oxidizes quickly to nitrogen dioxide (NO2) in the presence of O2. NO is normally produced in the human body from l-arginine in a reaction catalyzed by the enzyme NO synthase. NO activates guanylate cyclase, which catalyzes the production of cyclic guanosine 3′,5′-monophosphate (cGMP). The end result is that increased cGMP levels cause vascular smooth muscle relaxation.53
The effects of inhaled NO are limited to the pulmonary circulation. After diffusing into the capillaries, free NO immediately binds to hemoglobin, forming nitrosylhemoglobin. Nitrosylhemoglobin is rapidly oxidized to methemoglobin, which eventually undergoes conversion to reduced hemoglobin.53
Indications
After several years of clinical testing, inhaled NO was approved in December 1999 by the U.S. Food and Drug Administration (FDA) for treating selected neonates. Specifically, NO, in conjunction with such therapies as ventilatory support, has been approved for the treatment of term and near-term (>34 weeks) neonates with hypoxic (type I) respiratory failure with associated pulmonary hypertension. As a result of the clinical benefits of reduced pulmonary vascular resistance, improved oxygenation, and less need for a highly invasive method for increasing tissue oxygenation known as extracorporeal membrane oxygenation, inhaled NO is emerging as the standard of care for near-term neonates with this type of respiratory failure.54
In adults, studies have shown that inhaled NO has been effective in treating pulmonary hypertension associated with acute respiratory distress syndrome (ARDS). However, these benefits seem to be short-lived, and no significant improvement in clinical outcomes, including mortality, has been shown to date. As a result of these findings and lower cost alternative drug therapies, including inhaled epoprostenol sodium (Flolan) and similar medications (discussed in Chapter 32), the use of inhaled NO in adults has neither been approved by the FDA nor gained widespread acceptance, However, potential indications and various delivery methods for inhaled NO continue to be examined. Potential indications for inhaled NO are listed in Box 38-9.54,55
Dosing
The amount of NO needed to improve oxygenation or decrease pulmonary vascular pressure in neonates is relatively low. The therapeutic range of NO is 2 to 20 ppm, and an initial dose of 20 ppm is commonly used. Treatment should be continued until underlying oxygenation desaturation has resolved. For many patients, dosages often can be reduced to less than 20 ppm at the end of 4 hours of initial treatment, as tolerated. At these levels, NO has minimal toxicity.54 In clinical trials, higher doses were not shown to be more effective and placed the patient at a higher risk of complications.53–55
Toxicity and Adverse Effects
The toxicity of NO is caused by its own direct action and its chemical by-products. In high concentrations (5000 to 20,000 ppm), NO causes acute pulmonary edema that can be fatal. Inhalation of a lower concentration has been associated with direct cellular damage and impaired surfactant production.55,56
Most of the toxic effects of NO are caused by its chemical by-products, especially NO2. NO2 is produced spontaneously whenever NO is exposed to O2. NO2 is more toxic than NO. Levels greater than 10 ppm can cause cell damage, hemorrhage, pulmonary edema, and death. The U.S. Occupational Safety and Health Administration has set the safety limit for NO2 exposure at 5 ppm. The clinical goal is to keep NO2 exposure less than 2 ppm during administration of NO.56
Potential adverse effects associated with NO therapy are listed in Box 38-10.56 A poor or paradoxical response to NO has been observed in some patients. Of patients with ARDS, 40% do not have initial improvement in oxygenation with NO therapy, and some patients have experienced more severe hypoxemia (probably because of a worsening ventilation/perfusion imbalance when no shunt was present). NO inhibits platelet agglutination, and the result is an antithrombotic effect. However, no significant increase in bleeding time has been reported in NO trials with human subjects. Because it can quickly reduce right ventricular afterload, NO may increase left ventricular filling pressure in some patients. In the presence of congestive heart failure, this effect could cause or worsen pulmonary edema. Concerns involving increased left heart pressures also account for inhaled NO being contraindicated for neonates with certain cardiac and vascular anomalies such as coarctation of the aorta.54 In certain patients, the withdrawal of NO has resulted in development of hypoxemia and pulmonary hypertension, perhaps worse than they were before therapy was started. This phenomenon is known as a rebound effect. This rebound effect occurs because the administration of nitric oxide depresses the body’s normal production of NO. NO after use for more than a few hours should always be slowly weaned over hours. When NO is finally discontinued, FiO2 frequently needs to be initially increased then slowly reduced to baseline over 1 or 2 hours.53
Although NO has been used safely with other drugs and treatments such as dopamine, steroids, surfactant, and high-frequency ventilation, the interaction of NO with other medications is still being studied. One investigational area may involve the study of patients receiving both inhaled NO and other NO-related compounds such as nitroglycerin and the possible development of methemoglobinemia or systemic hypotension. Likewise, the carcinogenic, mutagenic, and other adverse effects of NO are still under investigation.55
Methods of Administration
Before inhaled NO administration, the patient ideally should be stabilized as much as possible. To stabilize the patient, thought must be given to clinical considerations such as FiO2, blood pH, sedation, and possibly muscle relaxation. After the patient is prepared, NO is administered to mechanically ventilated patients through a system with the capability for operator-determined concentration of NO in the breathing gas, a constant concentration throughout the breathing cycle, and a concentration that does not cause generation of excessive inhaled NO2. Features of an ideal NO delivery system are listed in Box 38-11.
The INOmax DS (Delivery System) (Ikaria, Clinton, New Jersey) shown in Figure 38-26 provides these features.55,57 The INOmax DS delivers INOmax (NO for inhalation) into the inspiratory limb of the patient’s breathing circuit in a manner that provides a constant concentration of NO, as preset by the clinician, throughout inspiration. Through a dual-channel design, the first channel uses a delivery mechanism, flow controller, and injector module to ensure precise delivery of NO. The highly specialized injector module allows tracking of the ventilator flow waveforms and the delivery of synchronized and proportional dose of NO. The second channel uses a separate monitoring mechanism, electrochemical gas sensors, and a graphically enhanced user interface to monitor O2, NO2, and NO continuously. The INOmax DS uses several alarm systems to alert clinicians when problems arise, including alarms for high and low NO, high NO2, and high and low O2. Other alarms can be set to notify clinicians of a significant decrease in source gas pressure, if the electrochemical cells fail, and when calibration is required.58
The INOmax DS can also be interfaced with specialty ventilators including high-frequency oscillators and jet ventilators and anesthesia machines. Inhaled NO has also been used with noninvasive ventilation, but rebreathing inherent in this mode of ventilation can cause NO2 levels to increase beyond acceptable levels. Other special considerations apply, and resources such as ventilator procedure manuals and department policy and procedures should be reviewed to help ensure proper setup and patient safety.57
The administration of inhaled NO to spontaneously breathing patients is also possible. This use and some other uses are described in the research literature but have not yet officially been approved by the FDA and are regarded as off-label use. INOmax DS can be used with a nasal cannula and a face mask. The maximum delivered NO via cannula is generally 20 ppm. When titrating NO via cannula, it is recommended that the concentration not be increased by more than 5 ppm in a 5-minute period, and the clinician should be aware that excessive flow can cause back pressure leading to monitoring failure alarms. Inhaled NO delivery via face mask is similar to a cannula setup except that it is important to use a tight-fitting mask and a flow sufficient to prevent entrainment of air into the mask.57
Withdrawing Therapy
Care must be taken when NO therapy is withdrawn to prevent the rebound effect. First, the NO level should be reduced to the lowest effective dose (ideally ≤5 ppm). Second, the patient’s condition should be hemodynamically stable, and the patient should be able to maintain adequate oxygenation while breathing a moderate FiO2 (≤0.4) on low levels of positive end-expiratory pressure. Third, the patient should be hyperoxygenated (FiO2 0.6 to 0.7) just before discontinuation of NO inhalation. Preparation should also be made to provide hemodynamic support in the event the patient needs it. Close monitoring of patients and use of these measures usually avoid any untoward effect of NO withdrawal.54
Helium-Oxygen Therapy
Indications
The value of helium as a therapeutic gas is based solely on its low density. As detailed in Chapter 6, when flow is turbulent, driving pressure varies with the square of the flow. Because flow in the large airways is mainly turbulent, breathing a low-density gas mixture can decrease the driving pressure needed to move gas in and out of this area. With less pressure needed to move gas through the large airways, the patient’s work of breathing decreases. However, this effect is limited to large airway obstruction (flow in the small airways is not turbulent).
Helium-O2 has been used for more than 70 years as an adjunct tool in the management of large airway obstruction.59 Although the effectiveness of heliox therapy in treating conditions such as COPD is inconclusive, it has been shown to be effective in treating other obstructive disorders.60,61 Either alone or combined with other therapies such as bronchodilators, helium-O2 therapy has been shown to decrease the respiratory rate, the level of dyspnea, and the need for intubation and mechanical ventilation in patients with reversible obstructive disorders.62 Specifically, heliox therapy has yielded promising results in the management of acute upper airway obstruction of varying origin,63 postextubation stridor in pediatric trauma patients,64 acute severe asthma, and croup.65
Guidelines for Use
Heliox combinations can be prepared at the bedside, or commercially available cylinders of premixed gases can be used. The premixed cylinders are commonly available in an 80 : 20 or a 70 : 30 combination. The 70% helium and 30% O2 mixture has a density of 0.554 g/L and can provide additional O2 for the management of the hypoxemia that can occur with large airway obstruction. Other combinations such as 60 : 40 and 65 : 35 mixtures are being examined and show promising results.62,66
Several methods have been used to mix heliox at the bedside, although many are improvised setups. One method involves running a nebulizer connected to an O2 flowmeter at 10 L/min or more, bleeding in 100% helium via a small-bore connection, and titrating the flow to achieve the desired FiO2. An O2 analyzer with active alarms should always be used to measure continuously the FiO2 of the heliox mixture output flowing to the patient. Continued use of improvised setups or setups that are not approved by the FDA for heliox administration can place the patient, clinician, and hospital at risk. Devices cleared by the FDA for use with heliox should be integrated into clinical practice.62,67
The low-density benefit of heliox is the same attribute that presents challenges in selecting a delivery device. Because helium is highly diffusible, administration through low-flow systems such as a nasal cannula tends not to deliver sufficient concentrations to treat obstructive disorders in adults. However, heliox administered via cannulas with an adequate seal at the nares has shown to be effective in some infants.68 Generally, however, heliox should be delivered to most spontaneously breathing patients via a tight-fitting nonrebreathing mask with a fully functional valved exhalation port. The delivery system should be high flow, sufficient to meet or exceed the patient’s minute ventilation and peak inspiratory flow requirements. Closed systems with demand valves and reservoirs or the use of demand regulators has proved to be suitable for delivering heliox to patients with artificial airways.67
Helium mixtures can be given through a cuffed tracheal airway with a positive-pressure ventilator. However, the performance of ventilators in delivering heliox tends to vary significantly by model, and only some of them have received FDA clearance for such use. Consequently, RTs should ensure that an appropriate ventilator is being used to administer heliox, determine if a conversion factor is needed to adjust settings, and ensure that the patient is monitored closely while such an approach is being used.69
Troubleshooting and Hazards
A more serious problem is hypoxemia associated with breathing helium mixtures.67,70 Although this problem may have been caused by using too low an O2 concentration (20%), there is another possibility. Very rarely, some commercial helium-O2 cylinders stored for long periods of time have been found to contain these gases in an unmixed, or separated, state. The only way to avoid this potential hazard is to analyze the O2 concentration coming from the cylinder before administering the gas.
As clinical applications for heliox have expanded, other hazards have emerged. One potential problem is volume-induced lung injury when heliox is administered via a mechanical ventilator. This risk can be addressed by using only ventilators approved by the FDA for heliox administration. The use of heliox for bronchodilator nebulization may present another possible hazard. The lower density of helium-O2 mixtures may result in greater variability in medication delivery to the airways. Careful patient monitoring during such therapy can help minimize this concern. Another rare but possible problem is hypothermia to infants receiving heliox via an oxyhood. This risk results from the high thermal conductivity of helium and can be avoided by warming and humidifying the heliox gas.67
Carbon Dioxide–Oxygen (Carbogen) Therapy
Although rarely used, CO2-O2 mixtures (carbogen) have been employed to treat hiccups and carbon monoxide poisoning and to prevent complete washout of CO2 during cardiopulmonary bypass. More recently, it has been investigated as a treatment for hearing loss and seizures. However, the application of carbogen in clinical settings has been quite limited given the potential adverse effects of hypoxemia, premature ventricular contractions, hypertension, and muscle twitching.71
Carbogen is supplied in compressed gas cylinders as either 5%:95% or 7%:93% CO2-O2 mixtures. It can be administered to patients with a snug-fitting nonrebreathing mask, with a flow sufficient to prevent the reservoir from collapsing during inhalation. Because of the potential adverse effects, patients receiving carbogen should be monitored closely, especially at 7%:93% mixtures, with special attention paid to pulse, respiratory rate, pulse oximetry, blood pressure, and mental status. If any significant adverse effects are noted, the therapy should be stopped, and the patient should be monitored closely.71