Chapter 19 Injury to the Developing Preterm Brain
Intraventricular Hemorrhage and White Matter Injury
Introduction
As newborn intensive care approaches its sixth decade, preterm birth has emerged as a major pediatric public health problem [Behrman and Stith Butler, 2007]. There were almost 129 million births worldwide in 2005, and 9.6 percent of them were premature [Beck et al., 2010]. Survival is increasing for neonates of all gestational ages, and the prevalence of children with cognitive impairments at school age continues to rise [Allen, 2008; Behrman and Stith Butler, 2007; Hack et al., 2009; Robertson et al., 2009]. At age 8 years, more than 50 percent of infants with birth weights < 1000 g require special classroom assistance in the classroom, 20 percent are in special education, and 15 percent have repeated at least one school grade [Allen, 2008; Aylward, 2005; Bhutta et al., 2002; Larroque et al., 2008; Neubauer et al., 2008; Saigal and Doyle, 2008; Voss et al., 2007]. In addition, 10–20 percent have cerebral palsy [Larroque et al., 2008].
Brain injury is common in preterm neonates and may perturb the genetically prescribed program of cerebral development in the prematurely born [Volpe, 2009a]. Magnetic resonance imaging (MRI) has permitted noninvasive high-resolution evaluation of the developing brain, and numerous investigators have reported that very low birth weight (VLBW) preterm infants experience both macrostructural alterations in postnatal brain development, as well as in microstructural connectivity when compared to term neonates [De Vries et al., 1999; Hagmann et al., 2009; Huppi et al., 1996; Maalouf et al., 1999; Mercuri et al., 1996; Woodward et al., 2006]. To date, however, both the pathophysiology of these changes and the relationship of MRI studies to neurodevelopmental outcome data remain poorly understood [Allin et al., 2004; Boardman et al., 2007; Huppi et al., 1998; Inder et al., 1999, 2005; Mewes et al., 2006; Peterson et al., 2000]. These data suggest that strategies for identifying and preventing causes of disability in this patient population are critically important to both physicians and parents.
The neuropathologic processes that most commonly affect the prematurely born neonate include intraventricular hemorrhage (IVH) and white matter injury, or the periventricular leukomalacia complex [Volpe, 2009a]. IVH, or hemorrhage into the germinal matrix tissues of the developing brain, is detected in approximately 10–25 percent of VLBW neonates [Fanaroff et al., 2007; O’Shea et al., 2008], while white matter injury (WMI) and the global neuronal and axonal deficits that may accompany it are found in 50 percent or more of this vulnerable population [Dyet et al., 2006; Maalouf et al., 1998; Woodward et al., 2006]. Because both IVH and WMI are unique to the preterm population and represent a significant burden of disease, the pathophysiology, outcome, and prevention of these two injuries will be reviewed separately.
Intraventricular Hemorrhage
Although IVH occurs in 10–25 percent of all VLBW neonates, it is most significantly a problem for those infants at the lowest limits of viability [Fanaroff et al., 2007]. Both the incidence and the severity of IVH are inversely related to birth weight and gestational age, and almost 25 percent of neonates of 501–750 g birth weight experience the highest grades of hemorrhage [Fanaroff et al., 2003, 2007]. High-grade IVH is more common in male infants than females [Hintz et al., 2006; Synnes et al., 2001; Tioseco et al., 2006], and although the risk period for IVH is in the first 4–5 postnatal days, data from several centers indicate that approximately half of all hemorrhages occur within the first 6–8 postnatal hours [Dolfin et al., 1983; Ment et al., 1993; Perlman and Volpe, 1986; Perlman and Rollins, 2000; Shaver et al., 1992] – a time of intense medical instability for those neonates with the lowest gestational ages.
Many neonatal intensive care units perform routine head ultrasound screening of preterm neonates using the system developed by Papile for grading IVH, as shown in Table 19-1 [O’Shea et al., 2008; Papile et al., 1978]. Employing this nomenclature, grade 1, or germinal matrix hemorrhage (GMH), describes blood in the germinal matrix only. Grade 2 is blood filling the lateral ventricles without distension, grade 3 is blood filling and acutely distending the ventricular system, and grade 4 describes hemorrhages with parenchymal involvement of hemorrhage. When associated with grades 1–3 IVH, unilateral grade 4 hemorrhages are considered in most cases to represent venous infarction of the periventricular white matter [de Vries et al., 2001], and the nomenclature of Volpe [Volpe, 2001], also shown in Table 19-1, provides a more pathophysiologic approach to parenchymal events.
In the newborn period, 5–10 percent of preterm infants with IVH suffer seizures and as many as 50 percent of infants with grade 4 IVH experience posthemorrhagic hydrocephalus [Volpe, 2009a]; mortality is also higher in those infants with IVH. Finally, although the long-term neurodevelopmental outcome for infants with lower grades of hemorrhage remains uncertain at this time, most observers agree that infants with parenchymal hemorrhage are at high risk for neurodevelopmental handicap (Figure 19-1) [Luu et al., 2009a; Saigal et al., 2003; Sherlock et al., 2005, 2008].
Pathophysiology
Intraventricular Hemorrhage is a Complex Disorder
IVH has been attributed to alterations in cerebral blood flow to the immature germinal matrix microvascular network [Shalak and Perlman, 2002; Whitelaw, 2001], as shown in Figure 19-2, and studies addressing the etiology of IVH have identified numerous environmental and medical risk factors (Box 19-1). These include low gestational age, absence of antenatal steroid exposure, maternal antenatal hemorrhage, chorioamnionitis/infection/inflammation and/or fertility treatment, neonatal transport to a tertiary care center following birth, maternal sepsis, seizures, hypotension requiring medical therapy, hypoxemia, hypercapnia, and acidosis [du Plessis, 2008; Fabres et al., 2007; Limperopoulos et al., 2008; McCrea and Ment, 2008; O’Leary et al., 2009]. As this lesion is unique to preterm infants, examination of those developmental, environmental, and genetic events that result in IVH is critical. These include anatomic, physiologic, and genetic factors.
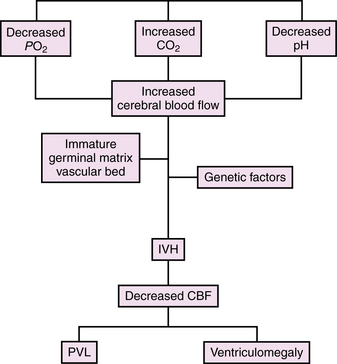
Fig. 19-2 Model for the development of intraventricular hemorrhage in the preterm infant.
CBF, cerebral blood flow; IVH, intraventricular hemorrhage; PVL, periventricular leukomalacia.
Anatomic Factors are Permissive for Hemorrhage
During the late second and third trimesters, the developing brain almost triples in volume, and there are multiple developmental events that occur during this time interval. The germinal matrix and the adjacent subventricular zone (SVZ) are the sites of proliferation of both glial and neuronal precursors. The SVZ generates predominately GABAergic cortical interneurons, although recent data suggest that the germinal matrix also produces GABAergic neurons that migrate to the dorsal thalamus during the risk period for IVH. (For review, please see Volpe [2009a].)
By 24 weeks of gestation, almost but not all of the cortical neurons have migrated from the germinal zones and axonal ingrowth begins [Kostovic, 1990; Kostovic and Rakic, 1990; Kostovic et al., 2002, 1989]. The elaboration of dendritic arbors is at an active stage and synaptic contacts are beginning to form [Huttenlocher and de Courten, 1987; Huttenlocher and Dabholkar, 1997; Huttenlocher et al., 1982]. The germinal matrix remains relatively robust through 32–34 weeks of gestation but almost completely involutes by term [Donat et al., 1978; Rorke, 1982; Whitelaw, 2001].
During this period of rapid cell genesis, the metabolically active germinal matrix requires a rich blood supply, as shown in Figure 19-3, yet its vessels are neuropathologically immature and present numerous risk factors for hemorrhage (Table 19-2). The developing blood–brain barrier is characterized by basement membrane proteins, endothelial tight junctions, capillary pericytes, and astrocytic endfeet [Ballabh et al., 2004]. Immunohistologic and electron microscopic examinations comparing germinal matrix microvessels with those in the periventricular white matter and cortical mantle demonstrate a paucity of fibronectin, fewer pericytes, and decreased perivascular coverage by glial fibrillary acidic protein (GFAP)-positive astrocytic endfeet [Ballabh et al., 2005; Braun et al., 2007; El-Khoury et al., 2006; Wei et al., 2000; Xu et al., 2008]. Since GFAP provides both shape and mechanical strength to the endfeet of astrocytes, its decrease, in combination with alterations in basement membrane proteins and pericyte investiture, suggests a structural weakening of the germinal matrix blood–brain barrier. In contrast, studies of fetuses and preterm neonates during the second and third trimesters of gestation have demonstrated no difference in the primary endothelial tight junction molecules, claudin-5, occludin, and JAM-1, in the germinal matrix compared to cortical gray and white matter regions [Ballabh et al., 2005; Xu et al., 2008].
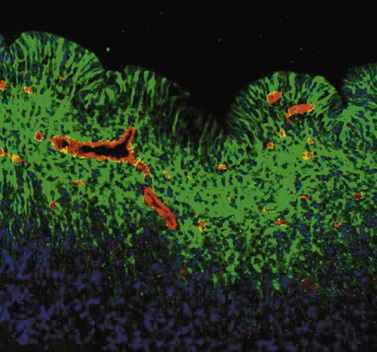
Fig. 19-3 Morphology of the germinal matrix.
(Courtesy of Praveen Ballabh, M.D., Department of Pediatrics, Anatomy, and Cell Biology, New York Medical College-Westchester Medical Center, Valhalla, NY.)
Table 19-2 Germinal Matrix Risk Factors for Intraventricular Hemorrhage
Factor | Developmental Regulation in GM | Changes in GM |
---|---|---|
Vascular density and area | Increase with GA | GM > cortex, WM |
Blood–brain barrier | ||
Tight junction proteins Claudin-5 Occludin Junction adhesion molecule 1 |
No change No change No change |
No differential expression No differential expression No differential expression |
Basement membrane proteins Laminin Collagen IV Fibronectin |
No change Increase with GA No change |
GM > cortex, WM No change GM < cortex, WM |
Glial endfeet Glial fibrillary acidic protein |
Increase with GA | GM < cortex, WM |
Pericytes Vascular coverage |
Increase with GA | GM < cortex, WM |
Angiogenic factors VEGF Angiopoietin-2 |
N/a N/a |
GM > cortex, WM GM > cortex, WM |
GA, gestational age; GM, germinal matrix; N/a, not available; VEGF, vascular endothelial growth factor; WM, white matter.
The germinal matrix has a greater vascular density than other developing brain regions, and recent reports suggest rapid angiogenesis in the germinal matrix, perhaps to support its greater metabolic rate and oxygen requirement [Ballabh et al., 2004]. This angiogenesis is induced by the high levels of vascular endothelial growth factor (VEGF) and angiopoietin (ANGPT)-2 found selectively in the germinal matrix compared to both the cortex and periventricular white matter [Carmeliet, 2003; Ferrara et al., 2003]. Since preclinical studies demonstrate that an increase in the expression of ANGPT-2 in the presence of VEGF promotes the sprouting of immature vessels lacking both basement membrane proteins and pericyte investiture [Carmeliet, 2003; Yancopoulos et al., 2000], the increased presence of these factors in the highly vascular germinal matrix provides further evidence for microvessels lacking a competent blood–brain barrier [Ballabh et al., 2007].
Finally, the venous circulation of the preterm brain may also contribute to the susceptibility to intraparenchymal hemorrhage. Venous blood from the periventricular white matter flows through a fan-shaped array of both short and long medullary veins into the veins of the germinal matrix, and subsequently into the terminal veins found inferior to this highly proliferative zone. The somewhat tortuous anatomy of these veins permits venous stasis, with subsequent hemorrhagic venous infarction of the periventricular white matter [Gould et al., 1987; Takashima et al., 1986; Taylor, 1995].
Alterations in Cerebral Blood Flow Contribute to IVH
Both experimental animal models (i.e., “preclinical data”) and clinical data suggest that alterations in cerebral blood flow may play an important role in the pathophysiology of IVH. (For review see du Plessis [2008].) Cerebral blood flow was reported to be pressure-passive in the asphyxiated preterm infant over four decades ago [Lou et al., 1979], yet the factors that contribute to cerebral autoregulatory systems in the prematurely born are just beginning to be explained. Further, the definition of the cerebral pressure-flow autoregulatory plateau, or that range of cerebral perfusion pressures (mean arterial pressure − cerebral venous pressure) over which cerebral blood flow is normally maintained, is poorly defined for the critically ill, extremely low birth weight preterm neonate. None the less, experimental animal data suggest that at perfusion pressures above this reportedly relatively narrow plateau, cerebral blood flow becomes pressure-passive.
Changes in perfusion pressure, alterations in circulating oxygen and carbon dioxide levels, and the magnitude of these respiratory fluctuations are perhaps the most important regulators of cerebral blood flow in the preterm neonate. In response to these physiologic alterations, cerebral blood flow rises, hemorrhage begins within the germinal matrix, and blood may rupture into the ventricular system. After ventricular distension by an acute hemorrhage event, cerebral blood flow falls. Venous stasis within the periventricular white matter ensues, and parenchymal venous infarction may soon develop [McCrea and Ment, 2008; Volpe, 2001].
Prostaglandins, and particularly the cyclo-oxygenase 2 (COX-2) system, are important modulators of cerebral blood flow in the developing brain [Leffler et al., 1985, 1986]. Hypoxia, hypotension, growth factors, including transforming growth factor-β and epidermal growth factor, and inflammatory modulators, such as interleukin (IL)-6, IL-1β, tumor necrosis factor α (TNFα), and nuclear factor κβ, may all induce COX-2 [Baier, 2006; Dammann and Leviton, 2004; Heep et al., 2003, 2005; Ribeiro et al., 2004]. The resultant increase in prostanoids results in the release of VEGF, the potent angiogenic factor associated in preclinical and clinical studies with IVH.
Following hemorrhage, these same triggers set into motion a cascade of events leading to disruption of tight junction proteins, increased blood–brain barrier permeability, and microglial activation within the developing white matter. The damaged endothelium releases IL-1β and TNFα, and these cytokines promote transmigration of leukocytes across the emerging blood–brain barrier. The activated microglia release reactive oxygen species (ROS), which in turn promote endothelial damage, alter hemostasis, and increase metabolism. (For review, please see du Plessis [2008] and Chua et al. [2009].) The preterm brain is very sensitive to ROS because of the immaturity of those enzyme systems designed to detoxify them, and experimental animal studies suggest that ROS play a significant role in the generation of periventricular parenchymal infarction [Zia et al., 2009].
Clinical events believed to be associated with increases in cerebral blood flow in the preterm infant and the presumed postnatal onset of IVH include endogenous and exogenous events. Vigorous resuscitation, rapid volume re-expansion, endotracheal tube repositioning, recurrent suctioning, complex nursing care procedures, and the administration of sodium bicarbonate, as well as respiratory distress syndrome, hypoxemia, extremes of PCO2 levels (i.e., both hypo- and hypercapnia), and acidosis have all been associated with IVH [Ancel et al., 2005; Hall et al., 2005; Kaiser et al., 2006; Kluckow, 2005; Kuint et al., 2009; Linder et al., 2003; O’Leary et al., 2009; Osborn and Evans, 2004; Synnes et al., 2001; Whitelaw, 2001] (Box 19-2). Routine screening by bedside cranial ultrasonography has demonstrated IVH after seizures and pneumothoraces in infants who were previously known to have no evidence of hemorrhage [Cooke, 1981; Hill and Volpe, 1981; Hill et al., 1982]. Finally, cerebrovasoactive cytokines are released in association with sepsis and may contribute to IVH.
Cerebral blood flow is markedly diminished after hemorrhage [Del Toro et al., 1991; Volpe, 1997]. Both xenon-133 inhalation and positron emission tomography (PET) have demonstrated significant hypoperfusion for longer than the first postnatal week in preterm infants with IVH [Ment et al., 1984; Volpe et al., 1983]. The cerebral hypoxemia and ischemia that accompany this depression in flow may result in damage not only to the periventricular white matter but also to those neural and glial precursors still residing in the germinal matrix and SVZ. Oligodendroglia and their precursors are among the cells in the developing cortex most sensitive to hypoxia, and their loss is postulated to result in secondary, gestational age-dependent alterations in connectivity in the developing brain [Gimenez et al., 2006; Miller et al., 2002; Nosarti et al., 2009]. Consistent with this hypothesis are reports of significantly decreased cortical gray matter volumes at term equivalent in VLBW preterm infants with grades 1–3 IVH compared to those with no IVH [Vasileiadis et al., 2004], and of decreases in cortical gray and white matter volumes, as well as callosal areas, in preterm subjects at 8–15 years of age [Kesler et al., 2004; Nosarti et al., 2008].
Candidate Genes for IVH
Although the environmental risk factors for IVH have been well studied, not all infants of a given birth weight range or those who suffer a known hypoxemic event experience IVH. Further, the incidence of high-grade IVH has not changed during the past 10 years despite improvements in care (Table 19-3) [Fanaroff et al., 2003, 2007]. Finally, twin analyses show that intraventricular hemorrhage is familial in origin, and over 40 percent of the variance in liability for IVH can be accounted for by shared genetic and environmental factors [Bhandari et al., 2006]. For these reasons, several investigators have tested the hypothesis that the etiology of IVH is multifactorial, involving both environmental and genetic events.
Table 19-3 Selected Perinatal Information for Infants Born with Birth Weights 500–1000 g in the National Institute of Child Health and Human Development (NICHD) Neonatal Research Network in Three Epochs
The description of the thrombophilias associated with the factor V Leiden and prothrombin G2021A mutations, and the implication of both abnormalities in fetal and neonatal stroke, suggested to several investigators that these might also be candidate genes for IVH [Debus et al., 1998; Gopel et al., 2001, 2002; Petaja et al., 2001]. Examination of other target genes in the presumptive pathway to IVH shortly followed. As shown in Table 19-4, these include genes contributing to hemostasis, vascular stability, and inflammation [Baier, 2006].
Gene | Allele | Effect |
---|---|---|
Thrombophilia and Coagulation Genes | ||
Factor V Leiden | Gln506-FV | Decreased inactivation of factor V Increased thrombin generation Candidate gene for perinatal stroke and IVH |
Prothrombin | G20210A | Increased thrombin Candidate gene for perinatal stroke and IVH |
Factor XIII | 34 Leu allele | High fibrinolytic activity in germinal matrix Associated with hemorrhagic events in adults Candidate gene for IVH |
MTHFRC mutations | Result in increased plasma homocysteine Increased thrombosis Candidate gene for IVH |
|
Vascular Stability Genes | ||
COL4A1 | Decreased vascular stability Candidate gene for fetal stroke and IVH |
|
Proinflammatory Cytokines | ||
TNF-α | Biallelic G to A at -308 | A allele confers high gene transcription A allele is candidate gene for IVH |
IL-1β | -511 CT | Increased production T allele is a candidate gene for IVH |
IL-6 | 174 G or C | CC genotype increases production CC genotype is candidate gene for IVH |
Thrombophilia and coagulation genes
Numerous investigators have examined the role of factor V Leiden (FVL) mutation, polymorphisms in those genes responsible for prothrombin, factor XIII, MTHFRC, and total homocysteine in the genesis of IVH [Debus et al., 1998; Gopel et al., 2001, 2002; Komlosi et al., 2005; Petaja et al., 2001].
A point mutation in the factor V gene resulting in the arginine 506 to glutamine substitution in factor V (Gln506-FV) is known as the FVL mutation. FVL is common in the Caucasian population and causes decreased inactivation of factor V, leading to markedly increased thrombin generation [Bertina et al., 1994]. Importantly, FVL has been associated with thrombotic conditions in both adult and pediatric populations.
The G20210A polymorphism in the prothrombin gene results in a G to A substitution at nucleotide 20210; this mutation increases thrombin and thus also increases the risk for thrombosis. FVL and prothrombin G20210A have been reported in children and infants with ischemic stroke, porencephaly, and other thromboembolic events. Gopel hypothesized that relative hypercoagulability would protect preterm neonates from the extension of grade 1 IVH to higher grades of hemorrhage [Gopel et al., 2001]. In this study of 305 infants with birth weights <1500 g, the FVL mutation was protective for grades 2–4 IVH. In contrast, Petaja hypothesized that the hypercoagulable state associated with FVL would promote periventricular venous infarction and render the infant susceptible to parenchymal involvement of IVH; this study of 51 infants of <32 weeks gestational age suggested that FVL was associated with grade 4 IVH (odds ratio 5.9) [Petaja et al., 2001]. Aronis evaluated 55 preterm infants of <32 weeks gestational age for FVL and prothrombin mutations, and noted a “trend” for thrombophilias among infants with the latter mutation [Aronis et al., 2002].
Factor XIII levels are low in the preterm neonate, and this finding may result in high fibrinolytic activity in the germinal matrix. The factor XIII 34 Leu allele has been reported to cause hemorrhage events in adults and increased IVH in preterm neonates [Gopel et al., 2002].
Finally, mutations in the 5,10 methylenetetrahydrofolate reductase gene (MTHFRC) lead to increased plasma homocysteine levels, a well-recognized risk factor for increased thrombosis. None the less, several authors have reported no association with either these mutations or total homocysteine levels at birth in preterm neonates with IVH [Aronis et al., 2002; Gopel et al., 2001].
Mutations in vascular stability genes
Numerous vascular, astrocytic, and pericytic proteins contribute to the maturation of the developing blood–brain barrier, and allelic alterations in these genes and their impact on IVH are just beginning to be described. Recent data suggest that mutations in collagen IVA1 result in IVH in neonatal mice, and fetal stroke and porencephalies in human infants [de Vries et al., 2009; Gould et al., 2005, 2006]. More recently, a mutation in this important vascular structural protein has been reported in preterm twins with grade 4 IVH [Bilguvar et al., 2009].
Mutations in proinflammatory cytokines
Proinflammatory cytokines are believed to represent important mediators of perinatal brain injury, and TNFα is known to be a central mediator in the inflammatory cascade. The degree of TNFα production may in part be genetically driven. A biallelic G to A polymorphism at position −308 in the TNFα promoter region confers either high (A) or low (G) gene transcription [Kroeger et al., 1997]. Hypoxia and ischemia, both of which are known to contribute to the pathogenesis of IVH, are associated with the upregulation of TNFα. Baier examined the role of this mutation in ventilated VLBW preterm neonates and found that the TNFα-308A allele was associated with an increased risk of IVH (40 percent for infants with an A allele compared to 24 percent with the GG genotype) [Adcock et al., 2003].
Polymorphisms in interleukins may also be possible genetic modifiers for IVH, although the results require further validation. These include mutations in IL-1β, IL-6, and IL-4. The IL-1β-511 CT mutation results in increased production of this proinflammatory cytokine and has been reported to increase the risk of IVH in ventilated preterm neonates with the T allele (33 percent vs. 14 percent for neonates with the C allele) [Baier, 2006]. Similarly, IL-6 mutations are believed to be strong candidate genes for IVH. Position 174 can be either a G or a C, and IL-6 production is greater in neonates with the CC genotype, but studies investigating IVH rates and/or and neurodevelopmental outcome in this subject population are contradictory [Gopel et al., 2006; Harding et al., 2004]. Similarly, a polymorphism at position 472 of the IL-6 gene has been shown in a small study to be associated with alterations in neurodevelopmental outcome in preterm infants at 2 years of age, although the study infants did not appear to have an increased incidence of IVH [Harding et al., 2005]. Finally, in a single study, mutations in IL-4 have been associated with a decreased incidence of IVH in African-American neonates [Baier, 2006].
Neuropathology
Over 90 percent of all IVH in preterm infants originates in the germinal matrix tissues located between the caudate nucleus and the thalamus at the level of, or slightly posterior to, the foramen of Monro in the developing brain [Donat et al., 1978; Volpe, 1997]. A small percentage of hemorrhages in preterm neonates may also originate from the choroid plexus, which is the most common site of IVH in term infants [Rorke, 1982].
Although it is often difficult to differentiate the neuropathologic findings that are directly attributable to IVH from those that frequently accompany it, most authors would agree that germinal matrix destruction, periventricular hemorrhagic infarction, porencephaly, and posthemorrhagic hydrocephalus (PHH) are the direct neuropathologic consequences of IVH [Shalak and Perlman, 2002; Whitelaw, 2001]. In contrast, periventricular leukomalacia and ventriculomegaly, to be described later in this chapter, are frequently detected in infants with IVH but are multifactorial in etiology.
Germinal matrix hemorrhage (GMH), solely confined to the germinal layer, occurs equally in both hemispheres and is found to be bilateral in approximately half of cases [Leech and Kohnen, 1974]. Not infrequently, there are multiple hemorrhages over one caudate nucleus, and injection techniques have demonstrated that all of the major vessels supplying the germinal matrix, including the artery of Heubner, the anterior choroidal artery, the lateral striate arteries, and the terminal vein, remain intact after an initial GMH [Pape and Wigglesworth, 1979]. Such a hemorrhage is associated with destruction of the capillary bed, and secondary germinal matrix cyst formation is common [Hambleton and Wigglesworth, 1976].
After rupture of a GMH, the spread of blood throughout the ventricular system is variable. Sonograms may demonstrate clots attached not only to the site of rupture but also to the choroid plexus, and variable spread of blood throughout the entire ventricular system. A characteristic pathologic finding is a mass of clot within the subarachnoid spaces at the base of the brain (Figure 19-4), and extension of blood through the subarachnoid pathways into the sylvian fissure and over the convexities of the developing hemispheres.
Serial cranial sonograms suggest that as many as 10–20 percent of preterm infants with GMH develop intraparenchymal abnormalities [Fanaroff et al., 2003]. The neuropathologic correlates of these abnormalities are most easily understood as arising not only from the acute increase in intracranial pressure attributable to the GMH itself, but also from the profound decreases in regional blood flow that may follow it. In 10–20 percent of infants with extensive GMH, the branches of the terminal vein that typically drain through the area of hemorrhage become grossly congested, and venous hemorrhagic infarction of the periventricular white matter and even cortical tissues may occur during the first several days after IVH [Pape and Wigglesworth, 1979]. These periventricular white matter infarctions are frequently termed intraparenchymal echodensities (IPE) when examined by cranial ultrasonography or MRI. In addition, some investigators believe that the parenchymal involvement of IVH readily visible by cranial ultrasonography represents a direct pressure-mediated extension of hemorrhage from either a massive GMH or blood within the ventricular system, but the close clinical association of ischemia and hemorrhage and the timing of the development of intraparenchymal echodense lesions on ultrasonography suggest that these lesions represent venous infarction of the periventricular white matter. These lesions are difficult to distinguish at the time of postmortem examination and have been variously named hemorrhagic intracerebral involvement, parenchymal involvement of hemorrhage, or grade 4 IVH. As proposed by Volpe, while GMH may result in the destruction of the germinal matrix and loss of neuronal and glial cells residing there, extension of hemorrhage into the SVZ and periventricular white matter is associated with not only the loss of additional precursor cells but also axonal and glial necrosis [Volpe, 2009a].
PHH represents a not uncommon sequela of IVH [Whitelaw, 2001; Whitelaw et al., 2004]. The meninges are thickened and show an infiltration by hemosiderin-laden macrophages [Deonna et al., 1975; Larroche, 1972]. These findings result in occlusion of the arachnoid villi, obstruction to flow of cerebrospinal fluid (CSF) through the foramina of Luschka and Magendie, and impairment of flow through the tentorial notch (see Figure 19-4). Occasionally, aqueductal obstruction is caused by an acute blood clot, ependymal disruption, or reactive gliosis [Cherian et al., 2004; Whitelaw, 2001].
Neuroimaging
Intraventricular Hemorrhage
Routine cranial ultrasonography via the anterior fontanel is performed in neonatal intensive and special care units around the world, and this modality represents the method of choice for the diagnosis and monitoring of IVH and its complications in preterm infants. Most nurseries use the standard grading system found in Table 19-1, which was originally developed for computed tomography (CT) scanning but fairly quickly applied to ultrasonography (Figure 19-5) [Papile et al., 1978]. Although grade 4 hemorrhages (shown in Figure 19-6) have been noted within the first 6 postnatal hours, most infants initially experience low-grade hemorrhage, which can be seen to progress over the course of time by ultrasonography. The most common sites for parenchymal hemorrhage are the frontal and parietal regions; approximately 50 percent of such hemorrhages occur bilaterally [Shalak and Perlman, 2002].
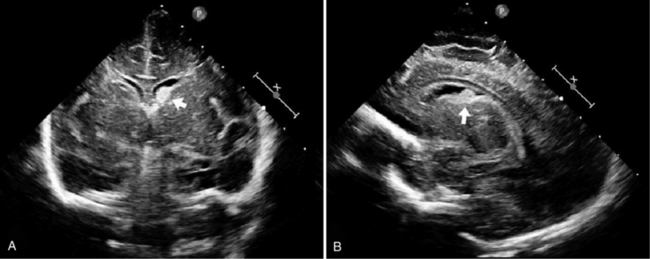
Fig. 19-5 Germinal matrix hemorrhage (GMH).
(Courtesy of Walter C Allan, M.D., Maine Medical Center, Portland, ME.)
Intraparenchymal Echodensities
Cranial ultrasonography and MRI are also used for the diagnoses of IPE and porencephaly. IPE has been described as an asymmetric white matter lesion that may accompany the onset of IVH or follow it. Rarely found without GMH or IVH, IPE is believed to represent hemorrhagic venous infarction of the periventricular white matter and generally occurs in infants of the youngest gestational ages during the first postnatal week [de Vries et al., 2001]. The ultrasound appearance, found in Figure 19-7, is an echogenic juxtaventricular white matter lesion superior and lateral to the lateral ventricle and extending toward the cortex in a flare pattern without evidence for mass effect on the surrounding brain. Most often, IPE eventually cavitates or atrophies to form either a porencephaly or adjacent ex vacuo ventriculomegaly [De Vries et al., 2004].
Improvements in MRI technology and the use of special MRI-compatible incubators and ventilators have permitted MR imaging of hemorrhage in the developing brain. In the newborn period, MR studies demonstrate a single hemorrhagic lesion adjacent to or communicating with the lateral ventricle in addition to IVH [Dyet et al., 2006] (Figure 19-8).
Porencephaly
Porencephalies (Greek for “holes in the brain”) are hemispheric cavitary lesions. In neonates, porencephalies follow grade 4 hemorrhages, IPEs, or periventricular venous infarctions. Although most porencephalies are freely communicating with the ventricular system, a porencephaly may present as a fluid-filled cyst that causes increased intracranial pressure in the preterm infant. A typical porencephaly is shown by ultrasound and MRI in Figure 19-9.
Clinical Findings
Incidence
While the incidence of IVH depends on a number of factors previously discussed, most centers report that 20–25 percent of VLBW neonates experience IVH, and 6 to more than 20 percent experience grades 3–4 IVH [Fanaroff et al., 2007; Zeitlin et al., 2009]. The incidence of IVH is inversely related to birth weight and gestational age [Sarkar et al., 2009], and data for 18,153 neonates of 501–1500 g birth weight from the National Institute of Child Health and Human Development (NICHD) Neonatal Network between 1 January 1997 and 12 December 2002 suggest that 24 percent of infants of 501–750 g birth weight, 14 percent of infants of 751–1000 g birth weight, 9 percent of those with 1001–1250 g birth weight, and 5 percent of infants with birth weights between 1251 and 1500 g experienced grades 3–4 IVH [Fanaroff et al., 2003, 2007]. Grades 3–4 IVH are more common in male neonates [Leijser et al., 2009]; when Synnes evaluated 3772 neonates of <33 weeks gestational age, 317 (8.3 percent) were found to have grade 3–4 IVH. In this cohort, male gender was an independent and important predictor of IVH (OR = 1.5, 95 percent CI = 1.1–1.9) [Synnes et al., 2006].
Timing of IVH
The risk period for IVH for preterm infants is the first 4–5 postnatal days [Ment et al., 2002; Perlman and Rollins, 2000]. Over 50 percent of all hemorrhages are detectable within the first 6 postnatal hours, and the work of Shaver suggests that almost all of these early hemorrhages are in fact detectable within the first postnatal hour [Shaver et al., 1992]. Less than 5 percent of hemorrhages occur after the fourth or fifth postnatal day (see Figure 19-9). The risk period for IVH is independent of gestational age, and between 10 and 65 percent of infants experience progression, or extension, of their hemorrhages over the first several postnatal days [Whitelaw, 2001]. Extension of IVH and the development of high-grade IVH are twice as common in those infants with the earliest onset of IVH. This progression of hemorrhage has been linked to known clinical events, such as pneumothoraces and seizures, which have been demonstrated to increase cerebral blood flow [du Plessis, 2008].
Clinical Manifestations
The clinical manifestations of IVH are variable, and in one large series of postmortem examinations, over 75 percent of hemorrhages were clinically unrecognized [Allan and Sobel, 2004; Perlman and Rollins, 2000; Volpe, 1997]. None the less, as shown in Table 19-5, infants with large hemorrhages generally are noted to suffer a profound decrease in peripheral hematocrit and may experience coma, seizures, abnormal eye findings, including dilated and nonreactive pupils and loss of eye movements, and changes in tone and reflexes [Dubowitz et al., 1998]. Apneic and bradycardic spells may be attributable to either increased intracranial pressure or changes in cerebral blood flow to brainstem respiratory control centers. Of note, infants with even low-grade IVH may have significantly elevated serum glucose values and evidence for inappropriate secretion of antidiuretic hormone [Moylan et al., 1978; Shalak and Perlman, 2002]. Finally, persistent, unremitting metabolic acidosis unresponsive to alkali therapy or pressor agents is the hallmark of high-grade hemorrhage.
Table 19-5 Clinical Symptoms of High-Grade Intraventricular Hemorrhage
Symptom | Incidence |
---|---|
Decrease in hematocrit | 75% |
Seizures | 10–15% |
Changes in tone | 75–90% |
Abnormal eye signs | 33–95% |
Apnea/bradycardia | 50–75% |
Hyperglycemia | >50% |
Hyponatremia | >50% |
Metabolic acidosis | >75% |
Ment unpublished data, NS27116 Randomized Indomethacin IVH Prevention Trial, 1997.
Neonatal Outcome
In the neonatal period, infants with IVH are at risk for the development of seizures and PHH [Allan and Sobel, 2004; Whitelaw et al., 2004]. Depending on the birth weights and gestational ages of the cohorts studied and the investigator’s method for determining seizures, the incidence of seizures in preterm infants with IVH has been reported to range from 5 to 45 percent. Of note, a recent continuous amplitude-integrated EEG (aEEG) study of preterm neonates suggests that the mean age of seizure detection is 28 hours in infants with IVH, and only 2 of 8 neonates with IVH and seizures detected by aEEG had clinically recognizable events [Shah et al., 2010].
Posthemorrhagic hydrocephalus
Posthemorrhagic hydrocephalus, shown by ultrasound in Figure 19-10, is diagnosed in the infant who meets all of the following criteria: presence of intraventricular blood, increasing ventriculomegaly (most commonly diagnosed by cranial ultrasonography), and evidence of increased intracranial pressure (defined as an opening pressure of greater than 140 mm H2O by either lumbar puncture or, if indicated, cerebral ventricular tap) [McCrea and Ment, 2008; Whitelaw, 2001; Whitelaw et al., 2004]. In addition, in infants with PHH, the ventricular width at the intraventricular foramen by sonographic measurement exceeds 4 mm over the 97th percentile for gestational age [Levene, 1981]. PHH is most common in those infants with high grades of hemorrhage and less frequent in infants with the lowest gestational ages [Kazan et al., 2005]. Because of the compliance of the neonatal brain, the signs and symptoms of hydrocephalus may not be evident for several weeks following hemorrhage, and routine ultrasonographic monitoring is recommended in those infants at risk for PHH.
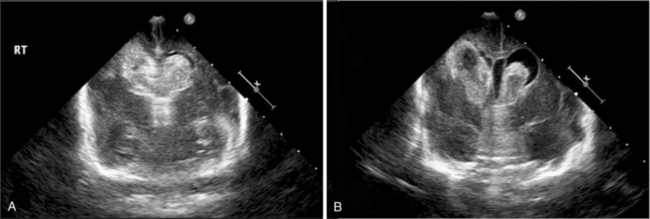
Fig. 19-10 Intraventricular hemorrhage leading to posthemorrhagic hydrocephalus.
(Courtesy of Walter C Allan, MD, Maine Medical Center, Portland, ME.)
In most instances, the sequence of events resulting in PHH begins with multiple small clots throughout the ventricles. Although PHH is traditionally thought to represent a communicating hydrocephalus with obstruction to the passage of CSF at the level of the arachnoid villi, it is also probable that the CSF is reabsorbed across the ependyma into small penetrating vessels within the developing white matter. Obstruction to flow may thus also occur at the level of these vessels, or less commonly, at the foramina of Luschka and Magendie in the posterior fossa [Cherian et al., 2004]. In the presence of an obstruction in the normal CSF flow pathways, ventriculomegaly develops and periventricular white matter damage may ensue. Suggested mechanisms for periventricular white matter injury following PHH include raised pressure and edema, damaging effects of the free iron released into the CSF during clot lysis, and/or proinflammatory cytokines [Savman et al., 2001]. In addition, a small percentage of infants with IVH may develop acute obstruction at either the foramen of Monro, as shown in Figure 19-11, or the aqueduct secondary to the presence of clots obstructing CSF flow; these infants present with signs of acutely increased intracranial pressure (i.e., apnea, bulging fontanel, split sutures, and lethargy or coma). These infants require immediate neurosurgical attention, as shown in Video 19-1.
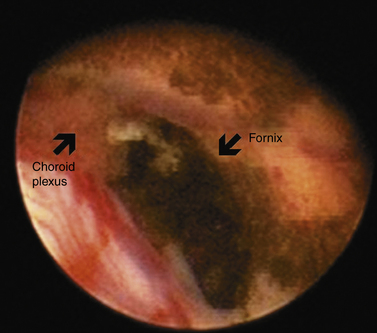
Fig. 19-11 Endoscopic view of the foramen of Monro.
(Courtesy of Charles C Duncan, MD, Department of Neurosurgery, Yale University School of Medicine, New Haven, CT.)
Randomized controlled trials have evaluated several treatment strategies either to prevent or to reduce the extent of PHH. These include intraventricular streptokinase, repeated lumbar or ventricular punctures, and DRIFT (drainage, irrigation and fibrinolytic therapy), but none of these has yet proved effective [Shooman et al., 2009; Whitelaw, 2000a, 2000b; Whitelaw and Odd, 2007; Whitelaw et al., 2001, 2007].
When treating infants with PHH, the physician must both protect the neonate from damage secondary to increased intracranial pressure and avoid the need for permanent CSF drainage procedures. (For review see Shooman et al. [2009].) Infants with intraventricular and/or intraparenchymal hemorrhage should undergo frequent determinations of occipitofrontal circumference and ultrasonographic determinations of ventricular size. Because prolonged increased intracranial pressure may result in poor feeding, lethargy, apnea, and, ultimately, optic atrophy, if ventricular size increases, neurosurgical consultation is recommended. Further, although some investigators have recommended ventricular puncture with the acute removal of 10–20 mL/kg of CSF for symptomatic infants with PHH, others have explored temporizing measures, such as subgaleal shunt placement, ventricular reservoir placement for intermittent tapping, or third ventriculostomy (Video 19-2). These strategies aim to avoid permanent ventriculoperitoneal shunt placement, and a small retrospective review of these interventions in neonates with IVH reported that 91 percent of those infants with subgaleal shunt placement and 62 percent of those with reservoir taps required permanent shunt placement. Of importance, infection rates were similar in the two populations, but future randomized trials are required to explore these treatment options.
Long-Term Outcome
Although recent advances in neonatal intensive care have resulted in increased survival rates for many critically ill and very preterm neonates, the neurodevelopmental fate of VLBW infants remains uncertain. Depending on the birth weight of the patient cohort and the years in which they were born, the incidence of minor developmental impairment is diagnosed in 30–40 percent and major disabilities are found in almost 20 percent of preterm children [Allen, 2008; Larroque et al., 2008; Neubauer et al., 2008; Saigal and Doyle, 2008; Voss et al., 2007]. Over half require special assistance in the classroom, 20 percent are in special education, and 15 percent have repeated at least one grade in school [Aylward, 2005; Bhutta et al., 2002]. Cerebral palsy has long been recognized as a frequent sequela of preterm birth, but significant cognitive and behavioral abnormalities are also being recognized at school age and beyond [Allen, 2008; Hack et al., 2009; Marlow et al., 2005; Robertson et al., 2009; Taylor et al., 2004].
Cerebral Palsy
Cerebral palsy (CP) is an important and well-established outcome of preterm infants that, in some centers, has been monitored for more than 45 years [Saigal and Doyle, 2008]. Recent data suggesting that the prevalence of neurodevelopmental handicap is increasing in the United States have attributed this finding to the increasing survival rates of many preterm and VLBW infants [Allen, 2008; Behrman and Stith Butler, 2007; Larroque et al., 2008].
Several recent studies have assessed the incidence of CP in children solely with IVH. In all, the incidence of CP is reported to increase with the grade of IVH. In pre-surfactant era studies, Pinto Martin reported that grade 4 IVH was strongly associated with CP (odds ratio = 15.4; 95 percent CI = 7.6–31.1), and in the study of Hansen and colleagues, the odds ratio for CP with grades 3 or 4 IVH was 19.9 (CI = 6.1–64.8) [Hansen et al., 2004; Pinto-Martin et al., 1999]. Further, in Pinto-Martin’s study, any grade IVH alone was associated with CP (odds ratio = 3.14; 95 percent CI = 1.5–6.5).
More recently, Sherlock evaluated 270 neonates of <1000 g birth weight or <28 weeks gestational age at 8 years of age and found CP in 100 percent of those children with grade 4 IVH [Sherlock et al., 2005, 2008]. In contrast, approximately one-fifth of neonates with grades 2 or 3 IVH developed CP, and those with grade 1 hemorrhage experienced no increase in incidence of motor handicap when compared to children with no IVH. Similarly, Patra examined 362 children with birth weights <1000 g at 20 months of age; 104 had experienced grade 1–2 IVH [Patra et al., 2006]. Abnormal neurological examinations, including CP, were significantly more common in the IVH group (odds ratio = 2.60, 95 percent CI = 1.06–6.36).
Although most investigators include the results of all scans performed during the infant’s hospitalization, Allan has reported that CP can be predicted from postnatal day 3 onward in preterm infants in whom serial ultrasonography is performed [Allan et al., 1997]. Further, although preterm infants with CP have traditionally been found to suffer spastic diplegia, concomitant with the rising rate of disabling spastic motor handicap, several investigators have also noted that CP among preterm infants is almost equally divided among the three classical forms: spastic diplegia, hemiplegia, and quadriplegia [Allan et al., 1997; Saigal and Doyle, 2008]. It is believed that this change in distribution reflects more focal and diffuse CNS injuries producing proportional increases in hemiplegias and quadriplegia.
Cognitive Outcome in Neonates with Intraventricular Hemorrhage
IVH is a significant cause of disability in the prematurely born. As shown in Figure 19-12, preterm subjects with grade 4 IVH have significantly worse cognitive abilities and educational performance when compared to those without IVH, as well as to those with lesser grades of hemorrhage [Luu et al., 2009a; Neubauer et al., 2008]. In Sherlock’s study of 298 infants with birth weight <1000 g or gestational age <28 weeks, 100 percent of children with grade 4 IVH had full-scale IQ scores more than 1 SD below the population mean, compared to 60 percent of subjects with grade 3 IVH and approximately 40 percent of all other study participants (no IVH, grade 1 IVH, and grade 2 hemorrhage) [Sherlock et al., 2005, 2008]. Similarly, in Patra’s study of 362 preterm neonates of the same birth weight, 45 percent of children with grades 1–2 IVH had mental scores >2 SD below the population mean, compared to 25 percent for those with no evidence of hemorrhage in the newborn period (odds ratio = 2.0, 95 percent CI = 1.20–3.60) [Patra et al., 2006].
Alterations in Brain Development
The advent of MRI has permitted assessment of the long-term influence of a neonatal injury such as IVH on the developing brain [Mewes et al., 2006; Nosarti et al., 2002; Reiss et al., 2004; Woodward et al., 2006]. Preterm children have smaller brain volumes at adolescence and beyond, and lower cortical gray, cortical white, deep gray, and cerebellar volumes when compared to term controls. In addition, IVH has been associated with significant additional reductions in cerebral gray volumes in preterm subjects during childhood and adolescence [Kesler et al., 2008; Nosarti et al., 2008; Reiss et al., 2004; Vasileiadis et al., 2004]. Studying the largest cohort of subjects born preterm at age 14 years and comparing them with age- matched and socioeconomically matched term adolescents, Nosarti also noted widespread alterations in white matter volumes throughout the developing hemispheres [Nosarti et al., 2008]. All areas of differential volumes between the preterm and term subjects were functionally related, and subjects with IVH and ventriculomegaly had the greatest white matter changes.
Prevention of Intraventricular Hemorrhage
The increasing rates of preterm birth and handicap for preterm neonates, and particularly those at the lower limits of viability, enforce the importance of preventing injury in the developing brain. Although the most efficacious means for preventing IVH would be the prevention of preterm birth [Behrman and Stith Butler, 2007], environmental, genetic, and pharmacologic interventions must be considered (Table 19-6).
Etiology | Strategies |
---|---|
Environmental | Maternal transport Bag and mask ventilation, early CPAP Monitoring of blood gases to prevent hypoxemia, hypocarbia, hypercarbia, and/or acidosis Continuous blood pressure monitoring – prevention of arterial hypotension No routine tracheal suctioning or chest physical therapy |
Pharmacologic | Complete course of antenatal steroid exposure Administration of surfactant within the first 15 min Postnatal indomethacin Avoid sodium bicarbonate exposure |
CPAP, continuous positive airways pressure.
Environmental Prevention Strategies
The risk factors for IVH have been well described [du Plessis, 2008; Leijser et al., 2009; Shalak and Perlman, 2002; Soraisham et al., 2009; Synnes et al., 2001, 2006]. When preterm birth is certain, transport of the mother and fetus to a tertiary perinatal center specializing in high-risk obstetric care is mandatory; for over three decades, neonatologists have recognized that those infants who were “outborn” and required transport to the neonatal intensive care unit have consistently higher rates of IVH than “inborn” patients [Hohlagschwandtner et al., 2001].
Abrupt increases in blood pressure, either by volume re-expansion or pharmacologic means, result in the loss of cerebral autoregulation and thus increased cerebral blood flow and are to be avoided. Blood pressure and transcutaneous oxygen pressure should be continuously monitored to prevent hypoxemia, hypotension, and secondary acidosis. Similarly, hypocarbia with PaCO2 levels below 30 mmHg have also been significantly associated with severe IVH. (For review please see du Plessis [2008].) Depending on the gestational age and the postnatal day, preterm infants are known to have a high incidence of patent ductus arteriosus, and previous studies have suggested that the pharmacologic closure of this lesion is less likely to be associated with abrupt changes in cerebral blood flow than the surgical approach might produce [Weiss et al., 1995].
Pharmacologic Prevention
Antenatal corticosteroid exposure
Corticosteroids administered prior to preterm birth have been shown both to lower the incidence of respiratory distress syndrome and to decrease neonatal mortality [Crowley, 2000, 2003]. The incidence of IVH is also reported to be lower in infants with antenatal corticosteroid exposure. In the Cochrane Review of 13 trials including 2827 neonates, corticosteroid therapy consisted of 24 mg of betamethasone, 24 mg of dexamethasone, or 2 g of hydrocortisone. In this large meta-analysis, the fetal exposure to antenatal steroids was associated with a relative risk of any IVH of 0.54 (CI = 0.43–0.69), and the relative risk for severe IVH was 0.28 (95 percent CI = 0.16–0.05). These benefits extend to a broad range of gestational ages and are not limited by gender or race. Further, no adverse consequences of a single course of prophylactic corticosteroid for preterm birth have been reported, although the action and long-term consequences of multiple courses of antenatal steroid exposure have been reported to result in decreases in weight length and head circumference at birth. For these reasons, the National Institutes of Health Consensus Development Conference recommended the widespread use of antenatal steroids for all preterm infants and stated that the significant benefits of this therapy outweigh possible adverse outcomes [Crowley, 1994, 1995].
Indomethacin
Indomethacin, a potent inhibitor of the cyclo-oxygenase pathway of prostaglandin synthesis, decreases both the incidence and severity of IVH in VLBW preterm infants [Ment et al., 1994; Schmidt et al., 2001; Yanowitz et al., 2003]. In Fowlie’s meta-analysis of 19 clinical trials looking at the postnatal use of indomethacin in 2872 infants, postnatal indomethacin treatment reduced the overall risk for IVH by almost 50 percent and was found to be most efficacious for prevention of grades 3–4 IVH [pooled RR = 0.66 (90.53, 0.82)] [Fowlie and Davis, 2002; 2003]. In addition, the ability of indomethacin to decrease both the incidence and severity of IVH was not found to be gestational age-dependent.
A synthetic indole derivative with a half-life of 11–30 hours in preterm infants, indomethacin decreases cerebral blood flow and blood-flow velocity in both animal and human studies [Hammerman et al., 1995; McCormick et al., 1993]. Experimental animal studies have demonstrated a reduction in the normal pattern of cerebral blood flow reactivity to episodes of asphyxia or hypertension, including reducing the normal hyperemia induced by asphyxia [Leffler et al., 1985, 1986; van Bel et al., 1993]. None the less, Fowlie’s meta-analysis demonstrated no evidence of difference in rates of necrotizing enterocolitis, excessive bleeding, or sepsis in treated infants compared to controls [Fowlie and Davis, 2002, 2003]. An increased incidence of oliguria was seen with prophylactic indomethacin [RR = 1.90 (1.45, 2.47)], but there was no evidence for major renal impairment.
Despite the obvious effect in preventing IVH and the low incidence of sequelae, the long-term cognitive benefit of indomethacin therapy has become more controversial. Although Luu found improved cognitive testing scores for preterm males randomized to indomethacin [Luu et al., 2009b], Schmidt reported that indomethacin did not prevent a combined outcome of neonatal death or developmental delay in preterm neonates at 18 months corrected age [Schmidt et al., 2001]. Similarly, Harding noted that prematurely born subjects with a COX-2 C765 allele, associated with reduced COX-2 activity, had decreased cognitive performance at school age and may be vulnerable to the cerebral blood flow effects of indomethacin [Harding et al., 2007].
None the less, current recommendations in many neonatal intensive care units around the world suggest that all infants of 1250 g birth weight or less who are clinically stable and lack contraindications for indomethacin treatment be treated with prophylactic indomethacin 0.1 mg/kg/dose beginning at 6–12 postnatal hours, and with two additional doses 24 and 48 hours after the first administration. Indomethacin might be best administered after the administration of surfactant when there is evidence for acceptable oxygenation and ventilation; indomethacin should be infused slowly, preferably over 30 minutes or more [Bada, 1996; Clyman, 1996].
Activated factor VII
Recombinant activated factor VII (rFVIIa) was originally developed as a hemostatic agent for patients with hemophilia and acts in the clotting cascade, through a variety of tissue factor-dependent and independent mechanisms, to accelerate the formation of thrombin clot in damaged tissues [Labattaglia and Ihle, 2007; Macik et al., 1993]. Because factor VII has been shown to be an effective agent for the prevention of bleeding in a diverse array of situations [Ghorashian and Hunt, 2004], it has also been proposed as a potential treatment for neonates with low-grade IVH, but large-scale safety and efficacy trials have yet to be reported [Greisen and Andreasen, 2003; Mitsiakos et al., 2007; Robertson, 2006].
Erythropoietin
In preclinical studies, in vitro experiments, and adult human trials, erythropoietin (EPO) has been shown to be protective against both hypoxic-ischemic and inflammatory-mediated cerebral injury. Numerous safety trials of EPO suggest no adverse effects in the preterm population, and multicenter trials have been proposed to test the ability of EPO to prevent IVH and adverse neurodevelopmental outcome in the prematurely born [Fauchere et al., 2008; Strunk et al., 2004].
Other pharmacologic prevention strategies
Additional agents evaluated have included antenatal and postnatal phenobarbital, ibuprofen, ethamsylate, vitamin E, and pavulon. After over 15 years of study, the first two agents have been found not to influence the incidence of IVH in preterm infants [Donn et al., 1981; Shankaran et al., 1996].
Ibuprofen has been shown in preclinical studies to prevent cerebral blood flow autoregulation and close the patent ductus arteriosus. However, one study has found that ibuprofen was not effective with respect to IVH prevention in preterm neonates [Aranda and Thomas, 2006].
Ethamsylate is a water-soluble, nonsteroidal drug that has been used in Europe for several decades to reduce capillary bleeding during surgery. There is some evidence that it may increase platelet adhesiveness and capillary resistance by causing polymerization of hyaluronic acid in the capillary basement membrane and may also modify prostaglandin biosynthesis [Vinazzer, 1980]. Several clinical studies have been performed in Europe to evaluate the efficacy of postnatally administered ethamsylate in preventing IVH [Benson et al., 1986; Morgan et al., 1981; EC Ethamsylate Trial Group, 1994]. In these trials, ethamsylate reduced the risk of overall IVH without significant reduction in severe IVH, death, or neurological abnormality. Similarly, vitamin E, a well-recognized antioxidant, has also been shown to decrease the rate of IVH, although the effect on high-grade IVH has not been specifically examined [Chiswick et al., 1991]. Finally, pavulon (pancuronium) also has been evaluated for the prevention of IVH in mechanically ventilated newborns. By inducing muscular paralysis, pavulon is believed to prevent asynchronous breathing and the hypoxemia and secondary increases in cerebral blood flow associated with this phenomenon in the prematurely born [Cools and Offringa, 2005].
Combined Environmental and Pharmacologic Strategies
Repeated reports from numerous investigators have documented the variations in IVH rate and grade from institution to institution across Europe and the United States [Express Group et al., 2009; Fanaroff et al., 2007; Zeitlin et al., 2009]. Variances in patient populations, availability of prenatal care, and perinatal strategies may all contribute to these findings. Most recently, Obladen reported the results of a prospective monitoring trial for neonates of <1000 g birth weight [Obladen et al., 2008]. Monthly care conferences including all obstetric and neonatology personnel were held, and the hypothesis was that a rigidly adhered-to standard of care would lower the incidence of IVH in this vulnerable patient population. Mandatory care strategies included the following:
Employing this strategy, Obladen noted a decrease in IVH rate from 34 to 13 percent.
Cerebellar Hemorrhage
Hemorrhage into the developing cerebellum is a recently described complication of prematurity. Cerebellar hemorrhage (CBH), shown in Figure 19-13, has been reported in 20 percent or more of VLBW preterm neonates, and as many as three-quarters of neonates with cerebellar hemorrhages also experience IVH [Limperopoulos et al., 2005; Sehgal et al., 2009; Steggerda et al., 2009]. The timing of these hemorrhages may differ, however, suggesting differences in pathophysiology of these injuries in the developing brain; Limperopoulos has reported that the mean age for diagnosis of CBH is 5.2 days, compared to 1.7 days for IVH [Limperopoulos et al., 2005]. The risk factors for CBH include lack of antenatal steroid exposure, emergent cesarian section, hypotension requiring medical therapy, sepsis, patent ductus arteriosus, and lower 5-day minimum pH values for the neonates with CBH.
Infants who experience CBH are at high risk for neurodevelopmental handicap [O’Shea et al., 2008]. Limperopoulos reported that children with CBH had significantly more severe motor disabilities, language delays, and cognitive deficits at 2–3 years of age than preterm control subjects of similar gestational age, although preterm infants with CBH and grade 4 IVH were not at overall greater risk for neurodevelopmental disabilities [Limperopoulos et al., 2007].
White Matter Injury of the Premature Newborn
White matter injury (WMI) is the predominant lesion of the premature newborn brain, resulting in much of the cognitive and behavioral impairments seen in children born prematurely, and to a lesser extent, the motor impairments. Notably, the true incidence of this injury is not known, largely because detection of the mild form of this lesion is difficult using conventional neuroimaging and because the threshold for determining signal abnormality in the cerebral white matter has not been rigorously defined. Studies have shown a decline in the incidence of cystic WMI, which is easily detected by ultrasound, but the incidence of clinically important noncystic WMI is unknown [Groenendaal et al., 2010; Hamrick et al., 2004]. White matter injury is used increasingly in place of the traditional term periventricular leukomalacia (PVL) or periventricular leukoencephalopathy, although the term PVL is still commonly used. WMI is a somewhat broader term than PVL in that it denotes the diffuse lesion of the cerebral white matter that extends beyond the periventricular regions defined in initial neuropathologic and ultrasonographic studies, and is often a noncystic lesion. An even more encompassing term, “encephalopathy of prematurity,” was proposed by Volpe to include the findings of neuronal abnormalities in gray matter structures demonstrated by neuropathology and neuroimaging studies in addition to the white matter injury [Volpe, 2005, 2009a]. This term is not yet in widespread use in the literature, but it reflects increasing evidence that premature newborns suffer a brain injury that affects many gray matter structures in addition to the cerebral white matter. Although WMI with a similar imaging pattern to WMI in the preterm infant has been reported in infants born at term [Kwong et al., 2004] and in term newborns with congenital heart disease [Galli et al., 2004], this section of the chapter will focus exclusively on the pathology, pathogenesis, and clinical features of WMI of the premature newborn.
Neuropathology
The neuropathology of WMI was first described by Banker and Larroche in their classic 1962 report detailing the histological findings in 51 autopsy specimens [Banker and Larroche, 1962]. They described PVL as including bilateral areas of focal necrosis, gliosis, and disruption of axons, with the so-called “retraction clubs and balls.” They noted the topographical distribution of the lesions to be in the periventricular white matter dorsolateral to the lateral ventricles, primarily anterior to the frontal horns and dorsolateral to the occipital horns. They noted that a severe anoxic episode occurred in 50 of 51 infants and that the lesions were consistently observed in the location of the border zone of the vascular supply, which they suggested indicated that hypoxia-ischemia affecting the watershed regions of the white matter was a major pathogenetic factor. Second, their observation that 75 percent of the infants were born prematurely suggested a particular vulnerability of the periventricular white matter of the immature brain.
Further neuropathological studies extended these initial observations, demonstrating that, in many cases, WMI consists of areas of both focal necrosis (which become cystic) in the deep periventricular white matter, and a diffuse gliosis of the cerebral white matter, often extending well beyond the periventricular region [Deguchi et al., 1997; Gilles and Murphy, 1969; Takashima et al., 2009]. The acute lesions of WMI are characterized by axonal swellings, microglial activation, and reactive astrocytosis [Takashima et al., 2009]. Older lesions of remote WMI consist of astrogliosis, microgliosis, and loss of myelination, and may contain cystic foci. The cysts may be quite small, measuring <1 mm in some cases [Pierson et al., 2007], which may not be detectable by conventional imaging studies. In addition, there may be neovascularization around necrotic foci [Takashima et al., 2009]. The diffuse gliotic lesion of WMI consists of proliferation of hypertrophic, reactive astrocytes and activated microglia, loss of oligodendrocytes, and axonal damage. Both the cystic and gliotic white matter lesions are followed by an overall decrease in the volume of myelin (hypomyelination), and often a decrease in cerebral white matter volume with accompanying increase in ventricular volume (atrophic ventriculomegaly). Immunomarkers of axonal pathology, such as β-amyloid precursor protein and fractin, show that this reduction in white matter volume is related to a diffuse injury to axons beyond necrotic regions, and not only to loss of oligodendrocytes and subsequent hypomyelination [Arai et al., 1995; Haynes et al., 2008].
The recognition of a significant component of neuronal loss and injury occurred relatively recently, as early neuropathological studies were focused on the obvious white matter pathology and reported little in the way of overt neuronal injury in premature newborns [Banker and Larroche, 1962; Gilles and Murphy, 1969]. Findings from neuroimaging research studies were a factor that prompted re-examination of neuronal injury in preterm newborns with WMI, since quantitative MRI studies showed that WMI injury was accompanied by marked reductions in the volume of cortical and subcortical gray matter, with relatively smaller reductions in white matter volume [Boardman et al., 2006; Inder et al., 1999, 2005; Kapellou et al., 2006]. Recent neuropathologic studies have now demonstrated that there is significant neuronal loss and gliosis in the thalamus, basal ganglia, and cerebral cortex associated with WMI in human infants born prematurely [Andiman et al., 2010; Ligam et al., 2008; Pierson et al., 2007]. In addition, gliosis and neuronal loss were found in the cerebellar white matter, cortex, and nuclei, and selected brainstem nuclei of some infants with WMI [Pierson et al., 2007].
It is still not entirely clear whether the neuronal loss and gliosis are predominantly a consequence of a primary injury to axons of the cerebral white matter, or whether there is a separate direct injury to neurons of the cortical, subcortical, and cerebellar gray matter, or both. Volpe’s elegant review synthesizes the neuropathologic findings of WMI in the preterm brain, together with the current understanding of brain development at this age to present a comprehensive thesis of how the interaction of these destructive and developmental processes produces the “encephalopathy of prematurity” [Volpe, 2009a]. He proposes that WMI in the preterm brain involves primary destructive effects, as well as widespread disturbances of brain maturation and development, producing a diffuse brain “lesion.” Volpe introduced the term “encephalopathy of prematurity” to reflect the widespread nature of this combined gray and white matter pathology of the immature brain. In particular, he explained how the numerous critical developmental processes occurring during the vulnerable period of 24–36 weeks’ gestation may be disturbed by WMI, affecting specific cortical, subcortical, and cerebellar neurons by direct and indirect mechanisms. He proposed that primary axonal injury and loss in the cerebral white matter are likely responsible for some degree of secondary neuronal injury and loss in the cortex, thalamus, and cerebellum. An autopsy study demonstrated that there is a marked reduction of pyramidal neurons in layer V of the cortex of preterm newborns, which was hypothesized to occur secondary to axonal injury and loss [Andiman et al., 2010]. However, there may also be direct injury to and loss of developing neurons, such as subplate neurons located in the subcortical white matter [Volpe, 2005], which peak at gestational age 26–29 weeks and have been shown to be particularly vulnerable to hypoxia-ischemia in the rodent [McQuillen et al., 2003]. Similarly, a small autopsy series of preterm newborns showed a loss of cortical GABAergic neurons that normally migrate through the cerebral white matter in the third trimester [Robinson et al., 2006]. Further work is needed to delineate the precise nature and etiology of the neuronal abnormalities in preterm WMI, but it is now clear that the “injury” to the developing brain of the preterm newborn extends beyond the well-recognized white matter pathology.
Pathogenesis
The distinctive lesion of WMI found in the immature white matter of preterm newborns likely results from the interaction of multiple pathogenetic factors. Numerous risk factors for the development of WMI have been identified, but in most cases three major factors contribute to the pathogenesis of WMI in premature newborns (Box 19-3):
Hypoxia-Ischemia
Banker and Larroche originally suggested that WMI occurred in the regions of vascular border zones in the cerebral white matter, and that ischemia would thus be expected to affect these zones preferentially [Banker and Larroche, 1962]. Subsequent neuropathological studies employed postmortem injections of the vessels to demonstrate vascular border and end zones in the periventricular white matter where WMI is typically found [De Reuck, 1984; Takashima and Tanaka, 1978]. These watershed zones are thought to render the periventricular white matter vulnerable to ischemic injury during periods of low cerebral perfusion. This tenuous vascular structure is notable, given that cerebral blood flow is quite low in the white matter of preterm newborns [Borch and Greisen, 1998]. Moreover, there is evidence to suggest that critically ill premature newborns may have at least an intermittently pressure-passive circulation, which puts them at risk for episodes of cerebral ischemia [Lou et al., 1979; Soul et al., 2007]. Indeed, a higher incidence of WMI (as well as IVH) was found in one study of newborns who demonstrated evidence of a pressure-passive circulation [Tsuji et al., 2000]. In a larger prospective study, episodes of pressure-passivity were found in the first 5 days after birth in 97 percent of critically ill newborns with birth weights of <1500 g, with some of the sickest newborns of gestational age <29 weeks showing frequent episodes of pressure-passivity (>50 percent) and hypotension (>40 percent) [Soul et al., 2007]. Notably, these fluctuations in pressure-passivity did not necessarily correlate with periods of hypotension, which occurred less often than episodes of pressure-passivity. These data suggest that a normal blood pressure does not necessarily guarantee adequate cerebral perfusion, particularly given the uncertainty regarding normal or ideal blood pressure ranges for preterm newborns [Laughon et al., 2007].
In addition to the intrinsic properties of the cerebral circulation in preterm newborns, the occurrence of other medical illnesses may contribute to episodes of cerebral ischemia. Newborns are often hypotensive in the first hours and days after birth for a variety of reasons, and a patent ductus arteriosus in particular may contribute to systemic and cerebral hypoperfusion. Serious postnatal infections, such as necrotizing enterocolitis, sepsis, and/or meningitis often affect both systemic and cerebral hemodynamics. Hypoxia and hypercarbia from acute and chronic lung disease, and the impact of various ventilation strategies, suctioning, medications, infusions, transfusions, and other interventions may all exert a deleterious effect on cerebral hemodynamics and contribute to the pathogenesis of WMI [Greisen et al., 1987a; Hoecker et al., 2002; Murase and Ishida, 2005; Patel et al., 2000; Perlman and Volpe, 1983; Perlman et al., 1983; van Alfen-van der Velden et al., 2006].
Inflammation/Infection
Both epidemiological and experimental studies suggest a role for inflammation and/or infection in the pathogenesis of WMI [Dammann and Leviton, 1997; Leviton et al., 1999b, 2010; Van Marter et al., 2002; Wu and Colford, 2000; Yoon et al., 1996]. Studies have shown an association between maternal infection, prolonged rupture of membranes, cord blood IL-6 levels, placental inflammation/infection, and WMI, suggesting that maternal infection is an important etiologic factor in the pathogenesis of WMI [Dammann and Leviton, 1997; Yoon et al., 1996]. Similarly, postnatal infection has been found to be associated with WMI [Shah et al., 2008; Van Marter et al., 2002]. Neuropathologic data showing that there is an abundance of activated microglia in the white matter of the preterm brain suggest a potential mechanism by which WMI may be mediated [Billiards et al., 2006]. Indeed, rodent studies show that activated microglia are necessary to mediate impaired development and death of immature oligodendrocytes by lipopolysaccharide [Lehnardt et al., 2002; Pang et al., 2010]. Peroxynitrite produced by inducible nitric oxide synthase and nicotinamide adenine dinucleotide phosphate (NADPH) oxidase in activated microglia likely plays a role in lipopolysaccharide-induced death of oligodendroglia [Li et al., 2005], and excitotoxicity mediated by N-methyl-D-aspartate (NMDA) receptors on microglia appears to mediate astrocyte death [Tahraoui et al., 2001]. Experimental studies suggest a role for cytokines, such as interferon-γ, in the pathogenesis of WMI. For example, apoptotic death of immature oligodendrocytes has been demonstrated in the presence of interferon-γ, a cytokine generated by macrophages and reactive astrocytes that likely acts on the premyelinating oligodendrocytes through receptor-mediated mechanisms [Baerwald and Popko, 1998; Folkerth et al., 2004a]. These experimental data demonstrate mechanisms by which inflammation/infection may result in WMI, given the observed association between infection and WMI in human epidemiological studies. It should be noted that hypoxia-ischemia can result in activation of microglia, providing another mechanism for oligodendrocyte injury and death, and that significant infection may result in hypoxia-ischemia; thus there is likely an interaction or even synergism between these two mechanisms of injury. There are likely to be genetic factors that modify the degree to which inflammation/infection contributes to WMI, as demonstrated by a study where specific IL-6 genotypes were associated with mental retardation in children with WMI [Resch et al., 2009]. Thus there is increasing evidence of the important role of antenatal and postnatal inflammation/infection in the pathogenesis of WMI; further work is needed to elucidate the specific mechanisms involved and potential preventive therapies.
Vulnerability of Immature White Matter, Particularly Immature Oligodendrocytes
The hypothesis that the periventricular white matter of the premature newborn may be more vulnerable to anoxia than the mature brain was also proposed by Banker and Larroche in their seminal paper [Banker and Larroche, 1962]. A maturational vulnerability of the periventricular white matter is suggested by the finding that WMI occurs much more commonly in the preterm newborn than in the term newborn. Specifically, the observation that the diffuse gliotic lesion of WMI affects the immature oligodendrocyte predominantly, with relative preservation of other cellular elements, suggests that the immature (premyelinating) oligodendrocyte is the cell most vulnerable to injury at this age. Immature oligodendrocytes are susceptible to injury and apoptotic cell death by free radical attack [Back et al., 1998; Oka et al., 1993]. Indeed, apoptosis was demonstrated to be the mechanism of free radical-mediated cell death by cystine deprivation [Back et al., 1998], which was an important finding because apoptosis is postulated to be the mechanism of cell death by moderate ischemia, rather than necrosis, which typically results from severe ischemic insults [Ankarcrona et al., 1995]. A relative lack of antioxidant defenses in the immature oligodendrocyte is one reason why this cell population is particularly vulnerable to free radical attack [Folkerth et al., 2004b]. In addition to free radical attack, premyelinating oligodendrocytes are also susceptible to injury or death by excitotoxicity [Deng et al., 2006; Follett et al., 2004; Husson et al., 2005]. This vulnerability to excitotoxicity likely results from high levels of extracellular glutamate due to high expression of glutamate transporter and because of high expression of glutamate receptors (AMPA and NMDA) by immature oligodendrocytes [Deng et al., 2006; Desilva et al., 2007; Follett et al., 2004; Husson et al., 2005; Karadottir and Attwell, 2007; Rosenberg et al., 2003]. Thus there is cellular and biochemical evidence to support the original postulate that the preterm newborn’s white matter displays a maturational vulnerability that contributes to the high incidence of WMI.
Additional Risk Factors
There are numerous risk factors for WMI that have been identified in epidemiologic and prospective studies of preterm newborns, but the precise mechanism by which each risk factor exerts its effect is not always clear. Lower gestational age at birth and birth weight are associated with a higher risk of WMI and associated neuronal abnormalities, but these two factors are likely related to the three major pathogenetic factors listed above, as well as to other genetic and acquired risk factors [Inder et al., 2003b, 2005]. Chronic ventilation for chronic lung disease in preterm newborns has been identified as a risk factor for later neurodevelopmental deficits [Short et al., 2003], which may be mediated by the respiratory, hemodynamic, and inflammatory disturbances known to be associated with chronic lung disease and ventilator support.
Intraventricular hemorrhage
As described in the previous section on IVH, it is difficult to separate IVH and WMI in many neuropathology, imaging, and clinical studies of preterm newborns because they are often both present [Armstrong et al., 1987; Takashima et al., 1989]. In fact, it is likely that IVH increases the risk of WMI, not only because these two entities share some of the same pathogenetic risk factors, but also because IVH may exacerbate WMI [Takashima et al., 1989]. For example, one study showed that ventricular dilatation in the presence of IVH by neonatal ultrasonography was associated with worse cognitive and motor outcome when compared with isolated ventricular dilatation, suggesting that IVH may increase the severity of WMI [Vollmer et al., 2006]. One hypothesis to explain this observation is that non-protein-bound iron from IVH contributes to the generation of free radicals, which causes or exacerbates WMI via free radical attack [O’Donovan and Fernandes, 2004; Savman et al., 2001].
Postnatal corticosteroid use
Clinical studies of postnatal corticosteroids given to treat lung disease have shown an association with worse neurodevelopmental outcome in preterm treated compared to untreated newborns [Barrington, 2001; Wood et al., 2005]. Postnatal hydrocortisone may have a less deleterious effect on the developing brain than dexamethasone [Lodygensky et al., 2005; Watterberg et al., 2007]. It is currently unclear how postnatal steroids exert their effect on the brain: for example, whether steroids contribute to WMI or whether steroids have an independent deleterious effect on the developing brain. Dexamethasone may exacerbate WMI through mechanisms such as exacerbation of ischemic injury, but may also directly impair neurogenesis and neuronal maturation [Baud, 2004]. As a result of published data showing the adverse effect of postnatal steroids on neurodevelopmental outcome, there has been a significant decline in the routine use of steroids to treat lung disease in most centers, except in cases of very severe lung disease. In contrast, antenatal steroid use decreases neonatal death and does not worsen neurodevelopmental outcome [Foix-L’Helias et al., 2008], and thus is administered routinely to women in preterm labor.
Nutrition
Relatively little attention has been paid to the effects of nutrition on brain injury and development in preterm newborns, but it is likely that nutritional factors, such as total caloric intake, as well as specific vitamins and nutrients, are key to optimal brain development and neurologic outcome. A trial comparing two nutritional approaches demonstrated that energy deficit at 28 days of age correlated with total brain volume and developmental outcome, even though there was no difference in measures of brain volumes or outcome between the two groups [Tan et al., 2008]. Further work is needed in this critically important field of early life nutrition and its impact on brain development.
Clinical and EEG Findings
WMI is a typically a clinically silent lesion, evolving over days to weeks with few or no outward neurologic signs until weeks to months later when spasticity is first detected, or at an even later age when children present with cognitive difficulties at preschool or school age. With moderate to severe WMI, some degree of spasticity in the lower extremities may be detected by careful examination by term age or earlier, and there may be axial flexor weakness or proximal appendicular weakness when WMI is severe. Most commonly, however, WMI is diagnosed in the neonatal period by routine cranial ultrasonography or by MRI [Maalouf et al., 2001]. Since detection of WMI is not possible by routine clinical examination during the newborn period in the majority of cases, imaging studies are needed to detect WMI (as well as IVH), as recommended in standard published guidelines [Ment et al., 2002]. The importance of detecting WMI in the newborn period relates to the institution of early monitoring and intervention services, which may improve the care and long-term outcome of children born prematurely.
There have been numerous reports regarding the utility of EEG in the identification of brain injury by background or focal abnormalities, such as the transient appearance of sharp waves. Although there has been increasing use of one- or two-channel EEG monitoring (aEEG), this reduced montage EEG is most useful for identifying significant abnormalities of background patterns or trends over time, rather than focal abnormalities such as positive rolandic sharp waves [Greisen et al., 1987b]. Early conventional EEG studies reported that positive rolandic sharp waves appeared to be a highly specific but not highly sensitive marker of WMI in premature newborns [Aso et al., 1989; Clancy and Tharp, 1984; Marret et al., 1992; Novotny et al., 1987]. Positive rolandic sharp waves have also been observed in some newborns with large IVH, although this finding is likely related to coexisting WMI rather than IVH alone [Clancy and Tharp, 1984; Novotny et al., 1987]. Significant abnormalities of the EEG background pattern, particularly in the first days after birth, also have been shown to correlate with WMI and later neurodevelopmental impairments. In particular, the severity of EEG abnormalities has been shown to correlate with the severity of WMI [Aso et al., 1989; Kidokoro et al., 2009]. Serial EEG studies are more useful than a single EEG for the identification of either the rolandic sharp waves or background abnormalities that correlate with WMI and long-term neurologic deficits [Hellstrom-Westas and Rosen, 2005; Kidokoro et al., 2009]. In particular, the combination of an early EEG in the first days after birth to identify acute abnormalities, followed by EEG in the second week and 1–2 months later, is best for identifying the evolution of EEG abnormalities associated with WMI [Kidokoro et al., 2009]. Interestingly, the observation that WMI is associated with early acute EEG changes, followed by later chronic abnormalities (coinciding with cyst formation, when that occurs), supports the idea that the insult resulting in WMI occurs around or very soon after birth [Volpe, 2009b].
Neuroimaging
Ultrasound
The evolution of echogenic (or hyperechoic) lesions in the periventricular white matter over the first few weeks after birth, with or without cyst formation (which are echolucent, or hypoechoic), is the classic description of WMI detected by serial cranial ultrasound studies (Figure 19-14). None the less, the detection of WMI by cranial ultrasound remains problematic, in that interobserver variability is high even in rigorous studies of WMI by cranial ultrasound, particularly with regard to the detection of hyperechoic lesions, with less than 50 percent agreement among observers in one study [Kuban et al., 2007]. Furthermore, there is no general agreement regarding the number, size, location, and persistence of hyperechoic lesions defining WMI diagnosed by serial cranial ultrasound [Kuban et al., 2001]. In contrast, hypoechoic or echolucent lesions denoting cysts and the presence of ventriculomegaly are easily and reliably detected by readers of cranial ultrasound [Kuban et al., 2007]. Notably, cystic lesions and significant ventriculomegaly are significantly associated with later cerebral palsy [Kuban et al., 2009]. Ventriculomegaly resulting from atrophy of the periventricular white matter (i.e., volume loss) is usually present within weeks after birth (see Figure 19-14), although it may not be observed by cranial ultrasound until late in the newborn period, suggesting that a cranial ultrasound performed prior to discharge from the neonatal intensive care unit provides additional diagnostic benefit, particularly when previous studies have been normal. Notably, isolated ventriculomegaly is associated with an increased risk of CP [Allan et al., 1997], suggesting that ventriculomegaly without radiologically evident white matter abnormalities likely indicates WMI, particularly in the absence of IVH. Studies correlating ultrasound and autopsy data have demonstrated that the incidence of WMI is underestimated by cranial ultrasound [Carson et al., 1990; Hope et al., 1988], and in the living newborn, MRI has been shown to be superior for the detection of WMI and other brain lesions in the preterm newborn.
Magnetic Resonance Imaging
MRI has been increasingly used to detect WMI in newborns and children born prematurely, although cranial ultrasound remains the preferred imaging modality for most centers because of its portability, safety, and lower cost. Conventional T1- or T2-weighted MRI sequences easily demonstrate cystic lesions that are the hallmark of the focal necrotic lesions of WMI. Noncystic WMI is also detected by MRI, evident as high signal intensity in the cerebral white matter by T2-weighted MRI, and low signal intensity by T1-weighted sequences in the newborn period. Diffusion weighted imaging (DWI) shows restricted diffusion if the MRI is obtained very early in the newborn period [Bozzao et al., 2003], but scans at later ages show increased diffusion in areas of WMI [Counsell et al., 2003]. Punctate lesions have also been detected by MRI in the white matter, often in the corona radiata and posterior periventricular white matter (and occasionally the gray matter) [Dyet et al., 2006; Ramenghi et al., 2007]. The etiology and neuropathology of these punctate lesions are currently unknown, although they have been hypothesized to represent tiny infarctions (possibly venous infarcts), small hemorrhages, or areas of activated microglia or increased cellularity [Rutherford et al., 2010]. Similarly, the clinical significance of these punctate lesions is unknown and follow-up studies are needed to determine if these lesions are associated with neurodevelopmental impairments. MRI performed in infancy or childhood can be useful for the detection of WMI, particularly for children who present with neurodevelopmental impairments but whose neonatal cranial ultrasound studies were normal or only mildly abnormal. MRI studies beyond the newborn period rarely show cysts but typically show increased signal intensity on T2-weighted and fluid-attenuated inversion-recovery (FLAIR) images in areas of gliotic white matter with delayed or decreased myelination (Figure 19-15). Thinning of the corpus callosum usually indicates a decrease in cerebral white matter volume (atrophy) associated with WMI.
MRI has been demonstrated to be more sensitive than cranial ultrasound for the detection of WMI in preterm newborns, especially for the noncystic form of WMI [Inder et al., 2003a; Maalouf et al., 2001; Roelants-van Rijn et al., 2001]. As for cranial ultrasound studies, there is no universally accepted measure of the severity or extent of signal abnormality by MRI that defines WMI. While it is clear that greater severity of WMI is correlated with a higher incidence of later neurodevelopmental deficits, there is a broad range of outcomes for mild, moderate, and severe WMI [Woodward et al., 2006], and the threshold for defining clinically significant WMI has not been determined. For example, one study reported diffuse excessive high signal intensity (DEHSI) by T2-weighted MRI within the cerebral white matter at term age in 80 percent of infants born at 23–30 weeks gestational age [Dyet et al., 2006]. It has not yet been demonstrated that this high signal intensity on T2-weighted MRI is correlated with either neuropathological correlates of WMI (such as gliosis) or clinically significant WMI, although the authors reported a correlation between DEHSI and mild developmental delay at 18 months of age [Dyet et al., 2006]. Thus caution is needed when prognosticating outcome based on neuroimaging findings in preterm newborns.
Numerous MRI research studies have been helpful in elucidating the timing, distribution, and effects of WMI in children born prematurely. MRI scans performed soon after birth may be useful for demonstrating acute evidence of WMI, although later MRI studies (e.g., at term age) are superior for demonstrating the full extent of any WMI, gray matter abnormalities, or other congenital or acquired structural brain abnormalities. Diffusion tensor imaging (DTI) has been useful to demonstrate normal white matter development, as well as acute and chronic white matter abnormalities, showing the disruption of normal white matter architecture with WMI [Counsell et al., 2003; Huppi et al., 2001; Yoo et al., 2005]. These DTI abnormalities also have been shown to correlate with developmental outcome [Krishnan et al., 2007]. Similarly, volumetric analysis of brain MRI data has been used to demonstrate normal brain development and the specific gray and white matter volume changes related to WMI. Volumetric MRI data analysis was key to the discovery of the marked reduction in cortical and subcortical gray matter volumes accompanying WMI that had been underappreciated prior to the availability of these quantitative measurement techniques [Abernethy et al., 2004; Inder et al., 2005; Peterson et al., 2000]. Volumetric MRI studies of older children born preterm have been particularly useful in determining the clinical correlates of these volumetric changes, since the larger, mostly myelinated brain of the older child or adolescent can be analyzed in greater detail and more precisely than that of the newborn, and cognitive, social, and behavioral outcome measures are also more definitive and specific than at the young ages often used in neonatal outcome studies [Abernethy et al., 2002; Nosarti et al., 2008; Peterson et al., 2000]. Such studies have demonstrated that, compared with term-born matched controls, children born preterm showed reduced volumes of the white matter, hippocampus, parieto-occipital and sensorimotor cortex, cerebellum, and basal ganglia, and larger ventricular volumes [Nosarti et al., 2008; Peterson et al., 2000]. There was some variability in the findings among studies, which may be related to differences in study populations and measurement techniques, but volumes of the hippocampus, caudate, and sensorimotor cortex correlated with measures of cognitive outcome such as IQ [Nosarti et al., 2008; Peterson et al., 2000]. There is much more to be learned about the pathogenesis and outcome of WMI by detailed quantitative analysis of volumetric, DTI, and functional MRI data obtained at both newborn and later ages, particularly when correlated with perinatal/neonatal risk factors for WMI and with functional outcome measures.
Recommendations for Imaging the Preterm Neonate and Child Born Preterm
Current recommendations for imaging the preterm neonate suggest that screening cranial ultrasound should be performed on all infants with gestational ages <30 weeks at 7–14 days of age, and should be repeated at 36–40 weeks postmenstrual age [Ment et al., 2002]. This recommendation by the Quality Standards Subcommittee of the American Academy of Neurology and the Practice Committee of the Child Neurology Society is designed to detect both clinically unsuspected IVH, which may require additional clinical and/or radiologic monitoring and changes in management plans, and evidence for PVL and/or ventriculomegaly, which are useful for prognosis and best seen when the infants are examined at term. Although older published guidelines do not recommend routine MRI scans for all premature newborns [Ment et al., 2002], emerging data suggest that cerebral MRI with DTI at term equivalent age may provide important prognostic information about developmental and motor handicap in the prematurely born. A brain MRI may also be performed in an older infant or child born prematurely to confirm clinically suspected WMI when there are cognitive, motor, and/or sensory impairments of unclear etiology (see Figure 19-15).
Outcome
WMI is the principal cause of the cognitive, behavioral, motor, and sensory impairments found in children born at <32 weeks gestational age [Volpe, 2003] (Box 19-4). Most outcome studies of children born prematurely are not restricted just to children with WMI, so it is difficult to quantify precisely the specific outcome attributable to WMI alone. That being said, it is likely that the great majority of cognitive, behavioral, motor, and sensory impairments and disabilities are related to WMI, with hemorrhagic venous infarction (grade 4 IVH) being the other major lesion that contributes significantly to neurologic disabilities in children born prematurely. In general, children with a severe neurologic disability in one domain often have multiple disabilities; for example, children with severe CP are more likely to have cognitive and sensory impairments than children without CP. The incidence of neurologic impairments increases with lower gestational age and birth weight, but there are many other antenatal and neonatal risk factors related to later neurodevelopmental impairments, including postneonatal factors, such as chronic medical illness, and environmental factors, such as socioeconomic status and maternal education [Vohr et al., 2003].
Box 19-4 Neurologic Problems Likely Related to White Matter Injury
Cognitive
Lower overall IQ and many specific types of cognitive impairments have been found in children born prematurely when compared with term-born children. Lower gestational age and birth weight are major risk factors for cognitive impairments, as demonstrated in a study of extremely low birth weight infants (<1000 g) showing that only 30 percent of such children were performing at grade level without extra support at 8 years of age [Bowen et al., 2002]. Chronic lung disease, in particular, has been associated with worse cognitive function and greater need for special education and therapies when comparing children born prematurely with and without lung disease [Short et al., 2003]. Deficits of attention and organization, math skills, and reading comprehension are much more common in children born prematurely than in term-born age-matched controls [Johnson et al., 2009]. Most large prospective studies of the cognitive outcome of older children born prematurely do not associate cognitive measures with specific brain lesions (such as WMI) by neonatal cranial ultrasound studies, but quantitative imaging studies to date suggest that it is indeed WMI and its gray matter correlates that underlie much of these cognitive impairments (see Neuroimaging section above) [Abernethy et al., 2002; Nosarti et al., 2008; Peterson et al., 2000]. Knowledge of neuroanatomy and the distribution of abnormalities with WMI allows for hypotheses regarding the potential neural substrate of various types of cognitive impairments. For example, deficits of attention and working memory may relate, at least in part, to involvement of the mediodorsal and reticular nuclei of the thalamus noted in one neuropathologic study of WMI [Ligam et al., 2008].
Social/Behavioral
There has been increasing recognition that children born prematurely have higher rates of social and behavioral difficulties than do term-born children [Delobel-Ayoub et al., 2006]. These include attention deficit/hyperactivity, emotional, anxiety, autism spectrum, and perhaps conduct disorders, particularly in children born extremely prematurely [Delobel-Ayoub et al., 2006; Johnson et al., 2010]. Of these, attentional problems are the most commonly recognized, with anxiety and other similar emotional disorders being increasingly identified and diagnosed. Various rates of these disorders have been reported, likely related in part to the age at evaluation and the method used to define or test for these disorders.
Motor
Lower gestational age at birth is also a risk factor for motor disability. The incidence of CP is much higher in children born extremely prematurely, occurring in approximately 20 percent of children born at ≤26 weeks gestational age, but in only 4 percent of children born at 32 weeks gestational age [Ancel et al., 2006; Wood et al., 2000]. Notably, one study showed that 25 percent of newborns diagnosed with WMI by neonatal cranial ultrasound had later CP, whereas only 4 percent with normal neonatal cranial ultrasound had CP, supporting the notion that significant motor impairments are typically related to WMI [Ancel et al., 2006]. Spastic diparesis is the most common form of CP in children born prematurely, which may present as quadriparesis when severe [Ancel et al., 2006; Kuban et al., 2008]. Hemiparesis is found less frequently than spastic diparesis, and is more likely to result from periventricular hemorrhagic infarction, but may result from asymmetric WMI [Ancel et al., 2006]. The topography of the cerebral white matter is such that the axons subserving the lower extremities are located closest to the ventricle, the axons of the upper extremities are situated lateral to them, and the axons of the facial musculature are located furthest from the ventricle. Since WMI is typically more severe proximal to the ventricle, WMI results in greater abnormalities of tone and power in the lower extremities than the upper extremities and face, i.e., a spastic diparesis. Dystonia is a frequent component of spastic diparesis caused by PVL, and occasionally hypotonia, ataxia, or some other motor abnormality is observed. In the absence of overt CP, coordination difficulties and other abnormalities of motor function or development may be found in children born prematurely.
Visual
While some children born prematurely have retinopathy of prematurity affecting their vision, WMI and other cerebral lesions alone can result in strabismus, nystagmus and other disorders of ocular motility, decreased acuity, visual field deficits, and perceptual difficulties, some of which may not be recognized until school age or later [Jacobson et al., 2002; Jacobson and Dutton, 2000; Pike et al., 1994]. Inferior visual field deficits may be found as a result of WMI, particularly since the optic radiations subserving the lower visual field and the white matter of the association areas of visual cortical regions are frequently affected by WMI [Jacobson et al., 1998]. Thalamic injury has also been shown to be associated with cerebral visual impairment in infants with WMI, separate from the effect of WMI alone on visual function [Ricci et al., 2006]. Children with WMI may have later visual perceptual defects or other higher-order visual impairments that contribute to their cognitive impairments, or in other words, worsen their cognitive and school function, so these are particularly important to detect [Jacobson et al., 1996].
Epilepsy
Children with severe WMI occasionally develop epilepsy, although epilepsy is more commonly related to gray matter lesions, such as periventricular venous infarction. In one study of 5-year-old children born at <26 weeks gestational age, 6 percent developed recurrent nonfebrile seizures [Wood et al., 2000]. Those premature newborns who develop later epilepsy likely have significant neuronal injury, such as the child whose MRI is shown in Figure 19-14, who developed infantile spasms.
Prevention
At the time of writing, there are no specific neuroprotective therapies or medications available during the newborn period to prevent or minimize WMI. Experimental data from animal models of WMI suggest promising neuroprotective agents to prevent or minimize WMI [Deng et al., 2006; Follett et al., 2004; Husson et al., 2005; Oka et al., 1993], but human trials of such agents are probably still years away. Current efforts are directed at prevention of WMI by addressing the known modifiable risk factors and pathogenetic mechanisms described above.
Preventive efforts ideally should begin antenatally, with prevention of maternal infection and other antenatal risk factors for the development of WMI. While known bacterial infections (such as group B streptococcus) are routinely treated by most obstetricians, there are no randomized trials to show that routine administration of antibiotics to women in preterm labor can prevent or minimize WMI in their newborns. Antenatal steroid administration appears to have a protective effect on the development of WMI, but this therapy is already administered to the vast majority of mothers in preterm labor as standard care [Foix-L’Helias et al., 2008; Leviton et al., 1999a]. After birth, maintenance of normal cerebral perfusion should be attempted by careful management of systemic hemodynamics, including blood pressure, intravascular volume, oxygenation, and ventilation, and avoidance of treatments or procedures that result in sudden changes in systemic and cerebral hemodynamics. There is significant controversy about management of blood pressure in the premature infant and even about what constitutes a normal or “ideal” blood pressure for a given gestational and postnatal age [Laughon et al., 2007]. Furthermore, a normal blood pressure does not necessarily imply normal cerebral perfusion, given the known impairments of cerebral pressure autoregulation in some premature newborns [Soul et al., 2007]. In addition, medications that improve blood pressure and systemic perfusion may have little or no effect on cerebral perfusion [Lundstrom et al., 2000]. The management of systemic and cerebral hemodynamics remains an area of active research, as studies have yet to provide guidelines to improve neurologic outcome. Avoidance and prompt treatment of infection may also minimize WMI, although no studies have demonstrated the effect of such interventions on WMI.
References
The complete list of references for this chapter is available online at www.expertconsult.com.
Abernethy L.J., Palaniappan M., Cooke R.W. Quantitative magnetic resonance imaging of the brain in survivors of very low birth weight. Arch Dis Child. 2002;87(4):279-283.
Abernethy L.J., Cooke R.W., Foulder-Hughes L. Caudate and hippocampal volumes, intelligence, and motor impairment in 7-year-old children who were born preterm. Pediatr Res. 2004;55(5):884-893.
Adcock K., Hedberg C., Loggins J., et al. The TNF-alpha-308, MCP-1 -2518 and TGF-beta1 +915 polymorphisms are not associated with the development of chronic lung disease in very low birth weight infants. Genes Immun. 2003;4(6):420-426.
Allan W.C., Sobel D.B. Neonatal intensive care neurology. Semin Pediatr Neurol. 2004;11(2):119-128.
Allan W.C., Vohr B., Makuch R.W., et al. Antecedents of cerebral palsy in a multicenter trial of indomethacin for intraventricular hemorrhage [see comments]. Arch Pediatr Adolesc Med. 1997;151(6):580-585.
Allen M.C. Neurodevelopmental outcomes of preterm infants. Curr Opin Neurol. 2008;21(2):123-128.
Allin M., Henderson M., Suckling J., et al. Effects of very low birthweight on brain structure in adulthood. Dev Med Child Neurol. 2004;46(1):46-53.
Ancel P.Y., Marret S., Larroque B., et al. Are maternal hypertension and small-for-gestational age risk factors for severe intraventricular hemorrhage and cystic periventricular leukomalacia? Results of the EPIPAGE cohort study. Am J Obstet Gynecol. 2005;193(1):178-184.
Ancel P.Y., Livinec F., Larroque B., et al. Cerebral palsy among very preterm children in relation to gestational age and neonatal ultrasound abnormalities: the EPIPAGE cohort study. Pediatrics. 2006;117(3):828-835.
Andiman S.E., Haynes R.L., Trachtenberg F.L., et al. The Cerebral Cortex Overlying Periventricular Leukomalacia: Analysis of Pyramidal Neurons. Brain Pathol. 2010.
Ankarcrona M., Dypbukt J.M., Bonfoco E., et al. Glutamate-induced neuronal death: a succession of necrosis or apoptosis depending on mitochondrial function. Neuron. 1995;15(4):961-973.
Arai Y., Deguchi K., Mizuguchi M., et al. Expression of beta-amyloid precursor protein in axons of periventricular leukomalacia brains. Pediatr Neurol. 1995;13(2):161-163.
Aranda J.V., Thomas R. Systematic review: intravenous Ibuprofen in preterm newborns. Semin Perinatol. 2006;30(3):114-120.
Armstrong D.L., Sauls C.D., Goddard-Finegold J. Neuropathologic findings in short-term survivors of intraventricular hemorrhage. Am J Dis Child. 1987;141(6):617-621.
Aronis S., Bouza H., Pergantou H., et al. Prothrombotic factors in neonates with cerebral thrombosis and intraventricular hemorrhage. Acta Paediatr Suppl. 2002;91(438):87-91.
Aso K., Scher M.S., Barmada M.A. Neonatal electroencephalography and neuropathology. J Clin Neurophysiol. 1989;6(2):103-123.
Aylward G.P. Neurodevelopmental outcomes of infants born prematurely. J Dev Behav Pediatr. 2005;26(6):427-440.
Back S.A., Gan X., Li Y., et al. Maturation-dependent vulnerability of oligodendrocytes to oxidative stress-induced death caused by glutathione depletion. J Neurosci. 1998;18(16):6241-6253.
Bada H.S. Routine indomethacin prophylaxis: has the time come? Pediatrics. 1996;98(4 Pt 1):784-785.
Baerwald K.D., Popko B. Developing and mature oligodendrocytes respond differently to the immune cytokine interferon-gamma. J Neurosci Res. 1998;52(2):230-239.
Baier R.J. Genetics of perinatal brain injury in the preterm infant. Front Biosci. 2006;11:1371-1387.
Ballabh P., Braun A., Nedergaard M. The blood-brain barrier: an overview: structure, regulation, and clinical implications. Neurobiol Dis. 2004;16(1):1-13.
Ballabh P., Hu F., Kumarasiri M., et al. Development of tight junction molecules in blood vessels of germinal matrix, cerebral cortex, and white matter. Pediatr Res. 2005;58(4):791-798.
Ballabh P., Xu H., Hu F., et al. Angiogenic inhibition reduces germinal matrix hemorrhage. Nat Med. 2007;13(4):477-485.
Banker B., Larroche J. Periventricular Leukomalacia of Infancy. Arch Neurol. 1962;7:386-410.
Barrington K.J. The adverse neuro-developmental effects of postnatal steroids in the preterm infant: a systematic review of RCTs. BMC Pediatr. 2001;1(1):1.
Baud O. Postnatal steroid treatment and brain development. Arch Dis Child Fetal Neonatal Ed. 2004;89(2):F96-F100.
Beck S., Wojdyla D., Say L., et al. The worldwide incidence of preterm birth: a systematic review of maternal mortality and morbidity. Bull World Health Organ. 2010;88(1):31-38.
Behrman R.E., Stith Butler A. Institute of Medicine Committee on Understanding Premature Birth and Assuring Healthy Outcomes Board on Health Sciences Outcomes: Preterm Birth: Causes, Consequences and Prevention. Washington DC: The National Academies Press; 2007.
Benson J.W., Drayton M.R., Hayward C., et al. Multicentre trial of ethamsylate for prevention of periventricular haemorrhage in very low birthweight infants. Lancet. 1986;2(8519):1297-1300.
Bertina R.M., Koeleman B.P., Koster T., et al. Mutation in blood coagulation factor V associated with resistance to activated protein C. Nature. 1994;369(6475):64-67.
Bhandari V., Bizzarro M.J., Shetty A., et al. Familial and genetic susceptibility to major neonatal morbidities in preterm twins. Pediatrics. 2006;117(6):1901-1906.
Bhutta A.T., Cleves M.A., Casey P.H., et al. Cognitive and behavioral outcomes of school-aged children who were born preterm: a meta-analysis. JAMA. 2002;288(6):728-737.
Bilguvar K., DiLuna M.L., Bizzarro M.J., et al. COL4A1 mutation in preterm intraventricular hemorrhage. J Pediatr. 2009;155(5):743-745.
Billiards S.S., Haynes R.L., Folkerth R.D., et al. Development of microglia in the cerebral white matter of the human fetus and infant. J Comp Neurol. 2006;497(2):199-208.
Boardman J.P., Counsell S.J., Rueckert D., et al. Abnormal deep grey matter development following preterm birth detected using deformation-based morphometry. Neuroimage. 2006;32(1):70-78.
Boardman J.P., Counsell S.J., Rueckert D., et al. Early growth in brain volume is preserved in the majority of preterm infants. Ann Neurol. 2007;62(2):185-192.
Borch K., Greisen G. Blood flow distribution in the normal human preterm brain. Pediatr Res. 1998;43(1):28-33.
Bowen J.R., Gibson F.L., Hand P.J. Educational outcome at 8 years for children who were born extremely prematurely: a controlled study. J Paediatr Child Health. 2002;38(5):438-444.
Bozzao A., Di Paolo A., Mazzoleni C., et al. Diffusion-weighted MR imaging in the early diagnosis of periventricular leukomalacia. Eur Radiol. 2003;13(7):1571-1576.
Braun A., Xu H., Hu F., et al. Paucity of pericytes in germinal matrix vasculature of premature infants. J Neurosci. 2007;27(44):12012-12024.
Carmeliet P. Angiogenesis in health and disease. Nat Med. 2003;9(6):653-660.
Carson S.C., Hertzberg B.S., Bowie J.D., et al. Value of sonography in the diagnosis of intracranial hemorrhage and periventricular leukomalacia: a postmortem study of 35 cases. Am J Neuroradiol. 1990;11(4):677-683.
Cherian S., Whitelaw A., Thoresen M., et al. The pathogenesis of neonatal post-hemorrhagic hydrocephalus. Brain Pathol. 2004;14(3):305-311.
Chiswick M., Gladman G., Sinha S., et al. Vitamin E supplementation and periventricular hemorrhage in the newborn. Am J Clin Nutr. 1991;53(1 Suppl):370S-372S.
Chua C.O., Chahboune H., Braun A., et al. Consequences of Intraventricular Hemorrhage in a Rabbit Pup Model. Stroke. 2009.
Clancy R.R., Tharp B.R. Positive rolandic sharp waves in the electroencephalograms of premature neonates with intraventricular hemorrhage. Electroencephalogr Clin Neurophysiol. 1984;57(5):395-404.
Clyman R.I. Recommendations for the postnatal use of indomethacin: an analysis of four separate treatment strategies. J Pediatr. 1996;128(5 Pt 1):601-607.
Cooke R.W. Factors associated with periventricular haemorrhage in very low birthweight infants. Arch Dis Child. 1981;56(6):425-431.
Cools F., Offringa M. Neuromuscular paralysis for newborn infants receiving mechanical ventilation. Cochrane Database Syst Rev. (2):2005. CD002773
Counsell S.J., Allsop J.M., Harrison M.C., et al. Diffusion-weighted imaging of the brain in preterm infants with focal and diffuse white matter abnormality. Pediatrics. 2003;112(1 Pt 1):1-7.
Crowley P. Antenatal corticosteroids–current thinking. BJOG. 2003;110(Suppl 20):77-78.
Crowley P. Prophylactic corticosteroids for preterm birth. Cochrane Database Syst Rev. (2):2000. CD000065
Crowley P. Update of the antenatal steroid meta-analysis: current knowledge and future research needs. The effect of antenatal steroids for fetal maturation on perinatal outcomes. NIH Consens Statement. 1994;12(2):161-205.
Crowley P.A. Antenatal corticosteroid therapy: a meta-analysis of the randomized trials, 1972 to 1994. Am J Obstet Gynecol. 1995;173(1):322-335.
Dammann O., Leviton A. Inflammatory brain damage in preterm newborns–dry numbers, wet lab, and causal inferences. Early Hum Dev. 2004;79(1):1-15.
Dammann O., Leviton A. Maternal intrauterine infection, cytokines, and brain damage in the preterm newborn. Pediatr Res. 1997;42(1):1-8.
Debus O., Koch H.G., Kurlemann G., et al. Factor V Leiden and genetic defects of thrombophilia in childhood porencephaly. Arch Dis Child Fetal Neonatal Ed. 1998;78(2):F121-F124.
Deguchi K., Oguchi K., Takashima S. Characteristic neuropathology of leukomalacia in extremely low birth weight infants. Pediatr Neurol. 1997;16(4):296-300.
Delobel-Ayoub M., Kaminski M., Marret S., et al. Behavioral outcome at 3 years of age in very preterm infants: the EPIPAGE study. Pediatrics. 2006;117(6):1996-2005.
Del Toro J., Louis P.T., Goddard-Finegold J. Cerebrovascular regulation and neonatal brain injury. Pediatr Neurol. 1991;7(1):3-12.
Deng W., Yue Q., Rosenberg P.A., et al. Oligodendrocyte excitotoxicity determined by local glutamate accumulation and mitochondrial function. J Neurochem. 2006;98(1):213-222.
Deonna T., Payot M., Probst A., et al. Neonatal intracranial hemorrhage in premature infants. Pediatrics. 1975;56(6):1056-1064.
De Reuck J.L. Cerebral angioarchitecture and perinatal brain lesions in premature and full-term infants. Acta Neurol Scand. 1984;70(6):391-395.
Desilva T.M., Kinney H.C., Borenstein N.S., et al. The glutamate transporter EAAT2 is transiently expressed in developing human cerebral white matter. J Comp Neurol. 2007;501(6):879-890.
De Vries L.S., Groenendaal F., van Haastert I.C., et al. Asymmetrical myelination of the posterior limb of the internal capsule in infants with periventricular haemorrhagic infarction: an early predictor of hemiplegia. Neuropediatrics. 1999;30(6):314-319.
De Vries L.S., Van Haastert I.L., Rademaker K.J., et al. Ultrasound abnormalities preceding cerebral palsy in high-risk preterm infants. J Pediatr. 2004;144(6):815-820.
de Vries L.S., Roelants-van Rijn A.M., Rademaker K.J., et al. Unilateral parenchymal haemorrhagic infarction in the preterm infant. Eur J Paediatr Neurol. 2001;5(4):139-149.
de Vries L.S., Koopman C., Groenendaal F., et al. COL4A1 mutation in two preterm siblings with antenatal onset of parenchymal hemorrhage. Ann Neurol. 2009;65(1):12-18.
Dolfin T., Skidmore M.B., Fong K.W., et al. Incidence, severity, and timing of subependymal and intraventricular hemorrhages in preterm infants born in a perinatal unit as detected by serial real-time ultrasound. Pediatrics. 1983;71(4):541-546.
Donat J.F., Okazaki H., Kleinberg F., et al. Intraventricular hemorrhages in full-term and premature infants. Mayo Clin Proc. 1978;53(7):437-441.
Donn S.M., Roloff D.W., Goldstein G.W. Prevention of intraventricular haemorrhage in preterm infants by phenobarbitone. A controlled trial. Lancet. 1981;2(8240):215-217.
Dubowitz L., Dubowitz V., Mercuri E. The neurological assessment of the preterm and full-term newborn infant. Cambridge: McKeith Press; 1998.
du Plessis A.J. Cerebrovascular injury in premature infants: current understanding and challenges for future prevention. Clin Perinatol. 2008;35(4):609-641. v
Dyet L.E., Kennea N., Counsell S.J., et al. Natural history of brain lesions in extremely preterm infants studied with serial magnetic resonance imaging from birth and neurodevelopmental assessment. Pediatrics. 2006;118(2):536-548.
El-Khoury N., Braun A., Hu F., et al. Astrocyte end-feet in germinal matrix, cerebral cortex, and white matter in developing infants. Pediatr Res. 2006;59(5):673-679.
Express Group, Fellman V., Hellstrom-Westas L., et al. One-year survival of extremely preterm infants after active perinatal care in Sweden. JAMA. 2009;301(21):2225-2233.
Fabres J., Carlo W.A., Phillips V., et al. Both extremes of arterial carbon dioxide pressure and the magnitude of fluctuations in arterial carbon dioxide pressure are associated with severe intraventricular hemorrhage in preterm infants. Pediatrics. 2007;119(2):299-305.
Fanaroff A.A., Hack M., Walsh M.C. The NICHD neonatal research network: changes in practice and outcomes during the first 15 years. Semin Perinatol. 2003;27(4):281-287.
Fanaroff A.A., Stoll B.J., Wright L.L., et al. Trends in neonatal morbidity and mortality for very low birthweight infants. Am J Obstet Gynecol. 196(2), 2007. 147 e1–147 e8
Fauchere J.C., Dame C., Vonthein R., et al. An approach to using recombinant erythropoietin for neuroprotection in very preterm infants. Pediatrics. 2008;122(2):375-382.
Ferrara N., Gerber H.P., LeCouter J. The biology of VEGF and its receptors. Nat Med. 2003;9(6):669-676.
Foix-L’Helias L., Marret S., Ancel P.Y., et al. Impact of the use of antenatal corticosteroids on mortality, cerebral lesions and 5-year neurodevelopmental outcomes of very preterm infants: the EPIPAGE cohort study. BJOG. 2008;115(2):275-282.
Folkerth R.D., Keefe R.J., Haynes R.L., et al. Interferon-gamma expression in periventricular leukomalacia in the human brain. Brain Pathol. 2004;14(3):265-274.
Folkerth R.D., Haynes R.L., Borenstein N.S., et al. Developmental lag in superoxide dismutases relative to other antioxidant enzymes in premyelinated human telencephalic white matter. J Neuropathol Exp Neurol. 2004;63(9):990-999.
Follett P.L., Deng W., Dai W., et al. Glutamate receptor-mediated oligodendrocyte toxicity in periventricular leukomalacia: a protective role for topiramate. J Neurosci. 2004;24(18):4412-4420.
Fowlie P.W., Davis P.G. Prophylactic indomethacin for preterm infants: a systematic review and meta-analysis. Arch Dis Child Fetal Neonatal Ed. 2003;88(6):F464-F466.
Fowlie P.W., Davis P.G. Prophylactic intravenous indomethacin for preventing mortality and morbidity in preterm infants. Cochrane Database Syst Rev. (3):2002. CD000174
Galli K.K., Zimmerman R.A., Jarvik G.P., et al. Periventricular leukomalacia is common after neonatal cardiac surgery. J Thorac Cardiovasc Surg. 2004;127(3):692-704.
Ghorashian S., Hunt B.J. “Off-license” use of recombinant activated factor VII. Blood Rev. 2004;18(4):245-259.
Gilles F.H., Murphy S.F. Perinatal telencephalic leucoencephalopathy. J Neurol Neurosurg Psychiatry. 1969;32(5):404-413.
Gimenez M., Junque C., Narberhaus A., et al. White matter volume and concentration reductions in adolescents with history of very preterm birth: a voxel-based morphometry study. Neuroimage. 2006;32(4):1485-1498.
Gopel W., Gortner L., Kohlmann T., et al. Low prevalence of large intraventricular haemorrhage in very low birthweight infants carrying the factor V Leiden or prothrombin G20210A mutation. Acta Paediatr. 2001;90(9):1021-1024.
Gopel W., Kattner E., Seidenberg J., et al. The effect of the Val34Leu polymorphism in the factor XIII gene in infants with a birth weight below 1500 g. J Pediatr. 2002;140(6):688-692.
Gopel W., Hartel C., Ahrens P., et al. Interleukin-6-174-genotype, sepsis and cerebral injury in very low birth weight infants. Genes Immun. 2006;7(1):65-68.
Gould D.B., Phalan F.C., Breedveld G.J., et al. Mutations in Col4a1 cause perinatal cerebral hemorrhage and porencephaly. Science. 2005;308(5725):1167-1171.
Gould D.B., Phalan F.C., van Mil S.E., et al. Role of COL4A1 in small-vessel disease and hemorrhagic stroke. N Engl J Med. 2006;354(14):1489-1496.
Gould S.J., Howard S., Hope P.L., et al. Periventricular intraparenchymal cerebral haemorrhage in preterm infants: the role of venous infarction. J Pathol. 1987;151(3):197-202.
Greisen G., Andreasen R.B. Recombinant factor VIIa in preterm neonates with prolonged prothrombin time. Blood Coagul Fibrinolysis. 2003;14(1):117-120.
Greisen G., Munck H., Lou H. Severe hypocarbia in preterm infants and neurodevelopmental deficit. Acta Paediatr Scand. 1987;76(3):401-404.
Greisen G., Hellstrom-Westas L., Lou H., et al. EEG depression and germinal layer haemorrhage in the newborn. Acta Paediatr Scand. 1987;76(3):519-525.
Groenendaal F., Termote J.U., van der Heide-Jalving M., et al. Complications affecting preterm neonates from 1991 to 2006: what have we gained? Acta Paediatr. 2010;99(3):354-358.
Hack M., Taylor H.G., Schluchter M., et al. Behavioral outcomes of extremely low birth weight children at age 8 years. J Dev Behav Pediatr. 2009;30(2):122-130.
Hagmann C.F., De Vita E., Bainbridge A., et al. T2 at MR imaging is an objective quantitative measure of cerebral white matter signal intensity abnormality in preterm infants at term-equivalent age. Radiology. 2009;252(1):209-217.
Hall R.W., Kronsberg S.S., Barton B.A., et al. Morphine, hypotension, and adverse outcomes among preterm neonates: who’s to blame? Secondary results from the NEOPAIN trial. Pediatrics. 2005;115(5):1351-1359.
Hambleton G., Wigglesworth J.S. Origin of intraventricular haemorrhage in the preterm infant. Arch Dis Child. 1976;51(9):651-659.
Hammerman C., Glaser J., Schimmel M.S., et al. Continuous versus multiple rapid infusions of indomethacin: effects on cerebral blood flow velocity. Pediatrics. 1995;95(2):244-248.
Hamrick S.E., Miller S.P., Leonard C., et al. Trends in severe brain injury and neurodevelopmental outcome in premature newborn infants: the role of cystic periventricular leukomalacia. J Pediatr. 2004;145(5):593-599.
Hansen B.M., Hoff B., Uldall P., et al. Perinatal risk factors of adverse outcome in very preterm children: a role of initial treatment of respiratory insufficiency? Acta Paediatr. 2004;93(2):185-189.
Harding D., Brull D., Humphries S.E., et al. Variation in the interleukin-6 gene is associated with impaired cognitive development in children born prematurely: a preliminary study. Pediatr Res. 2005;58(1):117-120.
Harding D.R., Dhamrait S., Whitelaw A., et al. Does interleukin-6 genotype influence cerebral injury or developmental progress after preterm birth? Pediatrics. 2004;114(4):941-947.
Harding D.R., Humphries S.E., Whitelaw A., et al. Cognitive outcome and cyclo-oxygenase-2 gene (-765 G/C) variation in the preterm infant. Arch Dis Child Fetal Neonatal Ed. 2007;92(2):F108-F112.
Haynes R.L., Billiards S.S., Borenstein N.S., et al. Diffuse axonal injury in periventricular leukomalacia as determined by apoptotic marker fractin. Pediatr Res. 2008;63(6):656-661.
Heep A., Behrendt D., Nitsch P., et al. Increased serum levels of interleukin 6 are associated with severe intraventricular haemorrhage in extremely premature infants. Arch Dis Child Fetal Neonatal Ed. 2003;88(6):F501-F504.
Heep A., Schueller A.C., Kattner E., et al. Association of two tumour necrosis factor gene polymorphisms with the incidence of severe intraventricular haemorrhage in preterm infants. J Med Genet. 2005;42(7):604-608.
Hellstrom-Westas L., Rosen I. Electroencephalography and brain damage in preterm infants. Early Hum Dev. 2005;81(3):255-261.
Hill A., Volpe J.J. Seizures, hypoxic-ischemic brain injury, and intraventricular hemorrhage in the newborn. Ann Neurol. 1981;10(2):109-121.
Hill A., Perlman J.M., Volpe J.J. Relationship of pneumothorax to occurrence of intraventricular hemorrhage in the premature newborn. Pediatrics. 1982;69(2):144-149.
Hintz S.R., Kendrick D.E., Vohr B.R., et al. Gender differences in neurodevelopmental outcomes among extremely preterm, extremely-low-birthweight infants. Acta Paediatr. 2006;95(10):1239-1248.
Hoecker C., Nelle M., Poeschl J., et al. Caffeine impairs cerebral and intestinal blood flow velocity in preterm infants. Pediatrics. 2002;109(5):784-787.
Hohlagschwandtner M., Husslein P., Klebermass K., et al. Perinatal mortality and morbidity. Comparison between maternal transport, neonatal transport and inpatient antenatal treatment. Arch Gynecol Obstet. 2001;265(3):113-118.
Hope P.L., Gould S.J., Howard S., et al. Precision of ultrasound diagnosis of pathologically verified lesions in the brains of very preterm infants. Dev Med Child Neurol. 1988;30(4):457-471.
Huppi P.S., Schuknecht B., Boesch C., et al. Structural and neurobehavioral delay in postnatal brain development of preterm infants. Pediatr Res. 1996;39(5):895-901.
Huppi P.S., Warfield S., Kikinis R., et al. Quantitative magnetic resonance imaging of brain development in premature and mature newborns. Ann Neurol. 1998;43(2):224-235.
Huppi P.S., Murphy B., Maier S.E., et al. Microstructural brain development after perinatal cerebral white matter injury assessed by diffusion tensor magnetic resonance imaging. Pediatrics. 2001;107(3):455-460.
Husson I., Rangon C.M., Lelievre V., et al. BDNF-induced white matter neuroprotection and stage-dependent neuronal survival following a neonatal excitotoxic challenge. Cereb Cortex. 2005;15(3):250-261.
Huttenlocher P.R., Dabholkar A.S. Regional differences in synaptogenesis in human cerebral cortex. J Comp Neurol. 1997;387(2):167-178.
Huttenlocher P.R., de Courten C. The development of synapses in striate cortex of man. Hum Neurobiol. 1987;6(1):1-9.
Huttenlocher P.R., de Courten C., Garey L.J., et al. Synaptogenesis in human visual cortex–evidence for synapse elimination during normal development. Neurosci Lett. 1982;33(3):247-252.
Inder T.E., Huppi P.S., Warfield S., et al. Periventricular white matter injury in the premature infant is followed by reduced cerebral cortical gray matter volume at term. Ann Neurol. 1999;46(5):755-760.
Inder T.E., Anderson N.J., Spencer C., et al. White matter injury in the premature infant: a comparison between serial cranial sonographic and MR findings at term. Am J Neuroradiol. 2003;24(5):805-809.
Inder T.E., Wells S.J., Mogridge N.B., et al. Defining the nature of the cerebral abnormalities in the premature infant: a qualitative magnetic resonance imaging study. J Pediatr. 2003;143(2):171-179.
Inder T.E., Warfield S.K., Wang H., et al. Abnormal cerebral structure is present at term in premature infants. Pediatrics. 2005;115(2):286-294.
Jacobson L., Ek U., Fernell E., et al. Visual impairment in preterm children with periventricular leukomalacia-visual, cognitive and neuropaediatric characteristics related to cerebral imaging. Dev Med Child Neurol. 1996;38(8):724-735.
Jacobson L., Lundin S., Flodmark O., et al. Periventricular leukomalacia causes visual impairment in preterm children. A study on the aetiologies of visual impairment in a population-based group of preterm children born 1989–95 in the county of Varmland, Sweden. Acta Ophthalmol Scand. 1998;76(5):593-598.
Jacobson L., Ygge J., Flodmark O., et al. Visual and perceptual characteristics, ocular motility and strabismus in children with periventricular leukomalacia. Strabismus. 2002;10(2):179-183.
Jacobson L.K., Dutton G.N. Periventricular leukomalacia: an important cause of visual and ocular motility dysfunction in children. Surv Ophthalmol. 2000;45(1):1-13.
Johnson S., Hennessy E., Smith R., et al. Academic attainment and special educational needs in extremely preterm children at 11 years of age: the EPICure study. Arch Dis Child Fetal Neonatal Ed. 2009;94(4):F283-F289.
Johnson S., Hollis C., Kochhar P., et al. Psychiatric disorders in extremely preterm children: longitudinal finding at age 11 years in the EPICure study. J Am Acad Child Adolesc Psychiatry. 2010;49(5):453-463 e1.
Kaiser J.R., Gauss C.H., Pont M.M., et al. Hypercapnia during the first 3 days of life is associated with severe intraventricular hemorrhage in very low birth weight infants. J Perinatol. 2006;26(5):279-285.
Kapellou O., Counsell S.J., Kennea N., et al. Abnormal cortical development after premature birth shown by altered allometric scaling of brain growth. PLoS Med. 2006;3(8):e265.
Karadottir R., Attwell D. Neurotransmitter receptors in the life and death of oligodendrocytes. Neuroscience. 2007;145(4):1426-1438.
Kazan S., Gura A., Ucar T., et al. Hydrocephalus after intraventricular hemorrhage in preterm and low-birth weight infants: analysis of associated risk factors for ventriculoperitoneal shunting. Surg Neurol. 2005;64(Suppl 2):S77-S81. discussion S
Kesler S.R., Ment L.R., Vohr B., et al. Volumetric analysis of regional cerebral development in preterm children. Pediatr Neurol. 2004;31(5):318-325.
Kesler S.R., Reiss A.L., Vohr B., et al. Brain volume reductions within multiple cognitive systems in male preterm children at age twelve. J Pediatr. 2008;152(4):513-520. 20 e1
Kidokoro H., Okumura A., Hayakawa F., et al. Chronologic Changes in Neonatal EEG Findings in Periventricular Leukomalacia. Pediatrics. 2009.
Kluckow M. Low systemic blood flow and pathophysiology of the preterm transitional circulation. Early Hum Dev. 2005;81(5):429-437.
Komlosi K., Havasi V., Bene J., et al. Increased prevalence of factor V Leiden mutation in premature but not in full-term infants with grade I intracranial haemorrhage. Biol Neonate. 2005;87(1):56-59.
Kostovic I. Structural and histochemical reorganization of the human prefrontal cortex during perinatal and postnatal life. Prog Brain Res. 1990;85:223-239. discussion 39–40
Kostovic I., Rakic P. Developmental history of the transient subplate zone in the visual and somatosensory cortex of the macaque monkey and human brain. J Comp Neurol. 1990;297(3):441-470.
Kostovic I., Lukinovic N., Judas M., et al. Structural basis of the developmental plasticity in the human cerebral cortex: the role of the transient subplate zone. Metab Brain Dis. 1989;4(1):17-23.
Kostovic I., Judas M., Rados M., et al. Laminar organization of the human fetal cerebrum revealed by histochemical markers and magnetic resonance imaging. Cereb Cortex. 2002;12(5):536-544.
Krishnan M.L., Dyet L.E., Boardman J.P., et al. Relationship between white matter apparent diffusion coefficients in preterm infants at term-equivalent age and developmental outcome at 2 years. Pediatrics. 2007;120(3):e604-e609.
Kroeger K.M., Carville K.S., Abraham L.J. The -308 tumor necrosis factor-alpha promoter polymorphism effects transcription. Mol Immunol. 1997;34(5):391-399.
Kuban K., Adler I., Allred E.N., et al. Observer variability assessing US scans of the preterm brain: the ELGAN study. Pediatr Radiol. 2007;37(12):1201-1208.
Kuban K.C., Allred E.N., Dammann O., et al. Topography of cerebral white-matter disease of prematurity studied prospectively in 1607 very-low-birthweight infants. J Child Neurol. 2001;16(6):401-408.
Kuban K.C., Allred E.N., O’Shea M., et al. An Algorithm for Identifying and Classifying Cerebral Palsy in Young Children. J Pediatr. 2008;153(4):466-472.
Kuban K.C., Allred E.N., O’Shea T.M., et al. Cranial ultrasound lesions in the NICU predict cerebral palsy at age 2 years in children born at extremely low gestational age. J Child Neurol. 2009;24(1):63-72.
Kuint J., Barak M., Morag I., et al. Early treated hypotension and outcome in very low birth weight infants. Neonatology. 2009;95(4):311-316.
Kwong K.L., Wong Y.C., Fong C.M., et al. Magnetic resonance imaging in 122 children with spastic cerebral palsy. Pediatr Neurol. 2004;31(3):172-176.
Labattaglia M.P., Ihle B. Recombinant activated factor VII: current perspectives and Epworth experience. Heart Lung Circ. 2007;16(Suppl 3):S96-S101.
Larroche J.C. Post-haemorrhagic hydrocephalus in infancy. Anatomical study. Biol Neonate. 1972;20(3):287-299.
Larroque B., Ancel P.Y., Marret S., et al. Neurodevelopmental disabilities and special care of 5-year-old children born before 33 weeks of gestation (the EPIPAGE study): a longitudinal cohort study. Lancet. 2008;371(9615):813-820.
Laughon M., Bose C., Allred E., et al. Factors associated with treatment for hypotension in extremely low gestational age newborns during the first postnatal week. Pediatrics. 2007;119(2):273-280.
Leech R.W., Kohnen P. Subependymal and intraventricular hemorrhages in the newborn. Am J Pathol. 1974;77(3):465-475.
Leffler C.W., Busija D.W., Fletcher A.M., et al. Effects of indomethacin upon cerebral hemodynamics of newborn pigs. Pediatr Res. 1985;19(11):1160-1164.
Leffler C.W., Busija D.W., Beasley D.G., et al. Effects of indomethacin on cardiac output distribution in normal and asphyxiated piglets. Prostaglandins. 1986;31(2):183-190.
Lehnardt S., Lachance C., Patrizi S., et al. The toll-like receptor TLR4 is necessary for lipopolysaccharide-induced oligodendrocyte injury in the CNS. J Neurosci. 2002;22(7):2478-2486.
Leijser L.M., Steggerda S.J., de Bruine F.T., et al. Brain imaging findings in very preterm infants throughout the neonatal period: part II. Relation with perinatal clinical data. Early Hum Dev. 2009;85(2):111-115.
Levene M.I. Measurement of the growth of the lateral ventricles in preterm infants with real-time ultrasound. Arch Dis Child. 1981;56(12):900-904.
Leviton A., Dammann O., Allred E.N., et al. Antenatal corticosteroids and cranial ultrasonographic abnormalities. Am J Obstet Gynecol. 1999;181(4):1007-1017.
Leviton A., Paneth N., Reuss M.L., et al. Maternal infection, fetal inflammatory response, and brain damage in very low birth weight infants. Developmental Epidemiology Network Investigators. Pediatr Res. 1999;46(5):566-575.
Leviton A., Allred E.N., Kuban K.C., et al. Microbiologic and histologic characteristics of the extremely preterm infant’s placenta predict white matter damage and later cerebral palsy. the ELGAN study. Pediatr Res. 2010;67(1):95-101.
Li J., Baud O., Vartanian T., et al. Peroxynitrite generated by inducible nitric oxide synthase and NADPH oxidase mediates microglial toxicity to oligodendrocytes. Proc Natl Acad Sci USA. 2005;102(28):9936-9941.
Ligam P., Haynes R.L., Folkerth R.D., et al. Thalamic Damage in Periventricular Leukomalacia: Novel Pathologic Observations Relevant to Cognitive Deficits in Survivors of Prematurity. Pediatr Res. 2008.
Limperopoulos C., Benson C.B., Bassan H., et al. Cerebellar hemorrhage in the preterm infant: ultrasonographic findings and risk factors. Pediatrics. 2005;116(3):717-724.
Limperopoulos C., Bassan H., Gauvreau K., et al. Does cerebellar injury in premature infants contribute to the high prevalence of long-term cognitive, learning, and behavioral disability in survivors? Pediatrics. 2007;120(3):584-593.
Limperopoulos C., Gauvreau K.K., O’Leary H., et al. Cerebral hemodynamic changes during intensive care of preterm infants. Pediatrics. 2008;122(5):e1006-e1013.
Linder N., Haskin O., Levit O., et al. Risk factors for intraventricular hemorrhage in very low birth weight premature infants: a retrospective case-control study. Pediatrics. 2003;111(5 Pt 1):e590-e595.
Lodygensky G.A., Rademaker K., Zimine S., et al. Structural and functional brain development after hydrocortisone treatment for neonatal chronic lung disease. Pediatrics. 2005;116(1):1-7.
Lou H., Lassen N., Friis-Hansen B. Impaired autoregulation of cerebral blood flow in the distressed newborn infant. J Pediatr. 1979;94(1):118-121.
Lundstrom K., Pryds O., Greisen G. The haemodynamic effects of dopamine and volume expansion in sick preterm infants. Early Hum Dev. 2000;57(2):157-163.
Luu T.M., Ment L.R., Schneider K.C., et al. Lasting effects of preterm birth and neonatal brain hemorrhage at 12 years of age. Pediatrics. 2009;123(3):1037-1044.
Luu T.M., Vohr B.R., Schneider K.C., et al. Trajectories of receptive language development from 3 to 12 years of age for very preterm children. Pediatrics. 2009;124(1):333-341.
Maalouf E.F., Counsell S., Battin M., et al. Magnetic resonance imaging of the neonatal brain. Hosp Med. 1998;59(1):41-45.
Maalouf E.F., Duggan P.J., Rutherford M.A., et al. Magnetic resonance imaging of the brain in a cohort of extremely preterm infants. J Pediatr. 1999;135(3):351-357.
Maalouf E.F., Duggan P.J., Counsell S.J., et al. Comparison of findings on cranial ultrasound and magnetic resonance imaging in preterm infants. Pediatrics. 2001;107(4):719-727.
Macik B.G., Lindley C.M., Lusher J., et al. Safety and initial clinical efficacy of three dose levels of recombinant activated factor VII (rFVIIa): results of a phase I study. Blood Coagul Fibrinolysis. 1993;4(4):521-527.
Marlow N., Wolke D., Bracewell M.A., et al. Neurologic and developmental disability at six years of age after extremely preterm birth. N Engl J Med. 2005;352(1):9-19.
Marret S., Parain D., Jeannot E., et al. Positive rolandic sharp waves in the EEG of the premature newborn: a five year prospective study. Arch Dis Child. 1992;67(7):948-951.
McCormick D., Edwards A., Brown G., et al. Effect of indomethacin on cerebral oxidized cytochrome oxidase in preterm infants. Pediatr Res. 1993;33(6):603-608.
McCrea H.J., Ment L.R. The diagnosis, management, and postnatal prevention of intraventricular hemorrhage in the preterm neonate. Clin Perinatol. 2008;35(4):777-792. vii
McQuillen P.S., Sheldon R.A., Shatz C.J., et al. Selective vulnerability of subplate neurons after early neonatal hypoxia-ischemia. J Neurosci. 2003;23(8):3308-3315.
Ment L.R., Duncan C.C., Ehrenkranz R.A., et al. Intraventricular hemorrhage in the preterm neonate: timing and cerebral blood flow changes. J Pediatr. 1984;104(3):419-425.
Ment L.R., Oh W., Ehrenkranz R.A., et al. Risk period for intraventricular hemorrhage of the preterm neonate is independent of gestational age. Semin Perinatol. 1993;17(5):338-341.
Ment L.R., Oh W., Ehrenkranz R.A., et al. Low-dose indomethacin and prevention of intraventricular hemorrhage: a multicenter randomized trial [see comments]. Pediatrics. 1994;93(4):543-550.
Ment L.R., Bada H.S., Barnes P., et al. Practice parameter: neuroimaging of the neonate: report of the Quality Standards Subcommittee of the American Academy of Neurology and the Practice Committee of the Child Neurology Society. Neurology. 2002;58(12):1726-1738.
Mercuri E., Jongmans M., Henderson S., et al. Evaluation of the corpus callosum in clumsy children born prematurely: a functional and morphological study. Neuropediatrics. 1996;27(6):317-322.
Mewes A.U., Huppi P.S., Als H., et al. Regional brain development in serial magnetic resonance imaging of low-risk preterm infants. Pediatrics. 2006;118(1):23-33.
Miller S.P., Vigneron D.B., Henry R.G., et al. Serial quantitative diffusion tensor MRI of the premature brain: Development in newborns with and without injury. J Magn Reson Imaging. 2002;16(6):621-632.
Mitsiakos G., Papaioannou G., Giougi E., et al. Is the use of rFVIIa safe and effective in bleeding neonates? A retrospective series of 8 cases. J Pediatr Hematol Oncol. 2007;29(3):145-150.
Morgan M.E., Benson J.W., Cooke R.W. Ethamsylate reduces the incidence of periventricular haemorrhage in very low birth-weight babies. Lancet. 1981;2(8251):830-831.
Moylan F.M., Herrin J.T., Krishnamoorthy K., et al. Inappropriate antidiuretic hormone secretion in premature infants with cerebral injury. Am J Dis Child. 1978;132(4):399-402.
Murase M., Ishida A. Early hypocarbia of preterm infants: its relationship to periventricular leukomalacia and cerebral palsy, and its perinatal risk factors. Acta Paediatr. 2005;94(1):85-91.
Neubauer A.P., Voss W., Kattner E. Outcome of extremely low birth weight survivors at school age: the influence of perinatal parameters on neurodevelopment. Eur J Pediatr. 2008;167(1):87-95.
Nosarti C., Al-Asady M.H., Frangou S., et al. Adolescents who were born very preterm have decreased brain volumes. Brain. 2002;125(Pt 7):1616-1623.
Nosarti C., Giouroukou E., Healy E., et al. Grey and white matter distribution in very preterm adolescents mediates neurodevelopmental outcome. Brain. 2008;131(Pt 1):205-217.
Nosarti C., Shergill S.S., Allin M.P., et al. Neural substrates of letter fluency processing in young adults who were born very preterm: alterations in frontal and striatal regions. Neuroimage. 2009;47(4):1904-1913.
Novotny E.J.Jr, Tharp B.R., Coen R.W., et al. Positive rolandic sharp waves in the EEG of the premature infant. Neurology. 1987;37(9):1481-1486.
Obladen M., Metze B., Henrich W., et al. Interdisciplinary surveillance of intraventricular haemorrhage associated conditions in infants <1000 g. Acta Paediatr. 2008;97(6):731-737.
O’Donovan D.J., Fernandes C.J. Free radicals and diseases in premature infants. Antioxid Redox Signal. 2004;6(1):169-176.
Oka A., Belliveau M.J., Rosenberg P.A., et al. Vulnerability of oligodendroglia to glutamate: pharmacology, mechanisms, and prevention. J Neurosci. 1993;13(4):1441-1453.
O’Leary H., Gregas M.C., Limperopoulos C., et al. Elevated cerebral pressure passivity is associated with prematurity-related intracranial hemorrhage. Pediatrics. 2009;124(1):302-309.
Osborn D.A., Evans N. Early volume expansion for prevention of morbidity and mortality in very preterm infants. Cochrane Database Syst Rev. (2):2004. CD002055
O’Shea T.M., Kuban K.C., Allred E.N., et al. Neonatal cranial ultrasound lesions and developmental delays at 2 years of age among extremely low gestational age children. Pediatrics. 2008;122(3):e662-e669.
Pang Y., Campbell L., Zheng B., et al. Lipopolysaccharide-activated microglia induce death of oligodendrocyte progenitor cells and impede their development. Neuroscience. 2010;166(2):464-475.
Pape K.E., Wigglesworth J.S.. Haemorrhage, Ischemia, and the Perinatal Brain. Philadelphia: J.B. Lippincott Co.; 1979:61-84.
Papile L.A., Burstein J., Burstein R., et al. Incidence and evolution of subependymal and intraventricular hemorrhage: a study of infants with birth weights less than 1,500 gm. J Pediatr. 1978;92(4):529-534.
Patel J., Roberts I., Azzopardi D., et al. Randomized double-blind controlled trial comparing the effects of ibuprofen with indomethacin on cerebral hemodynamics in preterm infants with patent ductus arteriosus [see comments]. Pediatr Res. 2000;47(1):36-42.
Patra K., Wilson-Costello D., Taylor H.G., et al. Grades I-II intraventricular hemorrhage in extremely low birth weight infants: effects on neurodevelopment. J Pediatr. 2006;149(2):169-173.
Perlman J.M., Rollins N. Surveillance protocol for the detection of intracranial abnormalities in premature neonates. Arch Pediatr Adolesc Med. 2000;154(8):822-826.
Perlman J.M., Volpe J.J. Suctioning in the preterm infant: effects on cerebral blood flow velocity, intracranial pressure, and arterial blood pressure. Pediatrics. 1983;72(3):329-334.
Perlman J.M., Volpe J.J. Intraventricular hemorrhage in extremely small premature infants. Am J Dis Child. 1986;140(11):1122-1124.
Perlman J.M., McMenamin J.B., Volpe J.J. Fluctuating cerebral blood-flow velocity in respiratory-distress syndrome. Relation to the development of intraventricular hemorrhage. N Engl J Med. 1983;309(4):204-209.
Petaja J., Hiltunen L., Fellman V. Increased risk of intraventricular hemorrhage in preterm infants with thrombophilia. Pediatr Res. 2001;49(5):643-646.
Peterson B.S., Vohr B., Staib L.H., et al. Regional brain volume abnormalities and long-term cognitive outcome in preterm infants. JAMA. 2000;284(15):1939-1947.
Pierson C.R., Folkerth R.D., Billiards S.S., et al. Gray matter injury associated with periventricular leukomalacia in the premature infant. Acta Neuropathol. 2007;114(6):619-631.
Pike M.G., Holmstrom G., de Vries L.S., et al. Patterns of visual impairment associated with lesions of the preterm infant brain. Dev Med Child Neurol. 1994;36(10):849-862.
Pinto-Martin J.A., Whitaker A.H., Feldman J.F., et al. Relation of cranial ultrasound abnormalities in low-birthweight infants to motor or cognitive performance at ages 2, 6, and 9 years. Dev Med Child Neurol. 1999;41(12):826-833.
Ramenghi L.A., Fumagalli M., Righini A., et al. Magnetic resonance imaging assessment of brain maturation in preterm neonates with punctate white matter lesions. Neuroradiology. 2007;49(2):161-167.
Reiss A.L., Kesler S.R., Vohr B., et al. Sex differences in cerebral volumes of 8-year-olds born preterm. J Pediatr. 2004;145(2):242-249.
Resch B., Radinger A., Mannhalter C., et al. Interleukin-6 G(–174)C polymorphism is associated with mental retardation in cystic periventricular leucomalacia in preterm infants. Arch Dis Child Fetal Neonatal Ed. 2009;94(4):F304-F306.
Ribeiro M.L., Ogando D., Farina M., et al. Epidermal growth factor modulation of prostaglandins and nitrite biosynthesis in rat fetal membranes. Prostaglandins Leukot Essent Fatty Acids. 2004;70(1):33-40.
Ricci D., Anker S., Cowan F., et al. Thalamic atrophy in infants with PVL and cerebral visual impairment. Early Hum Dev. 2006.
Robertson C.M., Howarth T.M., Bork D.L., et al. Permanent bilateral sensory and neural hearing loss of children after neonatal intensive care because of extreme prematurity: a thirty-year study. Pediatrics. 2009;123(5):e797-e807.
Robertson J.D. Prevention of intraventricular haemorrhage: a role for recombinant activated factor VII? J Paediatr Child Health. 2006;42(6):325-331.
Robinson S., Li Q., Dechant A., et al. Neonatal loss of gamma-aminobutyric acid pathway expression after human perinatal brain injury. J Neurosurg. 2006;104(6 Suppl):396-408.
Roelants-van Rijn A.M., Groenendaal F., Beek F.J., et al. Parenchymal brain injury in the preterm infant: comparison of cranial ultrasound, MRI and neurodevelopmental outcome. Neuropediatrics. 2001;32(2):80-89.
Rorke L.B.. Pathology of perinatal brain injury. New York: Raven Press; 1982:146.
Rosenberg P.A., Dai W., Gan X.D., et al. Mature myelin basic protein-expressing oligodendrocytes are insensitive to kainate toxicity. J Neurosci Res. 2003;71(2):237-245.
Rutherford M.A., Supramaniam V., Ederies A., et al. Magnetic resonance imaging of white matter diseases of prematurity. Neuroradiology. 2010;52(6):505-521.
Saigal S., Doyle L.W. An overview of mortality and sequelae of preterm birth from infancy to adulthood. Lancet. 2008;371(9608):261-269.
Saigal S., den Ouden L., Wolke D., et al. School-age outcomes in children who were extremely low birth weight from four international population-based cohorts. Pediatrics. 2003;112(4):943-950.
Sarkar S., Bhagat I., Dechert R., et al. Severe intraventricular hemorrhage in preterm infants: comparison of risk factors and short-term neonatal morbidities between grade 3 and grade 4 intraventricular hemorrhage. Am J Perinatol. 2009;26(6):419-424.
Savman K., Nilsson U.A., Blennow M., et al. Non-protein-bound iron is elevated in cerebrospinal fluid from preterm infants with posthemorrhagic ventricular dilatation. Pediatr Res. 2001;49(2):208-212.
Schmidt B., Davis P., Moddemann D., et al. Long-term effects of indomethacin prophylaxis in extremely-low-birth-weight infants. N Engl J Med. 2001;344(26):1966-1972.
Sehgal A., El-Naggar W., Glanc P., et al. Risk factors and ultrasonographic profile of posterior fossa haemorrhages in preterm infants. J Paediatr Child Health. 2009;45(4):215-218.
Shah D.K., Doyle L.W., Anderson P.J., et al. Adverse neurodevelopment in preterm infants with postnatal sepsis or necrotizing enterocolitis is mediated by white matter abnormalities on magnetic resonance imaging at term. J Pediatr. 2008;153(2):170-175. 5 e1
Shah D.K., Zempel J., Barton T., et al. Electrographic seizures in preterm infants during the first week of life are associated with cerebral injury. Pediatr Res. 2010;67(1):102-106.
Shalak L., Perlman J.M. Hemorrhagic-ischemic cerebral injury in the preterm infant: current concepts. Clin Perinatol. 2002;29(4):745-763.
Shankaran S., Papile L.-A., Wright L.L., et al. Does antenatal phenobarbital prevent neonatal intracranial hemorrhage in preterm infants?: randomized controlled trial (abstr). Pediatr Res. 1996;39:245A.
Shaver D.C., Bada H.S., Korones S.B., et al. Early and late intraventricular hemorrhage: the role of obstetric factors. Obstet Gynecol. 1992;80(5):831-837.
Sherlock R.L., Anderson P.J., Doyle L.W. Neurodevelopmental sequelae of intraventricular haemorrhage at 8 years of age in a regional cohort of ELBW/very preterm infants. Early Hum Dev. 2005;81(11):909-916.
Sherlock R.L., Synnes A.R., Grunau R.E., et al. Long-term outcome after neonatal intraparenchymal echodensities with porencephaly. Arch Dis Child Fetal Neonatal Ed. 2008;93(2):F127-F131.
Shooman D., Portess H., Sparrow O. A review of the current treatment methods for posthaemorrhagic hydrocephalus of infants. Cerebrospinal Fluid Res. 2009;6:1.
Short E.J., Klein N.K., Lewis B.A., et al. Cognitive and academic consequences of bronchopulmonary dysplasia and very low birth weight: 8-year-old outcomes. Pediatrics. 2003;112(5):e359.
Soraisham A.S., Singhal N., McMillan D.D., et al. A multicenter study on the clinical outcome of chorioamnionitis in preterm infants. Am J Obstet Gynecol. 2009;200(4):372. e1–e6
Soul J.S., Hammer P.E., Tsuji M., et al. Fluctuating pressure-passivity is common in the cerebral circulation of sick premature infants. Pediatr Res. 2007;61(4):467-473.
Steggerda S.J., Leijser L.M., Wiggers-de Bruine F.T., et al. Cerebellar injury in preterm infants: incidence and findings on US and MR images. Radiology. 2009;252(1):190-199.
Strunk T., Hartel C., Schultz C. Does erythropoietin protect the preterm brain? Arch Dis Child Fetal Neonatal Ed. 2004;89(4):F364-F366.
Synnes A.R., Chien L.Y., Peliowski A., et al. Variations in intraventricular hemorrhage incidence rates among Canadian neonatal intensive care units. J Pediatr. 2001;138(4):525-531.
Synnes A.R., Macnab Y.C., Qiu Z., et al. Neonatal intensive care unit characteristics affect the incidence of severe intraventricular hemorrhage. Med Care. 2006;44(8):754-759.
Tahraoui S.L., Marret S., Bodenant C., et al. Central role of microglia in neonatal excitotoxic lesions of the murine periventricular white matter. Brain Pathol. 2001;11(1):56-71.
Takashima S., Tanaka K. Development of cerebrovascular architecture and its relationship to periventricular leukomalacia. Arch Neurol. 1978;35(1):11-16.
Takashima S., Mito T., Ando Y. Pathogenesis of periventricular white matter hemorrhages in preterm infants. Brain Dev. 1986;8(1):25-30.
Takashima S., Mito T., Houdou S., et al. Relationship between periventricular hemorrhage, leukomalacia and brainstem lesions in prematurely born infants. Brain Dev. 1989;11(2):121-124.
Takashima S., Itoh M., Oka A. A history of our understanding of cerebral vascular development and pathogenesis of perinatal brain damage over the past 30 years. Semin Pediatr Neurol. 2009;16(4):226-236.
Tan M., Abernethy L., Cooke R. Improving head growth in preterm infants–a randomised controlled trial II: MRI and developmental outcomes in the first year. Arch Dis Child Fetal Neonatal Ed. 2008;93(5):F342-F346.
Taylor G.A. Effect of germinal matrix hemorrhage on terminal vein position and patency. Pediatr Radiol. 1995;25(Suppl 1):S37-S40.
Taylor H.G., Minich N.M., Klein N., et al. Longitudinal outcomes of very low birth weight: neuropsychological findings. J Int Neuropsychol Soc. 2004;10(2):149-163.
The EC Ethamsylate Trial Group. The EC randomised controlled trial of prophylactic ethamsylate for very preterm neonates: early mortality and morbidity. Arch Dis Child Fetal Neonatal Ed. 1994;70(3):F201-F205.
Tioseco J.A., Aly H., Essers J., et al. Male sex and intraventricular hemorrhage. Pediatr Crit Care Med. 2006;7(1):40-44.
Tsuji M., Saul J.P., du Plessis A., et al. Cerebral intravascular oxygenation correlates with mean arterial pressure in critically ill premature infants. Pediatrics. 2000;106(4):625-632.
van Alfen-van der Velden A.A., Hopman J.C., Klaessens J.H., et al. Effects of rapid versus slow infusion of sodium bicarbonate on cerebral hemodynamics and oxygenation in preterm infants. Biol Neonate. 2006;90(2):122-127.
van Bel F., Klautz R.J., Steendijk P., et al. The influence of indomethacin on the autoregulatory ability of the cerebral vascular bed in the newborn lamb. Pediatr Res. 1993;34(2):178-181.
Van Marter L.J., Dammann O., Allred E.N., et al. Chorioamnionitis, mechanical ventilation, and postnatal sepsis as modulators of chronic lung disease in preterm infants. J Pediatr. 2002;140(2):171-176.
Vasileiadis G.T., Gelman N., Han V.K., et al. Uncomplicated intraventricular hemorrhage is followed by reduced cortical volume at near-term age. Pediatrics. 2004;114(3):e367-e372.
Vinazzer H. Clinical and experimental studies on the action of ethamsylate on haemostasis and on platelet functions. Thromb Res. 1980;19(6):783-791.
Vohr B.R., Allan W.C., Westerveld M., et al. School-age outcomes of very low birth weight infants in the indomethacin intraventricular hemorrhage prevention trial. Pediatrics. 2003;111(4 Pt 1):e340-e346.
Vollmer B., Roth S., Riley K., et al. Neurodevelopmental outcome of preterm infants with ventricular dilatation with and without associated haemorrhage. Dev Med Child Neurol. 2006;48(5):348-352.
Volpe J.J. Brain injury in the premature infant. Neuropathology, clinical aspects, pathogenesis, and prevention. Clin Perinatol. 1997;24(3):567-587.
Volpe J.J. Perinatal brain injury: from pathogenesis to neuroprotection. Ment Retard Dev Disabil Res Rev. 2001;7(1):56-64.
Volpe J.J. Cerebral white matter injury of the premature infant-more common than you think. Pediatrics. 2003;112(1 Pt 1):176-180.
Volpe J.J. Encephalopathy of prematurity includes neuronal abnormalities. Pediatrics. 2005;116(1):221-225.
Volpe J.J. Brain injury in premature infants: a complex amalgam of destructive and developmental disturbances. Lancet Neurol. 2009;8(1):110-124.
Volpe J.J. Electroencephalography May Provide Insight Into Timing of Premature Brain Injury. Pediatrics. 2009.
Volpe J.J., Herscovitch P., Perlman J.M., et al. Positron emission tomography in the newborn: extensive impairment of regional cerebral blood flow with intraventricular hemorrhage and hemorrhagic intracerebral involvement. Pediatrics. 1983;72(5):589-601.
Voss W., Neubauer A.P., Wachtendorf M., et al. Neurodevelopmental outcome in extremely low birth weight infants: what is the minimum age for reliable developmental prognosis? Acta Paediatr. 2007;96(3):342-347.
Watterberg K.L., Shaffer M.L., Mishefske M.J., et al. Growth and neurodevelopmental outcomes after early low-dose hydrocortisone treatment in extremely low birth weight infants. Pediatrics. 2007;120(1):40-48.
Wei W., Xin-Ya S., Cai-Dong L., et al. Relationship between extracellular matrix both in choroid plexus and the wall of lateral ventricles and intraventricular hemorrhage in preterm neonates. Clin Anat. 2000;13(6):422-428.
Weiss H., Cooper B., Brook M., et al. Factors determining reopening of the ductus arteriosus after successful clinical closure with indomethacin. J Pediatr. 1995;127(3):466-471.
Whitelaw A. Intraventricular haemorrhage and posthaemorrhagic hydrocephalus: pathogenesis, prevention and future interventions. Semin Neonatol. 2001;6(2):135-146.
Whitelaw A. Intraventricular streptokinase after intraventricular hemorrhage in newborn infants. Cochrane Database Syst Rev. (2):2000. CD000498
Whitelaw A. Repeated lumbar or ventricular punctures for preventing disability or shunt dependence in newborn infants with intraventricular hemorrhage. Cochrane Database Syst Rev. (2):2000. CD000216
Whitelaw A., Odd D.E. Intraventricular streptokinase after intraventricular hemorrhage in newborn infants. Cochrane Database Syst Rev. (4):2007. CD000498
Whitelaw A., Kennedy C.R., Brion L.P. Diuretic therapy for newborn infants with posthemorrhagic ventricular dilatation. Cochrane Database Syst Rev. (2):2001. CD002270
Whitelaw A., Cherian S., Thoresen M., et al. Posthaemorrhagic ventricular dilatation: new mechanisms and new treatment. Acta Paediatr Suppl. 2004;93(444):11-14.
Whitelaw A., Evans D., Carter M., et al. Randomized clinical trial of prevention of hydrocephalus after intraventricular hemorrhage in preterm infants: brain-washing versus tapping fluid. Pediatrics. 2007;119(5):e1071-e1078.
Wood N.S., Marlow N., Costeloe K., et al. Neurologic and developmental disability after extremely preterm birth. EPICure Study Group. N Engl J Med. 2000;343(6):378-384.
Wood N.S., Costeloe K., Gibson A.T., et al. The EPICure study: associations and antecedents of neurological and developmental disability at 30 months of age following extremely preterm birth. Arch Dis Child Fetal Neonatal Ed. 2005;90(2):F134-F140.
Woodward L.J., Anderson P.J., Austin N.C., et al. Neonatal MRI to Predict Neurodevelopmental Outcomes in Preterm Infants. N Engl J Med. 2006;355(7):685-694.
Wu Y.W., Colford J.M. Chorioamnionitis as a risk factor for cerebral palsy: A meta-analysis. JAMA. 2000;284(11):1417-1424.
Xu H., Hu F., Sado Y., et al. Maturational changes in laminin, fibronectin, collagen IV, and perlecan in germinal matrix, cortex, and white matter and effect of betamethasone. J Neurosci Res. 2008;86(7):1482-1500.
Yancopoulos G.D., Davis S., Gale N.W., et al. Vascular-specific growth factors and blood vessel formation. Nature. 2000;407(6801):242-248.
Yanowitz T.D., Baker R.W., Sobchak Brozanski B. Prophylactic indomethacin reduces grades III and IV intraventricular hemorrhages when compared to early indomethacin treatment of a patent ductus arteriosus. J Perinatol. 2003;23(4):317-322.
Yoo S.S., Park H.J., Soul J.S., et al. In vivo visualization of white matter fiber tracts of preterm- and term-infant brains with diffusion tensor magnetic resonance imaging. Invest Radiol. 2005;40(2):110-115.
Yoon B.H., Romero R., Yang S.H., et al. Interleukin-6 concentrations in umbilical cord plasma are elevated in neonates with white matter lesions associated with periventricular leukomalacia. Am J Obstet Gynecol. 1996;174(5):1433-1440.
Zeitlin J., Ancel P.Y., Delmas D., et al. Changes in care and outcome of very preterm babies in the Parisian region between 1998 and 2003. Arch Dis Child Fetal Neonatal Ed. 2009.
Zia M.T., Csiszar A., Labinskyy N., et al. Oxidative-nitrosative stress in a rabbit pup model of germinal matrix hemorrhage: role of NAD(P)H oxidase. Stroke. 2009;40(6):2191-2198.