Formation of Germ Layers and Early Derivatives
As it is implanting into the uterine wall, the embryo undergoes profound changes in its organization. Up to the time of implantation, the blastocyst consists of the inner cell mass, from which the body of the embryo proper arises, and the outer trophoblast, which represents the future tissue interface between the embryo and mother. Both components of the blastocyst serve as the precursors of other tissues that appear in subsequent stages of development. Chapter 3 discusses the way in which the cytotrophoblast gives rise to an outer syncytial layer, the syncytiotrophoblast, shortly before attaching to uterine tissue (see Fig. 3.18). Not long thereafter, the inner cell mass begins to give rise to other tissue derivatives as well. The subdivision of the inner cell mass ultimately results in an embryonic body that contains the three primary embryonic germ layers: the ectoderm (outer layer), mesoderm (middle layer), and endoderm (inner layer). The process by which the germ layers are formed through cell movements is called gastrulation.
Two-Germ-Layer Stage
Just before the embryo implants into the endometrium early in the second week, significant changes begin to occur in the inner cell mass and in the trophoblast. As the cells of the inner cell mass become rearranged into an epithelial configuration, sometimes referred to as the embryonic shield, a thin layer of cells appears ventral to the main cellular mass (see Fig. 3.18). The main upper layer of cells is known as the epiblast, and the lower layer is called the hypoblast, or primitive endoderm (Fig. 5.1).
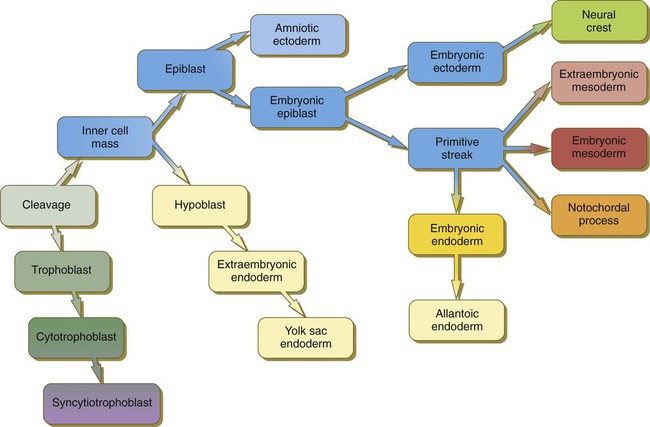
(Note: The colors in the boxes are found in all illustrations involving the embryonic and extraembryonic germ layers.)
How the hypoblast forms in human embryos is not understood, but studies on mouse embryos have shown that as early as the 64-cell stage, some cells of the inner cell mass express the transcription factor nanog, whereas others express Gata 6. These cells are arranged in a salt and pepper pattern within the inner cell mass (Fig. 5.2A). The nanog-expressing cells represent the precursors of the epiblast, and those expressing Gata 6 will become the hypoblast. The basis for the differentiation of these two distinct precursor cell types is not completely understood, but according to the “time inside–time outside” hypothesis, those cells that enter the inner cell mass earliest are biased to express nanog, which perpetuates their pluripotency. Possibly because of the influence of fibroblast growth factor-4 (FGF-4), secreted by these first arrivals to the inner cell mass, later immigrants are then biased to express Gata 6. The Gata 6–expressing cells produce molecules that increase their adhesive properties, as well as their mobility, and they make their way to the lower surface of the inner cell mass to form a thin epithelium, the hypoblast. Those Gata 6 cells that fail to reach the surface of the inner cell mass undergo apoptosis (cell death). The nanog-expressing cells of the inner cell mass also assume an epithelial configuration as they form the epiblast. Between the epiblast and hypoblast a basal lamina forms.
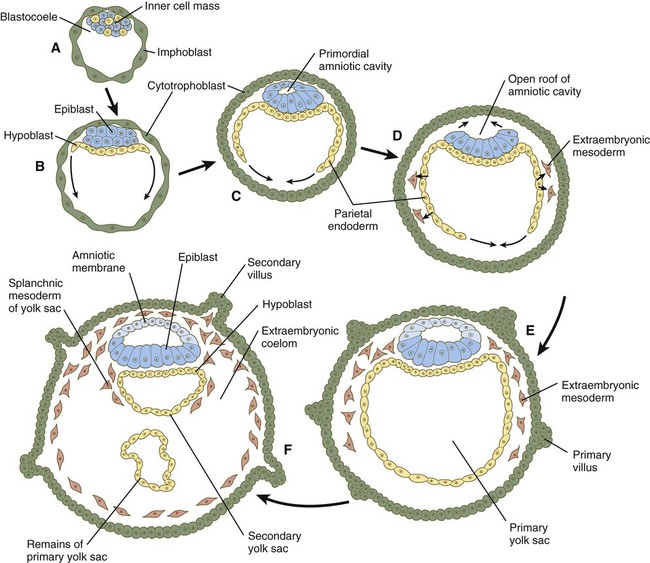
The syncytiotrophoblast is not shown. A, Late blastocyst. Within the inner cell mass, blue nanog-expressing pre-epiblastic cells and yellow Gata 6–expressing prehypoblastic cells are mixed in a salt and pepper pattern. B, Beginning of implantation at 6 days. The hypoblast has formed and is beginning to spread beneath the cytotrophoblast as the parietal endoderm. C, Implanted blastocyst at

A small group of hypoblast cells that becomes translocated to the future anterior end of the embryo (called anterior visceral endoderm by mouse embryologists) has been shown to possess remarkable signaling powers. The cells first secrete the signaling molecules, lefty-1 and Cerberus-1 (Cer-1), which inhibit the activity of the signaling molecules, nodal and Wnt, in the overlying epiblast but allow nodal and Wnt-3 expression in the posterior epiblast (see Fig. 5.8A). (Nodal signaling from the posterior epiblast stimulates the initial formation of the anterior visceral endoderm.) This represents the first clear expression of anteroposterior polarity in the embryo. It also forms two signaling domains within the early embryo. The anterior visceral endoderm soon begins to induce much of the head and forebrain and inhibits the formation of posterior structures. In the posterior part of the epiblast, nodal signaling activity stimulates the formation of the primitive streak (see next section), which is the focal point for gastrulation and germ layer formation. After the hypoblast has become a well-defined layer, and the epiblast has taken on an epithelial configuration, the former inner cell mass is transformed into a bilaminar disk, with the epiblast on the dorsal surface and the hypoblast on the ventral surface.
The epiblast contains the cells that make up the embryo itself, but extraembryonic tissues also arise from this layer. The next layer to appear after the hypoblast is the amnion, a layer of extraembryonic ectoderm that ultimately encloses the entire embryo in a fluid-filled chamber called the amniotic cavity (see Chapter 7). Because of the paucity of specimens, the earliest stages in the formation of the human amnion and amniotic cavity are not completely understood. Studies on primate embryos indicate that a primordial amniotic cavity first arises by cavitation (formation of an internal space) within the pre-epithelial epiblast; it is covered by cells derived from the inner cell mass (see Fig. 5.2). According to some investigators, the roof of the amnion then opens, thus exposing the primordial amniotic cavity to the overlying cytotrophoblast. Soon thereafter (by about 8 days after fertilization), the original amniotic epithelium reforms a solid roof over the amniotic cavity.
While the early embryo is still sinking into the endometrium (about 9 days after fertilization), cells of the hypoblast begin to spread and line the inner surface of the cytotrophoblast with a continuous layer of extraembryonic endoderm called parietal endoderm (Fig. 5.3; see Fig. 5.2). When the endodermal spreading is completed, a vesicle called the primary yolk sac has taken shape (see Fig. 3.18C). At this point (about 10 days after fertilization), the embryo complex constitutes the bilaminar germ disk, which is located between the primary yolk sac on its ventral surface and the amniotic cavity on its dorsal surface (Fig. 5.4). Shortly after it forms, the primary yolk sac becomes constricted, forming a secondary yolk sac and leaving behind a remnant of the primary yolk sac (see Figs. 3.18D and 5.2F).
Starting at about 12 days after fertilization, another extraembryonic tissue, the extraembryonic mesoderm, begins to appear (see Fig. 5.2). The first extraembryonic mesodermal cells seem to arise from a transformation of parietal endodermal cells. These cells are later joined by extraembryonic mesodermal cells that have originated from the primitive streak. The extraembryonic mesoderm becomes the tissue that supports the epithelium of the amnion and yolk sac and the chorionic villi, which arise from the trophoblastic tissues (see Chapter 7). The support supplied by the extraembryonic mesoderm is not only mechanical, but also trophic because the mesoderm serves as the substrate through which the blood vessels supply oxygen and nutrients to the various epithelia.
Gastrulation and the Three Embryonic Germ Layers
At the end of the second week, the embryo consists of two flat layers of cells: the epiblast and the hypoblast. As the third week of pregnancy begins, the embryo enters the period of gastrulation, during which the three embryonic germ layers form from the epiblast (see Fig. 5.1). The morphology of human gastrulation follows the pattern seen in birds. Because of the large amount of yolk in birds’ eggs, the avian embryo forms the primary germ layers as three overlapping flat disks that rest on the yolk, similar to a stack of pancakes. Only later do the germ layers fold to form a cylindrical body. Although the mammalian egg is essentially devoid of yolk, the morphological conservatism of early development still constrains the human embryo to follow a pattern of gastrulation similar to that seen in reptiles and birds. Because of the scarcity of material, even the morphology of gastrulation in human embryos is not known in detail. Nevertheless, extrapolation from avian and mammalian gastrulation can provide a reasonable working model of human gastrulation.
Gastrulation begins with the formation of the primitive streak, a linear midline condensation of cells derived from the epiblast in the posterior region of the embryo through an induction by cells at the edge of the embryonic disk in that region (see Fig. 5.4). Members of the transforming growth factor-β (TGF-β) and Wnt families of signaling molecules have been identified as likely inducing agents. Initially triangular, the primitive streak soon becomes linear and elongates, largely through a combination of proliferation and migration, as well as internal cellular rearrangements, called convergent-extension movements. With the appearance of the primitive streak, the anteroposterior (craniocaudal) and right-left axes of the embryo can be readily identified (see Fig. 5.4).
The primitive streak is a region where cells of the epiblast converge in a well-defined spatial and temporal sequence. As cells of the epiblast reach the primitive streak, they change shape and pass through it on their way to forming new layers beneath (ventral to) the epiblast (Fig. 5.5C). Marking studies have shown that cells entering the primitive streak form distinct lineages as they leave. The most posterior cells both to enter and leave the streak as it is beginning to elongate form the extraembryonic mesoderm lining the trophoblast and yolk sac, as well as that forming the blood islands (see Fig. 6.19). Another wave of mesoderm, arising later and more anteriorly in the primitive streak, forms the paraxial, lateral plate, and cardiac mesoderm. A final wave, which enters and leaves the anteriormost end of the primitive streak, gives rise to midline axial structures (the notochord, the prechordal plate, and the primitive node itself) and also the embryonic endoderm. The composite results of such marking experiments are organized into fate maps, such as that illustrated in Figure 5.5A.
The endodermal precursor cells that pass through the anterior primitive streak largely displace the original hypoblast, but research has shown that some of the original hypoblastic cells become integrated into the newly forming embryonic endodermal layer. The displaced hypoblastic cells form extraembryonic endoderm. The movement of cells through the primitive streak results in the formation of a groove (primitive groove) along the midline of the primitive streak. At the anterior end of the primitive streak is a small but well-defined accumulation of cells, called primitive node, or Hensen’s node.* This structure is of great developmental significance because, in addition to being the major posterior signaling center of the embryo (Box 5.1), it is the area through which cells migrate in a stream toward the anterior end of the embryo. These cells, called mesendoderm, soon segregate into a rodlike mesodermal notochord and the endodermal dorsal wall of the forming gut. Anterior to the notochord is a group of mesodermal cells called the prechordal plate (see Fig. 5.5A and B). (The important functions of the notochord and prechordal plate are discussed on p. 80.)
The specific craniocaudal characteristics of the structures arising from the newly formed paraxial mesoderm are specified by patterns of Hox gene expression, first in the epiblast and then in the mesodermal cells themselves. The transformations of morphology and the behavior of the cells passing through the primitive streak are associated with profound changes not only in their adhesive properties and internal organization, but also in the way that they relate to their external environment. Much of the extraembryonic mesoderm forms the body stalk, which connects the caudal part of the embryo to the extraembryonic tissues that surround it (see Figs. 5.4 and 7.1). The body stalk later becomes the umbilical cord.
The movements of the cells passing through the primitive streak are accompanied by major changes in their structure and organization (Fig. 5.6). While in the epiblast, the cells have the properties of typical epithelial cells, with well-defined apical and basal surfaces, and they are associated with a basal lamina that underlies the epiblast. As they enter the primitive streak, these cells elongate, lose their basal lamina, and take on a characteristic morphology that has led to their being called bottle cells. When they become free of the epiblastic layer in the primitive groove, the bottle cells assume the morphology and characteristics of mesenchymal cells, which are able to migrate as individual cells if they are provided with the proper extracellular environment (see Fig. 5.6). Included in this transformation is the loss of specific cell adhesion molecules (CAMs), in particular E-cadherin (see p. 254) as the cells convert from an epithelial to a mesenchymal configuration. This transformation is correlated with the expression of the transcription factor snail, which is also active in the separation of mesenchymal neural crest cells from the epithelial neural tube (see p. 254). As cells in the epiblast are undergoing epithelial-mesenchymal transition, they begin to express the CAM N-cadherin, which is necessary for their spreading out from the primitive streak in the newly forming mesodermal layer.
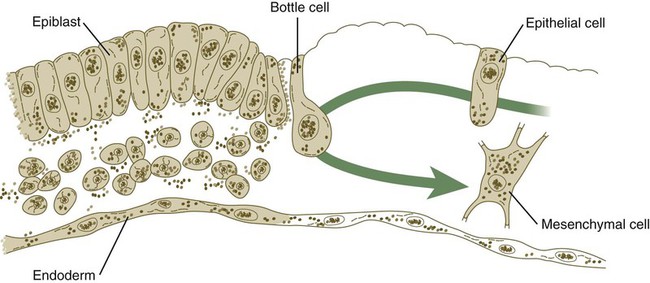
Changes in the shape of a cell as it migrates along the epiblast (epithelium), through the primitive streak (bottle cell), and away from the groove as a mesenchymal cell that will become part of the mesodermal germ layer. The same cell can later assume an epithelial configuration as part of a somite.
Starting in early gastrulation, cells of the epiblast produce hyaluronic acid, which enters the space between the epiblast and hypoblast. Hyaluronic acid, a polymer consisting of repeating subunits of D-glucuronic acid and N-acetylglucosamine, is frequently associated with cell migration in developing systems. The molecule has a tremendous capacity to bind water (up to 1000 times its own volume), and it functions to keep mesenchymal cells from aggregating during cell migrations. Although after leaving the primitive streak the mesenchymal cells of the embryonic mesoderm find themselves in a hyaluronic acid–rich environment, hyaluronic acid alone is not enough to support their migration from the primitive streak. In all vertebrate embryos that have been investigated to date, the spread of mesodermal cells away from the primitive streak or the equivalent structure is found to depend on the presence of fibronectin associated with the basal lamina beneath the epiblast. The embryonic mesoderm ultimately spreads laterally as a thin sheet of mesenchymal cells between the epiblast and hypoblast layers (see Fig. 5.5C).
Regression of the Primitive Streak
After its initial appearance at the extreme caudal end of the embryo, the primitive streak expands cranially until about 18 days after fertilization (see Fig. 5.4). Thereafter, it regresses caudally (see Fig. 5.11) and strings out the notochord in its wake. Vestiges remain into the fourth week. During that time, the formation of mesoderm continues by means of cells migrating from the epiblast through the primitive groove. Regression of the primitive streak is accompanied by the establishment and patterning of the paraxial mesoderm (see p. 97), which gives rise to the somites and ultimately the segmental axial structures of the trunk and caudal regions of the body. As regression of the primitive streak comes to a close, its most caudal extent is marked by a mass of mesenchymal cells, which form the tail bud. This structure plays an important role in forming the most posterior portion of the neural tube (see p. 93).
The primitive streak normally disappears without a trace, but in rare instances, large tumors called teratomas appear in the sacrococcygeal region (see Fig. 1.2A). Teratomas often contain bizarre mixtures of many different types of tissue, such as cartilage, muscle, fat, hair, and glandular tissue. Because of this, sacrococcygeal teratomas are thought to arise from remains of the primitive streak (which can form all germ layers). Teratomas also are found in the gonads and the mediastinum. These tumors are thought to originate from germ cells.
Notochord and Prechordal Plate
Cranial to the notochord is a small region where embryonic ectoderm and endoderm abut without any intervening mesoderm. Called the oropharyngeal membrane (see Fig. 5.5), this structure marks the site of the future oral cavity. Between the cranial tip of the notochordal process and the oropharyngeal membrane is a small aggregation of mesodermal cells closely apposed to endoderm, called the prechordal plate (see Fig. 5.5). In birds, the prechordal plate emits molecular signals that are instrumental in stimulating the formation of the forebrain, similar to the anterior visceral endoderm in mammals.
Both the prechordal plate and the notochord arise from the ingression of a population of epiblastic cells, which join other cells of primitive streak origin, within the primitive node. As the primitive streak regresses, the cellular precursors of first the prechordal plate and then the notochord migrate rostrally from the node, but they are left behind as a rodlike aggregation of cells (notochordal process; see Fig. 5.5A and B) in the wake of the regressing primitive streak. In mammals, shortly after ingression, the cells of the notochordal process temporarily spread out and fuse with the embryonic endoderm (Fig. 5.7). The result is the formation of a transitory neurenteric canal that connects the emerging amniotic cavity with the yolk sac. Later, the cells of the notochord separate from the endodermal roof of the yolk sac and form the definitive notochord, a solid rod of cells in the midline between the embryonic ectoderm and endoderm (see Fig. 5.7).
Induction of the Nervous System
Neural Induction
Deletion and transplantation experiments in amphibians set the stage for the present understanding of neural induction. (See Chapters 6 and 11 for further details on the formation of the nervous system.) In the absence of chordamesoderm moving from the dorsal lip of the blastopore (the amphibian equivalent of the primitive node), the nervous system does not form from the dorsal ectoderm. In contrast, if the dorsal lip of the blastopore is grafted beneath the belly ectoderm of another host, a secondary nervous system and body axis form in the area of the graft (Fig. 5.10).
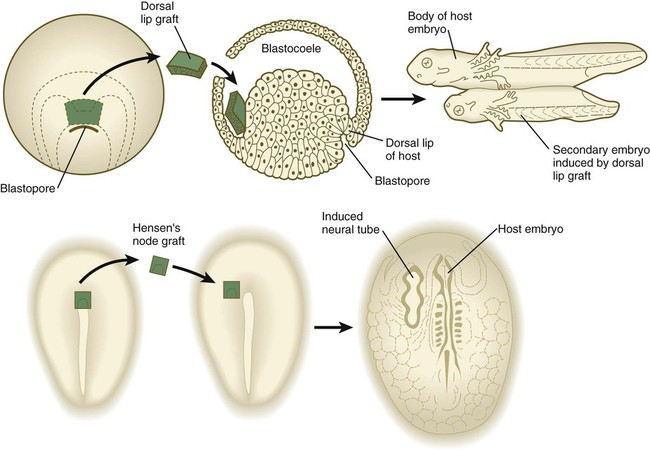
Top, Graft of the dorsal lip of the blastopore in a salamander embryo induces a secondary embryo to form. Bottom, Graft of Hensen’s node from one avian embryo to another induces the formation of a secondary neural tube. (Top based on studies by Spemann H: Embryonic development and induction, New York, 1938, Hafner; Bottom based on studies by Waddington C: J Exp Biol 10:38-46, 1933.)
The dorsal lip has been called the organizer because of its ability to stimulate the formation of a secondary body axis. Subsequent research has shown that the interactions occurring in the region of the dorsal lip in amphibians are far more complex than a single induction between chordamesoderm and ectoderm. Deletion and transplantation experiments have also been conducted on embryos of birds and mammals (see Fig. 5.10); clearly, the primitive node and the notochordal process in birds and mammals are homologous in function to the dorsal lip and chordamesoderm in amphibians. This means that, in higher vertebrates, the primitive node and the notochordal process act as the neural inductor, and the overlying ectoderm is the responding tissue. Over the years, embryologists have devoted an enormous amount of research to identifying the nature of the inductive signal that passes from the chordamesoderm to the ectoderm.
Early Formation of the Neural Plate
The first obvious morphological response of the embryo to neural induction is the transformation of the dorsal ectoderm overlying the notochordal process into an elongated patch of thickened epithelial cells called the neural plate (Fig. 5.11). The border of the neural plate is specified by exposure of those cells to a certain concentration of BMP. This is the region from which the neural crest (see p. 254) arises.
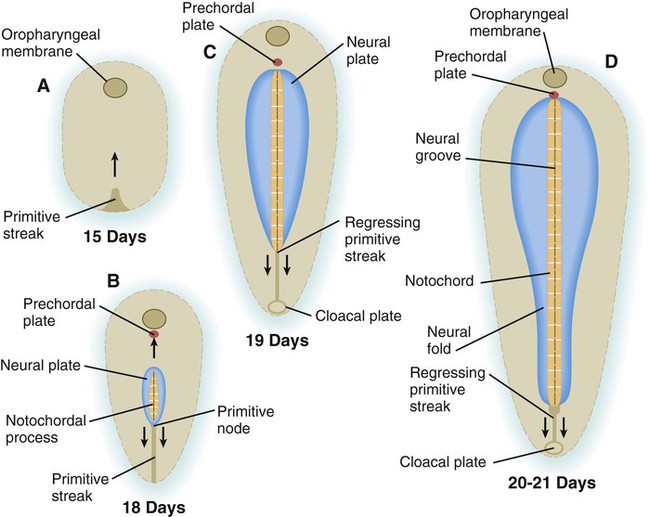
A, Day 15. B, Day 18. C, Day 19. D, Days 20 to 21.
As development progresses, certain decisions are made that narrow the developmental options of cells (Fig. 5.12). For example, at an early stage in cleavage, some cells become committed to the extraembryonic trophoblastic line and are no longer capable of participating in the formation of the embryo itself. At the point at which cells are committed to becoming trophoblast, a restriction event has occurred. When a group of cells has gone through its last restriction event (e.g., the transition from cytotrophoblast to syncytiotrophoblast), their fate is fixed, and they are said to be determined.* These terms, which were coined in the early days of experimental embryology, are now understood to reflect limitations in gene expression as cell lineages follow their normal developmental course. The rare instances in which cells or tissues strongly deviate from their normal developmental course, a phenomenon called metaplasia, are of considerable interest to pathologists and individuals who study the control of gene expression.
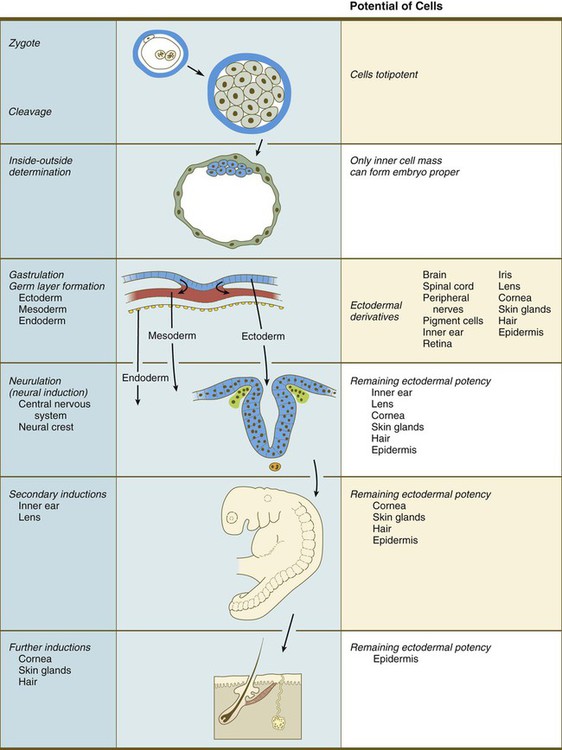
The labels on the right illustrate the progressive restriction of the developmental potential of cells that are in the line leading to the formation of the epidermis. On the left are developmental events that remove groups of cells from the epidermal track.
Cell Adhesion Molecules
Contemporary research has provided a molecular basis for many of the cell aggregation and sorting phenomena described by earlier embryologists. Of several families of CAMs that have been described, three are of greatest importance to embryonic development. The first are the cadherins, which are single transmembrane glycoproteins typically arranged as homodimers that protrude from the cell surface. In the presence of calcium (Ca++), cadherin dimers from adjacent cells adhere to one another and cause the cells to become firmly attached to one another (Fig. 5.16). One of the most ubiquitous is E-cadherin, which binds epithelial cells to one another (see Fig. 16.6). During epithelial-mesenchymal transformations, such as that shown in Figure 5.8, the epithelial cells lose their E-cadherins as they transform into mesenchymal cells, but if these cells reform an epithelium later in development, they re-express E-cadherins.
The immunoglobulin Ig (immunoglobulin)-CAMs are characterized by having varying numbers of immunoglobulinlike extracellular domains. These molecules adhere to similar (homophilic binding) or different (heterophilic binding) CAMs on neighboring cells, and they do so without the mediation of calcium ions (see Fig. 5.16). One of the most prominent members of this family is N-CAM, which is strongly expressed within the developing nervous system. Ig-CAMs do not bind cells as tightly as cadherins, and they provide for fine-tuning of intercellular connections. N-CAM is unusual in having a high concentration of negatively charged sialic acid groups in the carbohydrate component of the molecule, and embryonic forms of N-CAM have three times as much sialic acid as the adult form of the molecule.
In the early embryo, before primary induction of the central nervous system, the ectoderm expresses N-CAM and E-cadherin (formerly known as L-CAM). After primary induction, cells within the newly formed neural tube continue to express N-CAM, but they no longer express E-cadherin. They also strongly express N-cadherin. In contrast, the ectoderm ceases to express N-CAM, but it continues to express E-cadherin (Fig. 5.17).
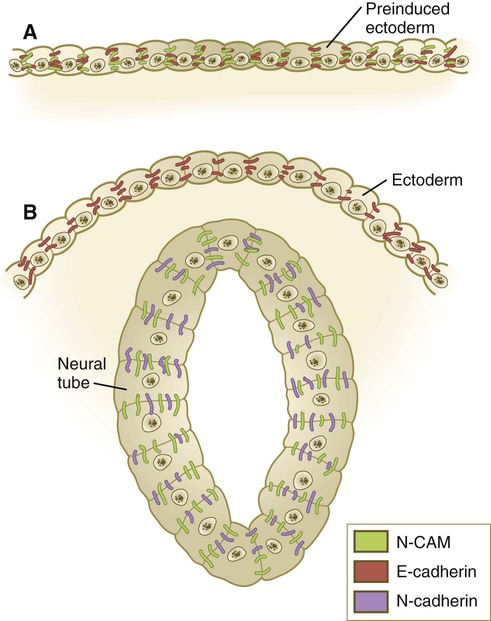
Preinduced ectoderm (A) after induction of the neural tube (B). CAM, cell adhesion molecule.
The third major family of CAMs, the integrins, attaches cells to components of basal laminae and the extracellular matrix (see Fig. 5.16). Integrins form heterodimers consisting of 1 of 16 α chains and 1 of 8 β chains. The matrix molecules to which they bind cells include fibronectin, laminin, and tenascin (see Fig. 12.3).
Summary
Just before implantation, the inner cell mass becomes reorganized as an epithelium (epiblast), and a second layer (hypoblast) begins to form beneath it. Within the epiblast, the amniotic cavity forms by cavitation; outgrowing cells of the hypoblast give rise to the endodermal lining of the yolk sac. Extraembryonic mesoderm seems to form by an early transformation of parietal endodermal cells and cells migrating through the primitive streak.
The pregastrula embryo sets up two signaling centers. The anterior visceral endoderm induces the head and inhibits anterior extension of the primitive streak. The posterior center induces the primitive streak and the formation of mesoderm.
During gastrulation, a primitive streak forms in the epiblast at the caudal end of the bilaminar embryo. Cells migrating through the primitive streak form the mesoderm and endoderm, and the remaining epiblast becomes ectoderm.
The primitive node, located at the cranial end of the primitive streak, is the source of the cells that become the notochord. It also functions as the organizer or primary inductor of the future nervous system.
As they pass through the primitive streak, future mesodermal cells in the epiblast change in morphology from epithelial epiblastic cells to bottle cells and then to mesenchymal cells. Extraembryonic mesodermal cells form the body stalk. The migration of mesenchymal cells during gastrulation is facilitated by extracellular matrix molecules such as hyaluronic acid and fibronectin.
Late in the third week after fertilization, the primitive streak begins to regress caudally. Normally, the primitive streak disappears, but sacrococcygeal teratomas occasionally form in the area of regression.
The essential elements of neural induction are the same in all vertebrates. In mammals, the primitive node and the notochordal process act as the primary inductors of the nervous system. Mesodermal induction occurs even earlier than neural induction. Growth factors such as Vg1 and activin are the effective agents in mesodermal induction.
Numerous signaling centers control the organization of many important embryonic structures during early development. Each is associated with a constellation of important developmental genes. The early gastrula organizer is involved in initiation of the primitive streak. The primitive node organizes the formation of the notochord and nervous system and many aspects of cellular behavior associated with the primitive streak. The notochord is important in the induction of many axial structures, such as the nervous system and somites. Formation of the head is coordinated by the anterior visceral endoderm (hypoblast) and the prechordal plate.
Early blastomeres are totipotent. As development progresses, cells pass through restriction points that limit their differentiation. When the fate of a cell is fixed, the cell is said to be determined. Differentiation refers to the actual expression of the portion of the genome that remains available to a determined cell, and the term connotes the course of phenotypic specialization of a cell.
Left-right asymmetry in the early embryo is accomplished by the action of ciliary currents at the node carrying nodal to the left side of the embryo. This releases a cascade of molecules, with Pitx-2 prominent, that causes the asymmetric formation of structures, such as the heart, liver, lungs, and stomach.
Embryonic cells of the same type adhere to one another and reaggregate if separated. The molecular basis for cell aggregation and adherence is the presence of adhesion molecules on their surfaces. The three main families are the cadherins and the Ig-CAMs, which mediate cell-to-cell adhesion, and the integrins, which bind cells to the surrounding extracellular matrix.
*Hensen’s node is the commonly used designation for the primitive node in avian embryos, but this term is sometimes used in the mammalian embryological literature as well. This structure is the structural and functional equivalent of the dorsal lip of the blastopore in amphibians.
*The term specified (specification) is becoming increasingly used as a near synonym to determination in referring to the fixation of the future fate of a cell.