Chemistry of Life
After reading this chapter, the student will be able to:
• Define/describe matter, element, atom, and ion
• Define/describe the atomic nucleus and define atomic weight, neutron, proton, electron, valence, and isotope
• Name, describe, and rank the different types of chemical bonds
• Describe the different types of chemical reactions
• Define the rules of chemical notation
• Discuss acid–base balance and the pH scale
• Discuss the properties of water and define solvent, solute, solution, hypertonic, hypotonic, isotonic, hydrophilic, and hydrophobic
• Describe the common properties of all organic molecules
• Name the monomers of carbohydrates and describe the structure and function of disaccharides and polysaccharides
• Describe the structures and functions of amino acids, peptides, and proteins
• Name and describe the structures and functions of the different lipids
• Describe the structures of nucleic acids and nucleotides; name and discuss the function of the different nucleic acids and explain complementary base pairing
Atoms and Ions
Elements
Knowledge of the chemistry of life begins with an understanding of those chemical principles that govern the processes occurring in matter. Matter is defined as anything that occupies space and has mass. It can be in liquid, gaseous, or solid form and is composed of elements, the smallest particles of which are atoms. Elements cannot be broken down further by natural forces. Oxygen, carbon, hydrogen, nitrogen, phosphorus, and sulfur are some of the elements most commonly found in living cells (Table 2.1). Although these chemical elements usually do not exist in free form, they do occur in combinations called chemical compounds. The shorthand expression of a chemical compound is its chemical formula. For example, the chemical formula of table salt or sodium chloride is NaCl (see Chemical Notations, below).
TABLE 2.1
Common Elements in Living Organisms
Element | Symbol | Atomic Number | Atomic Weight |
Hydrogen | H | 1 | 1 |
Carbon | C | 6 | 12 |
Nitrogen | N | 7 | 14 |
Oxygen | O | 8 | 16 |
Sodium | Na | 11 | 23 |
Magnesium | Mg | 12 | 24.3 |
Phosphorus | P | 15 | 31 |
Sulfur | S | 16 | 32.1 |
Chlorine | Cl | 17 | 35.5 |
Potassium | K | 19 | 39.1 |
Calcium | Ca | 20 | 40.1 |
Iron | Fe | 26 | 55.8 |
Cobalt | Co | 27 | 58.9 |
Copper | Cu | 29 | 63.5 |
Zinc | Zn | 30 | 65.4 |
Iodine | I | 53 | 126.9 |
Atomic Model
All atoms have the same fundamental structure consisting of a center, or atomic nucleus, and surrounding shells (Figure 2.1), but because of the different numbers of subatomic particles, each element has its own characteristic atomic structure. Located in the center of the atom is the atomic nucleus, which consists of positively charged particles called protons, and particles without charge called neutrons. The atomic weight (atomic mass) of an atom is equal to the sum of protons and neutrons. The atomic number indicates the number of protons in the atomic nucleus. Surrounding the atomic nucleus in shells are negatively charged subatomic particles called electrons. Electrons travel around the nucleus at high speed and occupy positions in a volume of space called an orbital or electron cloud. These orbitals form an energy level also referred to as shells, in which the electrons usually remain. Electrons fill the orbitals and shells in pairs, and each orbital within a shell can carry two electrons.
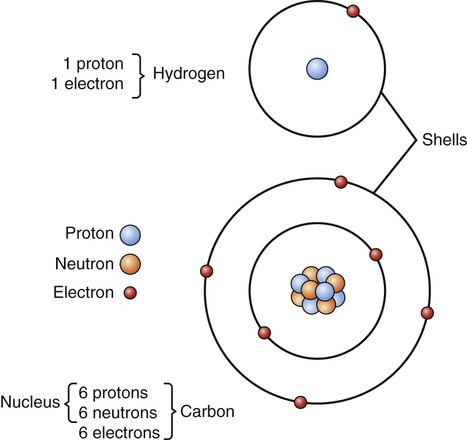
These simplified diagrams of hydrogen and carbon atoms show the atomic nucleus containing protons and neutrons in the center of the atom, and the electrons in the surrounding shells.
The nucleus of a given atom is surrounded by successive shells spaced further and further away from the nucleus. The energy level of electrons increases with the distance of their shells from the nucleus. The innermost (first) shell can be occupied by up to two electrons within one orbital, the second shell with up to eight within four orbitals, and each consecutive shell can potentially hold more electrons. However, most elements with biological significance need eight electrons to fill the outermost shell. The shells always fill sequentially from the inside out: two electrons in the first shell, eight in the next, and so on. For example, carbon with 6 electrons carries 2 electrons in the first shell and 4 in the second shell, and sodium with 11 electrons has 2 electrons in the first shell, 8 in the second, and 1 in the third shell (Figure 2.2).
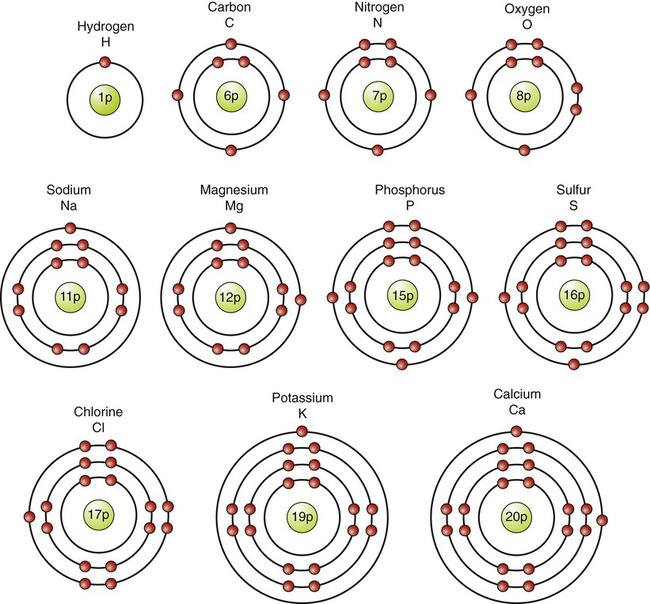
The diagrams illustrate that the number of protons equals the number of electrons in each of these atoms, which are found in association with living systems.
Isotopes are atoms with the same number of protons but a different number of neutrons. The atomic number of isotopes is unchanged because the number of protons remains the same and only the atomic weight is different. For example, the element hydrogen has two isotopes (Figure 2.3):
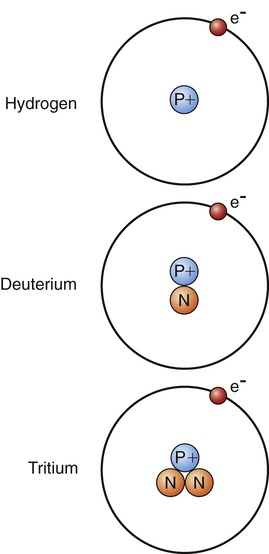
Hydrogen has one proton only, deuterium has one proton and one neutron, and tritium has one proton and two neutrons.
Ions
Ions are electrically charged atoms, molecules, or subatomic particles that are formed when one or more valence electrons are transferred from one atom to another (see Formation and Classification of Chemical Bonds and Forces, below). If an atom loses one or more electrons to another atom, it becomes positive (+), whereas the atom that gains the electron becomes negative (−). Positively charged ions are called cations, and in an electric field move toward the negative pole, the cathode. Negatively charged ions, referred to as anions, move toward the positive pole, or anode, of an electric field.
A substance that dissociates into free ions when dissolved in a solvent such as water is called an electrolyte. The solvent in which it is dissolved can then conduct an electric current and is referred to as an electrically conductive medium. Because these solvents contain ions or electrolytes they are called ionic solutions. Chemically they are acids, bases, or salts (see Acids, Bases, and the pH Scale, below).
Several cations and anions (Table 2.2) are important components of higher life forms. All of these higher life forms require a complex electrolyte balance, called an osmotic gradient, between their intercellular and extracellular fluid compartments (see Chapter 3, Cell Structure and Function). This maintenance of a precise internal balance of electrolytes to maintain the osmotic gradient is called homeostasis. It is required to regulate the hydration, blood pH, and nerve and muscle function of an organism.
TABLE 2.2
Common Ions in Living Organisms
Cations | Anions |
Sodium (Na+) | Chloride (Cl−) |
Potassium (K+ ) | Bicarbonate (HCO3−) |
Calcium (Ca2+ ) | Phosphate (PO43−) |
Magnesium (Mg2+ ) | Sulfate (SO42−) |
Chemical Bonds and Molecules
Formation and Classification of Chemical Bonds and Forces
Molecules made from atoms of different elements are called compounds. Compounds are new chemicals with properties that are different from those of the atoms of which they are composed. Groups of atoms that consistently form specific groups within compounds are referred to as functional groups. They have specific characteristics that are different from those of the individual participating atoms of that given group. Some molecules have more than one functional group, which may differ from one another. The most common functional groups found in molecules important to living organisms are shown in Table 2.3.
TABLE 2.3
Common Functional Groups in Living Organisms
Functional Group | Formula | Functional Group | Formula |
Acetyl | CH3 | Ethyl | C2H5 |
Aldehyde | CHO | Hydroxyl | OH |
Amino | NH2 | Keto | CO |
Ammonium | NH4 | Methyl | CH3 |
Bicarbonate | HCO3 | Nitrate | NO3 |
Carbonate | CO3 | Phosphate | PO4 |
Carboxyl | COOH | Sulfate | SO4 |
Covalent bonds result from a sharing of electrons between two atoms of the same element or between atoms of different elements. In bonds between identical atoms such as oxygen and hydrogen, the electrons are shared equally by each atom. Covalent bonds usually are the strongest chemical bonds. Because the electrons are equally distributed, the resulting molecule is nonpolar and the bond is called a nonpolar covalent bond (Figure 2.4, A). Carbon atoms play a significant role in large organic molecules because they form stable nonpolar covalent bonds with each other. This stable framework is the backbone of organic carbon-based molecules, providing the chemical foundation of organic chemistry and of life.
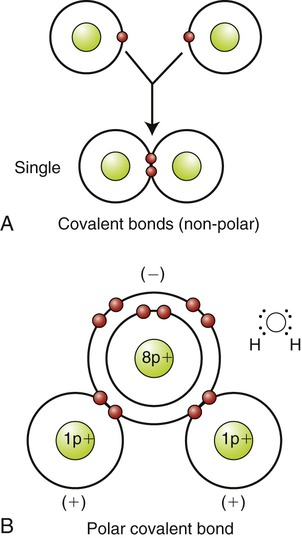
A, Nonpolar covalent bond between two hydrogen atoms (H2), formed by an equal sharing of electrons. B, Polar covalent bond between an oxygen atom and two hydrogen atoms (H2O), formed by an unequal sharing of electrons.
The covalent bonds between atoms of two different-sized elements are polar covalent bonds, in which the electrons are unequally distributed because they are pulled toward the larger atom. As a result, one end of the molecule becomes more negative compared with the other end (Figure 2.4, B). Oxygen, nitrogen, and phosphorus atoms have a tendency to form polar covalent bonds. Polar covalent bonds are somewhat weaker than nonpolar covalent bonds. Coordinate covalent bonds, such as occurs in the formation of the ammonium ion from ammonia, are formed when both electrons are from one atom. The molecule no living organism can exist without is water, in which the atoms hydrogen and oxygen are held together by polar covalent bonds. Some properties of water result from this type of bond.
Ionic bonds are formed when one or more electrons from one atom are transferred to another. If an atom loses one electron in the process it will have a charge of +1; if two electrons are lost the charge will be +2, because the protons in the nucleus will be unbalanced by the remaining electrons. The resulting anions and cations in an ionic bond are held together by attraction of their opposite charges and form an ionic compound. Ionic bonds can easily dissociate (break down) in water to form electrolyte solutions. For example, in water metals such as Na+, readily give up electrons, and nonmetals such as Cl− readily take up electrons (Na+ + Cl− → NaCl). If the water is evaporated, a solid crystal of NaCl, common table salt, is formed. Sodium with a total of 11 electrons (2 + 8 + 1) has only 1 electron in its outermost shell, whereas chlorine with a total of 17 electrons (2 + 8 + 7) only needs 1 electron to fill its outermost shell. The only electron in sodium’s outermost shell is therefore attracted to chlorine’s outermost shell and its transfer forms an ionic compound (Figure 2.5, A). The charged sodium chloride molecules and other salts form characteristic large crystal structures in which the atoms of the molecules alternate in a regular, geometric pattern (Figure 2.5, B). In water, NaCl readily dissociates to form an electrolyte.
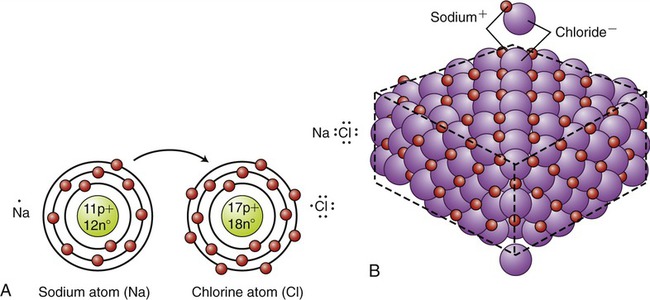
A, The electron in the outermost shell of the sodium atom is transferred to the chlorine atom, resulting in a sodium (+) and a chloride (−) ion. B, Crystal of sodium chloride (table salt). The sodium and chloride ions alternate in a definite, regular, geometric pattern.
Hydrogen bonds are weak chemical bonds with only about 5% of the strength of covalent bonds. However, when many hydrogen bonds are formed between two molecules, the resulting union can be strong enough to be stable. These bonds are formed by attraction forces between charged atoms within a large molecule or between adjacent molecules (Figure 2.6). Hydrogen bonds always involve a hydrogen atom with a slight positive charge and an oxygen or nitrogen atom with a slightly negative charge. Although hydrogen bonds do not form molecules they can alter the shapes of molecules or hold together different molecules. Examples of hydrogen bonds include bonds between water molecules, acetic acid molecules, amino acid molecules, and nucleic acid molecules. Hydrogen bonds are always indicated by dotted lines (—). The attraction created by hydrogen bonds keeps water in the liquid state over a wide range of temperatures.
Types of Chemical Reactions
• Dehydration synthesis (condensation)
• Endergonic (energy-requiring) reactions
• Exergonic (energy-producing) reactions
Dehydration synthesis, or condensation, is the formation of a larger compound (polymer) from smaller ones (monomers). Monomers are the unit molecules (building blocks) of these larger molecules, called polymers. These reactions require specific enzymes and the removal of water from the reactants, that is, a hydroxyl group (OH) is removed from one monomer and combined with a hydrogen (H) from the other (Figure 2.7). Enzymes (see Chapter 3, Cell Structure and Function) are biological catalysts and function to speed up the rate of chemical reactions without changing themselves. The synthesis of new compounds within a cell occurs during anabolism, which utilizes energy provided by catabolism (see Cellular Metabolism in Chapter 3, Cell Structure and Function). An example of synthesis is the production of complex sugars from simple sugars (see Carbohydrates, below).
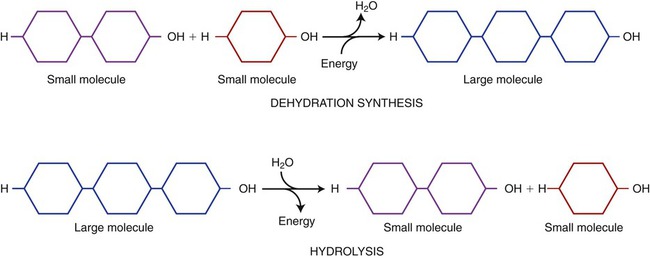
Dehydration synthesis is an anabolic reaction (requires energy) producing polymers (large molecules) from monomers (small molecules) by removing water. Hydrolysis is a catabolic reaction (releasing energy) in which polymers are broken down into monomers. These reactions require water.
< ?xml:namespace prefix = "mml" />



Oxidation–reduction (electron transfer) reactions are coupled. In other words, oxidation reactions occur with reduction reactions (Figure 2.8). Reduction is the gain of electrons. It is also catalyzed by enzymatic reactions and is written as follows:
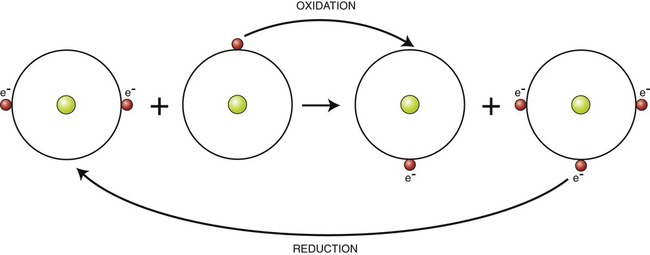
Oxidation refers to the loss of electrons, whereas reduction involves gaining electrons.


The balancing of chemical activities in living cells is a continuous, dynamic process. In general, however, breaking of bonds releases more energy than is required for synthesis. A living cell continuously undergoes hydrolysis and synthesis for the purpose of energy production such as occurs in cells during the breakdown of complex molecules (catabolism). Chemical bonds contain potential energy that is released when the bonds are broken. This energy is captured and used in reactions for the essential functions of a cell. Catabolic reactions are primarily oxidation reactions and are divided into different pathways, namely, glycolysis, the Krebs cycle (citric acid cycle), and the electron transport chain (see Chapter 3, Cell Structure and Function).
Chemical Notations
Chemical compounds and reactions are shown by “chemical shorthand” or chemical notation. The rules of the chemical notation are as follows (Box 2.1):
• The abbreviation of an element represents one atom of that element and is its chemical symbol.
• The number before the chemical symbol is the number of atoms; the number before the chemical formula is the number of molecules.
• The subscript after the chemical symbol of an element shows the number of that atom in the molecule.
• The reaction of the chemicals describes the interaction of the participants, called the reactants. Chemical reactions form one or more products. Arrows in the formula indicate the direction of the reaction, from reactant to product. Arrows in both directions indicate a reversible chemical reaction that can go in either direction. For example,

The equation indicates that two atoms of hydrogen and one atom of oxygen combine to form water (H2O). Another example:

Here one molecule of sodium hydroxide (NaOH) and one molecule of hydrochloric acid (HCl) form salt (NaCl) and water (H2O); the reaction is reversible.
• A superscript of plus or minus after the atomic symbol indicates an ion. A single plus or minus shows the charge of an ion. If more than one electron has been lost or gained the charge of the ions is shown with a number before the plus or minus sign.
• Chemical reactions do not form or destroy atoms; they just rearrange them into new combinations. In any given chemical equation the number of atoms of each element must be the same on both sides, resulting in a balanced equation.
Inorganic Compounds
Acids, Bases, and the pH Scale
Some chemical compounds dissociate in water as ions, carry an electric current, and display an electrical charge. Substances that release hydrogen ions (H+) are acids, and those that release hydroxyl ions (OH−) are bases. The strength of acids and bases is determined by the hydrogen ion concentration of the water in which they dissociate. The higher the hydrogen ion concentration in the solution the more acidic the solution is. A low hydrogen ion concentration of a solution indicates a basic solution (Box 2.2).
This acidity or alkalinity of a solution is measured by the pH scale (“potential hydrogens”). It is a chemical symbol that ranges from 0 to 14 and is the negative logarithm of the hydrogen ion concentration (Figure 2.9). A solution that has a neutral pH is one in which the concentrations of H+ and OH− ions are equal (10−7 M), and the chemical symbol for this negative logarithm is pH 7. The point of neutrality is standardized as the pH of pure water at 25° C. As the H+ concentration increases, the OH− concentration decreases and vice versa. A change one of 1 unit on the pH scale (the negative logarithm scale) represents a 10-fold change in the hydrogen ion concentration. The higher the hydrogen ion concentration of a solution, the lower the number on the pH scale and the more acid the solution; the higher the number on the scale, the higher the hydroxyl ion concentration and the more basic (alkaline) the solution.
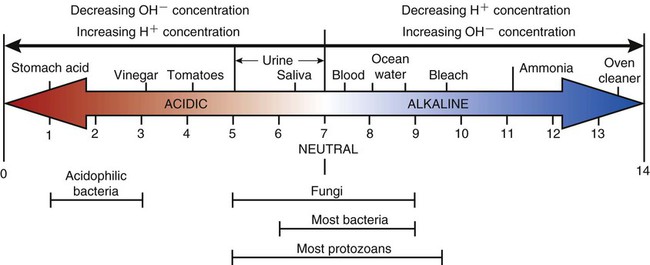
With increasing hydrogen ion concentrations a solution becomes more acidic and the pH value decreases. When the hydrogen ion concentration decreases the solution becomes more basic and the pH value increases.
Chemical reactions in a living cell respond to slight changes in the pH of their environment. The majority of microbes as well as human cells survive better in a neutral or slightly basic environment. This sensitivity of microbes to changes in pH is used in the control of microbial growth and in food preservation (see Chapter 19, Physical and Chemical Methods of Control). However, as mentioned in Chapter 1, some microorganisms are found to exist successfully in all environments, such as sulfur-oxidizing bacteria, which prefer a very acidic environment, and yeast, which flourishes under slightly acidic conditions.
Salts


Sometimes salts are referred to less specifically by the name of the cation (e.g., sodium salt, ammonium salt) or by the name of the anion (e.g., chloride, acetate). Common salt-forming cations and anions are shown in Table 2.4.
TABLE 2.4
Common Salt-forming Anions and Cations
Salt-forming Anions* | Salt-forming Cations | ||
Name | Formula | Name | Formula |
Acetate (acetic acid) | CH3COO− | Ammonium | NH4+ |
Carbonate (carbonic acid) | CO32− | Calcium | Ca2+ |
Chloride (hydrochloric acid) | Cl− | Iron | Fe2+ and Fe3+ |
Hydroxide | OH− | Magnesium | Mg2+ |
Nitrate (nitric acid) | NO3− | Potassium | K+ |
Oxide | O2− | Pyridinium | C5H5NH+ |
Phosphate (phosphoric acid) | PO43− | Quaternary ammonium | NR4+† |
Sulfate (sulfuric acid) | SO42− | Sodium | Na+ |
Salts are usually solid crystals but can exist as a liquid at room temperature and are called ionic liquids. Different salts can stimulate sensations of all five basic tastes: salty (sodium chloride), sweet (lead diacetate), sour (potassium bitartrate), bitter (magnesium sulfate), and umami (monosodium glutamate). Pure salts are odorless whereas impure salts may smell acidic (acetates) or basic (ammonium salts). Salts can be clear and transparent (sodium chloride), opaque (titanium dioxide), or metallic (iron disulfate). They also exist in different colors (Table 2.5). Most mineral, inorganic pigments and many synthetic organic dyes are salts.
TABLE 2.5
Name of Salt | Formula | Color |
Sodium chloride | NaCl | Clear, transparent |
Titanium dioxide | TiO2 | Opaque, white |
Iron disulfide | FeS2 | Metallic |
Sodium chromate | Na2Cr2O4·2H2O | Yellow |
Sodium dichromate | Na2Cr2O7·2H2O | Orange |
Mercury sulfide | HgS | Red |
Cobalt dichloride hexahydrate | CoCl2·6H2O | Mauve |
Copper sulfate pentahydrate | CuSO4·5H2O | Blue |
Ferric hexacyanoferrate | Fe4[Fe(CN)6]3 | Blue |
Nickel oxide | Ni2O3 | Green |
Magnesium sulfate | MgSO4 | Colorless |
Manganese dioxide | MnO2 | Black |
Water
Water is a contributor in most chemical reactions of cells and is essential to break down polymers into monomers by the process of hydrolysis. The amount of water needed for metabolic activities varies among different microorganisms. The availability of water influences microbial growth rates (see Chapter 6, Bacteria and Archaea). Some of the properties of water are shown in Box 2.3.
Water is a solvent and can dissolve many different substances, and is therefore often called the “universal solvent.” The substances dissolved in water or another solvent are solutes, and the combination of a solvent and its solutes is referred to as a solution (Figure 2.10). The solubility of molecules is determined by their molecular structure. Molecules that exhibit local differences in electrical properties, or polar areas, are water soluble and those that do not are insoluble in water. The survival of living cells depends on the appropriate concentration of solutes in a solution. Most organisms do not tolerate environments where the concentration of solutes is much higher than that in their intracellular environment. Depending on the amount of solutes within or outside a cell, the environment can be:
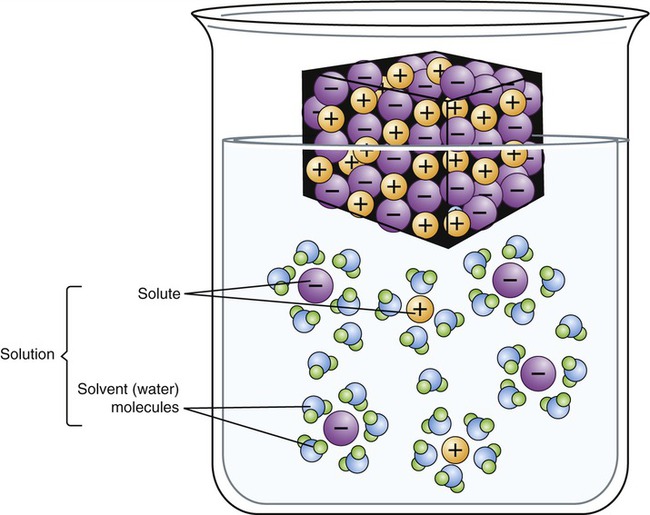
In this diagram water acts as the solvent, sodium and chloride ions are the solutes, and when solutes are dissolved (dissociated) in a solvent, a solution is formed.
• Isotonic: The solute concentration and hence the osmotic pressure within the cell (intracellular) is the same as it is outside of the cell (extracellular). A cell placed in an isotonic solution will not change its cell volume.
• Hypertonic: The solute concentration in the cell is less than in the extracellular environment, which causes a net loss of water from the cell, resulting in cell shrinkage. The cell shape becomes notched or crenated.
• Hypotonic: The solute concentration in the extracellular environment is less than that inside the cell (intracellular), causing the uptake of water into the cell, resulting in the bursting of the cell (Figure 2.11)
Because of their polarity, ions attract the polar water molecules that surround the ions, which in turn attract other water molecules to form hydration spheres around each ion (Figure 2.12). Formations of hydration spheres are responsible for the solubility of ions in water. Organic molecules such as glucose, amino acids, and others are water soluble if the covalent bonding pattern permits the formation of hydration spheres around their atoms of oxygen, nitrogen, and phosphorus. These molecules are hydrophilic (water loving) water-soluble compounds. Molecules held together by nonpolar covalent bonds are hydrophobic (water repelling) and insoluble in water because of their inability to form hydration spheres. Parts of drug molecules may be hydrophilic, conferring water solubility properties on them, and vice versa for hydrophobic parts of drug molecules (see Chapter 21, Pharmacology).
Organic Molecules
All organic molecules contain atoms of carbon and hydrogen. Organic molecules have a backbone of chains or rings formed by the carbon and hydrogen atoms, referred to as a hydrocarbon backbone. Carbons commonly form covalent bonds not only with hydrogen, but also with oxygen, nitrogen, sulfur, and phosphorus. It is this covalent bonding of carbons with other carbons that yields the immense number and variety of organic molecules and allows different arrangements of chains or rings (Figure 2.13). The major organic molecules in living organisms are carbohydrates, proteins, lipids, and nucleic acids. Each of these compounds is composed of specific unit molecules or monomers (Table 2.6).
TABLE 2.6
Organic Molecules and Their Monomers
Organic Molecule | Monomer |
Carbohydrates | Monosaccharides |
Proteins | Amino acids |
Lipids | Glycerol and fatty acids |
Nucleic acids | Nucleotides |
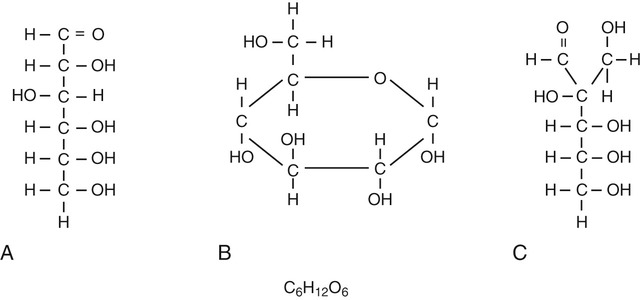
Carbon atoms can be arranged in (A) chains, (B) rings, or (C) branched chains.
Carbohydrates
Disaccharides (Figure 2.14, A) are compounds formed when two monosaccharides combine with the loss of a water molecule. Disaccharides include the following:
Polysaccharides (Figure 2.14, B) are formed when many monosaccharides combine to form a larger compound. Starch in plants and glycogen in animals are polysaccharide storage forms of glucose. The most abundant polysaccharide is cellulose, a major component of the cell walls of plants, fungi, and most algae.
Proteins
There are 20 known naturally occurring amino acids (Table 2.7), and they are the monomers of proteins. All amino acids consist of an amino group, a carboxyl group, and a variable side chain designated chemically as R (the R group; Figure 2.15). Two amino acids joined together form a dipeptide; 3 amino acids form a tripeptide; and a chain of 10 or more amino acids form a polypeptide.
TABLE 2.7
Naturally Occurring Amino Acids
Amino Acid | Abbreviation | Amino Acid | Abbreviation |
Alanine | Ala | Leucine | Leu |
Arginine | Arg | Lysine | Lys |
Asparagine | Asn | Methionine | Met |
Aspartic acid | Asp | Phenylalanine | Phe |
Cysteine | Cys | Proline | Pro |
Glutamic acid | Glu | Serine | Ser |
Glutamine | Gln | Threonine | Thr |
Glycine | Gly | Tryptophan | Trp |
Histidine | His | Tyrosine | Tyr |
Isoleucine | Ile | Valine | Val |
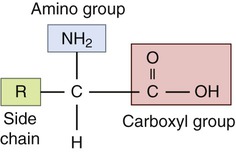
Amino acids contain amino and carboxyl groups as well as the variable R group side chain.
Recalling Crick’s central dogma that DNA makes mRNA and mRNA makes protein, the sequence of amino acids in a polypeptide is determined in the codons of messenger ribonucleic acid (mRNA), which translates codons (three-letter genetic words) into a polypeptide chain. The mRNA codons are transcribed from codons in deoxyribonucleic acid (DNA). All living things are dependent on proteins for structure and function (Box 2.4). Proteins can contain up to 10,000 amino acids. The sequence and folding of the amino acid chains determine the shape, which in turn determines the function and specificity of a protein. Different combinations of amino acids yield an infinite variety of polypeptides, which provides the chemical basis for the incredible biological diversity in structure and function among living organisms.
Proteins occur in four different structural arrangements (Figure 2.16):
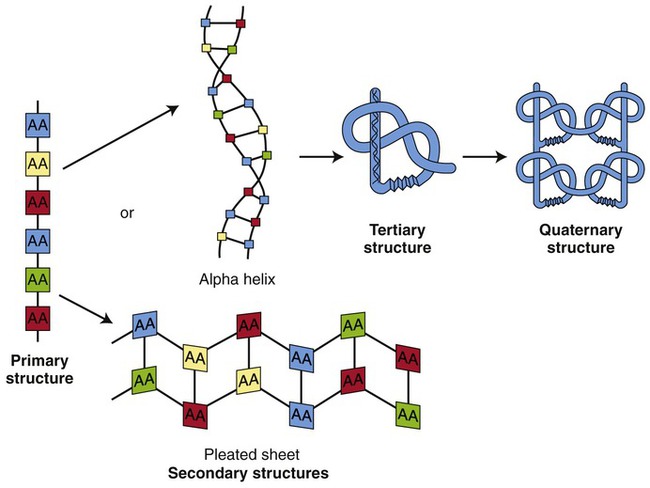
The primary structure consists of a chain of amino acids (AA), the secondary structure can be either an α helix or a β sheet, the tertiary structure has a globular shape, and the quaternary structure contains several different polypeptides forming a functional unit.
• A primary structure is represented by a single chain of amino acids. Examples of primary structure proteins are small hypothalamic hormones such as gonadotropin-releasing hormone (GnRH) and thyrotropin-releasing hormone (TRH).
• A secondary structure is made of polypeptide chains that are either folded into a β sheet (β conformation) or form an α helix. The right-handed helix makes a complete turn in a clockwise direction every 3.6 amino acids. The α helix is held together by hydrogen bonds. β Sheets consist of two or more polypeptide chains lying side by side and are also stabilized by hydrogen bonds. Examples of such helical proteins are keratin, myosin, and collagen; silk represents a β-pleated sheet.
• A tertiary structure has a globular shape because of the additional coiling of secondary structure proteins. This structure is stabilized by the formation of additional hydrogen, ionic, and disulfide bonds. Properties of solubility are determined by hydrophobic nonpolar chains, which are generally positioned on the inside of the protein, and by hydrophilic polar chains, which are positioned on the outside of protein molecules. Examples of tertiary structure proteins are enzymes and some peptide hormones such as insulin.
• A quaternary structure contains several polypeptides that form a functional unit. Such complexes can consist of several copies of the same polypeptide or different polypeptides. An example of a quaternary structure protein is the hemoglobin molecule.
Heat, pH, salts, radiation, and heavy metals can change the shape of a protein, causing protein denaturation. This process results in nonfunctioning protein compounds. Denatured enzymes (proteins) can no longer function as biological catalysts, and their metabolic reactions come to a stop. Denatured antibodies can no longer bind to an antigen and fail to produce the all-important antigen–antibody complex in immune reactions (see Chapter 20, The Immune System). Denatured hormones are no longer able to act on their target cells. And the denaturing of bacterial or viral proteins often will eliminate the microbe (see Chapter 22, Antimicrobial Drugs).
Lipids
Triglycerides (fats and oils) consist of glycerol and fatty acid chains (neutral fats). At room temperature fats are solid whereas oils are liquid. Structurally a fatty acid has a tail portion, which is a long hydrocarbon chain, and a head portion that consists of a carboxyl group (COOH). The tails of the fatty acids are hydrophobic and the heads are hydrophilic. When the head portion of the fatty acid is attached to a glycerol molecule to form fat, the entire molecule becomes hydrophobic and therefore insoluble in water (Figure 2.17).
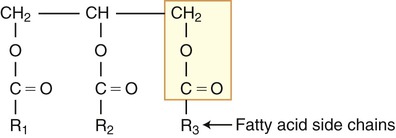
The triglyceride molecule consists of glycerol and fatty acid side chains.
Depending on the absence or presence of double bonds between the carbon atoms of the fatty acid chains, the fats are called saturated, monounsaturated, or polyunsaturated (Figure 2.18). In animal fats the carbons of the fatty acid chains are all bonded by single covalent bonds, meaning that all carbons are bonded to the maximal number of hydrogens. Therefore, animal fats are saturated, closely packed together, and solid at room temperature. On the other hand, plant lipids are oils. They have some double bonds between the carbons, causing bends in the shape of the molecule. These oils are unsaturated fats and are liquid at room temperature.
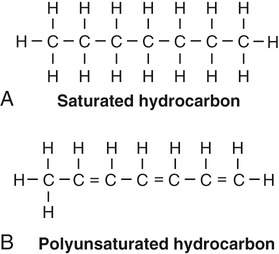
A, Saturated fat with no double bonds within the carbon chain. B, Polyunsaturated fat with several double bonds within the carbon chain.
HEALTHCARE APPLICATION
Selected Lipid Storage Diseases
Disease | Cause | Symptoms | Treatment |
Gaucher disease (three common clinical subtypes) | Glucocerebrosidase deficiency | Enlarged spleen and liver, liver malfunction, skeletal disorders, neurological complications, lymph node swelling, distended abdomen, low platelet count, yellow spots in eyes | Enzyme replacement; bone marrow transplant for nonneurological manifestations; splenectomy may be required; blood transfusion for anemia; no effective treatment for severe brain damage |
Niemann-Pick disease (four categories) | Accumulation of fat and cholesterol in cells of the liver, spleen, bone marrow, lungs, and sometimes brain; inherited in an autosomal recessive pattern | Enlarged spleen and liver, cherry red spot in the eye, neurological disorders, decline of motor skills | No cure, supportive treatment of symptoms |
Fabry disease | α-Galactosidase-A deficiency; buildup of fatty material in the autonomic nervous system, eyes, kidneys, and cardiovascular system | Burning pain in arms and legs, clouding of vision, impaired circulation, heart enlargement, progressive kidney impairment, gastrointestinal difficulties, fever | Drug treatment for pain (phenytoin, carbamazepine); kidney transplant or dialysis; enzyme replacement |
Farber’s disease | Ceramidase deficiency; accumulation of fatty material in joints, tissues, and central nervous system | Impaired mental ability; liver, heart, and kidneys may be affected; vomiting, arthritis, swollen lymph nodes, swollen joints, joint contractures | No specific treatment; corticosteroids to relieve pain |
Phospholipids consist of glycerol, two fatty acid chains, and a phosphate group at one end (Figure 2.19). These molecules are composed of polar (hydrophilic) heads and nonpolar (hydrophobic) tails. The fatty acid groups are hydrophobic whereas the phosphate group is hydrophilic. Phospholipids therefore have a water-soluble polar head and a nonpolar hydrophobic tail. Cell membranes, for example, are composed of a phospholipid bilayer with the tails facing toward each other and the heads facing outward, making their surfaces hydrophilic and their interior hydrophobic. This arrangement is the basis for their biological barrier properties.
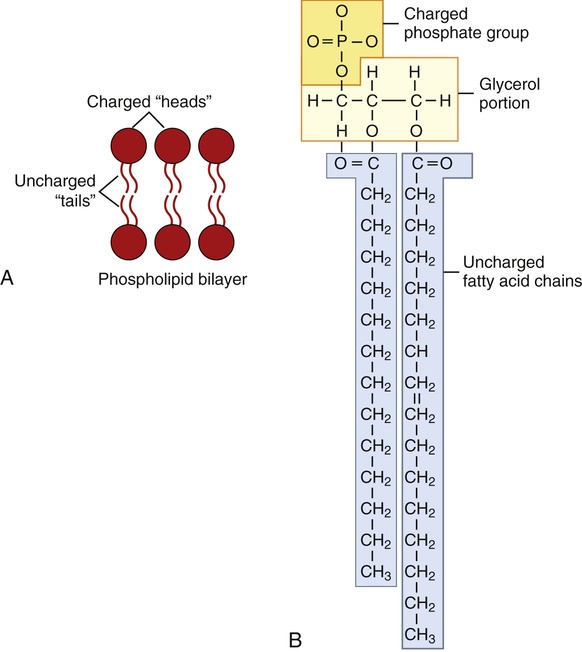
A, Phospholipid bilayer as seen in plasma membranes. B, The phospholipid molecule is composed of a polar, hydrophilic head and a nonpolar, hydrophobic tail.
Steroids (Figure 2.20) have a carbon skeleton consisting of four fused rings with various functional groups attached. Hundreds of different steroids have been found in plants, animals, and fungi. Categories are as follows:
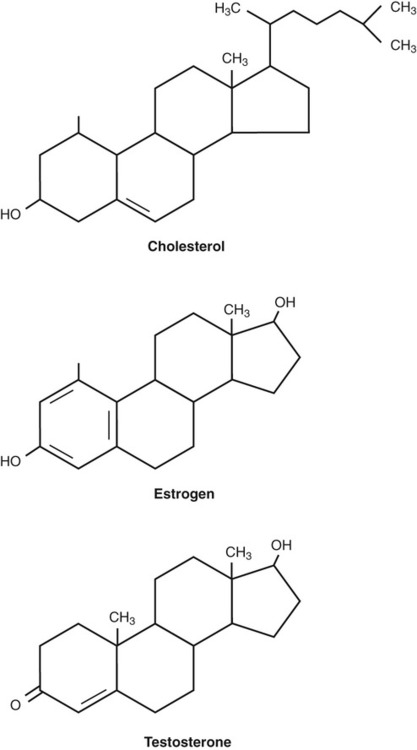
Various steroid molecules are shown, all with four fused carbon rings but differing in their functional groups.
• Anabolic steroids interact with androgen receptors to increase muscle and bone mass. Besides the naturally occurring anabolic steroids, synthetic ones exist that are used (sometimes illegally) by athletes in an attempt to enhance their performance.
• Sex steroids are responsible for the secondary sex characteristics of males and females. They include androgens, estrogens, and progesterones.
• Mineralocorticoids help maintain blood volume, electrolyte balance, and osmolarity by controlling the renal secretion of electrolytes.
• Glucocorticoids play a role in many aspects of metabolism and in immune function. They are often prescribed to reduce inflammatory conditions.
• Phytosterols are a group of steroid alcohols that naturally occur in plants such as yeasts and fungi. Plants contain a wide range of phytosterols that are a structural component in their cell membrane and serve the same function as cholesterol does in animal cells. Ergosterol, a phytosterol, is also referred to as provitamin D2. It is a biological precursor that is converted by ultraviolet irradiation into ergocalciferol, or vitamin D2.
Nucleic Acids
The monomers of nucleic acids are nucleotides, containing the elements carbon, hydrogen, oxygen, nitrogen, and phosphorus. Main functions of nucleic acids include the following: storage of genetic information (DNA), directing protein synthesis (RNA), and energy transfers (ATP and NAD). Nucleotides are composed of three units: a pentose sugar, a phosphate, and nitrogen base (Figure 2.21). The nucleotide structure can be broken down into two main functional parts: a sugar–phosphate backbone and the base. Individual nucleic acids are named according to the sugar they contain, a ribose (RNA) or a deoxyribose (DNA). Five possible nitrogen bases are subdivided into two main groups: purines with adenine (A) and guanine (G) that have a distinctive two-ring structure, and pyrimidines with cytosine (C), thymine (T), and uracil (U) with a single-ringed structure (see Figure 2.21). The bases in a nucleic acid polymer can form hydrogen bonds with the neighboring bases by a process called complementary base pairing. In DNA molecules, adenine always pairs with thymine and guanine with cytosine. In RNA molecules, thymine is replaced with uracil.
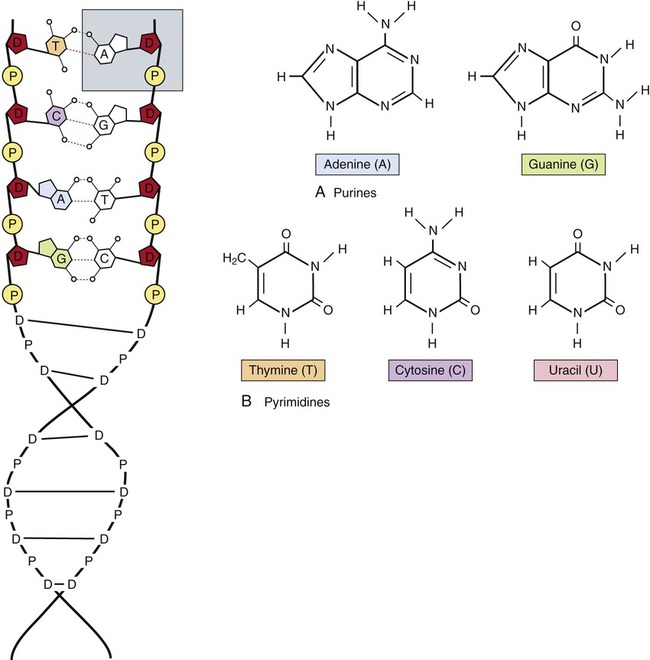
Deoxyribonucleic acid (DNA) is composed of nucleotides, each of which consists of a phosphate, a pentose sugar (deoxyribose), and a nitrogen base (shaded area). The nitrogen bases of nucleic acids are either (A) purines or (B) pyrimidines.
Deoxyribonucleic acid (DNA) is a nucleic acid with a double helix structure containing the sugar deoxyribose and 10 bases per turn (see Figure 2.21). DNA polymers can be thousands of bases long. DNA contains the genetic code and therefore serves for information storage. DNA is responsible for inherited characteristics, growth, and cell reproduction. It is present in both prokaryotic and eukaryotic cells as well as in a group of viruses. DNA contains the information necessary for protein synthesis. The language of DNA, the genetic code, consists of four letters that represent the nitrogen bases, C, G, A, and T, used in three-letter “words” called codons to indicate the 20 naturally occurring amino acids. The combination of codons can create an infinite variety of “sentences.” Each codon represents a specific amino acid and the combination of amino acids results in different polypeptides (see Table 3.6 in Chapter 3, Cell Structure and Function).
Ribonucleic acid (RNA) is similar to DNA but is a single-stranded molecule, its sugar is ribose, and uracil replaces thymine. RNA is specialized for the synthesis of proteins. Three different types of RNA are necessary for the process of protein synthesis: ribosomal RNA (rRNA), messenger RNA (mRNA), and transfer RNA (tRNA). Ribosomes composed of rRNA are made in the nucleus and transported into the cytoplasm, where they attach to rough endoplasmic reticulum (rER) or remain free in the cytoplasm as polyribosomes. Both polyribosomes and ribosomes on the rER (see Chapter 3, Cell Structure and Function) are sites of protein synthesis. Messenger RNA contains genetic information that encodes the sequence of amino acids in proteins. Base triplets, referred to as codons, direct the amino acid sequence in a polypeptide chain. The third type of RNA, tRNA, contains a triplet of nitrogen bases called the anticodon. Anticodons contain complementary bases to the codons on mRNA, and are necessary for the synthesis of polypeptides (see Protein Synthesis in Chapter 3).
Adenosine triphosphate (ATP) is the energy molecule of cells. When energy is released during catabolism it is captured in the high-energy bonds of ATP (Figure 2-22). In transferring energy to other molecules, ATP loses one or two of its phosphate groups, resulting in adenosine diphosphate (ADP) or adenosine monophosphate (AMP). This is an exergonic reaction. Both ADP and AMP can be converted back to ATP by photosynthesis or through chemical energy during anabolism. Photosynthetic microorganisms use sunlight as an energy source during their anabolism. Microorganisms that need nutrient molecules for ATP production are called chemotrophs and the ones utilizing sunlight for energy are called phototrophs (see Chapter 6, Bacteria and Archaea).
Summary
• Matter is anything that occupies space and has mass. It can be in solid, liquid, or gaseous form. All living and nonliving matter consists of elements.
• Atoms have an atomic nucleus containing protons, which are positively charged, and neutrons, which do not have an electrical charge. The nucleus is surrounded by concentric shells in which the negatively charged electrons reside. Because atoms have the same number of protons and electrons they are electrically neutral.
• Molecules are formed when two or more atoms combine through covalent, ionic, or hydrogen bonding.
• All chemical reactions in living organisms are part of metabolism organized in metabolic pathways. These chemical reactions include synthesis, hydrolysis, exchange reactions, oxidation and reduction, as well as endergonic and exergonic reactions. The “shorthand” of chemistry is expressed as chemical notation, which helps to describe the different chemical reactions.
• Although a few inorganic compounds do contain carbon, the vast majority of inorganic compounds do not. Organic compounds are large molecules that always contain carbon and hydrogen.
• Acids, bases, buffers, salts, and water are all inorganic molecules essential to life forms. The pH measures the acidity or alkalinity of a solution on a scale of 0 to 14, with pH 7 set as neutral.
• Carbohydrates store carbon as well as energy and provide part of cellular structures. This group of organic molecules includes monosaccharides, disaccharides, and polysaccharides. Bacteria prefer carbohydrates as an energy source when available.
• Proteins are made of different amino acids, their unit molecules. A protein can contain up to 10,000 amino acids and its sequence and folding pattern determine the shape, which in turn determines the function of the protein.
• The categories of lipids are triglycerides, phospholipids, steroids, cholesterol, and prostaglandins. Functionally, fats provide and store energy, are part of the cell membrane, and play a regulatory role as hormones.
• The monomers of nucleic acids are nucleotides, which consist of a phosphate, a sugar, and a nitrogen base. The functions of nucleic acids include genetic information storage (DNA), protein synthesis (RNA), and energy transfers (ATP and NAD).
Review Questions
1. The atomic number equals the number of:
2. A chemical bond in which electrons are equally shared is a(n):
3. The bond between water molecules is a(n):
4. The outermost shell of an atom can hold up to __________ electrons.
5. The bond between sodium and chlorine atoms in sodium chloride is a(n):
7. The unit molecules (monomers) of carbohydrates are:
8. The bond between amino acids is a(n):
9. The RNA nucleotide base that pairs with adenine of DNA is:
10. Glucose and fructose are examples of:
11. Neutrons are particles with a(n) __________ charge.
12. An atom with the same number of protons but a different number of neutrons is called a(n) __________.
13. A positively charged ion is a(n) __________.
14. The breakdown of large molecules into smaller ones in the presence of water is called __________.
15. Molecules that can absorb hydrogen ions are __________.
16. From the strongest to weakest, name and describe the different types of chemical bonds.
17. Describe anabolism and catabolism.
18. Name and describe the functions of proteins.
19. Compare and contrast saturated and unsaturated fats.
20. Describe complementary base pairing and compare DNA and RNA.