Chapter 96 Channelopathies
Myotonic Disorders and Periodic Paralysis
The term channelopathies has been extended to include disorders affecting all organ systems, but this chapter focuses on channelopathies of skeletal muscle. The initial identification of channelopathies of skeletal muscle began with a number of diseases associated with mutations in the genes for specific channels, including the channels for chloride [Fialto et al., 2007; Trip et al., 2008; Davies and Hanna, 2003; Fahlke et al., 1997a, 1997b; George et al., 1993; Koch et al., 1992], sodium [Cannon, 1996; Struyk and Cannon 2007; Struyk et al., 2008; Fournier et al., 2004; Carle et al., 2009; Jurkat-Rott et al., 2009; Kubota et al., 2009; Matthews et al., 2008], calcium [Matthews et al., 2009; Urbano et al., 2008; Tomlinson et al., 2009], and potassium [Cannon, 2002; Sansone and Tawil; 2007; Sacconi et al., 2009] ions. Periodic symptoms are typical for most channelopathies and occur in disorders other than muscle diseases. For example, familial migraine and hereditary episodic ataxia result from mutations in the calcium channel. These two diseases have joined a growing list of disorders, including familial hypokalemic periodic paralysis, malignant hyperthermia, central core disease, and Lambert–Eaton myasthenic syndrome, that are also calcium channelopathies [Urbano et al., 2008]. This chapter limits its discussion to the disorders listed in Box 96-1 and refers the reader to the preceding references for discussion of other channelopathies.
Box 96-1 General Classification of the Channelopathies Seen in Children: Myotonias and Periodic Paralyses
The Myotonic Dystrophies
The myotonic dystrophies represent a group of dominantly inherited, multisystem (eye, heart, brain, endocrine, gastrointestinal tract, uterus, skin) diseases that share the core features of myotonia, weakness, and early-onset cataracts (younger than 50 years). Table 96-1 summarizes myotonic dystrophy types 1 and 2. Clinicians considered myotonic dystrophy to be a single disease up until the mid-1990s. Steinert and colleagues described the disorder clearly in the early 1900s and that description is still valid [Harper, 2001]. The gene defect responsible for myotonic dystrophy of Steinert was discovered in 1992 and found to be caused by expansion of a CTG repeat in the 3′ untranslated region of DMPK, a gene encoding a protein kinase [Brook et al., 1992; Fu et al., 1992; Mahadevan et al., 1992]. After the discovery of this gene defect, DNA testing revealed a group of patients with dominantly inherited myotonia, proximal greater than distal weakness, and cataracts, who previously were diagnosed as having myotonic dystrophy but who, after testing, lacked a CTG repeat expansion on the DMPK gene. Subsequent clinical studies of kindreds with patients having these characteristics led to the use of new diagnostic labels for these patients: myotonic dystrophy type 2 [Thornton et al., 1994] and proximal myotonic myopathy (PROMM) [Ricker et al., 1994a]. Later studies demonstrated that many of the families identified as having myotonic dystrophy type 2 or PROMM had the same disease, a disorder that results from an unstable four-nucleotide repeat expansion (CCTG) on chromosome 3 [Liquori et al., 2001]. This disease is similar to, but distinct from, classic myotonic dystrophy of Steinert.
Table 96-1 Myotonias Due to Unstable Nucleotide Repeat Expansion
Clinical Features | Myotonic Dystrophy of Steinert (Myotonic Dystrophy Type 1, DM1) | Myotonic Dystrophy Type 2 (Proximal Myotonic Dystrophy, DM2) |
---|---|---|
Inheritance | Dominant | Dominant |
Gene defect | Chromosome 19; CTG expansion affecting a protein kinase; repeat size ranges from 50 to >2000; normal 5–37 repeats | Chromosome 3; CCTG expansion affecting the zinc finger 9 protein gene; repeat sizes vary from 78 to >11,000; normal <78 repeats |
Age of onset | Broad range of ages (infancy to adulthood), with infant onset in most severe cases | Broad range of ages (late childhood to late adulthood) |
Myopathy | Face, eyes, forearm, hands, and legs, with generalized weakness and hypotonia in affected infants | Mild; thighs, hips, neck flexors, occasional calf muscle, abdominal muscle hypertrophy |
Myotonia | Primarily affects hand and forearm muscles and tongue; occasionally affects respiratory muscles and smooth muscle, such as intestine or uterus; myotonia improves with heat and exercise; myotonia results from a decrease in chloride channel protein | Mainly in hands and thighs; varies; frequently hard to detect; pain occurs sometimes with and without myotonia; myotonia improves with repeated contractions; myotonia results from a decrease in chloride protein |
Provocative stimuli | Myotonia worsened by rest and cold; myotonia is relatively constant in severity and muscles affected | Myotonia worsened by rest but varies in severity, occasionally being absent on clinical examination; hand grip and thigh stiffness are usual sites |
Therapy for symptoms | Bracing; cataract removal; monitoring dysrhythmias and respiratory insufficiency; pacemaker; antimyotonia therapy (mexiletine); avoid depolarizing muscle relaxants, opiates, and barbiturates with surgery | Cataract removal; occasional need for pacemaker; antimyotonia therapy often not necessary (mexiletine); monitor carefully during and after surgery for muscle rigidity and rhabdomyolysis |
The existence of different types of myotonic dystrophy has created a need to develop a diagnostic classification. To address this need, the International Myotonic Dystrophy Consortium developed a new nomenclature and guidelines for DNA testing [IDMC, 2000]. Myotonic dystrophy of Steinert, the classic form of myotonic dystrophy that results from an unstable trinucleotide repeat expansion on chromosome 19, is now termed myotonic dystrophy type 1 (DM1). Patients with the clinical picture of myotonic dystrophy type 2/proximal myotonic myopathy (DM2), who have positive DNA testing for the unstable four-nucleotide repeat expansion on chromosome 3, are now classified as having DM2 [Day et al., 2003; Thornton et al., 1994].
Myotonic Dystrophy Type 1
Clinical Features and Subclassification
DM1 is a multisystem, autosomal-dominantly inherited, highly variable muscle disease with an incidence of 1 in 8000 individuals (see Table 96-1) [Harper, 2001; Moxley and Meola, 2008]. Childhood-onset DM1 may present as a severe early congenital form, or as a milder form with symptoms beginning in the early school years. The congenital form presents at birth with respiratory failure, poor feeding, generalized hypotonia, clubfoot deformity, and an increased risk of intracerebral hemorrhage and eventration of the diaphragm [Harper, 2001]. During gestation, there are often reduced fetal movements and a history of polyhydramnios [Harper, 2001; Zaki et al., 2007]. There is an increased frequency of placenta previa and miscarriage [Harper, 2001; Rudnik-Schoneborn and Zerres, 2004; Rudnik-Schoneborn et al., 2006], and, not uncommonly, a failed labor may require urgent cesarean section. During the perinatal period, infants with congenital myotonic dystrophy may require continuous ventilator support, and those remaining ventilator-dependent beyond 4 weeks of age have a poor prognosis for survival, although recent advances in neonatal intensive care have allowed many affected infants to survive, with subsequent slow improvement in function throughout childhood and teens [Campbell et al., 2004; Harper, 2001]. During the first 2 years of life, children with congenital myotonic dystrophy are at increased risk for aspiration pneumonitis and difficulties with feeding. Mental retardation and learning disabilities are common in congenital myotonic dystrophy. Determining the degree of cognitive deficit requires careful evaluation because these patients have marked facial weakness and are unable to speak and communicate well until mid- to late childhood. There is also an increased incidence of hearing loss [Harper, 2001]. These problems often complicate neuropsychologic testing. Figure 96-1A shows a mother with two daughters, both of whom have congenital myotonic dystrophy. Both children have delayed ability to speak and cognitive impairment. Their mother has mild ptosis, minimal grip myotonia, and minimal distal weakness. She is fully employed and has no cognitive impairment. This mother has the classic adult-onset form of DM1 [Harper, 2001].
The later, childhood-onset form usually is evident from nonmuscular manifestations of the disease, such as intellectual deficiency, difficulty with speech or hearing, clumsiness, or, rarely, with a cardiac dysrhythmia or postoperative apnea [Harper, 2001; Sinclair and Reed, 2009]. DM1 patients may have an increased sensitivity to sedative medications, especially barbiturates and opiates. and occasionally experience increased muscle stiffness/myotonia during general anesthesia [Harper and van Engelen, 2004; Sinclair and Reed, 2009]. Cardiac arrhythmias are more common complications of the adult-onset forms of DM1 [Breton and Mathieu, 2009; Groh et al., 2008; Harper, 2001]. However, other nonmuscular manifestations, such as gastrointestinal hypomotility with chronic constipation, and intermittent urinary tract symptoms, such as urgency or frequent urination, are common in childhood myotonic dystrophy [Harper, 2001; Harper and van Engelen, 2004].
Genetics
DM1 results from an unstable trinucleotide expansion in the 3′ untranslated region of a gene on chromosome 19 that codes for a serine/threonine protein kinase, DMPK [Brook et al., 1992; Fu et al., 1992; Mahadevan et al., 1992]. The exact function of this kinase remains unclear. The cause of the unstable CTG repeat expansion also is unknown. Recent research suggests that premutations arise from the upper normal range of CTG repeat region sizes and become pathologically enlarged within a few generations [Abbruzzese et al., 2002; Martorell et al., 2001]. Past investigations demonstrate that mitomycin C can enhance enlargement of pathologically expanded repeats and promote enlargement of normal repeats [Pineiro et al., 2003]. Inhibitors of replication also modulate instability of the CTG repeat in the DM1 gene in cultured cells [Yang et al., 2003]. These observations raise the possibility that environmental/external factors may influence the development of pathologic enlargement of CTG repeats at the DM1 locus. Recent investigations suggest that DNA “repair” proteins may also have a mutagenic role in CTG repeat instability [Slean et al., 2008; Pearson et al., 2005]. The normal size of the [CTG]n repeats ranges from 5 to 37 repeats. Children with the congenital form of DM1 have expansions typically greater than 1500 repeats [Harper, 2001]. Moderately affected adults with DM1 have [CTG]n sizes ranging from 300 to 1000 repeats, and mildly affected individuals have expansions from 50 to 200 repeats. There are no examples of point mutations in the DMPK gene locus that have caused the clinical picture of DM1. The same is true for DM2.
The unstable expansion of the DM1 gene provides an explanation for the well-described phenomenon of anticipation: that is, the earlier onset of more severe manifestations of disease in successive generations. Figure 96-1 emphasizes the phenomenon of anticipation in the mother with mild to moderate DM1 has had two children with severe congenital DM1.
Pathophysiology
The likely molecular pathomechanism that underlies both DM1 and DM2 relates to the nuclear accumulation of RNA containing the abnormally expanded repeats (CUG in DM1 and CCUG in DM2). These accumulations of RNA are unable to exit from the nucleus and undergo normal removal [Osborne et al., 2009; Wheeler, 2008]. The abnormal accumulation of the expanded repeat-containing RNA alters the normal functions of specific nuclear regulatory proteins, such as muscleblind [Mankodi and Thornton, 2002; Mankodi et al., 2003] and CUGBP1 [Timchenko et al., 2002; Savkur et al., 2001; Charlet et al., 2002]. The net effect is a novel toxic RNA disease mechanism that causes abnormal splicing of pre-mRNA in both DM1 and DM2 [Osborne et al., 2009; Wheeler, 2008]. The toxic RNA hypothesis builds heavily upon studies of animal models, as well as on findings in human tissues. The normal DMPK gene contains a short CTG repeat in the 3′ untranslated region of exon 15, and expression of this gene produces an mRNA containing a short CUG repeat [Wheeler, 2008]. The normal zinc finger protein 9 gene (ZNF9 gene), which contains, in intron 1, a large CCTG expansion that is responsible for DM2 [Liquori et al., 2001], and normally contains a short CCTG repeat in intron 1, gives rise to a pre-mRNA containing a CCUG repeat [Wheeler, 2008]. Due to removal of the introns during the editing of pre-mRNA, the CCUG repeat tract is absent from the ZNF9 mRNA [Margolis et al., 2006]. In the presence of nonexpanded CUG and CCUG repeats, the activities of muscleblind nuclear regulatory protein 1 (MBNL1) and the CUGBP1 protein are normal [Wheeler, 2008]. This allows normal regulation of pre-mRNA splicing by these nuclear regulatory proteins and normal production of the proper proteins in the cell [Wheeler, 2008].
The misregulation that occurs in DM1 and DM2 causes a “spliceopathy” that affects at least two-dozen transcripts [Osborne et al., 2009; Wheeler, 2008]. Myotonia caused by a marked reduction in the synthesis of the adult form of the skeletal muscle chloride channel [Charlet et al., 2002; Mankodi et al., 2002], and insulin resistance caused by deficient synthesis of the adult form of the insulin receptor [Savkur et al., 2001, 2004] occur in DM1 and DM2 as a result of this splicing defect, and it is likely that additional disease manifestations result from this alteration. In DM1, not DM2, excess phosphorylation of CUGBP1 occurs and results in higher total levels of this protein, gain of function, and in turn perhaps leads to the more pronounced muscle wasting often seen in DM1 [Wheeler, 2008].
The cause of the muscle wasting and weakness in DM1 remains a mystery. In the transgenic mouse model developed by Mankodi and colleagues, the mice do not develop muscle wasting or significant weakness [Mankodi et al., 2000, 2002]. In DM1 patients, there are hormonal abnormalities, including testosterone deficiency, insulin resistance, hyperinsulinemia, and altered release of growth hormone, which may contribute to the muscle wasting. To date, however, therapeutic trials restoring or exceeding physiologic levels of these hormones have failed to reverse weakness and wasting [Harper, 2001; Moxley et al., 2004].
Recently, two new mouse models have been developed and provide encouraging new discoveries related to skeletal muscle wasting and cardiac disease in DM1. One study describes an inducible mouse model of severe skeletal muscle wasting that expresses large tracts of CTG repeats in DMPK exon 15 [Orengo et al., 2008]. The results indicate that increased CUGBP1 protein levels are associated with increased DMPK-CUG RNA expression, and suggest a role for CUGBP1-specific splicing or cytoplasmic functional alterations as causes of muscle wasting [Orengo et al., 2008]. The investigators found that pentamidine partially rescues the defect in splicing of two pre-mRNAs (the skeletal muscle chloride channel and Serca1 pre-mRNA) in this mouse model [Orengo et al., 2008], raising the possibility of future therapeutic trials with pentamidine.
Another recent study demonstrates that, in addition to the sequestration of MBNL1 by the toxic RNA CUG repeat tract in DM1, these expanded CUG tracts activate the protein kinase C (PKC) signaling pathway, which leads to hyperphosphorylation and stabilization of CUGBP1 in DM1 heart and skeletal muscle tissues [Wang et al., 2009]. This elevation of hyperphosphorylated CUGBP1 may have an important pathophysiological role in causing cardiac muscle dysfunction in DM1. To explore this possibility, the investigators used an inducible DM1 mouse model, in which a transgene containing the last exon of DMPK with 960 CTG repeats is induced to express CUG repeat-containing RNA [Wang et al., 2009]. These mice exhibited higher mortality, conduction abnormalities, and systolic and diastolic dysfunction, as well as molecular changes typical of patients with DM1. One group of these mice received PKC inhibitors and exhibited increased survival that correlated with decreased phosphorylation and decreased steady-state levels of CUGBP1. Treatment with PKC inhibitors also reduced misregulation of splicing that was normally regulated specifically by CUGBP1, but not those splicing events regulated by MBNL1 [Wang et al., 2009]. The investigators suggest that pharmacological blockade of PKC activity mitigates the DM1 cardiac phenotype and they propose that the PKC pathway plays an important role in the pathogenesis of DM1. More investigation is necessary to confirm and extend these findings.
Clinical Laboratory Tests
Identification of the abnormal expansion of [CTG]n repeats in the DMPK gene on chromosome 19 establishes the diagnosis of DM1. Electromyography to detect myotonia is helpful in older children, but myotonia may be absent in infancy or in early childhood. Slit-lamp examination to identify the typical iridescent, spokelike posterior capsular cataracts is helpful. Muscle biopsy is not necessary to diagnose DM1 but may be useful to exclude other disorders. Careful examination of the mothers of patients with suspected congenital DM1 may reveal subtle clinical findings. Muscle pathology often demonstrates prominent central nuclei, scattered angular fibers, pyknotic nuclear clumps, and type 1 fiber atrophy. Creatine kinase levels may be normal or elevated and are not of primary value in the diagnosis [Heatwole et al., 2006]. With the discovery of the gene defect in DM1, it is possible to perform both prenatal and perinatal genetic counseling. Analysis of the DNA isolated from amniocytes or chorionic villus samples can predict the delivery of affected infants and the severity of illness [Harper, 2001; Harper and van Engelen, 2004].
Treatment
Treating patients with congenital myotonic dystrophy involves ventilatory support, use of a feeding tube, bracing for clubfoot deformities when present, and occasional corrective surgery for foot deformities (Figure 96-2) [Harper, 2001; Harper and van Engelen, 2004; Canavese and Sussman, 2009]. Figure 96-2 shows the molded plastic orthosis that helps treat foot deformity later in childhood. Speech problems and abdominal pain may improve with antimyotonia treatment. Mexiletine is sometimes helpful in alleviating some of the symptoms, especially in later childhood or adolescence when patients may have recurrent dislocation of the mandible, and pain and muscle spasm in the masseter muscles. Electrocardiographic monitoring is necessary on a regular basis, particularly during treatment with mexiletine, to search for covert dysrhythmias [Christensen et al., 2008; Otten et al., 2009; Gorog et al., 2005]. Treatment should be coordinated with the child’s school to ensure that cognitive deficiencies and hearing deficits are monitored. The increased risk for cardiac dysrhythmia and apnea after administration of general anesthesia for surgery requires overnight hospitalization with monitoring [White and Bass, 2003; Sinclair and Reed, 2009]. Apnea can develop several hours after a patient has been extubated [Harper and van Engelen, 2004]. No specific limitation on physical activity is necessary during childhood, provided the child has gained sufficient strength and coordination to carry out activities requested in the school environment.
Myotonic Dystrophy Type 2 (Formerly Proximal Myotonic Myopathy)
Clinical Features
DM2/PROMM typically appears in adult life and has variable manifestations, such as early-onset cataracts (younger than 50 years), varying grip myotonia, thigh muscle stiffness, and muscle pain, as well as weakness (hip flexors, hip extensors, abdominal muscles, or long flexors of the fingers) [Day et al., 2003; Moxley et al., 2002; Ricker et al., 1994a, 1995; Schoser et al., 2004b; Thornton et al., 1994]. Symptoms often appear between 20 and 70 years of age, and patients, as well as their care providers, ascribe them to overuse of muscles, “pinched nerves,” “sciatica,” arthritis, fibromyalgia, or statin use [George et al., 2004]. Younger patients may complain of stiffness or weakness when running up steps. In general, DM2 muscle pain has no consistent relationship to exercise or to the severity of myotonia found on clinical examination. The pain, which tends to come and go without obvious cause, usually fluctuates in intensity and distribution over the limbs. It can last for days to weeks. This pain seems qualitatively different from the muscle and musculoskeletal pain that occurs in patients with DM1.
Early in the presentation of DM2 there is only mild weakness of hip extension, thigh flexion, and finger flexion. Myotonia of grip and thigh muscle stiffness vary from minimal to moderate severity over days to weeks. Direct percussion of forearm extensor and thenar muscles is the most sensitive clinical test for myotonia in DM2 but may be absent. Myotonia of grip is sometimes prominent and often has a jerky quality that seems to differ from that in DM1 and the nondystrophic myotonias. Myotonia is often less apparent in DM2, compared to patients with DM1. In cases of late-onset DM2, myotonia may only appear on electromyographic testing after examination of several muscles [Day et al., 2003; Ricker et al., 1995]. Facial weakness is mild in DM2, as is muscle wasting in the face and limbs. Weakness of neck flexors is frequent. Trouble arising from a squat is common, especially as the disease progresses. Calf-muscle hypertrophy occasionally is prominent [Ricker et al., 1994a, 1995; Thornton et al., 1994]. Other manifestations, such as excessive sweating, hypogonadism, glucose intolerance, dysphagia, cardiac conduction disturbances, and cognitive/ neuropsychologic alterations, may also occur and worsen over time [Day et al., 2003; Meola et al., 1999, 2003; Schoser et al., 2004c]. A higher rate of premature labor and preterm deliveries also has been reported [Rudnik-Schoneborn et al., 2006].
At present there is no clear evidence of a congenital form of DM2 [Day et al., 2003]. However, there is evidence of anticipation, with earlier onset of more prominent symptoms, in studies of parent-child pairs [Schneider et al., 2000]. When symptoms occur in childhood, they typically are transient and relate to grip myotonia in the hands and thighs. Proximal weakness, muscle pain, cataracts, and occasional cardiac dysrhythmias usually do not develop until mid- or late adult life [Ricker et al., 1995; Thornton et al., 1994].
Genetics
DM2 results from an unstable four-nucleotide repeat expansion, CCTG, in intron 1 of the zinc finger protein 9 gene on chromosome 3q21 [Bachinski et al., 2003; Liquori et al., 2001, 2003]. The cause of the unstable expansion is unknown. The size of the CCTG repeat appears to increase over time in the same individual, and, like DM1, it is a dynamic gene defect [Day et al., 2003]. The size of the CCTG repeat in normal leukocyte DNA is less than 75 repeats [Day et al., 2003; Liquori et al., 2001]. The size in DM2 ranges from 78 to over 11,000 repeats [Day et al., 2003; Liquori et al., 2001]. The gene mutation responsible for DM2 appears to have arisen from a northern European founder [Bachinski et al., 2003; Liquori et al., 2003].
Pathophysiology
The molecular mechanisms leading to the manifestations of DM2 are believed to be similar to that in DM1, and relate to a toxic effect of the abnormally expanded RNA that accumulates in the muscle nuclei (see previous section on Pathophysiology of DM1). There is no apparent relationship between the putative functions of the zinc finger protein 9 gene at 3q21 and the DM1 gene at 19q13.3 or their gene products [Liquori et al., 2001]. Flanking genes are not similar for the DM2 and DM1 loci. A toxic effect of the ribonucleic acid made from the unstable, abnormally expanded, nucleotide repeats in the DM2 and DM1 genes is likely to serve as the common mechanism for their disease manifestations.
The cause of the weakness in DM2 is unclear. Patients with DM2, in contrast to patients with classic DM1, usually have only mild muscle wasting. However, there is an uncommon, adult-onset variant of DM2, termed proximal myotonic dystrophy [Udd et al., 1997], which causes severe wasting of proximal arm and thigh muscles as the illness progresses. Patients with the proximal myotonic dystrophy variant of DM2 have the identical CCTG repeat expansion at 3q21 that occurs in patients with DM2 [Bachinski et al., 2003]. The reason for the severe muscle wasting in this variant, compared with DM2 patients, is unknown. Whether individuals with proximal myotonic dystrophy [Udd et al., 1997] share an underlying mechanism responsible for muscle wasting that is similar to the mechanism in DM1 remains to be established. Two recent reports using cell models describe a reduction in the rate of protein translation in DM2 muscle cells, and propose that the accumulation of RNA containing long tracts of CCUG dysregulates translation and degradation of proteins in patients [Salisbury et al., 2009; Huichalaf et al., 2009]. More research is needed to confirm and extend these initial observations.
Clinical Laboratory Tests
Leukocyte DNA testing is available for DM2. DNA testing methodology used in the recent past to screen for DM2 may have failed to detect as many as 20 percent of affected individuals [Day et al., 2003]. Current methods use the more sensitive DNA testing methodologies for DM2 [Bachinski et al., 2003; Day et al., 2003].
Patients with elevated creatine kinase values may have greater postoperative complications [Ricker et al., 1995]. A recent comparison of muscle biopsy findings in DM2 with those in classic DM1 indicates that there is less severe atrophy of fibers in DM2, and that in DM2 there are subgroups of very small type 2 fibers and nuclear clumps [Vihola et al., 2003]. Both DM2 and DM1 display abundant central nuclei on their biopsies [Day et al., 2003; Harper, 2001; Vihola et al., 2003], but it appears that DM2 is predisposed to type 2 fiber atrophy and DM1 to type 1 fiber atrophy [Cardani et al., 2009; Pisani et al., 2008; Bassez et al., 2008; Schoser et al., 2004a; Vihola et al., 2003].
Treatment
In general, the management of DM2 is similar to that of DM1, but there is less need for supportive care, such as bracing, scooters, or wheelchairs. Cataracts require monitoring, and serial monitoring with an electrocardiogram (EKG) is necessary to check for covert dysrhythmia. Disturbances in cardiac rhythm are less frequent in DM2, but abnormalities do occur [Wahbi et al., 2009; Schoser et al., 2004b; Day et al., 2003; Moxley et al., 2002]. Hypogonadism and insulin resistance need monitoring, as in DM1. Myotonia tends to be less marked and less troublesome in DM2 but, in specific circumstances, antimyotonia therapy is helpful, especially if muscle stiffness is frequent and persistent or if pain is prominent. Cognitive difficulties also occur in DM2, as in DM1, but become manifest in adult life and appear to be associated with decreased cerebral blood flow to frontal and anterior temporal lobes [Meola and Sansone, 2007; Meola et al., 1999] and decreased brain volume [Akiguchi et al., 1999; Chang et al., 1998]. The changes are less severe than in DM1. Their etiology is unknown but may relate to the toxic effect of intranuclear accumulations of abnormally expanded RNA. Management of these brain symptoms is similar to that for DM1.
A frequent and difficult problem in DM2 is the peculiar muscle pain described earlier [Auvinen et al., 2008; George et al., 2004]. The exact mechanism underlying the pain is unknown, and there is no well-established, effective treatment. Carbamazepine or mexiletine, along with nonsteroidal anti-inflammatory medications or acetaminophen ameliorates this pain in some patients.
Experimental Therapeutics in Myotonic Dystrophy
Evaluating the differences and similarities between DM1 and DM2, both clinically and in their molecular mechanisms, presents opportunities for the development of potential new treatments and provides hope to researchers and patients that treatment trials may not be far away. Support for this optimistic statement comes from recent studies in mouse models of DM1 that include a demonstration of reversal of the spliceopathy. Treatment of transgenic mice that over-express CUG transcripts containing 250 repeats with an antisense 25-nucleotide morpholino oligonucleotide composed of CAG repeats led to the dispersal of the nuclear foci that contained accumulations of toxic RNA and freed up the nuclear regulatory protein, MBNL1 [Wheeler et al., 2009]. This treatment also reversed the spliceopathy and led to restoration of normal synthesis of the proper amount of the adult form of the skeletal muscle chloride channel. Myotonia also disappeared in the treated mice [Wheeler et al., 2009]. In another study, researchers used a myoblast-myotube cell model system of DM1 cells containing 300 CTG repeats and found that treatment with a 2′-O-methyl-phosphorothioate-modified (CAG)7 antisense oligonucleotide silenced mutant DMPK RNA expression and reduced the number of ribonuclear aggregates [Mulders et al., 2009]. Use of this same antisense oligonucleotide in vivo in a DM1 mouse model caused a significant reduction in the level of toxic (CUG) RNA and corrected the defect in splicing of pre-mRNA [Mulders et al., 2009]. In the future, it may be possible to develop a double-pronged therapeutic approach that combines antisense oligonucleotide treatment to free up sequestered MBNL1 nuclear regulatory protein from the toxic CUG repeat tract, and another antisense oligonucleotide that facilitates degradation of toxic RNA. Antisense oligonucleotides are already in clinical trials to treat other diseases, including Duchenne muscular dystrophy and familial hypercholesterolemia, and DM1 may soon be added to this list.
Autosomal-Dominant and Autosomal-Recessive Myotonia Congenita
Thomsen’s disease (autosomal-dominant myotonia congenita) and Becker’s disease (autosomal-recessive myotonia congenita) represent two forms of chloride channelopathies (Table 96-2). The chloride channelopathies that produce myotonia as their primary symptom resemble the sodium channel disorders without periodic paralysis, and may resemble mild forms of DM1 and DM2. Other causes of muscle stiffness or poor coordination, including central nervous system diseases affecting the frontal lobes, brainstem, and cerebellum, require initial consideration but are confused rarely with these channelopathies.
Clinical Features | Autosomal-Dominant Myotonia Congenita of Thomsen | Autosomal-Recessive Generalized Myotonia of Becker |
---|---|---|
Inheritance | Dominant | Recessive |
Gene defect | Chromosome 7; mutation in skeletal muscle chloride channel | Chromosome 7; mutation in skeletal muscle chloride channel |
Age of onset | Infancy to early childhood | Late childhood, occasionally starts earlier or begins in teens |
Myopathy | Muscle hypertrophy frequent; no myopathy, although variants uncommonly develop weakness | Occasional muscle wasting and weakness can occur late; hypertrophy of muscles frequently occurs in legs |
Myotonia | Generalized stiffness, especially after rest; improves with exercise; prominent myotonia of eye closure, but not paradoxical myotonia | Generalized stiffness, especially after rest; transient weakness is prominent after complete relaxation for several minutes; myotonia occurs in eyes; no paradoxical myotonia |
Provocative stimuli | Prolonged rest or maintenance of the posture | Prolonged rest or maintenance of the same posture |
Therapy for symptoms | Exercise; antimyotonia therapy (e.g., mexiletine); Achilles’ tendon stretching helps prevent need for heel cord-lengthening surgery | Exercise; especially avoiding prolonged rest; antimyotonia therapy (e.g., mexiletine); transient weakness does not improve after mexiletine |
Clinical Features
Generalized myotonia is the major clinical symptom in dominant and recessive forms of chloride channel myotonia congenita. Recent studies indicate that pain occurs in almost 30 percent of patients with chloride channel myotonia [Trip et al., 2009a], and as many as 75 percent have mild generalized muscle weakness that is slightly greater proximally [Trip et al., 2009a; Shalata et al., 2009; Bernard et al., 2008]. The pain has a close relation to fatigue and has a negative impact on the health status of those affected [Trip et al., 2009a]. Symptoms develop in the first or second decade of life. Myotonic stiffness occurs with sudden physical exertion after a period of rest. Repeated muscle contractions ameliorate the stiffness. This response is the “warm-up phenomenon,” which helps distinguish chloride channel myotonia congenita from certain forms of sodium channel myotonia that display increasing muscle stiffness with repeated contractions, a paramyotonic response. DM1 and DM2, like chloride channel myotonia, demonstrate the typical warm-up response.
In contrast to Thomsen’s disease, patients with autosomal-recessive myotonia congenita (Becker’s disease) have transient proximal muscle weakness. The transient weakness appears for a few seconds during the initial attempt at a specific movement after a period of inactivity [Zwarts and VanWeerden, 1989]. Muscle strength improves to normal after several strong contractions [Baumann et al., 1996; Zwarts and VanWeerden, 1989]. One study suggests that this transient weakness represents a transient depolarization block associated with prolonged recovery of muscle membrane excitability [McKay et al., 2006].
Genetics
Point mutations in the gene for the skeletal muscle chloride channel cause both autosomal-dominant [Trip et al., 2008, 2009b; Fialho et al., 2007; Fahlke et al., 1997a; Koch et al., 1992] and autosomal-recessive [Trip et al., 2008, 2009b; Fialho et al., 2007; Koch et al., 1992] forms of myotonia congenita. Autosomal-dominant myotonia congenita (Thomsen’s disease) may vary considerably in age of onset and severity of myotonia [Duno et al., 2004; Koty et al., 1996]. Two recent reports describe marked myotonia, generalized moderate weakness, and generalized muscle hypertrophy in homozygous individuals in kindreds with dominant chloride channel myotonia [Bernard et al., 2008; Shalata et al., 2009]. These results demonstrate a dose effect with disease severity. It is now possible to screen for known point mutations in the chloride channel gene on chromosome 7 [Trip et al., 2008, 2009b; Fialho et al., 2007], and extensive genetic testing for more than 80 mutations is available in two laboratories, one in the United Kingdom and one in the Netherlands. The group in the Netherlands recommends a tandem analysis for gene mutations. They first perform DNA analysis of all 23 exons in the skeletal muscle chloride channel gene, and then proceed with DNA analysis of those individuals who test negative for chloride channel mutations, with probing of the exons in the skeletal muscle sodium channel gene known to cause sodium channel myotonia [Trip et al., 2008]. This approach has proven very informative in establishing a diagnosis.
Pathophysiology
Electrophysiologic studies in myotonic goats [Lipicky and Bryant, 1993] and human muscle tissue from patients with autosomal-dominant and autosomal-recessive [Koch et al., 1992; Rudel et al., 1994] myotonia congenita demonstrate a decreased chloride conductance. The decreased chloride conductance across the transverse tubular system renders the muscle membrane hyperexcitable, which leads to after-depolarization and repetitive firing, creating clinical myotonia. Both autosomal-dominant myotonia congenita (Thomsen’s disease) and generalized autosomal-recessive myotonia congenita (Becker’s disease) involve mutations at the same locus [George et al., 1993; Koch et al., 1992]. Current models for the chloride channel indicate that two subunits combine to form a double-barreled entry gate for chloride ions and a single outflow channel [Duffield et al., 2003; Fahlke et al., 1997a, 1997b; Saviane et al., 1999]. Dominant mutations affect the common outflow channel (slow-flow channel), and recessive mutations require involvement of the two faster-flow entry gates [Duffield et al., 2003]. The model proposes that involvement of only one entry gate does not cause clinical disease. Recessive mutations cause a pathologic reduction in chloride current because both fast gates undergo blockade, and dominant mutations cause pathologically reduced current because they affect the slower common exit channel for chloride ions. Recessive mutations are much more frequent than dominant mutations. Over 80 mutations are known to cause chloride channel myotonia [Colding-Jorgensen, 2005]. More than 50 percent of chloride channel mutations occur in exons 3, 8, and 11 [Fialho et al., 2007; Trip et al., 2008]. The variations in the clinical severity of certain very rare, dominantly inherited forms of chloride channel myotonia, such as “myotonia levior” [Ryan et al., 2002] and “fluctuating myotonia” [Wagner et al., 1998], are interesting, and point to limitations in our current version of the model as an explanation for the clinical spectrum of findings. A more recent report further emphasizes the limitation of the model. This report describes two unrelated families with the Becker recessive form and two unrelated families with the Thomsen dominant form of myotonia congenita, all of whom have a common R894X mutation [Duno et al., 2004]. The dominant family with the most severe phenotype expressed twice the expected amount of the R894X mRNA allele. These findings suggest that allelic variation may be an important modifier of disease progression in myotonia congenita. One report of a family with Thomsen myotonia congenita describes a kindred with a T310M mutation and notes that family members with one mutated allele have mild manifestations, while the one family member who was homozygous for this mutation had severe stiffness, generalized muscle hypertrophy, and moderate weakness [Bernard et al., 2008]. Another more recent report also describes a gene dose effect, with worse disease severity (i.e., more severe myotonia and generalized weakness) in homozygous individuals who have a missense mutation of glycine to serine (G190S) in a well-conserved motif in helix D of the skeletal muscle chloride channel gene [Shalata et al., 2009].
The model for the chloride channel also does not provide an explanation for the transient weakness that is a prominent clinical feature in generalized autosomal-recessive (Becker’s myotonia) myotonia congenita. Its cause remains to be discovered. The model fails, as well, to provide an explanation for transient worsening that occurs occasionally during pregnancy. One potential clue comes from recent in vitro studies of testosterone and progesterone, which show rapid and reversible inhibition of both the normal and the mutated forms of skeletal muscle chloride channels expressed in Xenopus oocytes, caused by a prominent rightward shift in the voltage dependence of their open probability [Fialho et al., 2008]. Further study using more physiologic concentrations of these sex hormones in a more appropriate cell culture model, such as myoblasts or myotubes, is needed to establish the physiological significance of this effect.
Clinical Laboratory Tests
Distinguishing patients with chloride channel myotonia, either autosomal-dominant or autosomal-recessive forms, from those with sodium channel myotonic disorders is more difficult. Both cause myotonic stiffness and muscle hypertrophy, especially in the legs. One distinguishing feature of sodium channel myotonia is the paradoxical myotonia of the eyelids that develops with repeated forceful eye closure. Figure 96-3 shows the response to looking up and down and to eye closure in a patient with DM1. The patient in Figure 96-4 has sodium channel myotonia, exhibiting lid lag and persisting myotonia after forceful closure of the eyes. If this patient repeats the forceful closure of his eyes, his myotonia worsens. Persistent myotonia of the eyelids also occurred in this patient’s son. In contrast, patients with autosomal-dominant and autosomal-recessive forms of chloride channel myotonia exhibit warm-up after repeated contractions. One recent study comparing 32 patients with chloride channel myotonia to 30 patients with sodium channel myotonia observed the following differences between these two groups of patients [Trip et al., 2009b]. Compared to patients with sodium channel myotonia, patients with chloride channel myotonia have a higher frequency of: muscle weakness, 75 versus 37 percent; warm-up following repeated 3-second isotonic maximum grips, 100 versus 47 percent; and slowing in the time to arise from sitting to standing, 91 versus 67 percent. In contrast, compared to patients with chloride channel myotonia, patients with sodium channel myotonia had higher frequencies of: paradoxical myotonia, 50 versus 0 percent; and painful myotonia, 57 versus 28 percent [Trip et al., 2009b]. Patients with sodium channel myotonia also had earlier onset of symptoms: 4.4 years versus 10 years of age. Patients with chloride channel myotonia do not have a worsening of myotonic stiffness or paralysis after prolonged exposure of muscle to cold. To search for cold-induced paralysis, it is necessary to soak the hand or forearm muscles in cold water (15°C) for 15–20 minutes. If there is weakness with exercise after this cold exposure, this observation strongly favors sodium channel myotonia rather than chloride channel disease [Heatwole and Moxley, 2007; Carle et al., 2009; Webb and Cannon, 2008]. The subsequent section on sodium channel myotonia briefly describes the laboratory evaluation of the sodium channelopathy disorder, hyperkalemic periodic paralysis. Recent reports have provided protocols for complete screening of both the skeletal muscle chloride and sodium channel genes [Trip et al., 2008; Fialho et al., 2007]. The approach uses tandem analysis of the chloride channel gene, followed by analysis of the sodium channel gene, in individuals who already have had negative DNA analysis for DM1 and DM2, and have a negative history for periodic paralysis.
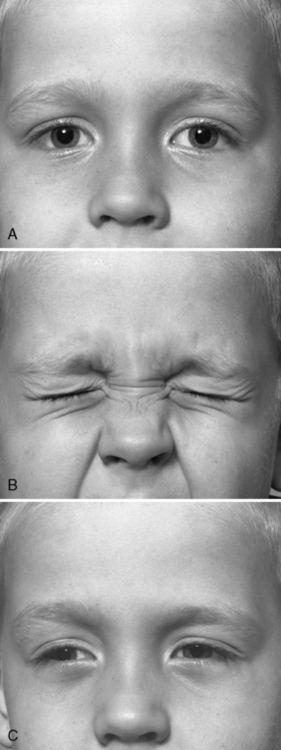
Fig. 96-4 A patient with acetazolamide-responsive sodium channel myotonia.
The patient appears in Figure 96-1 with his father, who is also shown in Figure 96-3. Sequential photographs of the patient before and after forced closure of the eyes. Myotonia appears and worsens after repeated eye closure (e.g., paramyotonia).
Treatment
Antimyotonia treatment with mexiletine is often helpful [Heatwole and Moxley, 2007]. Before treatment with mexiletine is initiated, a baseline EKG is necessary to identify patients with unsuspected cardiac conduction abnormalities. Side effects of mexiletine, which are usually mild and dose-related, include dysgeusia, lightheadedness, and diarrhea. Tocainide, another lidocaine derivative similar to mexiletine, was another useful alternative [Kwiecinski et al., 1992], but it is no longer available. Isolated reports suggest that carbamazepine may be a readily available alternative antimyotonia medication for myotonia congenita [Berardinelli et al., 2000; Sheela, 2000]. Children with moderately severe myotonia develop heel-cord shortening and contractures at their elbows. If these contractures do not respond to stretching and other physical therapy exercises, it is appropriate to initiate antimyotonia treatment, even when patients do not complain of stiffness.
Acetazolamide-Responsive Sodium Channel Myotonia and Myotonia Fluctuans
Two disorders that mimic myotonia congenita and are forms of sodium channel myotonia without periodic paralysis include acetazolamide-responsive sodium channel myotonia and myotonia fluctuans [Heatwole and Moxley, 2007] (Table 96-3).
Table 96-3 Sodium Channel Myotonias without Periodic Paralysis
Clinical Features | Acetazolamide-Responsive Sodium Channel Myotonia | Myotonia Fluctuans |
---|---|---|
Inheritance | Dominant | Dominant |
Gene defect | Chromosome 17; mutation in skeletal muscle sodium channel | Chromosome 17; mutation in skeletal muscle sodium channel |
Age of onset | First decade | First or second decade |
Myopathy | Rare | Rare, muscle hypertrophy common |
Myotonia | Face, paraspinal muscles, paradoxical myotonia of eyelids, grip limbs; varies in severity and often there is pain with myotonia | Face, limbs, eyelids; frequently fluctuates in severity; especially after exercise |
Provocative stimuli | Fasting, cold, oral potassium, infection | Exercise-rest-exercise, oral potassium |
Therapy for symptoms | Acetazolamide, mexiletine; avoid high-potassium diet; monitor during and after surgery for rigidity and rhabdomyolysis | Mexiletine; avoid high-potassium diet; monitor during and after surgery for rigidity and rhabdomyolysis |
The discovery that hyperkalemic periodic paralysis and paramyotonia congenita result from mutations in the gene for the skeletal muscle sodium channel [Cannon, 2002; Lehmann-Horn and Jurkat-Rott, 1999; Jurkat-Rott and Lehmann-Horn, 2007] has led to further investigations that have identified a group of patients with myotonia without episodic weakness, whose myotonia is worsened by potassium intake and who have mutations in the gene for the skeletal muscle sodium channel [Lerche et al., 1993; Ptacek et al., 1994a; Ricker et al., 1990, 1994b; Heatwole and Moxley, 2007; Colding-Jorgensen et al., 2006; Gay et al., 2008; Kubota et al., 2009]. This section reviews two of these disorders, acetazolamide-responsive myotonia [Ptacek et al., 1994b] and myotonia fluctuans [Ricker et al., 1990, 1994b], which resemble chloride channel myotonia. A review of two other rare nondystrophic myotonic disorders caused by mutations in the sodium channel, myotonia permanens and chronic myotonia [Lerche et al., 1993; Colding-Jorgensen et al. 2006], is not included. The reader is referred to the reports describing these uncommon disorders.
Clinical Features
Acetazolamide-responsive sodium channel myotonia often presents initially to the pediatrician rather than the neurologist with common complaints, such as clumsiness causing frequent falls, a lazy eye, growing pains, back muscle spasm, or stridor. Parents may observe that, after prolonged crying, an infant or young child will have “their eyes stuck.” This symptom is a manifestation of paradoxical eyelid myotonia that develops with repeated bouts of forceful closure of the eyes during crying. Myotonia is most apparent in the face, eyes, and larynx in very young patients. Stiffness in the hands, proximal limb muscles, and paraspinous muscles is more apparent in middle childhood. Muscle hypertrophy, especially in the legs, is frequent. Cerebellar function, sensory testing, tendon reflexes, and muscle strength are normal. Grip and percussion myotonia, as well as lid lag, are present. The severity of these complaints varies both in affected individuals within the same kindred and between kindreds. Episodes of painful muscle spasm occur in some patients, usually during or immediately after exercise. Pain is relatively infrequent in recessive and dominant chloride channel myotonias [Trip et al., 2009a – see section above on chloride channel myotonia]. In contrast, pain is common in acetazolamide-responsive sodium channel myotonia and DM2.
The clinical findings in myotonia fluctuans are similar to those described previously for acetazolamide-responsive sodium channel myotonia, with one important additional finding. The severity of the muscle stiffness fluctuates to a greater degree in this illness. On “good days,” the myotonia can vanish. The fluctuating myotonia also has an interesting relationship to prolonged exercise. A phenomenon termed exercise-induced delayed-onset myotonia occurs [Ricker et al., 1990]. Patients report that, after a period of rest following vigorous exercise, if they resume exercise “at the wrong time,” severe muscle stiffness may develop. The time interval between the period of rest and the resumption of exercise is critical. If it is only a few minutes or an hour or more, stiffness does not occur. During the intermediate time interval, the fluctuation and provocation of severe myotonia happen.
Genetics
Acetazolamide-responsive sodium channel myotonia results from a mutation in the gene for the skeletal muscle sodium channel [Ptacek et al., 1994b], as does myotonia fluctuans [Ricker et al., 1994b]. The mutations in these two disorders of the sodium channel are not identical. Separate mutations produce myotonia fluctuans [Ricker et al., 1994b]. Both disorders are autosomal-dominant.
Pathophysiology
Both of these nondystrophic sodium channel myotonic disorders worsen with an elevation in extracellular potassium. The myotonic stiffness can disable a patient totally under these circumstances. The specific mechanism by which the fluctuation in severity of myotonia occurs remains unclear, but it may relate in part to hormonal fluctuations. The levels of insulin, corticosteroids, and other hormones may exert an important influence on extracellular potassium levels [Ricker et al., 1994b].
Clinical Laboratory Tests
Routine DNA testing is not available; however, several research laboratories screen for known mutations in the gene for the sodium channel in selected patients [Trip et al., 2009b; Fialho et al., 2007; Fournier et al., 2006]. The remainder of the laboratory evaluation is as described previously for chloride channel myotonia.
Treatment
Acetazolamide often controls myotonic stiffness and pain in acetazolamide-responsive sodium channel myotonia [Heatwole and Moxley, 2007; Ptacek et al., 1994b]. This treatment usually ameliorates the muscle pain. Annual ultrasound of the abdomen is useful to detect early formation of kidney stones, which are a common complication of acetazolamide [Tawil et al., 1993]. Dysesthesia and dysgeusia may also occur with this medication. For attacks of severe muscle spasm and pain, cyclobenzaprine can be tried. Patients undergoing surgery require monitoring during and after the procedure for signs of worsening muscle stiffness. Maintaining plasma potassium levels between 3.8 and 4.2 mEq/L helps to decrease perioperative muscle stiffness. Stress, muscle tissue damage, and bleeding all elevate plasma potassium levels and worsen myotonia. Postoperatively, intravenous diazepam or lorazepam may be necessary to decrease myotonic stiffness, especially if patients are unable to take medications by mouth. Mexiletine is an effective alternative to acetazolamide and, if necessary, these drugs can be used in combination [Heatwole and Moxley, 2007].
Periodic Paralyses
This section focuses on those disorders listed in Tables 96-4 and 96-5, which are associated with periodic paralyses, including channelopathies affecting the sodium, potassium, and calcium channels in skeletal muscle. The general classification of the periodic paralyses is as primary (inherited) or secondary (acquired) forms, and according to the change in serum potassium level (i.e., hyperkalemic or hypokalemic). Paralytic attacks may last from less than 1 hour to several days. Weakness can be localized or generalized. Tendon reflexes decrease or disappear during attacks. Muscle fibers become unresponsive to either direct or indirect electrical stimulation. The generalized attacks of weakness usually begin in proximal muscles and spread to distal ones. Respiratory and cranial muscles tend to be spared but, occasionally, may become paralyzed. Rest after exercise tends to provoke weakness in the muscles that have undergone the exercise, but continued mild exercise may abort attacks. Exposure to cold may provoke weakness in certain sodium channel-related forms of periodic paralysis. Complete recovery usually occurs after attacks. Permanent weakness and irreversible pathologic changes sometimes occur.
Hyperkalemic Periodic Paralysis
Clinical Features
Table 96-4 outlines the major clinical and diagnostic features of hyperkalemic periodic paralysis, as well as important issues in management. All three forms are autosomal-dominant, with high penetrance in both sexes [Cannon, 2002; Lehmann-Horn and Jurkat-Rott, 1999; Moxley, 2000]. Attacks begin in childhood and usually are brief, being shorter and more frequent than attacks in hypokalemic periodic paralysis. The attacks can occur with or without myotonia and, in certain families, hyperkalemic paralysis occurs in combination with coexisting paramyotonia congenita [Jurkat-Rott and Lehmann-Horn, 2007; Heatwole and Moxley, 2007; Finsterer, 2008; Cannon, 2002; Lehmann-Horn and Jurkat-Rott, 1999; Moxley, 2000]. At the onset of an attack, myalgia may develop. Patients with hyperkalemic periodic paralysis plus myotonia frequently develop muscle stiffness and prominent paradoxical myotonia of the eyelids during attacks. Similar symptoms occur in patients with hyperkalemic periodic paralysis in association with paramyotonia congenita [Jurkat-Rott and Lehmann-Horn, 2007; Lehmann-Horn et al., 2004]. Most patients with hyperkalemic attacks manifest signs of myotonia and notice increasing muscle tension, especially in the paraspinous muscles.
Genetics
All clinical variants of hyperkalemic periodic paralysis result from mutations in the gene for the skeletal muscle sodium channel [Cannon, 2002; Lehmann-Horn and Jurkat-Rott, 1999; Miller et al., 2004; Ptacek et al., 1994b], but the exact mutation can differ, despite the fact that clinical symptoms are indistinguishable.
Pathophysiology
In hereditary primary hyperkalemic periodic paralyses with and without myotonia, the net movement of potassium is the opposite of that which occurs in hereditary hypokalemic periodic paralysis. There is an enhanced urinary excretion of potassium and sodium, a decrease in serum sodium concentration, and a decrease in or unaltered serum chloride level [Moxley, 1994]. These findings are consistent with the egress of potassium and entry of sodium into muscle cells during attacks. During attacks of weakness in both hyperkalemic periodic paralysis and in paramyotonia congenita, there is an abnormally increased sodium conductance, leading to depolarization of the muscle [Lehmann-Horn et al., 2004; Jurkat-Rott and Lehmann-Horn, 2007]. Patients with hyperkalemic periodic paralysis have a strong diurnal sensitivity to attacks of weakness, which occur primarily in the morning [Ricker et al., 1989]. This sensitivity may relate to circulating factors, such as glucocorticoids and beta-adrenergic hormones. Interestingly, salbutamol and metaproterenol, both beta-adrenergic drugs, are capable of increasing muscle strength during attacks [Lehmann-Horn et al., 2004; Heatwole and Moxley, 2007; Ricker et al., 1989].
Clinical Laboratory Tests
The primary laboratory evaluation involves monitoring of serum electrolytes during attacks to identify hyperkalemia or hypokalemia, and monitoring the EKG to search for any evidence of dysrhythmia or other conduction disturbance, such as the EKG changes that occur in Andersen–Tawil syndrome. A dominant family history helps to limit additional laboratory testing to a search for secondary forms of hyperkalemia, which might lead to muscle weakness. Laboratory evaluation for secondary hyperkalemic weakness should include a search for hormonal disturbances, such as insulin deficiency or adrenal insufficiency, or toxic effects of medications, such as beta-adrenergic antagonists, alpha-adrenergic agonists, digitalis intoxication, or the use of succinylcholine. Recently, a few research laboratories have established protocols for full gene screening for mutations in the skeletal muscle sodium, chloride, calcium, and potassium channel genes to search for a mutation to account for one of the forms of nondystrophic myotonia or one of the forms of periodic paralysis [Trip et al., 2009b; Fialho et al., 2007]. Genetic screening with DNA analysis needs to be pursued prior to performing provocative testing, since this testing requires hospitalization and monitoring by clinicians experienced in the management of attacks of periodic paralysis and their complications. In patients not observed during a spontaneous attack of weakness or posing a continuing problem in definite classification of the form of periodic paralysis, it may be necessary to perform provocative testing with oral potassium loading or exercise followed by potassium challenge [Moxley, 2000].
Treatment
Preventive treatment includes avoidance of fasting, exposure to cold, and over-exertion. A prudent consumption of frequent meals high in carbohydrate content is helpful. Diuretics that promote kaluresis, such as hydrochlorothiazide and carbonic anhydrase inhibitors, are good primary therapies [Moxley, 2000; Jurkat-Rott and Lehmann-Horn, 2007; Heatwole and Moxley, 2007; Sansone et al., 2008; Sansone and Tawil, 2007].
Paramyotonia Congenita
Clinical Features
Symptoms develop usually during the first decade of life and have a predilection for facial, lingual, neck, and hand muscles (see Table 96-4) [Lehmann-Horn et al., 2004; Lehmann-Horn and Jurkat-Rott, 1999; Moxley, 2000; Heatwole and Moxley, 2007]. Attacks of increased muscle stiffness followed by paralysis occur on exposure to cold followed by exercise. The hallmark clinical sign is paradoxical myotonia or paramyotonia: that is, stiffness that worsens with repeated muscle contraction. This sign is most prominent in the muscles of eye closure. Clinical myotonia may be restricted to the eye muscles and not be apparent in grip or after percussion. Recent reports describe severe variants with onset in infancy for some affected individuals with occasional deaths due to respiratory causes [Kubota et al., 2009; Gay et al., 2008]. These recent reports point out certain mutations in the sodium channel gene that cause earlier onset of enhanced sensitivity to cold-induced worsening of myotonia and weakness that contributes to a more severe phenotype [Carle et al., 2009; Kubota et al., 2009; Gay et al., 2008; Fournier et al., 2006]. Other mutations that cause cold exposure and exercise-sensitive myotonia have led to the onset of progressive proximal muscle weakness resembling DM2 later in life [Schoser et al., 2007]. These variants emphasize the need for caution in predicting the prognosis for disease severity and long-term course, until we have a more complete understanding of the genotype and phenotype in paramyotonia congenita.
Genetics
Mutations in the gene for the skeletal muscle sodium channel in several locations cause paramyotonia congenita [Matthews et al., 2010; Webb and Cannon, 2008; Carle et al., 2009; Kubota et al., 2009; Gay et al., 2008; Fournier et al., 2006; Cannon, 2002; Lehmann-Horn et al., 2004; Lehmann-Horn and Jurkat-Rott, 1999; Miller et al., 2004], but they tend to fall into the following two general groups:
Certain mutations cause characteristic patterns on short exercise testing before and after muscle cooling [Fournier et al., 2006]. The more severe, early-onset patients with paramyotonia mentioned above have mutations in the cytoplasmic loop between domains 3 and 4 [Gay et al., 2008], at the calcium-chelation loop of the EF-hand helix-loop motif of the C-terminus [Kubota et al., 2009], and a Q270K mutation on the outermost part of the S5 segment of domain I [Carle et al., 2009]. Patients with paramyotonia congenita associated with hyperkalemic periodic paralysis have mutations in the cytoplasmic loop between domains 3 and 4 [Jurkatt-Rott and Lehmann-Horn, 2007; Bouhours et al., 2004; Lehmann-Horn et al., 2004; Lehmann-Horn and Jurkat-Rott, 1999]. There is considerable variation in the severity of symptoms both within and between kindreds, and this variation does not correlate closely with the exact mutation [Jurkatt-Rott and Lehmann-Horn, 2007; Lehmann-Horn and Jurkat-Rott, 1999]. One recent report indicates that the combined form of hyperkalemic periodic paralysis and paramyotonia congenita, with the severe phenotype beginning in infancy, involves a specific mutation: a methionine substitution at codon 704 (T704M) [Brancati et al., 2003]. More studies are necessary to establish this phenotype–genotype correlation [Jurkatt-Rott and Lehmann-Horn, 2007; Matthews et al., 2010].
Clinical Laboratory Tests
Standardized testing, using immersion of the hand and forearm in cold water for 15 minutes followed by exercise, is effective in provoking paramyotonic stiffness and paralysis in most patients [Moxley, 2000; Ricker et al., 1990]. Most cooperative children can tolerate this testing. If no clear evidence of cold-induced muscle stiffness and weakness is apparent, further laboratory testing, as indicated previously under hyperkalemic periodic paralysis and as noted under laboratory tests for chloride channel myotonia, may be necessary. Repetitive nerve stimulation of the ulnar nerve at the wrist as a short exercise test before and after cold exposure helps to identify certain forms of sodium channel myotonia, including paramyotonia congenita, but it is uncomfortable and has been standardized only in adults [Fournier et al., 2006]. DNA analysis to search for known mutations in the gene for the sodium channel is available in selected research laboratories, and may become routinely available in the future [Matthews et al., 2010; Trip et al., 2009b; Jurkatt-Rott and Lehmann-Horn, 2007].
Pathophysiology
As in hyperkalemic periodic paralysis caused by sodium channel dysfunction, paramyotonia congenita also has increased sodium conductance and excessive depolarization of the muscle membrane [Webb and Cannon, 2008; Cannon, 2002; Bouhours et al., 2004; Lehmann-Horn and Jurkat-Rott, 1999]. The electrophysiologic defect in hyperkalemic periodic paralysis differs from that in paramyotonia congenita. This difference is apparent in the response of forearm muscle to cooling and exercise. In hyperkalemic periodic paralysis, cooling of forearm muscle, followed by forceful contractions, leads to mild weakness, but muscle fibers do not display the marked depolarization observed in the muscle of patients with paramyotonia congenita. Treatment with tocainide, which is effective in paramyotonia congenita, does not reduce the mild weakness provoked by cooling and exercise in hyperkalemic periodic paralysis, in contrast to its beneficial protective effect on forearm muscle strength in paramyotonia congenita [Rudel et al., 1994]. In paramyotonia congenita, exercise after exposure to cold provokes muscle stiffness and, occasionally, prolonged paralysis. Recent in vitro studies of a highly thermosensitive mutation causing paramyotonia congenita showed destabilization of mutant channel slow inactivation in combination with defective fast inactivation, which may predispose to prolonged membrane depolarization, in turn leading to membrane inexcitability and paralysis [Carle et al., 2009]. More research is necessary to clarify the mechanism of cold-induced weakness in paramyotonia and its variation within individuals in the same and different kindreds.
Treatment
Mexiletine usually is effective in preventing attacks of cold-induced weakness [Heatwole and Moxley, 2007; Moxley, 2000]. In vitro studies indicate that mexiletine, a class 1b antiarrhythmic [Wang et al., 2004], and flecanide, a class 1c antiarrhythmic [Desaphy et al., 2004], both act by producing an open channel block of persistent late sodium currents. Tocainide, another class 1b antiarrhythmic, is also effective, but is no longer available. Patients with the combination of paramyotonia congenita plus hyperkalemic periodic paralysis require additional treatment with either a thiazide diuretic or acetazolamide.
Hypokalemic Periodic Paralysis
Hypokalemic periodic paralysis is an autosomal-dominant disorder caused most commonly by mutations in the alpha subunit of the skeletal muscle calcium channel gene Cav1.1 [Matthews et al., 2009; Finsterer, 2008; Fontaine et al., 2007; Kim et al., 2007; Venance et al., 2006; Miller et al., 2004; Jurkat-Rott et al., 1994; Ptacek et al., 1994a]. A clinically identical form of hypokalemic periodic paralysis also can result from specific mutations of the alpha subunit of the Nav1.4 skeletal muscle sodium channel associated with hyperkalemic periodic paralysis [Jurkat-Rott et al., 2000; Struyk et al., 2000; Sternberg et al., 2001]. Hypokalemic periodic paralysis is the most common of the primary periodic paralyses, with approximately 60 percent of cases caused by missense mutations of the calcium channel gene, Cav1.1, and 15–20 percent caused by mutations in the sodium channel gene, Nav1.4. Up to 25 percent of mutations causing hypokalemic periodic paralysis remain unidentified [Fountaine et al., 2007; Jurkat-Rott et al., 1994; Ptacek et al., 1994a].
Clinical Features
Attacks of hypokalemic periodic paralysis usually have their onset in the first or second decade, and about 60 percent of patients are affected before the age of 16 years [Matthews et al., 2009; Kim et al., 2007; Venance et al., 2006; Chabrier et al., 2008; Moxley, 1994]. Rarely, attacks occur in infancy [Chabrier et al., 2008]. Initially, attacks tend to be infrequent but eventually may recur daily. Diurnal fluctuations in strength may develop, so that patients demonstrate greatest weakness during the night or early morning hours and gradually gain strength as the day passes. During major attacks, the serum potassium level decreases but not always to below normal. There is urinary retention of sodium, potassium, chloride, and water [Moxley, 1994]. Oliguria or anuria develops during such attacks, and patients tend to be constipated. Sinus bradycardia and EKG signs of hypokalemia appear when the serum potassium falls below normal. Usually during the fourth and fifth decades of life, attacks become less frequent and may cease. However, repeated attacks may leave the patient with permanent residual weakness [Dalakas and Engel, 1983; Fontaine et al., 2007; Venance et al., 2006; Jurkatt-Rott et al., 2009]. A recent report highlights specific phenotypic differences for hypokalemic periodic paralysis caused by mutations in the calcium channel, compared to those caused by mutations in the sodium channel [Fontaine et al., 2007]. There is an earlier onset of disease in the calcium channel mutations, while myalgias are more common in the sodium channel mutations. On muscle biopsy, there is predominance of tubular aggregates in patients with the sodium channel mutations, and vacuoles in those with calcium channel mutations. Treatment with acetazolamide aggravates hypokalemic periodic paralysis caused by sodium channel mutations [Fontaine et al., 2007].
Genetics
In most kindreds, familial hypokalemic periodic paralysis results from missense mutations at charged residues of the S4 voltage-sensing segments in either the L-type voltage-gated calcium channel, Cav1.1, or in the voltage-gated sodium channel, Nav1.4 [Struyk and Cannon, 2007, 2008; Matthews et al., 2009; Jurkat-Rott et al., 1994, 2000; Ptacek et al., 1994a; Sternberg et al., 2001]. The mutations affect segment S4 of domains 3 and 4. The prevalence of familial hypokalemic periodic paralysis is approximately 1 in 100,000. The symptoms are more severe in males, as is the case in hyperkalemic periodic paralysis.
Pathophysiology
In hereditary hypokalemic periodic paralysis, the muscle fiber develops reduced excitability and an increased sodium conductance that, unlike sodium channel forms of periodic paralysis, cannot be prevented by application of the sodium channel blocker tetrodotoxin [Moxley, 1994]. The in vitro electrophysiologic behavior of muscle fibers with sodium channel mutations associated with hypokalemic periodic paralysis appears to be similar to those with mutated calcium channels [Cannon, 2002]. The precise mechanism by which the skeletal muscle calcium channel permits this inexcitability of the muscle fiber remains unknown. One recent theory suggests that the pathological effect of mutations in both the calcium and sodium channels that cause hypokalemic periodic paralysis results from voltage sensor dysfunction and impaired gating of these channels. However, this theory does not account for the sarcolemmal depolarization or the hypokalemia that are characteristic of hypokalemic periodic paralysis. An alternative hypothesis is that the mutations of both the sodium and the calcium channels affect a common gating pore current that becomes leaky [Struyk and Cannon, 2007, 2008]. The leak sensitizes myofibers to reduced serum potassium levels, and results in membrane depolarization [Jurkatt-Rott et al., 2009]. The reduced serum potassium level leads to a new resting potential at −60 mV, and the cationic leak increases action potential generation from this level, resulting in weakness and paralysis. More research is needed to clarify and confirm this alternative hypothesis.
Clinical Laboratory Tests
Evaluation of serum electrolytes and EKG during attacks of weakness is essential to diagnosis and treatment. Muscle biopsy is usually not necessary but may reveal vacuoles, especially in patients with fixed weakness [Fontaine et al., 2007]. In patients not observed during attacks of weakness, it may be necessary to undertake careful provocative testing if DNA analysis does not identify the responsible mutation [Matthews et al., 2009]. This testing requires hospitalization and continuous monitoring of the EKG, as indicated in previously published protocols [Moxley, 1994, 2000]. Occasionally, hypokalemic paralysis develops from acquired, secondary causes. Causes of secondary hypokalemic weakness include hypokalemia in association with hyperthyroidism, beta-adrenergic stimulation, excessive insulin, alkalemia, barium poisoning, poor potassium intake (e.g., alcoholics, anorexia nervosa), excessive potassium excretion (e.g., diarrhea, laxative abuse, profuse sweating during athletic training), and renal loss of potassium (e.g., renal tubular acidosis, osmotic diuresis, and medication use, such as large doses of penicillin antibiotics) [Moxley, 1994].
Treatment
Acute attacks may respond to oral potassium chloride (0.2–0.4 mmol/kg), repeated at 15- to 30-minute intervals [Moxley, 1994]. Monitoring EKG and serum potassium levels and muscle strength helps determine the frequency of these doses. Milder attacks resolve spontaneously, and mild exercise of the weakened muscles speeds recovery. If a patient has a severe attack and is unable to swallow or is vomiting, very cautious intravenous infusion of potassium may be necessary. Treating physicians should keep in mind the fact that a patient’s serum potassium levels do not represent a total body deficit of potassium, but rather a temporary intracellular shift. Therefore, potassium replacement formulas tend to overestimate the replacement needs and result in potentially dangerous hyperkalemia. Preventive therapy for attacks of hypokalemic weakness is usually needed. A low-sodium (2–3 g/day) and low-carbohydrate (60–80 g/day) diet, avoidance of exposure to cold and overexertion, and supplemental doses of potassium chloride taken 2–4 times daily are preventive measures that may help [Moxley, 1994]. However, no convincing data are available to indicate that oral potassium chloride prevents attacks.
Acetazolamide in divided doses usually abolishes attacks of weakness in most patients when taken daily [Moxley, 1994; Sansone et al., 2008]. Dichlorphenamide is another carbonic anhydrase inhibitor that is useful as preventive treatment, if acetazolamide proves ineffective [Dalakas and Engel, 1983; Moxley, 1994; Tawil et al., 2000; Sansone et al., 2008]. Both of these carbonic anhydrase inhibitors produce a metabolic acidosis, and despite their kaluretic action and their tendency to lower serum potassium levels, they appear to act on skeletal muscle to stabilize potassium flux, especially during insulin stimulation [Corbett et al., 1984]. Side effects are as described in the section on acetazolamide-responsive sodium channel myotonia and myotonia fluctuans. There are rare familial cases of hypokalemic periodic paralysis in which acetazolamide exacerbates attacks of weakness [Torres et al., 1981]. This weakness appears to be a consistent but not universal feature of hypokalemic periodic paralysis families carrying sodium channel mutations [Cannon, 2002; Venance et al., 2004]. In these families, triamterene or spironolactone may control the attacks.
Periodic Paralysis with Cardiac Arrhythmia: Andersen–Tawil Syndrome
Clinical Features
Andersen syndrome [Sansone and Tawil, 2007; Bendahhou et al., 2003; Miller et al., 2004; Tristani-Firouzi et al., 2002] or, as recently recognized, Andersen–Tawil syndrome [Bendahhou et al., 2003], is a rare inherited disorder accounting for less than 10 percent of all periodic paralysis (1:500,000) [Sansone and Tawil, 2007]. It is characterized by periodic paralysis, long QT syndrome, ventricular dysrhythmias, and skeletal developmental abnormalities. The early reports of this disorder raised the possibility of hyperkalemia during attacks of weakness, but as more specific identification of kindreds with Andersen–Tawil syndrome has occurred, it is apparent that the disorder occurs with both hyper- and hypokalemia [Sansone and Tawil, 2007]. One form is a variant of hypokalemic periodic paralysis [Bendahhou et al., 2003; Miller et al., 2004; Tristani-Firouzi et al., 2002]. Patients with this type of hypokalemic weakness have dysmorphic features, such as short stature, clinodactyly, and microcephaly, as well as ventricular dysrhythmias (Figure 96-5). Attacks of weakness develop during the first or second decade [Andersen et al., 1971; Sansone et al., 1997; Tawil et al., 1994]. The attacks of limb weakness can vary from mild, resembling those in the sodium channel form of hyperkalemic periodic paralysis, to severe, resembling the typical calcium channel form of hypokalemic periodic paralysis. The major difference is that, occasionally, the episodes of weakness are associated with syncopal attacks and, rarely, sudden death. The cardiac arrhythmias and extrasystoles improve with an elevation in extracellular potassium, while hypokalemia tends to aggravate the cardiac dysrhythmias. The majority of patients with Andersen–Tawil syndrome have point mutations in the KCNJ2 gene. About 15 percent have hyperkalemic episodes, 20 percent have normokalemic attacks, and the remainder have hypokalemic episodes [Sansone and Tawil, 2007]. In carriers of the potassium channel mutation, Kir2.1, associated with Andersen–Tawil syndrome, 64 percent had attacks of weakness, 71 percent had long QT intervals, and 78 percent had dysmorphic features [Tristani-Firouzi et al., 2002]. Cardiac symptoms can be provoked by digitalis; are refractory to disopyramide phosphate, propranolol, and phenytoin; and may respond to imipramine [Sansone et al., 1997; Tawil et al., 1994]. Weakness and cardiac symptoms in some patients occur following corticosteroid treatment [Bendahhou et al., 2007]. Patients with Andersen–Tawil syndrome have a neurocognitive phenotype that includes deficits in executive function and abstract reasoning, relative to their siblings [Yoon et al., 2006].
Genetics
One of the responsible mutations involves the gene encoding the inward-rectifying potassium channel Kir2.1 [Ai et al., 2002; Andelfinger et al., 2002; Plaster et al., 2001; Sacconi et al., 2009]. Autosomal-dominant inheritance occurs with variable severity within families [Sansone and Tawil, 2007; Bendahhou et al., 2003; Miller et al., 2004; Tristani-Firouzi et al., 2002]. The mutation with the most potent dominant effect is the T75R missense mutation [Sansone and Tawil, 2007]. Aside from the individuals with known mutations in the Kir2.1 gene (over 30 identified), approximately 40 percent of cases with the clinical features of Andersen–Tawil syndrome have unknown mutations.
Pathophysiology
Kir2.1 channels are voltage-gated potassium channels called inward rectifiers. These comprise a family of voltage-related but not voltage-dependent channels, whose function is to stabilize the membrane potential and determine the shape of the terminal portion of the action potential [Sansone and Tawil, 2007]. KCNJ2 encodes the inward rectifier Kir2.1, a member of the Kir2x subfamily, which is the critical alpha subunit of cardiac Ik1, the inward rectifier current [Sansone and Tawil, 2007]. The channel is a dimer of subunits, each with two transmembrane-spanning domains called M1 and M2. The NH2 terminal is on the M1 segment and the COOH terminal is on the M2 segment. More than 30 point mutations have been described so far, and they are mostly localized on the intracellular COOH terminal [Sansone and Tawil, 2007].
The different mutations thus far identified appear to affect channel function through heterogeneous mechanisms [Sacconi et al., 2009; Sansone and Tawil, 2007]. These include:
Other gene mutations that cause Andersen–Tawil syndrome act by a mechanism of haploinsufficiency, probably affecting trafficking and assembly of second messengers via the interaction of abnormal amino-acid positioning along the muscle membrane where the channels are localized [Sansone and Tawil, 2007].
Clinical Laboratory Tests
Periodic monitoring of the EKG is important to identify covert dysrhythmias. DNA testing, as it becomes available, is helpful in establishing the diagnosis [Sansone and Tawil, 2007]. Secondary forms of periodic paralysis most be ruled out with assessments of thyroid, renal, and adrenal functions. Muscle biopsy may help to distinguish patients with Andersen–Tawil syndrome from those with other myopathic disorders, especially various congenital myopathies. Electrophysiologic cold studies are helpful in confirming the presence of skeletal muscle membrane and excitability using the exercise nerve conduction study, which, in many patients, can distinguish those with known sodium, chloride, and calcium channel mutations from individuals with Andersen–Tawil syndrome [Sansone and Tawil, 2007; Fournier et al., 2006].
Treatment
Acetazolamide in doses used to treat sodium channel forms of hyperkalemic periodic paralysis is sometimes effective in preventing attacks of weakness. Dichlorphenamide may provide an effective alternative if acetazolamide fails [Tawil et al., 2000; Sansone et al., 2008]. Cardiac dysrhythmias are less frequent if serum potassium is permitted to range from 4 to 4.4 mEq/L. However, this elevation in potassium may be difficult to achieve because it typically is not feasible with the use of carbonic anhydrase inhibitors. Occasionally, patients require the use of a spironolactone diuretic in combination with a carbonic anhydrase inhibitor to achieve a slightly higher serum potassium. Management of the cardiac dysrhythmia is best handled by a cardiologist. It is typically difficult to control.
Thyrotoxic Periodic Paralysis
Clinical Features
Attacks of thyrotoxic periodic paralysis are unusual in the first decade or early in the second decade of life, but tend to develop in the late teens and during the 20s. It is much more frequent in Asians and males; 95 percent of cases are sporadic [Ko et al., 1996; Moxley, 1994; Ober, 1992]. Precipitating factors include sleep, hot weather, and excessive physical activity [Hsieh et al., 2008]. Recurrent attacks of weakness may occur in over 60 percent of patients who have not returned to the euthyroid state [Hsieh et al., 2008]. Cardiac problems, including sinus tachycardia, atrial fibrillation, supraventricular tachycardia, and ventricular fibrillation, can occur [Hsu et al., 2003; Moxley, 1994; Ober, 1992].
Pathophysiology
Pathophysiology of thyrotoxic periodic paralysis remains a mystery, but it is clear that the hyperthyroid state is an essential factor in mediating the hypokalemia and attacks of weakness [Arimura et al., 2007; Moxley, 1994]. Patients have attacks only when they are hyperthyroid, and attacks cannot be precipitated by insulin and carbohydrate administration once patients become euthyroid, in contrast with patients with hereditary hypokalemic periodic paralysis [Moxley, 1994; Ober, 1992]. Even after patients have become euthyroid and symptom-free, paralytic attacks recur if the patient experiences a relapse into a thyrotoxic state or if hyperthyroidism is produced again with exogenous thyroid hormone [Arimura et al., 2007; Moxley, 1994; Ober, 1992]. Hyperthyroidism alters plasma membrane permeability to sodium and potassium, and increases responsiveness to catecholamines, probably through an increase in the sodium potassium adenosine triphosphatase in skeletal muscle [Ewart and Klip, 1995]. However, hypokalemia does not appear to be the primary regulator of weakness in thyrotoxic periodic paralysis because some patients regain their strength in the presence of persistent hypokalemia [Shayne and Hart, 1994]. Moreover, a degree of hypokalemia sufficient to provoke a paralytic attack in a hyperthyroid patient may have no effect when that same patient is euthyroid [Ober, 1992], emphasizing that the relationship between hypokalemia and thyrotoxic periodic paralysis is complex. The fact that propranolol can prevent paralytic attacks at a level of hypokalemia, which, in the same individual, typically precipitates weakness [Shiang et al., 2009; Ober, 1992], raises additional questions about the specific role of changes in serum potassium in the etiology of thyrotoxic periodic paralysis.
Genetics
The gene defect responsible for thyrotoxic hypokalemic periodic paralysis remains unclear. One report described an R83H mutation in the potassium channel gene, KCNE3 [Dias da Silva et al., 2002], in a man who developed symptoms with the onset of Graves’ disease. The mutation was found in 2 of 3 descendants. Three more recent reports have failed to confirm this observation. One study of 97 Chinese men with thyrotoxic periodic paralysis compared the genetic findings in this group to those in 77 Graves’ disease patients without periodic paralysis, and to 100 normal males [Kung et al., 2004]. None of the men with known thyrotoxic periodic paralysis had mutations in the calcium channel, sodium channel, or potassium channel genes previously reported to be associated with periodic paralysis. Another study describes an analysis of the entire coding sequence of the KCNE3 gene in 79 Chinese patients with thyrotoxic periodic paralysis and compares the findings with those from 111 men with thyrotoxicosis without periodic paralysis [Tang et al., 2004]. No mutations were found in the KCNE3 gene. A third report describes genetic studies in 19 Chinese men with thyrotoxic periodic paralysis and compares them to evaluations in 48 thyrotoxic patients and 32 normal individuals [Ng et al., 2004]. Recent studies of membrane excitability after exercise continue to support the hypothesis that paralytic attacks in thyrotoxic patients are due primarily to a pre-existing latent abnormal excitability of the muscle membrane, possibly genetic in origin [Arimura et al., 2007]. However, no mutations have been found in the calcium or sodium channel genes previously reported to cause hypokalemic periodic paralysis.
Treatment
Treatment consists of antithyroid therapy until the patient achieves the euthyroid state. Preventive measures should be undertaken after treatment of the acute attack, as described for primary familial hypokalemic periodic paralysis. Propranolol is often effective in preventing attacks in thyrotoxic periodic paralysis [Ober, 1992]. One recent report has emphasized the importance of propranolol as an initial treatment, and has suggested caution in the use of potassium replacement [Tassone et al., 2004]. A recent prospective study of 78 patients given intravenous potassium as the sole treatment for an acute attack in thyrotoxic periodic paralysis noted that approximately 75 percent of patients required relatively moderate doses of intravenous potassium chloride, that being a total of 63 ± 32 mmol infused at a rate of 10 mmoles/hour to restore muscle strength [Shiang et al., 2009]. However, about 25 percent of these patients experienced paradoxical hypokalemia during the infusion of potassium chloride, and these patients had more marked elevation of free thyroid hormone, higher blood pressure, and higher heart rate on presentation [Shiang et al., 2009]. It appears that paradoxical hypokalemia during the administration of intravenous potassium chloride indicates more severe hyperthyroidism and hyperadrenergic activity, which may require blockage of intracellular potassium shift to prevent rebound hyperkalemia [Shiang et al., 2009]. The investigators indicated that beta-blocker therapy was especially desirable for treatment of these patients. Acetazolamide is ineffective and may worsen or precipitate symptoms in thyrotoxic hypokalemic periodic paralysis; it should be avoided [Moxley, 1994]. In general, beta-blocking drugs are helpful to use throughout the early stages of management until the patient becomes euthyroid.
Myotonic-Like Disorders Affecting Peripheral and Central Nervous System or the Basement Membrane of Neuromuscular Junction and Cartilage
This section briefly describes those disorders that have myotonic-like skeletal muscle manifestations but have their primary defect in nonmuscle tissues. These disorders include Schwartz–Jampel syndrome [Aberfeld et al., 1965; Schwartz and Jampel, 1962], hereditary familial episodic ataxia type 1 [Brunt and van Weerden, 1990], acquired generalized peripheral nerve hyperexcitability [Hart, 2000; Hart et al., 2002], and stiff person syndrome [Dalakas et al., 2000; Koerner et al., 2004; Murinson et al., 2004]. Table 96-6 summarizes the characteristics and treatments of hereditary familial episodic ataxia type 1, acquired generalized peripheral nerve hyperexcitability, and stiff person syndrome. Schwartz–Jampel syndrome and episodic ataxia type 1 occur early in childhood, whereas acquired generalized peripheral nerve hyperexcitability, which usually presents in adults, occasionally has an onset in childhood or adolescence. Stiff person syndrome presents almost exclusively in adults but, in principle, the underlying pathophysiologic process can occur in children. Clinical features, especially the characteristics of the muscle contractions and the associated electromyographic findings, distinguish the myotonic-like disorders from the primary myotonic diseases and the periodic paralyses. Below are highlights of the clinical findings and comments about treatment that complement the information provided in Table 96-6.
Table 96-6 Disorders of Central and Peripheral Nervous System Causing Myotonic-Like Muscle Contractions
Myotonic Chondrodystrophy: Schwartz–Jampel Syndrome
Clinical Features
The phenotype of Schwartz–Jampel syndrome has considerable variability. Severe forms appear in infancy and milder forms usually develop manifestations between 2 and 3 years of age. Patients have a short neck, protuberant abdomen, and sparse subcutaneous tissue. Joint contractures develop, along with a typical facial appearance (small palpebral fissures, pursed lips, low hairline, and low-set ears). The abnormal facies becomes increasingly prominent with age. The bony abnormalities, such as pectus carinatum, kyphoscoliosis, pes planus, hip dysplasia, platybasia, and flattened small vertebrae often are apparent early in the disease. Patients have a stooped posture with their shoulders flexed forward. Muscle hypertrophy and hirsutism are common. Serum creatine kinase activity is mildly increased. In typical Schwartz–Jampel syndrome, range of motion at joints becomes progressively restricted, which may be due to unchecked myotonia. Although carpal tunnel syndrome is rare in childhood, it does occur with some frequency in Schwartz–Jampel syndrome [Van Meir and De Smet, 2003]. Malignant hyperpyrexia may occur, complicating orthopedic surgical procedures [Seay and Ziter, 1978]. Mental retardation is present in 25 percent of affected children, and developmental language disorder and attention-deficit disorder may be present [Paradis et al., 1997]. Severe microcephaly has been reported [Pinto-Escalante et al., 1997]. Orthopedic consultation may precede neurologic evaluation, and comments by a radiologist about the spinal x-ray appearance may point to the diagnosis of Schwartz–Jampel syndrome. On neurologic examination, the combination of action and percussion myotonia, hyporeflexia, typical facies and body appearance, and electromyographic evidence of continuous muscle fiber activity (high-frequency discharges both occurring at rest and exacerbated by muscle contraction) is diagnostic for Schwartz–Jampel syndrome.
Genetics
Genetic heterogeneity accompanies the phenotypic variability in Schwartz–Jampel syndrome. The Schwartz–Jampel syndrome is diagnosed in early childhood by the presence of myotonia, short stature, masklike facies, muscle pseudohypertrophy, skeletal dysplasia with bone and joint abnormalities, and growth retardation [Aberfeld et al., 1965; Cao et al., 1978; Schwartz and Jampel, 1962]. This classic form of Schwartz–Jampel syndrome, proposed as type 1A [Giedion et al., 1997], is autosomal-recessive and associated with 30 mutations, including truncated and missense mutations, in the HSPG2 gene that encodes perlecan. The responsible gene resides on chromosome 1p34–p35 [Rodgers et al., 2007; Echaniz-Laguna et al., 2009; Stum et al., 2006; Arikawa-Hirasawa et al., 2002]. Perlecan is a large heparin sulfate proteoglycan, present in all basement membranes. It participates in cell adhesion, growth factor signaling, and maintenance of the basement membrane [Iozzo, 2005]. The hypomorphic effects of these mutations result in a decrease in the amount of perlecan in basement membranes, but not in the complete absence of this protein [Echaniz-Laguna et al., 2009]. The defective protein that is encoded leads to abnormal cartilage development and anomalous neuromuscular activity [Rodgers et al., 2007; Echaniz-Laguna et al., 2009]. A similar Schwartz–Jampel syndrome, type 1B, is recognizable at birth with more pronounced bone dysplasia. A more severe form of the syndrome, designated type 2, manifests at birth with increased mortality and bone dysplasia. More severe skeletal abnormalities and feeding difficulties are present [Dagoneau et al., 2004; Al Gazali et al., 1996]. Death resulting from respiratory complications may occur in type 2 [Dagoneau et al., 2004]. This severe neonatal form, often referred to as SJS type 2, results from null mutations of the gene that encodes the leukemia inhibitory factor receptor (LIFR) [Dagoneau et al., 2004]. The LIFR is a receptor for a variety of neurotrophic cytokines, and a null mouse model showed a reduced number of astrocytes in the spinal cord and brainstem, suggesting that the muscle stiffness observed in SJS type 2 may be of central nervous system origin [Ware et al., 1995]. The presence in neonates of chondrodysplasia; congenital bowing of shortened femora and tibiae; and facial manifestations consisting of a small mouth, micrognathia, and pursed lips should suggest the diagnosis of Schwartz–Jampel syndrome [Spranger et al., 2000].
Pathophysiology
The electrophysiologic evidence noted earlier emphasizes that the hyperexcitability of nerve-muscle transmission in Schwartz–Jampel syndrome relates to alterations at the neuromuscular junction secondary to a deficiency of perlecan. Recent evidence indicates that perlecan is a key molecule for localizing acetylcholine esterase at the synapse of the neuromuscular junction [Echaniz-Laguna et al., 2009; Rodgers et al., 2007; Stum et al., 2008; Arikawa-Hirasawa et al., 2002]. Studies of muscle biopsies of Schwartz–Jampel syndrome patients demonstrate nonspecific alterations in muscle fibers on typical histochemical staining, and weak immunostaining of perlecan with domain-specific antiperlecan antibodies [Arikawa-Hirasawa et al., 2002]. It seems likely that a deficiency of perlecan at the neuromuscular junction in Schwartz–Jampel syndrome allows sufficient but altered regulation of neuromuscular transmission. This alteration, in turn, leads to continuous muscle fiber activity and myotonic-like muscle contractions. Absence of perlecan, in contrast, may not permit sufficient neuromuscular transmission and accounts for the rapid demise of the patient and the lack of clinical signs of myotonic-like muscle contractions. The electromyographic findings differ from classic myotonic discharges that disappear at rest, have a lower frequency, and wax and wane [Huttenlocher et al., 1969; Taylor et al., 1972]. Striking high-frequency potentials associated with clinical myotonia are demonstrated electrophysiologically. Discharges often occur in bursts of motor unit potentials firing at rates 150–300 Hz for a few seconds, and often start and stop abruptly. The amplitude of the response typically wanes, and discharges may occur spontaneously or be initiated by needle movement or voluntary contraction. These findings are more typical of neuromyotonia, not classical myotonia, in which potentials fire at rates of 20–80 Hz. Electrical silence does not occur during rest, during general anesthesia, or after curarization [Taylor et al., 1972]. Muscle biopsy may demonstrate myopathic findings [Huttenlocher et al., 1969]. Computed tomography of muscles reveals diffuse high attenuation in sternocleidomastoid muscles and low attenuation in the paraspinal, quadriceps, sartorius, soleus, and gastrocnemius muscles [Iwata et al., 2000]. Somatosensory-evoked potentials are delayed [Singh et al., 1997], although visual and brainstem auditory-evoked potentials and nerve conduction velocities are normal. Local infusion of curare abolishes continuous discharges in some patients [Edwards and Root, 1982; Taylor et al., 1972]. Recent studies in the mouse model of Schwartz–Jampel syndrome, caused from a missense mutation in the HSPG2 gene, demonstrate that a deficiency of perlecan provokes neuromyotonia that has its origin in distal portions of the axon, and that the neuromyotonia is abolished with local curare administration or axotomy [Echaniz-Laguna et al., 2009].
Treatment
Therapy in Schwartz–Jampel syndrome is supportive and focuses on specific symptoms and potential complications. Orthopedic consultation and physical and occupational therapy are necessary to treat the bony deformities and contractures. Carbamazepine (20 mg/kg/day) in infants [Squires and Prangley, 1996; Topaloglu et al., 1993] and extended release therapy in older children have been reported to control myotonia and continuous muscle fiber activity. Procainamide is another potential antimyotonia treatment [Huttenlocher et al., 1969] and phenytoin may provide a modest benefit [Edwards and Root, 1982; Taylor et al., 1972]. Response to other antimyotonia drugs, such as mexiletine, has not been reported. Some patients require nasal bilevel positive airway pressure for obstructive sleep apnea [Cook and Borkowski, 1997] and gain some benefit from levator aponeurosis surgery [Cruz et al., 1998]. Anesthesiology consultation before surgery is prudent, since difficulty with intubation [Cook and Borkowski, 1997] and malignant hyperthermia [Seay and Ziter, 1978] are potential complications. Early initiation of treatment tends to lessen skeletal malformations [Squires and Prangley, 1996]. Micrognathia and palate constriction hinder tooth alignment and lead to displacement, impaction, and crowding of teeth [Diaz-Serrano et al., 2006]. These conditions facilitate dental plaque accumulation and contribute to generalized gingivitis and the onset of multiple caries. Myotonia affects the masticatory muscles and leads to rigidity of temporomandibular joints and temporomandibular disorders [Diaz-Serrano et al., 2006]. Botulinum toxin A injections may have a synergistic interaction with carbamazepine therapy in selected patients and improve jaw opening and facial muscle control [Aburahma et al., 2009].
Hereditary Familial Episodic Ataxia Type 1
Clinical Features
There are six forms of familial episodic ataxia currently classified, all rare and autosomal-dominant; the most common is episodic ataxia type 2, caused by mutations in CACNA1A, a gene that encodes the alpha 1A subunit of the main transmembrane neuronal voltage-gated calcium channel [Strupp et al., 2007]. However, the form of episodic ataxia most likely to resemble a myotonic-like clinical appearance is episodic ataxia type 1. The classic description of episodic ataxia type 1 includes the appearance of sudden episodes of cerebellar ataxia, lasting a few minutes, often provoked by startle, and beginning in childhood [Tomlinson et al., 2009; Rajakulendran et al., 2007; Brunt and van Weerden, 1990]. Some patients display prominent myokymia [Van Dyke et al., 1975]. However, recent clinical studies demonstrate that there is considerable phenotypic variation in episodic ataxia type 1 [Eunson et al., 2000; Kinali et al., 2004; Klein et al., 2004]. Some patients have grip myotonia, muscle stiffness, limited jaw opening, contractures (elbows and ankles), muscle hypertrophy, and kyphoscoliosis [Kinali et al., 2004], a clinical picture that resembles the childhood form of Schwartz–Jampel syndrome. Some individuals within a kindred only display seizures and have no episodes of ataxia or muscle stiffness [Tomlinson et al., 2009; Rajakulendran et al., 2007; Eunson et al., 2000]. In contrast, one report describes a patient with prolonged episodes (10–12 hours in duration) of painful muscle stiffness, triggered by fever and exertion, and associated with wrist flexion, thumb adduction, and myokymia in the periorbital muscles [Klein et al., 2004]. Ataxia was not a feature of these episodes. Dysarthria and double vision, along with swelling of the feet, occurred at the onset of attacks in this patient [Klein et al., 2004]. Tendon reflexes may be diminished, normal, or hyperactive. Electromyographic studies reveal continuous myokymic discharges and occasional neuromyotonic discharges [Tomlinson et al., 2009; Gutmann and Gutmann, 2004]. Nerve conduction studies are normal. Usually, the clinical picture and the family history of a dominantly inherited episodic disorder confirm the diagnosis.
Genetics and Pathophysiology
Episodic ataxia type 1 is caused by mutations in the KCNA1 gene encoding the Kv1.1 potassium channel alpha subunit [Browne et al., 1994]. Over 20 heterozygous mutations of a single-exon KCNA1 gene on chromosome 12p13 have resulted in episodic ataxia type 1 [Tomlinson et al., 2009]. The majority are missense point mutations. Evidence suggests that the specific molecular defect and the phenotype vary with the location of the mutation [Tomlinson et al., 2009; Rajakulendran et al., 2007; Klein et al., 2004; Rea et al., 2002]. Mutations that cause typical episodic ataxia type 1 (T226A, T226M, T226R, T226K, V4041, I177N) or neuromyotonia (P244H) alone are associated with altered kinetics of the potassium channel [Rajakulendran et al., 2007; Rea et al., 2002]. A mutation (R417stop) that causes a severe medication-resistant form of episodic ataxia type 1 impairs both tetramerization of the R417stop with the wild type of hKv1.1 subunits and the membrane targeting of the heterotetramers [Eunson et al., 2000]. Two other mutations (T226R, A242P), which lead to a severe phenotype of episodic ataxia type 1, including seizures, produce a combination of defects in channel assembly, membrane trafficking, and abnormal channel kinetics [Rea et al., 2002]. Another mutation, which involves a single nucleotide change at position 785 T>C, causes prolonged (10–12 hours), painful episodes of stiffness triggered by fever and exertion. These clinical findings [Klein et al., 2004] are similar to those in patients with antibody-mediated acquired generalized peripheral nerve hyperexcitability [Hart et al., 2002], and have led the investigators to hypothesize that the 785 T>C mutation causes channel dysfunction similar to that in the autoimmune disorder [Klein et al., 2004]. Further research is necessary to establish phenotype–genotype relationships. Electromyographic studies are often helpful in distinguishing episodic ataxia type 1 from episodic ataxia type 2 by revealing the abnormal peripheral nerve excitability that is apparent from the neuromyotonic discharges observed [Tomlinson et al., 2009].
Treatment
Therapy is symptomatic. Reducing opportunities for stress, startle, and overexertion lessens the frequency of episodes. Physical therapy and orthopedic consultation are helpful in patients with contractures. Acetazolamide may reduce episodes of ataxia, but carbamazepine is necessary to control muscle stiffness and myokymia [Tomlinson et al., 2009; Klein et al., 2004]. Phenytoin can also ameliorate myokymia and stiffness [Kinali et al., 2004].
Acquired Generalized Peripheral Nerve Hyperexcitability
Clinical Features
Acquired generalized peripheral nerve hyperexcitability represents a heterogeneous group of disorders that have included diagnoses such as Isaacs’ syndrome, cramp-fasciculation syndrome, and neuromyotonia [Gutmann and Gutmann, 2004; Hart, 2000; Hart et al., 2002]. Autoimmune, toxic, degenerative, and hereditary forms are included in the classification [Hart et al., 2002]. The core clinical symptoms are muscle twitching, exercise-triggered muscle cramps, and muscle stiffness [Hart et al., 2002]. Delayed relaxation after muscle contraction (pseudomyotonia) occurs less commonly (36 percent) [Hart et al., 2002]. Acquired generalized peripheral nerve hyperexcitability is very uncommon in childhood [Gonzalez et al., 2008]. In a series of 42 patients, only 3 patients were younger than 20 years of age (5, 10, and 15 years of age) [Hart et al., 2002]. One of these patients developed symptoms in early childhood and went untreated for many years. He had growth retardation and presented for evaluation in a wheelchair with severe muscle stiffness and cramps that had been mistaken for spasticity. Patients typically have normoactive or diminished tendon reflexes, and approximately one-third have sensory complaints. Excessive sweating is common (50 percent), but specific alterations of the autonomic nervous system are not. At the time of presentation, most patients have muscle overactivity affecting the legs alone, or legs and trunk combined [Hart et al., 2002]. Distinction between neuromyotonia and other entities, such as cramp-fasciculation syndrome, stiff person syndrome, and rippling muscle disease (a form of caveolinopathy, an inherited myopathy) lies in the clinical and electromyographic evaluation, along with screening for antibodies for the voltage-gated potassium channel [Gonzalez et al., 2008]. Electromyography reveals spontaneous firing of motor units as doublet, triplet, or multiple discharges that have a high burst frequency (40–400 Hz), and in more than 90 percent of cases, the discharges occur at irregular intervals of 1–30 seconds [Hart, 2000]. Voluntary contractions or electrically evoked contractions often provoke prolonged after-discharges. Abnormal spontaneous activity can occur without visible myokymia. These discharges disappear after blockade of neuromuscular transmission, and lessen or disappear in some patients after epidural anesthesia or proximal nerve block [Hart, 2000]. These findings support the hypothesis that the spontaneous discharges result from peripheral nerve hyperactivity [Hart, 2000; Hart et al., 2002].
Pathophysiology
Immune-related diseases, such as myasthenia gravis, diabetes mellitus, and rheumatoid arthritis, are more frequent in acquired generalized peripheral nerve hyperexcitability, as is lung cancer. Approximately 40 percent of patients have antibodies to the voltage-gated potassium channel [Hart et al., 2002], and these antibodies appear to have a central role in the pathophysiology [Hart et al., 2002; Tomimitsu et al., 2004; Gonzalez et al., 2008]. Studies of cultured cell lines incubated with patient sera indicate that there is antibody-mediated suppression of outward-gated potassium current [Tomimitsu et al., 2004]. Recent in vitro investigations using neuronal cell lines indicate that the reduction in potassium currents by antibodies to the voltage-gated potassium channel is independent of added complement; however, F(ab′)2 fragments isolated from patient sera significantly reduce potassium currents. These findings make it likely that cross-linking of the voltage-gated potassium channels by divalent antibodies is an important mechanism in reducing the potassium current [Tomimitsu et al., 2004]. Most cases are caused by antibodies in the immunoglobulin G subclass, but some recent cases appear to be related to potassium channel antibodies only appearing in the immunoglobulin M-containing fraction [Kurono et al., 2006]. This raises the possibility of new treatment strategies directed at these patients in the future. Other circulating factors cause acquired generalized peripheral nerve hyperexcitability, such as timber rattlesnake envenomation [Gutmann and Gutmann, 2004]. The venom enters the circulation, causing generalized myokymia involving the face and extremities. Symptoms abate within hours of administering antivenin therapy [Gutmann and Gutmann, 2004].
Treatment
Carbamazepine and phenytoin are the primary symptomatic medications for the neuromyotonia and usually control symptoms of muscle overactivity [Hart, 2000]. Occasionally, effective control of the neuromyotonia alone is sufficient to produce resolution of symptoms [Gonzalez et al., 2008]. In refractory cases or in the initial management of severe cases, plasma exchange [Hart, 2000; Hayat et al., 2000] or immune globulin infusion [Hart, 2000] may produce rapid resolution of symptoms. Prednisone and azathioprine may prove useful for long-term treatment, as well as other immunosuppressive agents [Hart, 2000], but long-term controlled trials are necessary to confirm this clinical impression.
Stiff Person Syndrome
Clinical Features
Stiff person syndrome is a rare neurologic disorder with autoimmune features. Its primary manifestation is progressive, severe muscle rigidity or stiffness, most prominently affecting the spine and lower extremities [Dalakas et al., 2000; Murinson et al., 2004; Rakocevic et al., 2004; Murinson and Guarnaccia, 2008]. Occasionally, the muscle stiffness may resemble myotonia with a delay in relaxation, but the typical manifestations in skeletal muscle are distinguished readily from true myotonia. In stiff person syndrome, there is an insidious onset of muscular rigidity in limb and axial musculature [Dalakas et al., 2000]. The stiffness is most prominent in the abdominal and thoracolumbar muscles and causes difficulty in turning and bending. Electromyographic recordings demonstrate that there is continuous co-contraction of agonist and antagonist muscles, and the patient is unable to relax. Episodic muscle spasms that occasionally may resemble myotonic-like contractions are superimposed on this rigidity and are precipitated by unexpected noises, tactile stimuli, or emotional stress. These manifestations occur in the absence of any other neurologic disease or underlying chronic pain syndrome that might produce prolonged muscle rigidity and spasms. Many patients have an elevated titer of antibodies to glutamic acid decarboxylase antibodies, and they often have diabetes or other autoimmune diseases [Dalakas et al., 2000; Koerner et al., 2004; Murinson et al., 2004; Rakocevic et al., 2004]. Uncommonly, in perhaps 5 percent of stiff person syndrome patients, there is a paraneoplastic origin for the illness, associated with antibodies against amphiphysin [Petzold et al., 2004]. A recent study of 116 patients with stiff person syndrome related to glutamic acid decarboxylase antibodies compared their clinical findings to those of 11 patients with stiff person syndrome related to amphiphysin antibodies and found distinctively different patterns of muscle involvement in the two groups [Murinson and Guarnaccia, 2008]. For patients with high levels of glutamic acid decarboxylase antibodies, the pattern of greater muscle involvement was as follows: legs and spine > abdomen and arms > neck > bulbar. The patients with stiff person syndrome related to amphiphysin antibodies had a different pattern, frequently involving the arms and neck [Murinson and Guarnaccia, 2008]. Almost all the patients with stiff person syndrome related to glutamic acid decarboxylase antibodies had stiffness, rigidity, or increased tone, and the majority had pain [Murinson and Guarnaccia, 2008]. The age range for males was 14–82 years and for females 30–73 years.
Stiff person syndrome is rare in children [Murinson and Guarnaccia, 2008; Saiz et al., 2008; Murinson et al., 2004]. One recent study of 116 stiff person syndrome patients with elevated levels of glutamic acid decarboxylase antibodies included only 1 teenage patient; the remaining 115 patients were older than 30 years of age [Murinson et al., 2004]. In an earlier study of 20 selected patients, the average age was 41.2 years [Dalakas et al., 2000], and in another recent report of 16 patients with stiff person syndrome, the ages ranged from 37 to 62 years, with a median age of 52 years [Rakocevic et al., 2004].
Pathophysiology
Electromyographic investigations in patients with stiff person syndrome disclose the presence of low-frequency firing of normal motor units, especially in the muscles of the trunk, which increases for seconds or minutes to a dense pattern during a spontaneous or provoked muscle spasm [Koerner et al., 2004; Meinck et al., 1995]. These bursts of electrical activity are highly variable in location, duration, and intensity. Investigators have described a stereotyped motor response to stimulation of peripheral nerves in stiff person syndrome patients that consists of a sequence of 1–3 synchronous myoclonic bursts, 60–70 milliseconds after median nerve stimulation, followed by tonic decrescendo activity over a number of seconds [Meinck et al., 1995]. This response is called spasmodic reflex myoclonus, and explains certain aspects of the pathophysiology of stiff person syndrome. The stiffness appears to be a fragment of spasms, and both are due to a common neuronal mechanism of abnormal regulation of interneurons in the spinal gray matter [Meinck et al., 1995]. Other studies support this mechanism and suggest an important role for motor cortex hyperexcitability in stiff person syndrome [Koerner et al., 2004]. A study using transcranial magnetic stimulation methodology points out that the elevation of glutamic acid decarboxylase antibodies directly correlates with the enhanced cortical excitability and that gamma-aminobutyric acid-mimetic medications reduce intracortical facilitation [Koerner et al., 2004]. These observations suggest a clear role for glutamic acid decarboxylase antibodies in mediating the stiffness and muscle spasms. However, the precise pathophysiologic role of glutamic acid decarboxylase antibodies in stiff person syndrome requires further investigation [Saiz et al., 2008].
One recent study of 16 adults with stiff person syndrome notes that there is no correlation between the glutamic acid decarboxylase antibody titers in serum or cerebrospinal fluid and the severity of disease [Rakocevic et al., 2004]. The authors emphasize that an elevation of glutamic acid decarboxylase antibodies serve as an excellent marker for stiff person syndrome, but monitoring their titers during the course of the disease may not have practical value. Another recent study stresses the importance of establishing an appropriate threshold elevation of glutamic acid decarboxylase antibodies before making a diagnosis of stiff person syndrome [Murinson et al., 2004] because nonaffected individuals, such as some diabetic people, may have modest elevations of glutamic acid decarboxylase antibodies. This study also notes that elevation of glutamic acid decarboxylase antibodies do not correlate with the age or duration of stiff person syndrome [Murinson et al., 2004]. It is interesting to note that two children born to two different mothers with stiff person syndrome had high titers of glutamic acid decarboxylase antibodies for the first 24 months of life, and, having reached the ages of 6 and 8 years, they have not had any manifestations of stiff person syndrome [Nemni et al., 2004]. Clinical criteria remain the benchmark for the diagnosis of stiff person syndrome.
Treatment
Diazepam is an effective medication to control muscle spasms and the dose needs to be titrated to each patient [Murinson and Guarnaccia, 2008]. Its beneficial effect is likely mediated by its actions on the gamma-aminobutyric acid (GABAA) receptor. Baclofen, gabapentin, clonazepam, dandrolene, and vigabatrin have provided lesser benefit. Plasma exchange and intravenous immune globulin infusions have proved effective, especially in times of acute worsening or in patients who develop refractoriness to symptomatic therapy with these medications [Donofrio et al., 2009; Dalakas et al., 2000, 2001]. Controlled trials are needed to establish the role of long-term treatment with immunosuppressive medications, such as corticosteroids and mycophenolate, and are also needed to determine the comparative effectiveness of plasmapheresis and immune globulin therapy.
References
The complete list of references for this chapter is available online at www.expertconsult.com.
Abbruzzese C., Costanzi P.S., Mariani B., et al. Instability of a premutation allele in homozygous patients with myotonic dystrophy type 1. Ann Neurol. 2002;52(4):435-441.
Aberfeld D.C., Hinterbuchner L.P., Schneider M. Myotonia, dwarfism, diffuse bone disease and unusual ocular and facial abnormalities (a new syndrome). Brain. 1965;88(2):313-322.
Aburahma S.K., Al Khateeb T., Alrefai A., et al. Botulinum toxin A injections for the treatment of Schwartz-Jampel syndrome: a case series. J Child Neurol. 2009;24(1):5-8.
Ai T., Fujiwara Y., Tsuji K., et al. Novel KCNJ2 mutation in familial periodic paralysis with ventricular dysrhythmia. Circulation. 2002;105(22):2592-2594.
Akiguchi I., Nakano S., Shiino A., et al. Brain proton magnetic resonance spectroscopy and brain atrophy in myotonic dystrophy. Arch Neurol. 1999;56(3):325-330.
al Gazali L.I., Varghese M., Varady E., et al. Neonatal Schwartz-Jampel syndrome: a common autosomal recessive syndrome in the United Arab Emirates. J Med Genet. 1996;33(3):203-211.
Andelfinger G., Tapper A.R., Welch R.C., et al. KCNJ2 mutation results in Andersen syndrome with sex-specific cardiac and skeletal muscle phenotypes. Am J Hum Genet. 2002;71(3):663-668.
Andersen E.D., Krasilnikoff P.A., Overvad H. Intermittent muscular weakness, extrasystoles, and multiple developmental anomalies. A new syndrome. Acta Paediatr Scand. 1971;60(5):559-564.
Arikawa-Hirasawa E., Le A.H., Nishino I., et al. Structural and functional mutations of the perlecan gene cause Schwartz-Jampel syndrome, with myotonic myopathy and chondrodysplasia. Am J Hum Genet. 2002;70(5):1368-1375.
Arimura K., Arimura Y., Ng A.R., et al. Muscle membrane excitability after exercise in thyrotoxic periodic paralysis and thyrotoxicosis without periodic paralysis. Muscle Nerve. 2007;36(6):784-788.
Auvinen S., Suominen T., Hannonen P., et al. Myotonic dystrophy type 2 found in two of sixty-three persons diagnosed as having fibromyalgia. Arthritis Rheum. 2008;58(11):3627-3631.
Bachinski L.L., Udd B., Meola G., et al. Confirmation of the type 2 myotonic dystrophy (CCTG)n expansion mutation in patients with proximal myotonic myopathy/proximal myotonic dystrophy of different European origins: a single shared haplotype indicates an ancestral founder effect. Am J Hum Genet. 2003;73(4):835-848.
Bassez G., Chapoy E., Bastuji-Garin S., et al. Type 2 myotonic dystrophy can be predicted by the combination of type 2 muscle fiber central nucleation and scattered atrophy. J Neuropathol Exp Neurol. 2008;67(4):319-325.
Baumann P., Siira P., Vanharanta H., et al. Quantification of muscle strength in recessive myotonia congenita. Eur Neurol. 1996;36(5):284-287.
Bendahhou S., Donaldson M.R., Plaster N.M., et al. Defective potassium channel Kir2.1 trafficking underlies Andersen-Tawil syndrome. J Biol Chem. 2003;278(51):51779-51785.
Bendahhou S., Fournier E., Gallet S., et al. Corticosteroid-exacerbated symptoms in an Andersen’s syndrome kindred. Hum Mol Genet. 2007;16(8):900-906.
Berardinelli A., Gorni K., Orcesi S. Response to carbamazepine of recessive-type myotonia congenita. Muscle Nerve. 2000;23(1):138-139.
Bernard G., Poulin C., Puymirat J., et al. Dosage effect of a dominant CLCN1 mutation: a novel syndrome. J Child Neurol. 2008;23(2):163-166.
Bouhours M., Sternberg D., Davoine C.S., et al. Functional characterization and cold sensitivity of T1313A, a new mutation of the skeletal muscle sodium channel causing paramyotonia congenita in humans. J Physiol. 2004;554(Pt 3):635-647.
Brancati F., Valente E.M., Davies N.P., et al. Severe infantile hyperkalaemic periodic paralysis and paramyotonia congenita: broadening the clinical spectrum associated with the T704M mutation in SCN4A. J Neurol Neurosurg Psychiatry. 2003;74(9):1339-1341.
Breton R., Mathieu J. Usefulness of clinical and electrocardiographic data for predicting adverse cardiac events in patients with myotonic dystrophy. Can J Cardiol. 2009;25(2):e23-e27.
Brook J.D., McCurrach M.E., Harley H.G., et al. Molecular basis of myotonic dystrophy: Expansion of a trinucleotide (CTG) repeat at the 3′ end of a transcript encoding a protein kinase family member. Cell. 1992(68):799-808.
Browne D.L., Gancher S.T., Nutt J.G., et al. Episodic ataxia/myokymia syndrome is associated with point mutations in the human potassium channel gene, KCNA1. Nat Genet. 1994;8(2):136-140.
Brunt E.R., van Weerden T.W. Familial paroxysmal kinesigenic ataxia and continuous myokymia. Brain. 1990;113(Pt 5):1361-1382.
Campbell C., Sherlock R., Jacob P., et al. Congenital myotonic dystrophy: assisted ventilation duration and outcome. Pediatrics. 2004;113(4):811-816.
Canavese F., Sussman M.D. Orthopaedic manifestations of congenital myotonic dystrophy during childhood and adolescence. J Pediatr Orthop. 2009;29(2):208-213.
Cannon S.C. An expanding view for the molecular basis of familial periodic paralysis. Neuromuscul Disord. 2002;12(6):533-543.
Cannon S.C. Sodium channel defects in myotonia and periodic paralysis. Annu Rev Neurosci. 1996;19:141-164.
Cao A., Cianchetti C., Calisti L., et al. Schwartz-Jampel syndrome. Clinical, electrophysiological and histopathological study of a severe variant. J Neurol Sci. 1978;35(2–3):175-187.
Cardani R., Mancinelli E., Giagnacovo M., et al. Ribonuclear inclusions as biomarker of myotonic dystrophy type 2, even in improperly frozen or defrozen skeletal muscle biopsies. Eur J Histochem. 2009;53(2):107-111.
Carle T., Fournier E., Sternberg D., et al. Cold-induced disruption of Na+ channel slow inactivation underlies paralysis in highly thermosensitive paramyotonia. J Physiol. 2009;587(Pt 8):1705-1714.
Chabrier S., Monnier N., Lunardi J. Early onset of hypokalaemic periodic paralysis caused by a novel mutation of the CACNA1S gene. J Med Genet. 2008;45(10):686-688.
Chang L., Ernst T., Osborn D., et al. Proton spectroscopy in myotonic dystrophy: correlations with CTG repeats. Arch Neurol. 1998;55(3):305-311.
Charlet B., Savkur R.S., Singh G., et al. Loss of the muscle-specific chloride channel in type 1 myotonic dystrophy due to misregulated alternative splicing. Mol Cell. 2002;10(1):45-53.
Christensen A.H., Bundgaard H., Schwartz M., et al. Cardiac myotonic dystrophy mimicking arrhythmogenic right ventricular cardiomyopathy in a young sudden cardiac death victim. Circ Arrhythm Electrophysiol. 2008;1(4):317-320.
Colding-Jorgensen E. Phenotypic variability in myotonia congenita. Muscle Nerve. 2005;32(1):19-34.
Colding-Jorgensen E., Duno M., Vissing J. Autosomal dominant monosymptomatic myotonia permanens. Neurology. 2006;67(1):153-155.
Cook S.P., Borkowski W.J. Obstructive sleep apnea in Schwartz-Jampel syndrome. Arch Otolaryngol Head Neck Surg. 1997;123(12):1348-1350.
Corbett A., Kingston W., Griggs R.C., et al. Effect of acetazolamide on insulin sensitivity in myotonic disorders. Arch Neurol. 1984;41(7):740-743.
Cruz A.A., Souza C.A., Plastino Junior L.S. Levator aponeurosis surgery in Schwartz-Jampel syndrome. Ophthal Plast Reconstr Surg. 1998;14(4):271-276.
Dagoneau N., Scheffer D., Huber C., et al. Null leukemia inhibitory factor receptor (LIFR) mutations in Stuve-Wiedemann/Schwartz-Jampel type 2 syndrome. Am J Hum Genet. 2004;74(2):298-305.
Dalakas M.C., Engel W.K. Treatment of “permanent” muscle weakness in familial Hypokalemic Periodic Paralysis. Muscle Nerve. 1983;6(3):182-186.
Dalakas M.C., Fujii M., Li M., et al. High-dose intravenous immune globulin for stiff-person syndrome. N Engl J Med. 2001;345(26):1870-1876.
Dalakas M.C., Fujii M., Li M., et al. The clinical spectrum of anti-GAD antibody-positive patients with stiff-person syndrome. Neurology. 2000;55(10):1531-1535.
Davies N.P., Hanna M.G. The skeletal muscle channelopathies: distinct entities and overlapping syndromes. Curr Opin Neurol. 2003;16(5):559-568.
Day J.W., Ricker K., Jacobsen J.F., et al. Myotonic dystrophy type 2: molecular, diagnostic and clinical spectrum. Neurology. 2003;60(4):657-664.
Desaphy J.F., De Luca A., Didonna M.P., et al. Different flecainide sensitivity of hNav1.4 channels and myotonic mutants explained by state-dependent block. J Physiol. 2004;554(Pt 2):321-334.
Dias da Silva M.R., Cerutti J.M., Arnaldi L.A., et al. A mutation in the KCNE3 potassium channel gene is associated with susceptibility to thyrotoxic hypokalemic periodic paralysis. J Clin Endocrinol Metab. 2002;87(11):4881-4884.
Diaz-Serrano K.V., Brandao C.B., Brandao R.B., et al. Dental findings and muscular-skeletal features in Schwartz-Jampel syndrome: case report of two affected siblings. Spec Care Dentist. 2006;26(5):225-229.
Donofrio P.D., Berger A., Brannagan T.H., et al. Consensus statement: the use of intravenous immunoglobulin in the treatment of neuromuscular conditions report of the AANEM ad hoc committee. Muscle Nerve. 2009;40(5):890-900. III
Duffield M., Rychkov G., Bretag A., et al. Involvement of helices at the dimer interface in ClC-1 common gating. J Gen Physiol. 2003;121(2):149-161.
Duno M., Colding-Jorgensen E., Grunnet M., et al. Difference in allelic expression of the CLCN1 gene and the possible influence on the myotonia congenita phenotype. Eur J Hum Genet. 2004;12(9):738-743.
Echaniz-Laguna A., Rene F., Marcel C., et al. Electrophysiological studies in a mouse model of Schwartz-Jampel syndrome demonstrate muscle fiber hyperactivity of peripheral nerve origin. Muscle Nerve. 2009;40(1):55-61.
Edwards W.C., Root A.W. Chondrodystrophic myotonia (Schwartz-Jampel syndrome): report of a new case and follow-up of patients initially reported in 1969. Am J Med Genet. 1982;13(1):51-56.
Eunson L.H., Rea R., Zuberi S.M., et al. Clinical, genetic, and expression studies of mutations in the potassium channel gene KCNA1 reveal new phenotypic variability. Ann Neurol. 2000;48(4):647-656.
Ewart H.S., Klip A. Hormonal regulation of the Na(+)-K(+)-ATPase: mechanisms underlying rapid and sustained changes in pump activity. Am J Physiol. 1995;269(2 Pt 1):C295-C311.
Fahlke C., Beck C.L., George A.L.Jr. A mutation in autosomal dominant myotonia congenita affects pore properties of the muscle chloride channel. Proc Natl Acad Sci USA. 1997;94(6):2729-2734.
Fahlke C., Knittle T., Gurnett C.A., et al. Subunit stoichiometry of human muscle chloride channels. J Gen Physiol. 1997;109(1):93-104.
Fialho D., Kullmann D.M., Hanna M.G., et al. Non-genomic effects of sex hormones on CLC-1 may contribute to gender differences in myotonia congenita. Neuromuscul Disord. 2008;18(11):869-872.
Fialho D., Schorge S., Pucovska U., et al. Chloride channel myotonia: exon 8 hot-spot for dominant-negative interactions. Brain. 2007;130(Pt 12):3265-3274.
Finsterer J. Primary periodic paralyses. Acta Neurol Scand. 2008;117(3):145-158.
Fontaine B., Fournier E., Sternberg D., et al. Hypokalemic periodic paralysis: a model for a clinical and research approach to a rare disorder. Neurotherapeutics. 2007;4(2):225-232.
Fournier E., Arzel M., Sternberg D., et al. Electromyography guides toward subgroups of mutations in muscle channelopathies. Ann Neurol. 2004;56(5):650-661.
Fournier E., Viala K., Gervais H., et al. Cold extends electromyography distinction between ion channel mutations causing myotonia. Ann Neurol. 2006;60(3):356-365.
Fu Y.H., Pizzuti A., Fenwick R.G.. An unstable triplet repeat in a gene related to myotonic muscular dystrophy. Science. 1992(255):1256-1258.
Gay S., Dupuis D., Faivre L., et al. Severe neonatal non-dystrophic myotonia secondary to a novel mutation of the voltage-gated sodium channel (SCN4A) gene. Am J Med Genet A. 2008;146(3):380-383.
George A., Schneider-Gold C., Zier S., et al. Musculoskeletal pain in patients with myotonic dystrophy type 2. Arch Neurol. 2004;61(12):1938-1942.
George A.L.Jr, Crackower M.A., Abdalla J.A., et al. Molecular basis of Thomsen’s disease (autosomal dominant myotonia congenita). Nat Genet. 1993;3(4):305-310.
Giedion A., Boltshauser E., Briner J., et al. Heterogeneity in Schwartz-Jampel chondrodystrophic myotonia. Eur J Pediatr. 1997;156(3):214-223.
Gonzalez G., Barros G., Russi M.E., et al. Acquired neuromyotonia in childhood: case report and review. Pediatr Neurol. 2008;38(1):61-63.
Gorog D.A., Russell G., Casian A., et al. A cautionary tale: the risks of flecainide treatment for myotonic dystrophy. J Clin Neuromuscul Dis. 2005;7(1):25-28.
Groh W.J., Groh M.R., Saha C., et al. Electrocardiographic abnormalities and sudden death in myotonic dystrophy type 1. N Engl J Med. 2008;358(25):2688-2697.
Gutmann L., Gutmann L. Myokymia and neuromyotonia 2004. J Neurol. 2004;251(2):138-142.
Harper P.S. Myotonic Dystrophy. In: Karpati G., Hilton-Jones D., Griggs R.C., editors. Disorders of Voluntary Muscle. Cambridge: Cambridge University Press; 2001:541-559.
Harper P.S., van Engelen B. Myotonic dystrophy: Present management, future therapy. Oxford: Oxford University Press; 2004.
Hart I.K. Acquired neuromyotonia: a new autoantibody-mediated neuronal potassium channelopathy. Am J Med Sci. 2000;319(4):209-216.
Hart I.K., Maddison P., Newsom-Davis J., et al. Phenotypic variants of autoimmune peripheral nerve hyperexcitability. Brain. 2002;125(Pt 8):1887-1895.
Hayat G.R., Kulkantrakorn K., Campbell W.W., et al. Neuromyotonia: autoimmune pathogenesis and response to immune modulating therapy. J Neurol Sci. 2000;181(1–2):38-43.
Heatwole C.R., Miller J., Martens B., et al. Laboratory abnormalities in ambulatory patients with myotonic dystrophy type 1. Arch Neurol. 2006;63(8):1149-1153.
Heatwole C.R., Moxley R.T.III. The nondystrophic myotonias. Neurotherapeutics. 2007;4(2):238-251.
Hsieh M.J., Lyu R.K., Chang W.N., et al. Hypokalemic thyrotoxic periodic paralysis: clinical characteristics and predictors of recurrent paralytic attacks. Eur J Neurol. 2008;15(6):559-564.
Hsu Y.J., Lin Y.F., Chau T., et al. Electrocardiographic manifestations in patients with thyrotoxic periodic paralysis. Am J Med Sci. 2003;326(3):128-132.
Huichalaf C., Schoser B., Schneider-Gold C., et al. Reduction of the rate of protein translation in patients with myotonic dystrophy 2. J Neurosci. 2009;29(28):9042-9049.
Huttenlocher P.R., Landwirth J., Hanson V., et al. Osteo-chondro-muscular dystrophy. A disorder manifested by multiple skeletal deformities, myotonia, and dystrophic changes in muscle. Pediatrics. 1969;44(6):945-958.
International Myotonic Dystrophy Consortium (IDMC). New nomenclature and DNA testing guidelines for myotonic dystrophy type 1 (DM1). Neurology. 2000;54(6):1218-1221.
Iozzo R.V. Basement membrane proteoglycans: from cellar to ceiling. Nat Rev Mol Cell Biol. 2005;6(8):646-656.
Iwata H., Ozawa H., Kamei A., et al. Siblings of Schwartz-Jampel syndrome with abnormal muscle computed tomographic findings. Brain Dev. 2000;22(8):494-497.
Jurkat-Rott K., Lehmann-Horn F. Genotype-phenotype correlation and therapeutic rationale in hyperkalemic periodic paralysis. Neurotherapeutics. 2007;4(2):216-224.
Jurkat-Rott K., Lehmann-Horn F., Elbaz A., et al. A calcium channel mutation causing hypokalemic periodic paralysis. Hum Mol Genet. 1994;3(8):1415-1419.
Jurkat-Rott K., Mitrovic N., Hang C., et al. Voltage-sensor sodium channel mutations cause hypokalemic periodic paralysis type 2 by enhanced inactivation and reduced current. Proc Natl Acad Sci USA. 2000;97(17):9549-9554.
Jurkat-Rott K., Weber M.A., Fauler M., et al. K+-dependent paradoxical membrane depolarization and Na+ overload, major and reversible contributors to weakness by ion channel leaks. Proc Natl Acad Sci USA. 2009;106(10):4036-4041.
Kim J.B., Kim M.H., Lee S.J., et al. The genotype and clinical phenotype of Korean patients with familial hypokalemic periodic paralysis. J Korean Med Sci. 2007;22(6):946-951.
Kinali M., Jungbluth H., Eunson L.H., et al. Expanding the phenotype of potassium channelopathy: severe neuromyotonia and skeletal deformities without prominent Episodic Ataxia. Neuromuscul Disord. 2004;14(10):689-693.
Klein A., Boltshauser E., Jen J., et al. Episodic ataxia type 1 with distal weakness: a novel manifestation of a potassium channelopathy. Neuropediatrics. 2004;35(2):147-149.
Koch M.C., Steinmeyer K., Lorenz C., et al. The skeletal muscle chloride channel in dominant and recessive human myotonia. Science. 1992;257(5071):797-800.
Koerner C., Wieland B., Richter W., et al. Stiff-person syndromes: motor cortex hyperexcitability correlates with anti-GAD autoimmunity. Neurology. 2004;62(8):1357-1362.
Ko G.T., Chow C.C., Yeung V.T., et al. Thyrotoxic periodic paralysis in a Chinese population. QJM. 1996;89(6):463-468.
Koty P.P., Pegoraro E., Hobson G., et al. Myotonia and the muscle chloride channel: dominant mutations show variable penetrance and founder effect. Neurology. 1996;47(4):963-968.
Kubota T., Kinoshita M., Sasaki R., et al. New mutation of the Na channel in the severe form of potassium-aggravated myotonia. Muscle Nerve. 2009;39(5):666-673.
Kung A.W., Lau K.S., Fong G.C., et al. Association of novel single nucleotide polymorphisms in the calcium channel alpha 1 subunit gene (Ca(v)1.1) and thyrotoxic periodic paralysis. J Clin Endocrinol Metab. 2004;89(3):1340-1345.
Kurono A., Arimura K., Watanabe O., et al. IgM-containing fraction suppressed voltage-gated potassium channels in acquired neuromyotonia. Acta Neurol Scand. 2006;113(3):185-188.
Kwiecinski H., Ryniewicz B., Ostrzycki A. Treatment of myotonia with antiarrhythmic drugs. Acta Neurol Scand. 1992;86(4):371-375.
Lehmann-Horn F., Jurkat-Rott K. Voltage-gated ion channels and hereditary disease. [Review] [598 refs]. Physiol Rev. 1999;79(4):1317-1372.
Lehmann-Horn F., Rudel R., Jurkat-Rott K. Nondystrophic myotonias and periodic paralysis. In: Engel A.G., Franzini-Armstrong C., editors. Myology. New York: McGraw-Hill; 2004:1257-1300.
Lerche H., Heine R., Pika U., et al. Human sodium channel myotonia: slowed channel inactivation due to substitutions for a glycine within the III-IV linker. J Physiol. 1993;470:13-22.
Lipicky R., Bryant S. A biophysical study of the human myotonias. In: Desmedt J.E., editor. New developments in electromyography and clinical neurophysiology. Basel: S. Karger, 1993.
Liquori C.L., Ikeda Y., Weatherspoon M., et al. Myotonic dystrophy type 2: human founder haplotype and evolutionary conservation of the repeat tract. Am J Hum Genet. 2003;73(4):849-862.
Liquori C.L., Ricker K., Moseley M.L., et al. Myotonic dystrophy type 2 caused by a CCTG expansion in intron 1 of ZNF9. Science. 2001;293(5531):864-867.
Mahadevan M., Tsilfidis C., Sabourin L.. Myotonic dystrophy mutation: an unstable CTG repeat in the 3′ untranslated region of the gene. Science. 1992(255):1253-1255.
Mankodi A., Logigian E., Callahan L., et al. Myotonic dystrophy in transgenic mice expressing an expanded CUG repeat. Science. 2000;289:1769-1773.
Mankodi A., Takahashi M.P., Jiang H., et al. Expanded CUG repeats trigger aberrant splicing of ClC-1 chloride channel pre-mRNA and hyperexcitability of skeletal muscle in myotonic dystrophy. Mol Cell. 2002;10(1):35-44.
Mankodi A., Teng-Umnuay P., Krym M., et al. Ribonuclear inclusions in skeletal muscle in myotonic dystrophy types 1 and 2. Ann Neurol. 2003;54(6):760-768.
Mankodi A., Thornton C.A. Myotonic syndromes. Curr Opin Neurol. 2002;15(5):545-552.
Margolis J.M., Schoser B.G., Moseley M.L., et al. DM2 intronic expansions: evidence for CCUG accumulation without flanking sequence or effects on ZNF9 mRNA processing or protein expression. Hum Mol Genet. 2006;15(11):1808-1815.
Martorell L., Monckton D.G., Sanchez A., et al. Frequency and stability of the myotonic dystrophy type 1 premutation. Neurology. 2001;56(3):328-335.
Matthews E., Fialho D., Tan S.V., et al. The non-dystrophic myotonias: molecular pathogenesis, diagnosis and treatment. Brain. 2010;133(Pt 1):9-22.
Matthews E., Labrum R., Sweeney M.G., et al. Voltage sensor charge loss accounts for most cases of hypokalemic periodic paralysis. Neurology. 2009;72(18):1544-1547.
Matthews E., Tan S.V., Fialho D., et al. What causes paramyotonia in the United Kingdom? Common and new SCN4A mutations revealed. Neurology. 2008;70(1):50-53.
McKay O.M., Krishnan A.V., Davis M., et al. Activity-induced weakness in recessive myotonia congenita with a novel (696+1G>A) mutation. Clin Neurophysiol. 2006;117(9):2064-2068.
Meinck H.M., Ricker K., Hulser P.J., et al. Stiff man syndrome: neurophysiological findings in eight patients. J Neurol. 1995;242(3):134-142.
Meola G., Sansone V. Cerebral involvement in myotonic dystrophies. Muscle Nerve. 2007;36(3):294-306.
Meola G., Sansone V., Perani D., et al. Executive dysfunction and avoidant personality trait in myotonic dystrophy type 1 (DM-1) and in proximal myotonic myopathy (PROMM/DM-2). Neuromuscul Disord. 2003;13(10):813-821.
Meola G., Sansone V., Perani D., et al. Reduced cerebral blood flow and impaired visual-spatial function in proximal myotonic myopathy. Neurology. 1999(5):1042-1050.
Miller T.M., Dias da Silva M.R., Miller H.A., et al. Correlating phenotype and genotype in the periodic paralyses. Neurology. 2004;63(9):1647-1655.
Moxley R.T.III. Channelopathies. Curr Treat Options Neurol. 2000;2(1):31-47.
Moxley R.T.III. Metabolic and endocrine myopathies. In: Walton J.N., Karpati G., Hilton-Jones D., editors. Disorders of voluntary muscle. Edinburgh: Churchhill Livingstone; 1994:647.
Moxley R.T.III, Meola G. The Myotonic Dystrophies. In: Rosenberg R.N., DiMauro S., Paulson H.L., Ptacek L., Nestler E.J., editors. The Molecular and Genetic Basis of Neurologic and Psychiatric Disease. Philadelphia: Lippincott Williams & Wilkins; 2008:532-541.
Moxley R.T.III, Meola G., Udd B., et al. Report of the 84th ENMC Workshop: PROMM (Proximal Myotonic Myopathy) and Other Myotonic Dystrophy-Like Syndromes: 2nd Workshop. 13–15th October, 2000. Loosdrecht, The Netherlands. Neuromuscul Disord. 2002;12(3):306-317.
Moxley R.T.III, Pandya S., Thornton C.A., et al. Therapeutic Trials and Future Advances. In: Harper P.S., van Engelen B., Eymard B., Wilcox D., editors. Myotonic Dystrophy: Present Management, Future Therapy. Oxford: Oxford University Press, 2004.
Mulders S.A., van den Broek W.J., Wheeler T.M., et al. Triplet-repeat oligonucleotide-mediated reversal of RNA toxicity in myotonic dystrophy. Proc Natl Acad Sci USA. 2009;106(33):13915-13920.
Murinson B.B., Butler M., Marfurt K., et al. Markedly elevated GAD antibodies in SPS: effects of age and illness duration. Neurology. 2004;63(11):2146-2148.
Murinson B.B., Guarnaccia J.B. Stiff-person syndrome with amphiphysin antibodies: distinctive features of a rare disease. Neurology. 2008;71(24):1955-1958.
Nemni R., Caniatti L.M., Gironi M., et al. Stiff person syndrome does not always occur with maternal passive transfer of GAD65 antibodies. Neurology. 2004;62(11):2101-2102.
Ng W.Y., Lui K.F., Thai A.C., et al. Absence of ion channels CACN1AS and SCN4A mutations in thyrotoxic hypokalemic periodic paralysis. Thyroid. 2004;14(3):187-190.
Ober K.P. Thyrotoxic periodic paralysis in the United States. Report of 7 cases and review of the literature. [Review] [121 refs]. Medicine. 1992;71(3):109-120.
Orengo J.P., Chambon P., Metzger D., et al. Expanded CTG repeats within the DMPK 3′ UTR causes severe skeletal muscle wasting in an inducible mouse model for myotonic dystrophy. Proc Natl Acad Sci USA. 2008;105(7):2646-2651.
Osborne R.J., Lin X., Welle S., et al. Transcriptional and post-transcriptional impact of toxic RNA in myotonic dystrophy. Hum Mol Genet. 2009;18(8):1471-1481.
Otten R.F., Scherschel J.A., Lopshire J.C., et al. Arrhythmia exacerbation after sodium channel blockade in myotonic dystrophy type 1. Muscle Nerve. 2009;40(5):901-902.
Paradis C.M., Gironda F., Bennett M. Cognitive impairment in Schwartz-Jampel syndrome: a case study. Brain Lang. 1997;56(2):301-305.
Pearson C.E., Nichol E.K., Cleary J.D. Repeat instability: mechanisms of dynamic mutations. Nat Rev Genet. 2005;6(10):729-742.
Petzold G.C., Marcucci M., Butler M.H., et al. Rhabdomyolysis and paraneoplastic stiff-man syndrome with amphiphysin autoimmunity. Ann Neurol. 2004;55(2):286-290.
Pineiro E., Fernandez-Lopez L., Gamez J., et al. Mutagenic stress modulates the dynamics of CTG repeat instability associated with myotonic dystrophy type 1. Nucleic Acids Res. 2003;31(23):6733-6740.
Pinto-Escalante D., Ceballos-Quintal J.M., Canto-Herrera J. Identical twins with the classical form of Schwartz-Jampel syndrome. Clin Dysmorphol. 1997;6(1):45-49.
Pisani V., Panico M.B., Terracciano C., et al. Preferential central nucleation of type 2 myofibers is an invariable feature of myotonic dystrophy type 2. Muscle Nerve. 2008;38(5):1405-1411.
Plaster N.M., Tawil R., Tristani-Firouzi M., et al. Mutations in Kir2.1 cause the developmental and episodic electrical phenotypes of Andersen’s syndrome. Cell. 2001;105(4):511-519.
Ptacek L.J., Tawil R., Griggs R.C., et al. Dihydropyridine receptor mutations cause hypokalemic periodic paralysis. Cell. 1994;77(6):863-868.
Ptacek L.J., Tawil R., Griggs R.C., et al. Sodium channel mutations in acetazolamide-responsive myotonia congenita, paramyotonia congenita, and hyperkalemic periodic paralysis. Neurology. 1994;44(8):1500-1503.
Rajakulendran S., Schorge S., Kullmann D.M., et al. Episodic ataxia type 1: a neuronal potassium channelopathy. Neurotherapeutics. 2007;4(2):258-266.
Rakocevic G., Raju R., Dalakas M.C. Anti-glutamic acid decarboxylase antibodies in the serum and cerebrospinal fluid of patients with stiff-person syndrome: correlation with clinical severity. Arch Neurol. 2004;61(6):902-904.
Rea R., Spauschus A., Eunson L.H., et al. Variable K(+) channel subunit dysfunction in inherited mutations of KCNA1. J Physiol. 2002;538(Pt 1):5-23.
Ricker K., Camacho L.M., Grafe P., et al. Adynamia episodica hereditaria: what causes the weakness? Muscle Nerve. 1989;12(11):883-891.
Ricker K., Koch M.C., Lehmann-Horn F., et al. Proximal myotonic myopathy. Clinical features of a multisystem disorder similar to myotonic dystrophy. Arch Neurol. 1995;52(1):25-31.
Ricker K., Koch M.C., Lehmann-Horn F., et al. Proximal myotonic myopathy: a new dominant disorder with myotonia, muscle weakness, and cataracts. Neurology. 1994;44(8):1448-1452.
Ricker K., Moxley R.T.III, Heine R., et al. Myotonia fluctuans. A third type of muscle sodium channel disease. Arch Neurol. 1994;51(11):1095-1102.
Ricker K., Lehmann-Horn F., Moxley R.T. Myotonia fluctuans [see comments]. Arch Neurol. 1990;47(3):268-272.
Rodgers K.D., Sasaki T., Aszodi A., et al. Reduced perlecan in mice results in chondrodysplasia resembling Schwartz-Jampel syndrome. Hum Mol Genet. 2007;16(5):515-528.
Rudel R., Lehmann-Horn F., Ricker K. The nondystrophic myotonias. In: Engel A.G., Franzini-Armstrong C., editors. Myology. New York: McGraw-Hill; 1994:1291.
Rudnik-Schoneborn S., Schneider-Gold C., Raabe U., et al. Outcome and effect of pregnancy in myotonic dystrophy type 2. Neurology. 2006;66(4):579-580.
Rudnik-Schoneborn S., Zerres K. Outcome in pregnancies complicated by myotonic dystrophy: a study of 31 patients and review of the literature. Eur J Obstet Gynecol Reprod Biol. 2004;114(1):44-53.
Ryan A., Rudel R., Kuchenbecker M., et al. A novel alteration of muscle chloride channel gating in myotonia levior. J Physiol. 2002;545(Pt 2):345-354.
Sacconi S., Simkin D., Arrighi N., et al. Mechanisms underlying Andersen’s syndrome pathology in skeletal muscle are revealed in human myotubes. Am J Physiol Cell Physiol. 2009;297(4):C876-C885.
Saiz A., Blanco Y., Sabater L., et al. Spectrum of neurological syndromes associated with glutamic acid decarboxylase antibodies: diagnostic clues for this association. Brain. 2008;131(Pt 10):2553-2563.
Salisbury E., Schoser B., Schneider-Gold C., et al. Expression of RNA CCUG repeats dysregulates translation and degradation of proteins in myotonic dystrophy 2 patients. Am J Pathol. 2009;175(2):748-762.
Sansone V., Griggs R.C., Meola G., et al. Andersen’s syndrome: a distinct periodic paralysis. Ann Neurol. 1997;42(3):305-312.
Sansone V., Meola G., Links T.P., et al. Treatment for periodic paralysis. Cochrane Database Syst Rev. (1):2008. CD005045
Sansone V., Tawil R. Management and treatment of Andersen-Tawil syndrome (ATS). Neurotherapeutics. 2007;4(2):233-237.
Saviane C., Conti F., Pusch M. The muscle chloride channel ClC-1 has a double-barreled appearance that is differentially affected in dominant and recessive myotonia. J Gen Physiol. 1999;113(3):457-468.
Savkur R.S., Philips A.V., Cooper T.A. Aberrant regulation of insulin receptor alternative splicing is associated with insulin resistance in myotonic dystrophy. Nat Genet. 2001;29(1):40-47.
Savkur R.S., Philips A.V., Cooper T.A., et al. Insulin receptor splicing alteration in myotonic dystrophy type 2. Am J Hum Genet. 2004;74(6):1309-1313.
Schneider C., Ziegler A., Ricker K., et al. Proximal myotonic myopathy: evidence for anticipation in families with linkage to chromosome 3q13. Neurology. 2000;2000(3):383-388.
Schoser B.G., Kress W., Walter M.C., et al. Homozygosity for CCTG mutation in myotonic dystrophy type 2. Brain. 2004;127(Pt 8):1868-1877.
Schoser B.G., Ricker K., Schneider-Gold C., et al. Sudden cardiac death in myotonic dystrophy type 2. Neurology. 2004;63(12):2402-2404.
Schoser B.G., Schneider-Gold C., Kress W., et al. Muscle pathology in 57 patients with myotonic dystrophy type 2. Muscle Nerve. 2004;29(2):275-281.
Schoser B.G., Schroder J.M., Grimm T., et al. A large German kindred with cold-aggravated myotonia and a heterozygous A1481D mutation in the SCN4A gene. Muscle Nerve. 2007;35(5):599-606.
Schwartz O., Jampel R.S. Congenital blepharophimosis associated with a unique generalized myopathy. Arch Ophthalmol. 1962;68:52-57.
Seay A.R., Ziter F.A. Malignant hyperpyrexia in a patient with Schwartz-Jampel syndrome. J Pediatr. 1978;93(1):83-84.
Shalata A., Furman H., Adir V., et al. Myotonia congenita in a large consanguineous Arab family: Insight into the clinical spectrum of carriers and double heterozygotes of a novel mutation in the chloride channel CLCN1 gene. Muscle Nerve. 2009.
Shayne P., Hart A. Thyrotoxic periodic paralysis terminated with intravenous propranolol. Ann Emerg Med. 1994;24(4):736-740.
Sheela S.R. Myotonia congenita: response to carbamazepine. Indian Pediatr. 2000;37(10):1122-1125.
Shiang J.C., Cheng C.J., Tsai M.K., et al. Therapeutic analysis in Chinese patients with thyrotoxic periodic paralysis over 6 years. Eur J Endocrinol. 2009;161(6):911-916.
Sinclair J.L., Reed P.W. Risk factors for perioperative adverse events in children with myotonic dystrophy. Paediatr Anaesth. 2009;19(8):740-747.
Singh B., Biary N., Jamil A.A., et al. Schwartz-Jampel syndrome: evidence of central nervous system dysfunction. J Child Neurol. 1997;12(3):214-217.
Slean M.M., Panigrahi G.B., Ranum L.P., et al. Mutagenic roles of DNA “repair” proteins in antibody diversity and disease-associated trinucleotide repeat instability. DNA Repair (Amst). 2008;7(7):1135-1154.
Spranger J., Hall B.D., Hane B., et al. Spectrum of Schwartz-Jampel syndrome includes micromelic chondrodysplasia, kyphomelic dysplasia, and Burton disease. Am J Med Genet. 2000;94(4):287-295.
Squires L.A., Prangley J. Neonatal diagnosis of Schwartz-Jampel syndrome with dramatic response to carbamazepine. Pediatr Neurol. 1996;15(2):172-174.
Sternberg D., Maisonobe T., Jurkat-Rott K., et al. Hypokalaemic periodic paralysis type 2 caused by mutations at codon 672 in the muscle sodium channel gene SCN4A. Brain. 2001;124(Pt 6):1091-1099.
Strupp M., Zwergal A., Brandt T. Episodic ataxia type 2. Neurotherapeutics. 2007;4(2):267-273.
Struyk A.F., Cannon S.C. A Na+ channel mutation linked to hypokalemic periodic paralysis exposes a proton-selective gating pore. J Gen Physiol. 2007;130(1):11-20.
Struyk A.F., Markin V.S., Francis D., et al. Gating pore currents in DIIS4 mutations of NaV1.4 associated with periodic paralysis: saturation of ion flux and implications for disease pathogenesis. J Gen Physiol. 2008;132(4):447-464.
Struyk A.F., Scoggan K.A., Bulman D.E., et al. The human skeletal muscle Na channel mutation R669H associated with hypokalemic periodic paralysis enhances slow inactivation. J Neurosci. 2000;20(23):8610-8617.
Stum M., Davoine C.S., Vicart S., et al. Spectrum of HSPG2 (Perlecan) mutations in patients with Schwartz-Jampel syndrome. Hum Mutat. 2006;27(11):1082-1091.
Stum M., Girard E., Bangratz M., et al. Evidence of a dosage effect and a physiological endplate acetylcholinesterase deficiency in the first mouse models mimicking Schwartz-Jampel syndrome neuromyotonia. Hum Mol Genet. 2008;17(20):3166-3179.
Tang N.L., Chow C.C., Ko G.T., et al. No mutation in the KCNE3 potassium channel gene in Chinese thyrotoxic hypokalaemic periodic paralysis patients. Clin Endocrinol (Oxf). 2004;61(1):109-112.
Tassone H., Moulin A., Henderson S.O. The pitfalls of potassium replacement in thyrotoxic periodic paralysis: a case report and review of the literature. J Emerg Med. 2004;26(2):157-161.
Tawil R., McDermott M.P., Brown R.J., et al. Randomized trials of dichlorphenamide in the periodic paralyses. Working Group on Periodic Paralysis. Ann Neurol. 2000;47(1):46-53.
Tawil R., Moxley R.T., Griggs R.C. Acetazolamide-induced nephrolithiasis: implications for treatment of neuromuscular disorders. Neurology. 1993;43(6):1105-1106.
Tawil R., Ptacek L.J., Pavlakis S.G., et al. Andersen’s syndrome: potassium-sensitive periodic paralysis, ventricular ectopy, and dysmorphic features [see comments]. Ann Neurol. 1994;35(3):326-330.
Taylor R.G., Layzer R.B., Davis H.S., et al. Continuous muscle fiber activity in the Schwartz-Jampel syndrome. Electroencephalogr Clin Neurophysiol. 1972;33(5):497-509.
Thornton C.A., Griggs R.C., Moxley R.T. Myotonic dystrophy with no trinucleotide repeat expansion [see comments]. Ann Neurol. 1994;35(3):269-272.
Timchenko L.T., Tapscott S.J., Cooper T.A., et al. Myotonic dystrophy: discussion of molecular basis. Adv Exp Med Biol. 2002;516:27-45.
Tomimitsu H., Arimura K., Nagado T., et al. Mechanism of action of voltage-gated K+ channel antibodies in acquired neuromyotonia. Ann Neurol. 2004;56(3):440-444.
Tomlinson S.E., Hanna M.G., Kullmann D.M., et al. Clinical neurophysiology of the episodic ataxias: insights into ion channel dysfunction in vivo. Clin Neurophysiol. 2009;120(10):1768-1776.
Topaloglu H., Serdaroglu A., Okan M., et al. Improvement of myotonia with carbamazepine in three cases with the Schwartz-Jampel syndrome. Neuropediatrics. 1993;24(4):232-234.
Torres C.F., Griggs R.C., Moxley R.T., et al. Hypokalemic periodic paralysis exacerbated by acetazolamide. Neurology. 1981;31(11):1423-1428.
Trip J., de Vries J., Drost G., et al. Health status in non-dystrophic myotonias: close relation with pain and fatigue. J Neurol. 2009;256(6):939-947.
Trip J., Drost G., Ginjaar H.B., et al. Redefining the clinical phenotypes of non-dystrophic myotonic syndromes. J Neurol Neurosurg Psychiatry. 2009;80(6):647-652.
Trip J., Drost G., Verbove D.J., et al. In tandem analysis of CLCN1 and SCN4A greatly enhances mutation detection in families with non-dystrophic myotonia. Eur J Hum Genet. 2008;16(8):921-929.
Tristani-Firouzi M., Jensen J.L., Donaldson M.R., et al. Functional and clinical characterization of KCNJ2 mutations associated with LQT7 (Andersen syndrome). J Clin Invest. 2002;110(3):381-388.
Udd B., Krahe R., Wallgren-Pettersson C., et al. Proximal myotonic dystrophy – a family with autosomal dominant muscular dystrophy, cataracts, hearing loss and hypogonadism: heterogeneity of proximal myotonic syndromes?. Neuromuscul Disord. 1997(4):217-288.
Urbano F.J., Pagani M.R., Uchitel O.D. Calcium channels, neuromuscular synaptic transmission and neurological diseases. J Neuroimmunol. 2008;201–202:136-144.
VanDyke D.H., Griggs R.C., Murphy M.J., et al. Hereditary myokymia and periodic ataxia. J Neurol Sci. 1975;25(1):109-118.
Van Meir N., De Smet L. Carpal tunnel syndrome in children. Acta Orthop Belg. 2003;69(5):387-395.
Venance S.L., Cannon S.C., Fialho D., et al. The primary periodic paralyses: diagnosis, pathogenesis and treatment. Brain. 2006;129(Pt 1):8-17.
Venance S.L., Jurkat-Rott K., Lehmann-Horn F., et al. SCN4A-associated hypokalemic periodic paralysis merits a trial of acetazolamide. Neurology. 2004;63(10):1977.
Vihola A., Bassez G., Meola G., et al. Histopathological differences of myotonic dystrophy type 1 (DM1) and PROMM/DM2. Neurology. 2003;60(11):1854-1857.
Wagner S., Deymeer F., Kurz L.L., et al. The dominant chloride channel mutant G200R causing fluctuating myotonia: clinical findings, electrophysiology, and channel pathology. Muscle Nerve. 1998;21(9):1122-1128.
Wahbi K., Meune C., Becane H.M., et al. Left ventricular dysfunction and cardiac arrhythmias are frequent in type 2 myotonic dystrophy: a case control study. Neuromuscul Disord. 2009;19(7):468-472.
Wang G.K., Russell C., Wang S.Y. Mexiletine block of wild-type and inactivation-deficient human skeletal muscle hNav1.4 Na+ channels. J Physiol. 2004;554(Pt 3):621-633.
Wang G.S., Kuyumcu-Martinez M.N., Sarma S., et al. PKC inhibition ameliorates the cardiac phenotype in a mouse model of myotonic dystrophy type 1. J Clin Invest. 2009;119(12):3797-3806.
Ware C.B., Horowitz M.C., Renshaw B.R., et al. Targeted disruption of the low-affinity leukemia inhibitory factor receptor gene causes placental, skeletal, neural and metabolic defects and results in perinatal death. Development. 1995;121(5):1283-1299.
Webb J., Cannon S.C. Cold-induced defects of sodium channel gating in atypical periodic paralysis plus myotonia. Neurology. 2008;70(10):755-761.
Wheeler T.M. Myotonic dystrophy: therapeutic strategies for the future. Neurotherapeutics. 2008;5(4):592-600.
Wheeler T.M., Sobczak K., Lueck J.D., et al. Reversal of RNA dominance by displacement of protein sequestered on triplet repeat RNA. Science. 2009;325(5938):336-339.
White R.J., Bass S.P. Myotonic dystrophy and paediatric anaesthesia. Paediatr Anaesth. 2003;13(2):94-102.
Yang Z., Lau R., Marcadier J.L., et al. Replication inhibitors modulate instability of an expanded trinucleotide repeat at the myotonic dystrophy type 1 disease locus in human cells. Am J Hum Genet. 2003;73(6):1092-1105.
Yoon G., Quitania L., Kramer J.H., et al. Andersen-Tawil syndrome: definition of a neurocognitive phenotype. Neurology. 2006;66(11):1703-1710.
Zaki M., Boyd P.A., Impey L., et al. Congenital myotonic dystrophy: prenatal ultrasound findings and pregnancy outcome. Ultrasound Obstet Gynecol. 2007;29(3):284-288.
Zwarts M.J., van Weerden T.W. Transient paresis in myotonic syndromes. A surface EMG study. Brain. 1989;112(Pt 3):665-680.