Cell Structure and Function
After reading this chapter, the student will be able to:
• Describe the structure of prokaryotic and eukaryotic cells, and identify their differences
• Describe the structure of gram-positive and gram-negative cell walls and explain their differences
• Explain the formation of biofilms and discuss their importance in healthcare
• Differentiate between the extracellular and intracellular fluid compartments
• Name and describe the different membrane transport mechanisms across a cell membrane
• Describe the structure and function of enzymes, and factors influencing enzyme activity
• Describe aerobic and anaerobic cellular respiration, fermentation, and photosynthesis
• Explain the processes of transcription and translation, and the role of RNA during protein synthesis
• Describe the mechanisms of DNA replication
• Describe the different stages of the cell cycle and list the events that occur in the different stages of mitosis
• Describe meiosis and discuss its importance in sexual reproduction
General Structure of Prokaryotic and Eukaryotic Cells
Cells are the basic structural and functional unit of all living organisms. The name cell comes from the Latin word “cella,” a small room. Robert Hooke (see Chapter 1, Scope of Microbiology) selected this term in 1655, when he discovered cells in a piece of cork with his microscope, and compared the cork cells with small rooms. In 1838, Schleiden and Schwann proposed a formal cell theory, or cell doctrine, that marked the start of modern cell biology. The current cell theory states:
The major events leading to the knowledge of cell biology today are shown in Table 3.1.
TABLE 3.1
Year | Name | Event |
1655 | Robert Hooke | Observes cells of a cork |
1674 | Antony van Leeuwenhoek | Discovers protozoans |
1683 | Antony van Leeuwenhoek | Discovers bacteria |
1838 | Schleiden and Schwann | Propose the cell theory |
1855 | Carl Naegeli and Carl Cramer | Describe the cell membrane as a barrier essential to explain osmosis |
1857 | Rudolph Albert von Kölliker | Discovers mitochondria in muscle cells and describes them as conspicuous granules aligned between the striated myofibrils of muscle |
1890 | Richard Altman | Develops mitochondrial stain and postulates genetic autonomy |
1898 | Carl Benda | Develops crystal violet as mitochondria-specific stain and coins the name |
1882 | Robert Koch | Identifies tuberculosis- and cholera-causing bacteria with aniline dyes |
1898 | Camillo Golgi | Discovers the Golgi apparatus with a silver nitrate stain (Golgi stain) |
1931 | Ernst Ruska | Builds the first transmission electron microscope |
1965 | Cambridge Instrument Company | First commercial scanning electron microscope |
1997 | Roslin Institute, Scotland | Sheep cloned |
Two different types of cells exist, prokaryotes and eukaryotes, which are structurally and functionally different from one another (Table 3.2). However, both cell types share certain properties, and these are as follows:
TABLE 3.2
Comparison of Prokaryotic and Eukaryotic Cells
Characteristic | Prokaryotic Cell | Human Eukaryotic Cell |
Size | 0.2–60 µm | 5–100 µm |
Chromosome | One | Multiple |
Cell membrane | Yes | Yes |
Cell wall | Yes | Yes (prokaryotes) No (eukaryotes) |
Nucleus | No | Yes (except red blood cells) |
Nucleoid area | Yes | No |
Mitochondria | No | Yes |
Endoplasmic reticulum | No | Yes |
Golgi apparatus | No | Yes |
Cytoskeleton | No | Yes |
Ribosome | 70S | 80S |
Mode of reproduction | Asexual | Asexual and sexual |
• Methods of reproduction—cell division, binary fission, mitosis, or meiosis
• The presence of DNA and RNA for protein synthesis
• Cellular metabolism organized in specific metabolic pathways
• Response to external and internal stimuli (changes in temperature, pH, and nutrient levels)
Plasma Membrane and Cell Wall
Plasma Membrane
All living cells, both prokaryotes and eukaryotes, are surrounded by a plasma membrane, about 8 nm (nanometer) thick, creating an intracellular and extracellular environment/compartment. The intracellular and extracellular fluid compartments (ICF and ECF, respectively) are aqueous and the plasma membrane provides the barrier between them. The membrane is composed primarily of phospholipids and proteins. Phospholipids are polar molecules with a polar (hydrophilic) head and a nonpolar (hydrophobic) tail (see Chapter 2, Chemistry of Life). Because the environment on each side of the plasma membrane is aqueous, the hydrophobic tails face each other and the hydrophilic heads are exposed to water on both surfaces of the membrane. This results in the formation of a phospholipid bilayer, illustrated in Figure 3.1.
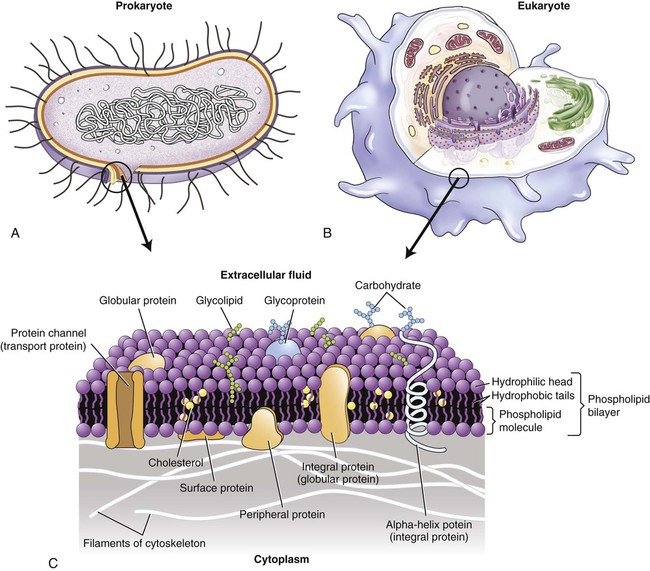
Both prokaryotic cells (A) and eukaryotic cells (B) are surrounded by a plasma membrane (C) composed of a phospholipid bilayer, cholesterol, proteins, and carbohydrates.
Embedded in the phospholipid bilayer are peripheral and integral (transmembrane) proteins and cholesterol. The peripheral proteins are partially embedded on one side of the membrane, whereas integral proteins extend from one side through the membrane to the other side. Although the specific lipid and protein components vary in different membranes, the basic structure and composition of the plasma membrane are the same, as are those membranes that surround cell organelles. Because the plasma membrane is not solid, its components are freely movable, presenting a constantly changing fluid-mosaic membrane structure (see Figure 3.1, A).
Glycolipids, also a component of membranes, project into the extracellular space. Functionally, these may protect, insulate, and serve as receptor-binding sites. In addition, the outer membrane of gram-negative cell walls (see Chapter 6, Bacteria and Archaea) contains lipopolysaccharide bacterial endotoxins that are released on lysis of these cells.
Glycocalyx
• Interaction with other extracellular matrix
• Anchoring of cells to the extracellular matrix
• Modulation of the immune responses
• Attachment site for bacteria to inert surfaces (formation of biofilms)
In the case of bacteria the glycocalyx is a coating of macromolecules that protects the cell and sometimes helps the bacteria to adhere to its environment. The chemical composition, thickness, and organization of the glycocalyx differ among bacteria. One specialized function of the bacterial glycocalyx is the formation of capsules (Figure 3.2). Capsules protect pathogens, such as Streptococcus pneumoniae and Bacillus anthracis, from phagocytosis by white blood cells, thus adding to their pathogenicity.
Cell Wall
The bacterial cell wall is a unique rigid structure that maintains the shape of bacteria and protects them from hostile environments, including protection from the immune system of a host (see Chapter 20, The Immune System). The strength of the cell wall is due to the presence of peptidoglycan (mucopeptide or murein), a mixed polymer of hexose sugars (N-acetylglucosamine and N-acetylmuramic acid) cross-linked by short peptide fragments (Figure 3.3). The amount and composition of peptidoglycan vary among the major bacterial groups and provide the basis for Gram staining (see Chapter 4, Microbiological Laboratory Techniques), dividing bacteria into so-called gram-positive and gram-negative organisms.
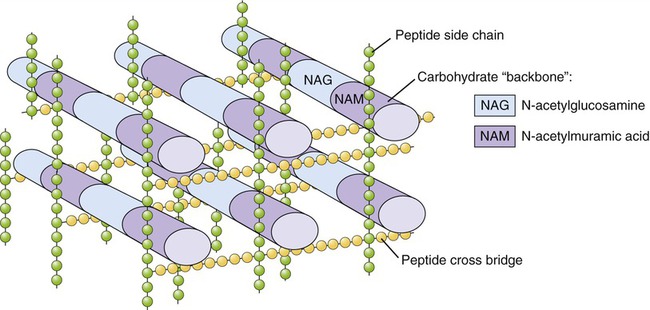
Peptidoglycan is composed of N-acetylglucosamine (NAG) and N-acetylmuramic acid (NAM) chains linked by peptide cross-bridges and peptide side chains to form a relatively rigid structure. The amount and exact composition of peptidoglycan vary among bacterial species.
However, some bacteria do not have a characteristic cell wall structure and some lack a cell wall altogether (i.e., Mycobacterium; see Chapter 6, Bacteria and Archaea). Differences in cell wall composition are an important consideration in the selection of antimicrobial drugs in treatment regimens of bacterial diseases. The cell wall structure is also important in choosing specific methods to control microbial growth in a variety of environments (see Chapter 19, Physical and Chemical Methods of Control).
Characteristics of cell walls in the different microorganisms are as follows:
Gram-positive bacteria have a thick peptidoglycan layer (20–80 nm) located external to the cell membrane (Figure 3.4, A). It also contains acidic polysaccharides such as teichoic acid and lipoteichoic acid, which aid in cell wall maintenance and contribute to an acidic cell surface. The small space between the plasma membrane and the cell wall is the periplasmic space, which can be minimal in some of the gram-positive bacteria.
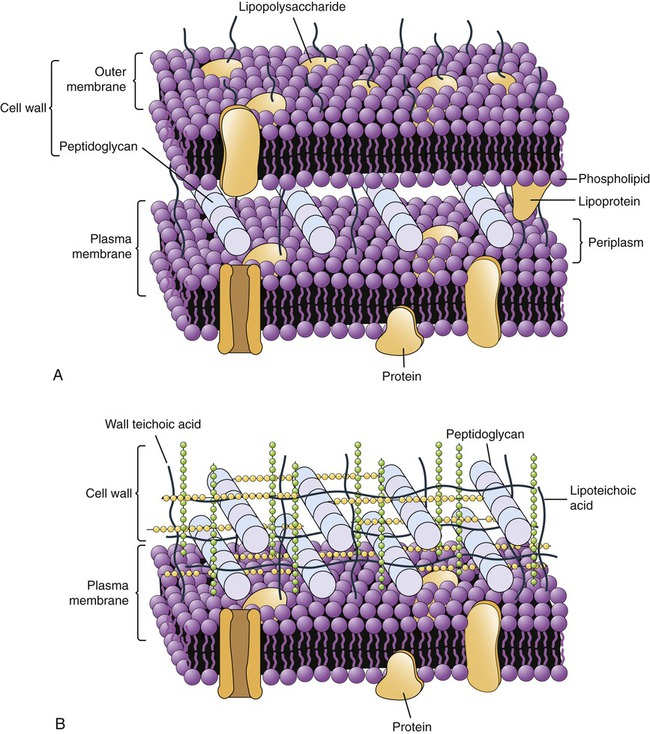
These illustrations show the location of the peptidoglycan layer in gram-positive and gram-negative cell walls along with other features unique to their structure. A, Gram-negative cell wall. B, Gram-positive cell wall.
Gram-negative bacteria have a thin (5- to 10-nm) peptidoglycan layer that is more complex because it has an outer membrane that provides a cover that is anchored to the lipoprotein molecules of the peptidoglycan layer (Figure 3.4, B). The outer membrane is similar in structure to the plasma membrane, but it contains lipopolysaccharides extending from its surface. These lipopolysaccharides can function as receptors or as antigens. In addition, the membrane contains porin proteins, which allow penetration only of small molecules. This serves as a defense mechanism against larger molecules such as antibiotics. The periplasmic space between the plasma membrane and the outer membrane may constitute up to 40% of the total cell volume. This space houses biochemical pathways for nutrient acquisition, peptidoglycan synthesis, electron transport, and detoxification of substances otherwise harmful to the cell.
• The cell wall of archaea does not contain peptidoglycan, but some may contain a similar chemical called pseudopeptidoglycan.
• Plant cell walls are generally made of polysaccharides, mostly cellulose, hemicellulose, and pectin.
• Algae have cell walls that contain cellulose and a variety of glycoproteins. Additional polysaccharide inclusions in their cell walls are used as an identification characteristic in algal taxonomy (see Chapter 8, Eukaryotic Microorganisms).
• The cell wall of fungi is a complex structure composed of chitin, glucans, and other polymers. Not all fungi have a cell wall but among those that do, the composition, properties, and form of it change during the cell cycle and during growth conditions (see Chapter 8, Eukaryotic Microorganisms).
Surface Appendages
Surface appendages are present in both prokaryotic and eukaryotic cells. Prokaryotic cells can have pili (fimbriae) and flagella, whereas cilia, flagella, and microvilli are common in eukaryotic cells. The functions of surface appendages include motility, attachment, absorption, and sensory capacity (Table 3.3).
TABLE 3.3
Surface Appendages of Prokaryotic and Eukaryotic Cells
Surface Appendage | Cell Type | Composition | Function |
Flagella | Eukaryotic cell | Nine pairs of peripheral microtubules made of protein tubulin + two central microtubules | Motility through whiplike action |
Prokaryotic cell | Single microtubule composed of flagellin subunits arranged in a helical formation around a hollow core | Motility through propeller-like rotation | |
Pili | Prokaryotic cell | Pilin proteins helically arranged around a hollow core. Similar to flagella but shorter and more rigid |
Bacterial Flagella
Bacterial flagella are long (3 to 12 micrometers [µm]) helical filaments about 12 to 30 nm in diameter, which they use for movement in their environment. The protein components are flagellins and are assembled to form a cylindrical structure with a hollow core. A flagellum consists of three parts (Figure 3.5):
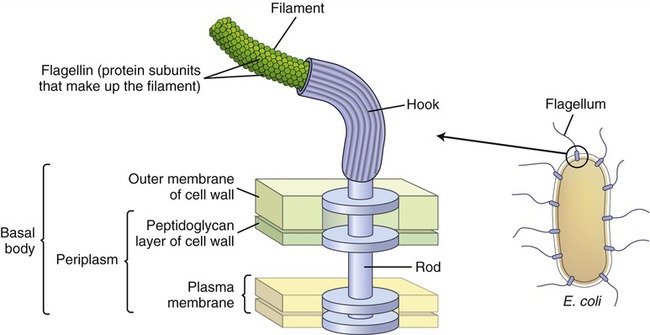
The illustration shows the parts and attachment of the flagellum in a gram-negative bacterium.
• A long filament, which lies external to the cell surface
• A hook located at the end of the filament; and
• A basal body to which the hook is anchored; the basal body consists of a rod and one or two pairs of disks
Flagellins are immunogenic and represent a group of antigens called the H antigens. These antigens are characteristic of a species, strain, or variant of an organism. The species specificity of the flagellins is the result of differences in the primary structures of the proteins (see Chapter 2, Chemistry of Life). The flagella may be present at the poles of the cells as a single polar structure at one end of the bacterium (monotrichous), or at each end (amphitrichous). Flagella also may be present in tufts at one or both ends of the organism, in which case such organisms are lophotrichous. If the flagella are distributed over the general cell surface, they are peritrichous (Figure 3.6).
Bacterial Mobility
The thrust by which the flagella propel the bacterial cell is produced by either clockwise or counterclockwise rotation of the basal body driven by energy from a proton-motive force rather than directly from adenosine-5′-triphosphate (ATP). Bacterial flagella do not rotate at a constant speed; the speed of rotation depends on the cell’s generation of energy. Bacteria can change speed and direction of their flagellar movements and as a result are capable of various patterns of motility. Movements are referred to as runs (swims) or tumbles. A bacterium moving in one direction for a period of time is said to run, and when the direction changes the bacterium tumbles (Figure 3.7). Any movement of bacteria toward or away from a particular stimulus is called taxis. As bacteria run, they may show properties of chemotaxis (response to chemical stimuli) or phototaxis (response to light). Both require sophisticated sensory receptors located in the cell surface and periplasm.
Pili
Pili (fimbriae) are another form of bacterial surface projection (Figure 3.8) and are more rigid than flagella. They are composed of pilin proteins. In some organisms, such as Shigella species and Escherichia coli, as many as 200 pili are distributed over a single cell surface. Pili come in two types: short, abundant common pili, and a smaller number (one to six) of very long sex pili. Sex pili attach male to female bacteria during conjugation. Pili of many enteric bacteria have adhesive properties that can attach to various epithelial surfaces and to surfaces of yeast and fungal cells. These adhesive properties of piliated bacteria play an important role as factors in the colonization of epithelial surfaces. Adhesion to host cells is dependent on specific interactions between the adhesins and molecules in the host cell membranes. For example, the adhesins of E. coli chemically interact with molecules of mannose (a sugar monomer) on the surface of intestinal epithelial cells.
Cilia
Cilia and eukaryotic flagella are structurally identical hairlike appendages, about 0.25 µm in diameter, made from microtubules (Figure 3.9). They are motile and either move the cell or move substances over or around the cell. Protozoans use cilia to collect food particles and for locomotion (see Chapter 8, Eukaryotic Microorganisms). The primary action of cilia in most animal species is to move fluid, mucus, or cells over their surface. The major difference between cilia and eukaryotic flagella is their length, which is much greater for flagella. Cilia can either be motile, constantly beating in one direction, or nonmotile, nonbeating sensors. Motile cilia rarely occur singly and they are usually present in large numbers on cell surfaces, beating in coordinated waves. Nonmotile cilia, on the other hand, generally are single structures per cell.
Microvilli
Microvilli are plasma membrane extensions on the surface of eukaryotic cells. Most often they occur in groups on the surface of absorptive and secretory epithelial cells such as in the kidney and intestine (Figure 3.10). They are approximately 0.08 µm in diameter, 1 µm long, and greatly increase the surface area of cells to facilitate absorption and secretion. Microvilli can also be found on the surface of white blood cells, where they aid in the migration of the cells.
Biofilms
A biofilm is a microbial community enclosed by an extracellular, mostly polysaccharide polymeric matrix (Figure 3.11). Noncellular materials such as mineral crystals, corrosive particles, blood, and other substances can also be found in the biofilm matrix. The kind of inclusions depends on the environment where a biofilm develops. Biofilms develop when free-floating microorganisms attach to a surface. The first colony initially adheres to a given surface by van der Waals forces (see Chapter 2, Chemistry of Life). If not immediately separated from that surface, the organisms can anchor themselves more permanently via adhesion molecules such as pili. Once adhered these microbes then provide various adhesion sites for other new incoming cells, resulting in the building of a matrix that holds the biofilm together. Microbial species that cannot attach to certain surfaces on their own can often attach to the biofilm matrix or to earlier colonized organisms. The biofilm then grows through a combination of cell division and recruitment. The cells on the surface of the biofilm are different from the ones within the matrix and the behavior of the embedded cells may change within the thickness of the biofilm.

Bacteria initially attach to a surface and if not removed they permanently attach, divide, employ other organisms, and ultimately form a biofilm.
Biofilms also provide an ideal environment for the exchange of plasmids (extrachromosomal bacterial DNA; see Chapter 6). Certain plasmids encode resistance to antimicrobial agents and their inclusion within a biofilm provides an opportunity to promote and spread bacterial resistance to antimicrobial agents. Repeated use of antimicrobial agents actually increases the possibility of resistance to biocides (see Chapter 19, Physical and Chemical Methods of Control).
Cytoplasm, Cytoskeleton, Cell Organelles, and Inclusions
Cytoskeleton
Structurally, the cytoskeleton (Figure 3.12) is not rigid but is dynamic and capable of rapid reorganization. This is essential for intracellular transport of vesicles and large molecules, as well as for the rearrangement of organelles. Furthermore, the cytoskeleton forms a spindle apparatus during cell division, which is discussed later in this chapter. The cytoskeleton is composed of actin filaments, intermediate filaments, and microtubules:
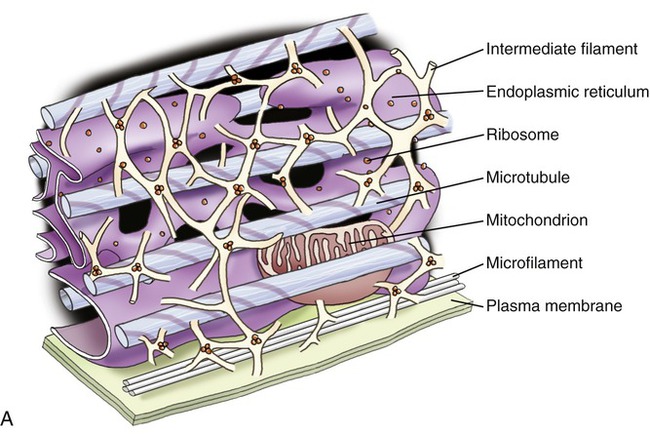
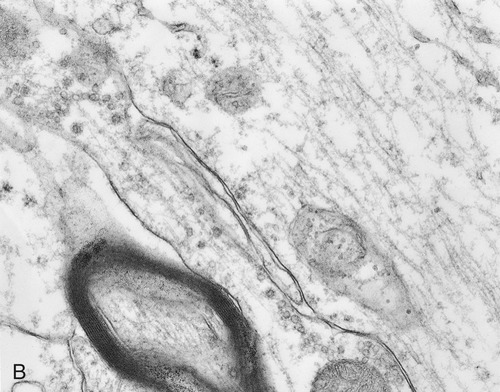
A, Illustration of the components of the cytoskeleton, showing microtubules, microfilaments, and intermediate filaments. B, Electron micrograph of cytoskeletal components of a nerve cell.
• Actin filaments (microfilaments) are made from the globular protein actin, which polymerizes in a helical fashion to form microfilaments. They are about 7 nm in diameter and provide mechanical support for the cell, determine the cell shape, and enable cell movements.
• Intermediate filaments are 8 to 11 nm in diameter and are the more stable components of the cytoskeleton. They organize the internal tridimensional structure of the cell. Intermediate filament proteins include keratins, vimentins, neurofilaments, and lamins.
• Microtubules are hollow cylinders with a diameter of about 24 nm and varying lengths, made of tubulin proteins. They serve as structural components within cells and take part in many cellular processes such as mitosis, cytokinesis, and vesicular transport.
Nucleus
The nucleus (Figure 3.13) is the control center of the eukaryotic cell, containing the DNA. A double membrane, the nuclear envelope, surrounds the nucleus, interrupted by nuclear pores. The nuclear envelope regulates molecular transport between the nucleus and the cytoplasm. The outer membrane of the nuclear envelope is continuous with the cell’s endoplasmic reticulum (ER). Within the nucleus is a prominent structure, the nucleolus, that synthesizes ribosomal RNA (rRNA) and combines the rRNA with proteins to form the subunits of ribosomes.
Endoplasmic Reticulum
The endoplasmic reticulum is part of the endomembrane system of eukaryotic cells. It consists of an elaborate network of membranous tubules and cisternae, continuous with the outer membrane of the nuclear envelope and held together by the cytoskeleton (Figure 3.14). The two forms of ER are rough ER (rER) and smooth ER (sER).
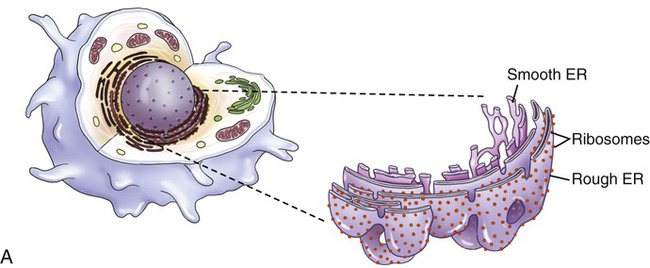
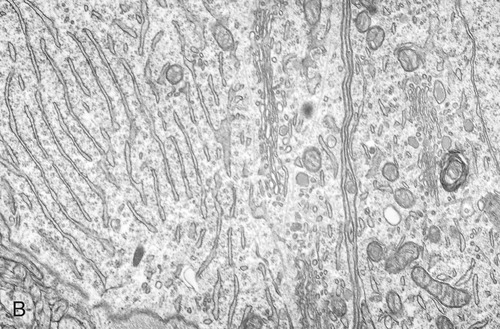
In both the drawing (A) and the electron micrograph (B) the rough endoplasmic reticulum (ER) can easily be identified by the presence of ribosomes on its cisternae.
• rER is called rough because of the attachment of ribosomes on the cytoplasmic side of its membranes. The rER manufactures membranes and proteins, processes the proteins, and passes them to the Golgi apparatus. The secretory proteins produced by the rER are destined primarily for “export” into the extracellular fluid for use by other cells. Cells actively involved in the synthesis of secretory proteins have a very prominent rER.
• sER lacks attached ribosomes and functions in various metabolic processes such as lipid and carbohydrate synthesis as well as in detoxification of drugs and poisons.
Golgi Apparatus
The Golgi apparatus (also called the Golgi complex) consists of flattened, disc-shaped sacs called cisternae with somewhat dilated peripheries that give rise to various secretory vesicles and lysosomes (Figure 3.15). The Golgi apparatus is always closely associated with the ER, the production site of organic molecules. In preparation for export, the Golgi complex modifies and packages the proteins, carbohydrates, and lipids that it receives from the ER. In addition, the Golgi apparatus produces lysosomes for the use within the cell.
Lysosomes
Lysosomes are membrane-bound organelles that pinch off from the Golgi apparatus. Lysosomes contain hydrolytic (digestive) enzymes for intracellular digestion of cell debris and food particles, as well as for the breakdown of invading microorganisms. Another function of lysosomes is to process products of receptor-mediated endocytosis (see Endocytosis, later in this chapter). Lysosomes can be classified into primary and secondary lysosomes, and residual bodies. The size and shape of lysosomes vary with the degree of degradative activity.
• Primary lysosomes are small because they contain digestive enzymes only.
• Secondary lysosomes are larger, as they fuse with particles that require further breakdown (Figure 3.16, A).
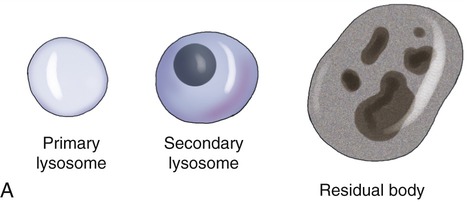
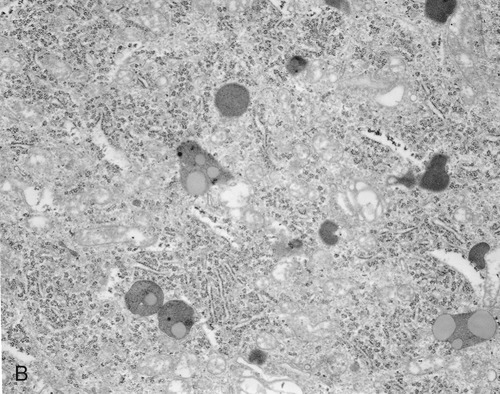
A, The drawing illustrates the primary lysosome containing enzyme only, the secondary lysosome with material to be digested, and the residual body containing material that cannot be further broken down. B, Electron micrograph.
• Residual bodies develop when the enzymes in the lysosome are insufficient or absent and cannot break down the material within the structure (Figure 3.16, B).
Mitochondria
Mitochondria are the cell’s power source for energy, without which none of the cellular activities could proceed. Their double-membrane structure is distinct among cell organelles. The outer membrane encloses the entire organelle and has a phospholipid-to-protein ratio similar to that of the plasma membrane and an inner membrane that has folds reaching inward to form numerous cristae (Figure 3.17). This organelle contains proteins for different functions, including those that carry out the reactions of the respiratory chain for ATP production. The mitochondrial matrix is the space enclosed by the inner membrane and contains a mixture of hundreds of enzymes, mitochondrial ribosomes, transfer RNA (tRNA), and several copies of the mitochondrial DNA genome. The mitochondrial genome encodes primarily proteins for oxidative phosphorylation, as well as rRNAs, tRNAs, and proteins involved in protein synthesis. Mitochondrial ribosomes are of the 70S type (also found in bacteria), distinct from the 80S ribosomes found elsewhere in the eukaryotic cell.
Chloroplasts
Chloroplasts are organelles found in algae and plant cells and are able to convert sun energy into chemical energy through the process of photosynthesis (see Photosynthesis later in this chapter). Chloroplasts are spherical or oval, measuring about 3 to 8 µm in diameter, surrounded by inner membrane system and outer membranes (Figure 3.18). The primary components of the inner membrane system are the thylakoids which consist of flattened disks stacked in parallel arrangements. They contain pigments such as chlorophyll and the enzymes necessary for photosynthesis. The stroma is the fluid within the chloroplast and contains small circular DNA, containing the chloroplast genome, and ribosomes. Chloroplast genes code for photosynthesis and autotrophy. Other genes in the chloroplast genome encode rRNA used in chloroplast ribosomes, tRNA used in translation, some proteins used in transcription and translation, and some other proteins. However, some proteins necessary for chloroplast function are encoded in the nuclear genes.
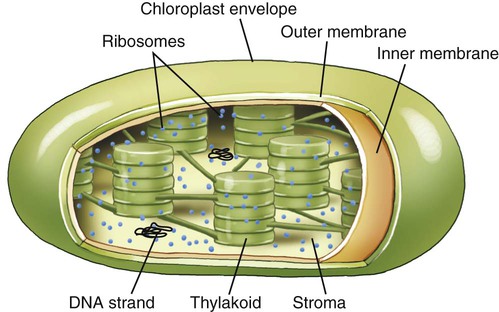
Photosynthesis takes place within chloroplasts. These organelles are surrounded by an envelope consisting of two membranes termed the inner and outer membranes, with a space between them. The stroma is the fluid within the chloroplast and the structures in which photosynthesis takes place are the thylakoids.
Ribosomes
Ribosomes are cell organelles common to both prokaryotic and eukaryotic cells. They consist of two subunits of ribosomal RNA and ribosomal proteins (Figure 3.19). The subunits fit together and operate in unison with tRNA to translate mRNA into a polypeptide chain during protein synthesis. Ribosomes in eukaryotic cells either attach to the ER, identifying it as rough ER, or float freely in the cytoplasm. Free ribosomes produce proteins for maintenance and repair within the cell or in the organelle in which they occur (i.e., mitochondria). The ribosomes in eukaryotic cells are of the 80S type, whereas prokaryotic cells have 70S ribosomes such as are found in mitochondria and chloroplasts.
Vacuoles
Vacuoles are membrane-bound structures in eukaryotic cells that serve a variety of functions. In general, vacuoles in animal cells are smaller than those of plants. Vacuoles store a variety of fluids, soluble materials, and ingested particles. Many freshwater protozoans contain contractive vacuoles that pump excess water out of the cell. Mature plant cells have a single central vacuole surrounded by a membrane called the tonoplast (Figure 3.20). Here the central vacuole often takes up more than 80% of the cell interior, leaving the rest of the cytoplasm confined in the space between the tonoplast and the plasma membrane. The vacuole plays a major role in the growth of plant cells, in that the cells elongate as the vacuole absorbs water to increase cell size with minimal increase in cytoplasm. Also, some vacuoles contain pigments that give color to the cells.
Vesicles
• Transport vesicles, which move molecules between locations inside the cell (i.e., proteins from the rER to the Golgi apparatus)
• Secretory vesicles packaged by the Golgi apparatus with substances to be secreted (i.e., hormones)
• Synaptic vesicles, located in presynaptic terminals of axons, for storing neurotransmitters prior to release during chemical neurotransmission.
HEALTHCARE APPLICATION
Some Toxin-producing Bacteria That Cause Damage to Eukaryotic Cells
Organism/Toxin | Target | Damage | Disease |
Aeromonas hydrophila/aerolysin | Glycophorin | Plasma membrane | Diarrhea |
Clostridium perfringens/perfringolysin O | Cholesterol | Plasma membrane | Gas gangrene |
Escherichia coli/hemolysins | Plasma membrane | Plasma membrane | Urinary tract infections |
Staphylococcus aureus/α-toxin | Plasma membrane | Plasma membrane | Abscesses |
Streptococcus pneumoniae/pneumolysin | Cholesterol | Plasma membrane | Pneumonia |
Streptococcus pyogenes/streptolysin O | Cholesterol | Plasma membrane | Strep throat |
Corynebacterium diphtheriae/diphtheria toxin | Elongation factor-2 | Protein synthesis | Diphtheria |
E. coli, Shigella dysenteriae/Shiga toxins | 28S rRNA | Protein synthesis | Hemorrhagic colitis; hemolytic uremic syndrome |
Fluid Compartments and Membrane Transport Mechanisms
Intracellular Fluid Compartment
ICF, the largest compartment, encompasses two thirds of the human body’s water. Normal values for body water, expressed as a percentage of total body weight, vary between 45% and 75%. These differences are due to age, fat content of the body, and gender. Adipose (fat) tissue contains the least amount of water among body tissues and therefore obese people have less body water per kilogram of weight than do slender ones. In contrast, muscle tissue contains the largest amount of water (65%). Old age is accompanied by loss of muscle mass and increases in body fat resulting in significant decreases in body water content, sometimes up to 45% of total body weight. The distribution of water in the body’s compartments is shown in Figure 3.21. Intracellular fluid has high concentrations of potassium, phosphate, and magnesium ions, and lesser amounts of sodium, chloride, and bicarbonate ions.
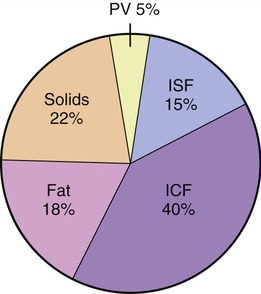
Forty percent is located in the intracellular fluid (ICF) compartment, 15% within the interstitial fluid (ISF), and 15% is plasma volume (PV). The ISF and the PV encompass the extracellular fluid (ECF) compartment. This distribution does change during the aging process because of the loss of muscle mass.
Extracellular Fluid Compartment
Osmotic pressure markedly influences the movement of water and electrolytes throughout the body’s fluid compartments. Whereas the composition of the ECF and ICF differ, the total solute concentration and water amounts are normally equivalent. Thus, net gains of water or of solutes osmotically cause shifts affecting balances in the intracellular and extracellular fluids. These shifts can have a marked influence on uptake, redistribution, or removal of substances, including antibiotics or other drugs and chemicals (see Chapter 21, Pharmacology).
Passive Transport
Diffusion
Diffusion, like other passive transport mechanisms, uses the concentration gradient to move molecules from an area of higher concentration to an area of lower concentration (Figure 3.22). Diffusion is an important mechanism by which cells can readily obtain diffusible materials such as oxygen and release carbon dioxide waste molecules into the external environment.
Facilitated Diffusion
Facilitated diffusion is a process necessary for molecules that cannot readily diffuse through cellular membrane barriers and require membrane transporters for movement across the membrane. As with diffusion, this type of transport does not require energy from ATP. The necessary transport molecules can either be specific membrane carriers or transmembrane channels. Carrier-mediated transport (Figure 3.23):
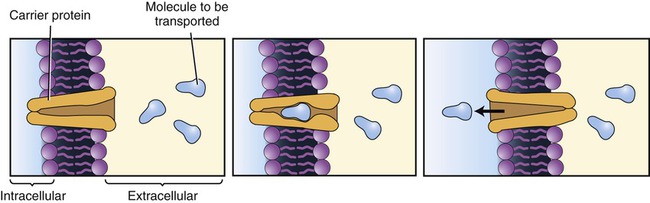
This transport mechanism requires a carrier protein located in the plasma membrane. The carrier protein can transport only one molecule at a time and the molecules to be transported will compete for the carrier.
• Requires specific protein carrier molecules with binding sites for a particular molecule (i.e., glucose, sodium ion)
• Can become saturated because of limited numbers of carrier molecules, limiting the number of molecules that can be transported at a given time
• Can exhibit competition for binding sites on the carrier molecule when two similarly shaped molecules compete for the same binding site. Although the molecules with a better fit show a preference or affinity for the binding site, increases in concentration of the molecules with lesser affinity can facilitate their transport
Osmosis
Osmosis is the diffusion of water across a selectively permeable membrane. The plasma membrane of cells is selectively permeable to water molecules, allowing them to diffuse but excluding substances dissolved in the water. When the concentration of water on one side of the plasma membrane is greater than that on the other side, water will follow its concentration gradient and diffuse to the area of lower water concentration (Figure 3.24). In living cells, however, isotonicity between the ICF and ECF avoids excessive movement of water either into or out of the cells (see Figure 2.11 in Chapter 2, Chemistry of Life). Although water is the solvent molecule that moves across the membrane during osmosis, the solute concentrations of the ECF and ICF dictate how much water is available for diffusion. For example, a 30% solution contains 30 parts of solute and 70 parts of water and a 3% solution contains 3 parts of solute and 97 parts of water. When these two solutions are placed on the opposite sides of a selectively permeable membrane, water will diffuse from an area of higher concentration of water molecules (the 3% solution) to an area of lower concentration of water molecules (the 30% solution).
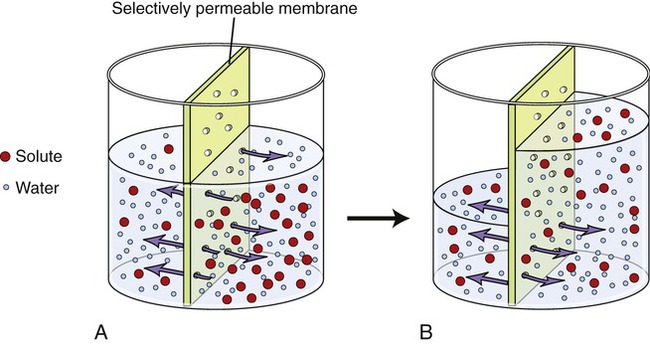
Water molecules will move from an area of high concentration to an area of lower concentration. A, The left side of the selectively permeable membrane contains more water molecules than solutes, whereas the right side of the membrane contains fewer water molecules and more solutes. Thus the water molecules will move from the left to the right side of the membrane until equilibrium is reached (B). The solutes are unable to cross the selectively permeable membrane.
Filtration
Filtration is the movement of molecules through a membrane along a concentration gradient from an area of high hydrostatic pressure to an area of lower hydrostatic pressure. The driving force for filtration can be gravity or, as exemplified in the human body, by the hydrostatic effects of blood pressure on blood plasma, filtering it from the blood capillaries into the ECF. Another important example is filtration from the capillaries of the kidneys into the collecting ducts during urine formation. In microbiology, filtration systems can eliminate microbes from liquid environments (see Chapter 4, Microbiological Laboratory Techniques).
Active Transport
Pump Transport
• Binding of the ion or molecule to be transported to a specific recognition site or receptor on the carrier protein
• Initiation of ATP hydrolysis, which phosphorylates the carrier protein
• Changing of the carrier protein shape, which activates its transport function
• Carrying of the ion or molecule to the other side of the membrane, where it is released
Active pumping systems are important for cells because they allow the cells to move needed ions and other molecules to specific areas. The calcium pump, for example, moves calcium ions to specific areas within the muscle cell during muscle contraction and relaxation. The sodium–potassium pump removes three sodium ions (Na+) out of a cell while it transports two potassium ions (K+) into the cell. Both ions are transported against their chemical concentration gradient, which maintains an electrical charge on the cell membrane, referred to as membrane potential. Most cells contain many Na+ /K+ pumps that are constantly active to maintain the membrane potential. The steep gradient of sodium and potassium ion concentration across cell membranes is essential for conduction and transmission of electrochemical impulses in nerve and muscle cells. If the active extrusion of Na+ is blocked or markedly impeded, the increased Na+ concentration in the cell promotes osmosis, a challenge to isotonic conditions that could result in cell damage, including rupture of the membrane or crenation (see Figure 2.11 in Chapter 2, Chemistry of Life).
Endocytosis
Extracellular material may be brought into the cell by endocytosis, a bulk transport mechanism that uses vesicles. Some eukaryotic cells transport large molecules, particles, liquids, or other cells across the cell membrane via this process. Typically, a cell surrounds and encloses a particle with its membrane, forming an endocytotic vesicle, and then engulfs it. Whole cells or large particles of solid matter are brought into the cell by the process of phagocytosis (“cell eating”; Figure 3.25, A) (see Chapter 20, The Immune System). Liquids, or molecules dissolved in liquids, are transported into the cell by pinocytosis (“cell drinking”; Figure 3.25, B). If it is necessary for a molecule to bind to a membrane receptor before endocytosis can occur, it is called receptor-mediated endocytosis (Figure 3.25, C).
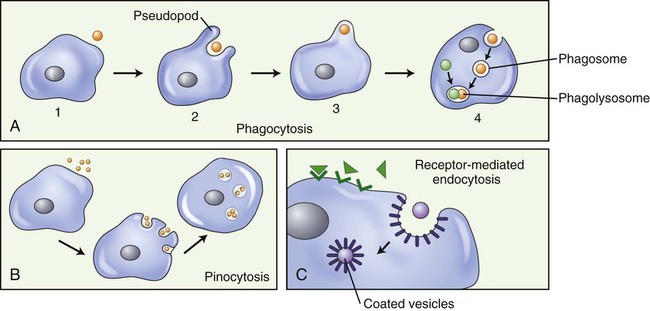
A, Phagocytosis: Large particles (i.e., bacteria) (1), are surrounded by pseudopods (2), engulfed (3), and form a phagosome (4). The phagosome will merge with a lysosome, forming a phagolysosome and the digestion of the large particle can begin. B, Pinocytosis: Fluid is pinocytosed by small extensions of the cell membrane forming pinocytotic vesicles. C, Receptor-mediated endocytosis: Molecules in the extracellular space bind to membrane receptors, resulting in the plasma membrane being pulled inward by the cytoskeleton, forming a pocket around the substance to be transported into the cell. The edges of the pocket then fuse to form a coated vesicle.
Exocytosis
Large molecules, such as polypeptides, proteins, and others, may be excreted from the cell via the process of exocytosis (Figure 3.26). Unicellular organisms (i.e., protozoans) use exocytosis for the elimination of waste products. In multicellular organisms exocytosis has a signaling or regulatory function. Vesicles for exocytosis are produced by the Golgi apparatus, from which they are transported to the cell membrane. Here the vesicles fuse with the cell membrane and their contents are emptied into the extracellular space or into a capillary. In addition to its transport function, exocytosis also adds material to the plasma membrane.
Cellular Metabolism
Cellular metabolism includes all chemical reactions within a cell, which are organized in sequences called metabolic pathways. Metabolic pathways that break down large molecules into smaller ones and release energy in the process are collectively part of catabolism. The pathways that produce larger molecules from smaller ones are part of anabolism and use energy released during catabolic reactions (also see Chapter 2, Chemistry of Life). Nutrients or sunlight supply the energy required to generate ATP molecules. Organisms that utilize energy from the breakdown of nutrient molecules are chemotrophs; those that use sunlight for photosynthesis are phototrophs and they release energy during the process. According to the first law of thermodynamics, energy is neither created nor destroyed; it is only transferred from one form into another. This includes energy for cellular metabolism and energy in general.
Enzymes
Enzymes are biological catalysts that initiate the chemical reactions necessary within the metabolic pathways of cells. All chemical reactions within a cell require enzymes. The mechanism of enzyme action is to lower the energy of activation needed to start a chemical reaction (Figure 3.27). Chemically, enzymes are proteins with a specific structure, usually in a tertiary or quaternary configuration (see Chapter 2, Chemistry of Life). The substances that enzymes act on are referred to as substrates, and the end result of the reaction is a product. Expressed in chemical notation:
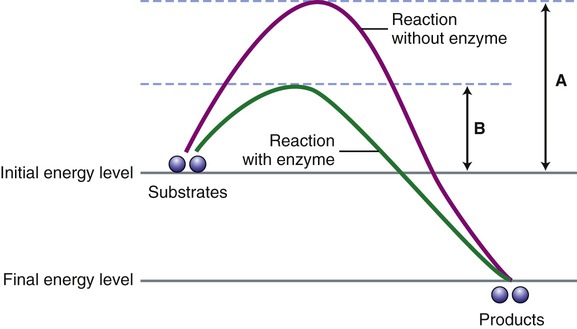
For a chemical reaction to occur a certain energy level must be reached by the substrates for the reaction to start. This diagram illustrates the energy level necessary without the presence of an enzyme (A) and when an enzyme is present (B). Note that enzymes speed up a chemical reaction by lowering the activation energy for the reaction.
< ?xml:namespace prefix = "mml" />


The site to which substrates bind to an enzyme is called the active site and is specific for each substrate. After binding an enzyme–substrate complex is formed and the chemical reaction occurs resulting in a product. At the end of the reaction the enzyme itself is unchanged (Figure 3.28).
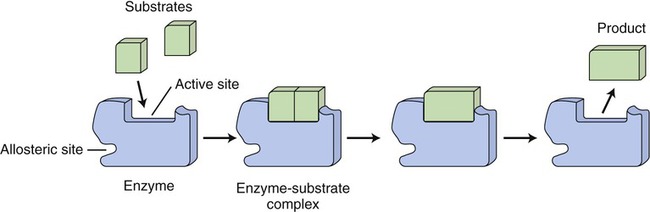
The shape of the active site of an enzyme is specific for its substrate(s). The substrate(s) bind with the active site of the enzyme to form an enzyme–substrate complex and the substrate(s) is/are transformed into a product. The enzyme remains the same after the chemical reaction.
Classification and Naming of Enzymes
• Oxidoreductases catalyze the transfer of electrons in oxidation–reduction reactions.
• Transferases modify molecules and transfer energy-rich bonds from one molecule to another. This occurs in synthesis and decomposition reactions, in which functional groups are transferred from one substrate to another.
• Hydrolases catalyze hydrolysis reactions, in which they break chemical bonds in the presence of water to release energy.
• Lyases remove functional groups from a substrate without adding water, or add functional groups to a double bond.
• Isomerases rearrange atoms within molecules, changing the configuration of the atoms.
• Ligases form bonds between individual monomers to form polymers.
Cofactors and Coenzymes
In many cases the enzymes require nonprotein coenzymes or cofactors, that is, other ions or molecules, in order to catalyze a reaction. The protein portion of an enzyme is the apoenzyme and the nonprotein portion is a coenzyme if it is an organic molecule (usually a derivative from water-soluble vitamins); if it is a metal ion, it is a cofactor. Holoenzymes are enzymes that may require one or more cofactors or coenzymes when combined (Figure 3.29).
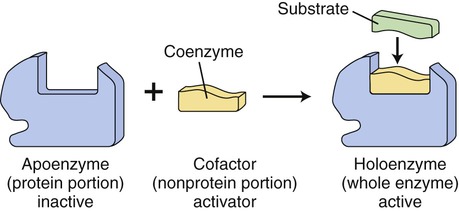
Many enzymes require coenzymes or cofactors to catalyze a reaction. The protein portion of such an enzyme is referred to as the apoenzyme and is inactive; the coenzyme or cofactor is the nonprotein portion. Together the apoenzyme and the coenzyme/cofactor produce the active holoenzyme.
Microorganisms require specific metal ions as trace elements and certain organic growth factors for survival (see Chapter 6, Bacteria and Archaea). The need for these substances occurs because of their roles as cofactors. Enzymes that require cofactors do not have an appropriate shape for the active site until they combine with the cofactor. Metals, therefore, activate enzymes by bringing the active site and the substrate closer together and then participate directly in the chemical reaction as a part of the enzyme–substrate complex. Coenzymes, on the other hand, remove a functional group from one substrate molecule and add it to another substrate. Coenzymes often function as intermediate carriers of hydrogen atoms, electrons, carbon dioxide, and amino groups. An example would be the role of nicotinamide adenine dinucleotide (NAD) in the transfer of electrons in coupled oxidation–reduction reactions (see Cellular Respiration, later in this chapter). Because coenzymes are vitamin derivatives, it might explain the importance of vitamins in our diet; a vitamin deficiency prevents the completion of the holoenzyme and the chemical reaction to be catalyzed fails to occur.
Regulation of Enzyme Activity
• Temperature: Rates of most chemical reactions generally speed up at higher temperatures, and slow down at lower temperatures. However, high temperatures risk denaturing (changing the shape of) the protein portion of enzymes, causing them to lose specificity and functionality (see Chapter 2, Chemistry of Life).
• pH: Enzymes work best within an optimal pH range to promote the most rapid chemical reaction. For the majority of microbial enzymes, this is near pH 7 (neutral).
• Substrate concentration: If the amount of enzyme is fixed, then high concentrations of substrate speed the rate of reaction until the active sites on the enzymes saturate with substrate.
• Enzyme concentration: If the amount of substrate is constant, then the reaction rate initially rises but levels off as the substrate available for the reaction binds to the enzyme.
• Product concentration: Because many enzymes catalyze reactions in both forward and reverse directions, high concentrations may allow the enzymes to catalyze the reverse reaction. However, if the substrates are used in other reactions, then the direction of the first enzyme reaction remains forward.
• Cofactors and coenzymes: Depending on the presence (in sufficient numbers) or absence of cofactors and coenzymes, the rates of enzyme reactions will either increase or slow.
In addition, a variety of other substances such as drugs can either inhibit or reduce the rate of a reaction. The effectiveness of such an inhibitory substance depends on its reversible or irreversible binding properties with the enzyme. For example, in irreversible inhibition, the inhibitor competes with the substrate for the active site of the enzyme (Figure 3.30, A). The degree of inhibition is dependent on the relative concentrations of the inhibitor and the substrate; increasing the amount of substrate may result in reversible inhibition. Competitive enzyme inhibition is the basis for treatment of some infectious diseases by selectively inhibiting a specific metabolic pathway required for the pathogen but not necessary for the human. Antimetabolites function this way, and in so doing they provide an effective weapon against infectious diseases.
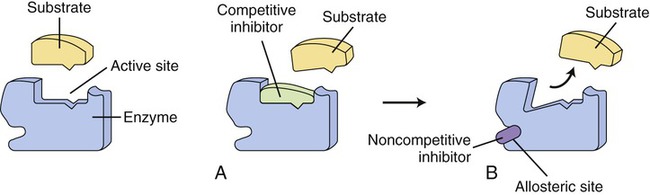
On the left side of the illustration an uninhibited enzyme and its substrate are shown. Enzyme action can be inhibited in two ways: A, by a competitive inhibitor that blocks the active site of the enzyme so that the substrate cannot bind; or B, by noncompetitive inhibition in which a substance binds to the allosteric site of an enzyme, resulting in a change of the shape of the active site so that the substrate cannot bind.
Another mechanism that influences the rate of enzyme reactions is noncompetitive inhibition, in which the substance binds to an allosteric site of an enzyme, causing a conformational change of the enzyme’s active site (Figure 3.30, B). The process is irreversible and the degree of inhibition is dependent on the concentration of the inhibitor. Also, enzyme activity is often regulated at specific points of the metabolic pathways by the process of end-product inhibition—when the amount of product formed is sufficient the reaction shuts down. This is negative feedback inhibition and is an example of allosteric inhibition. In other words, in end-product inhibition, the inhibiting substance is the product formed by the initial enzyme activity. The amount of product formed then feeds back to slow down the reaction and prevent excessive accumulation of more final product (Figure 3.31).
Cellular Respiration and Photosynthesis
Cellular Respiration

Glycolysis, the first pathway of the series, occurs in the cytoplasm and uses 10 enzyme reactions to break down glucose to produce a net yield of 2 molecules of pyruvic acid, 2 molecules of ATP, and 2 molecules of reduced NAD (Figure 3.32). The sequence of the principal steps of glycolysis that form ATP is as follows:
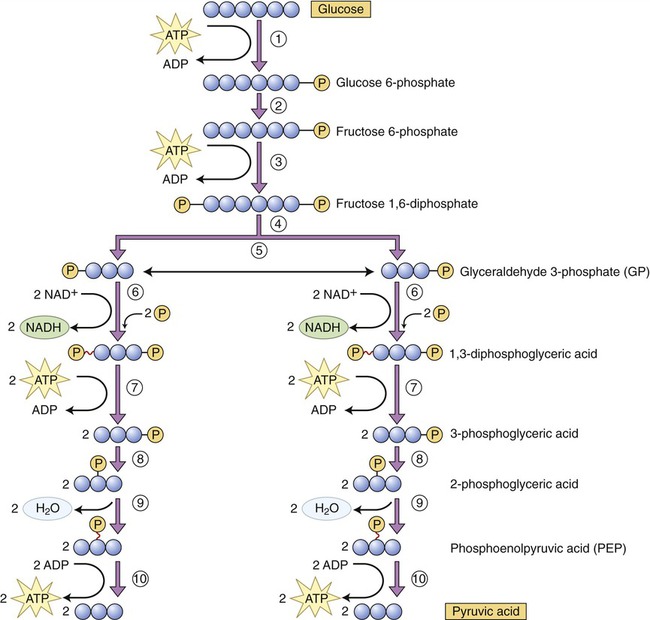
(1) Once in the cell glucose is phosphorylated into glucose 6-phosphate, using a molecule of ATP. (2) Glucose 6-phosphate is converted into fructose 6-phosphate. (3) Using ATP, fructose 6-phosphate is phosphorylated into fructose 1,6-diphosphate. (4) This step represents the enzymatic conversion of fructose 1,6-diphosphate into two three-carbon molecules, dihydroxyacetone phosphate and glyceraldehyde 3-phosphate (GP). (5) Dihydroxyacetone phosphate is easily converted to glyceraldehyde 3-phosphate. (6) Each GP molecule is then converted to 1,3-diphosphoglyceric acid, with the formation of two NADH. (7) One phosphate is removed from each 1,3-diphosphoglyceric acid molecule, forming ATP and 3-phosphoglyceric acid. (8) The phosphate of the 3-phosphoglyceric acid is relocated by enzymatic action. (9) Water is removed from 3-phosphoglyceric acid to form phosphoenolpyruvic acid (PEP). (10) The phosphate is removed from PEP to form ATP and pyruvic acid.
1. Phosphorylation (addition of a phosphate group to a molecule) of glucose to yield glucose 6-phosphate. Phosphorylated organic molecules do not cross the plasma membrane and glucose now remains in the cell.
2. Conversion of glucose 6-phosphate into fructose 6-phosphate.
3. Use of another ATP molecule to phosphorylate fructose 6-phosphate into fructose 1,6-diphosphate.
4. Splitting of fructose 1,6-diphosphate into two three-carbon molecules of 3-phosphoglyceraldehyde.
5. Conversion of each molecule of 3-phosphoglyceraldehyde to 1,3-biphosphoglyceric acid (coenzyme NAD picks up hydrogen from each 3-phosphoglyceraldehyde molecule and forms NADH to which inorganic phosphate [Pi] is added).
6. Biphosphoglyceric acid donates a high-energy phosphate to ADP via substrate-level phosphorylation, forming an ATP molecule. The product of this reaction is 3-phosphoglyceric acid.
7. Isomerization (rearrangement of the chemicals in a given compound) of 3-phosphoglyceric acid produces 2-phosphoglyceric acid.
8. The removal of a water molecule from 2-phosphoglyceric acid produces phosphoenolpyruvic acid.
9. In the final step of glycolysis a phosphate group is removed from each phosphoenolpyruvic acid molecule and donated to ADP, forming another ATP molecule. The product of this reaction is pyruvic acid.
The overall equation for glycolysis is as follows:

This is followed by:

The Krebs cycle is the second pathway in aerobic cellular respiration. It occurs in the plasma membrane of prokaryotes and within the matrix of mitochondria in eukaryotes. After acetyl-CoA enters the Krebs cycle it combines with oxaloacetic acid to form citric acid. Through a series of reactions involving the elimination of two carbons and four oxygens (two CO2 molecules) and the removal of hydrogens, citric acid is eventually converted to oxaloacetic acid to complete this metabolic cycle (Figure 3.33).
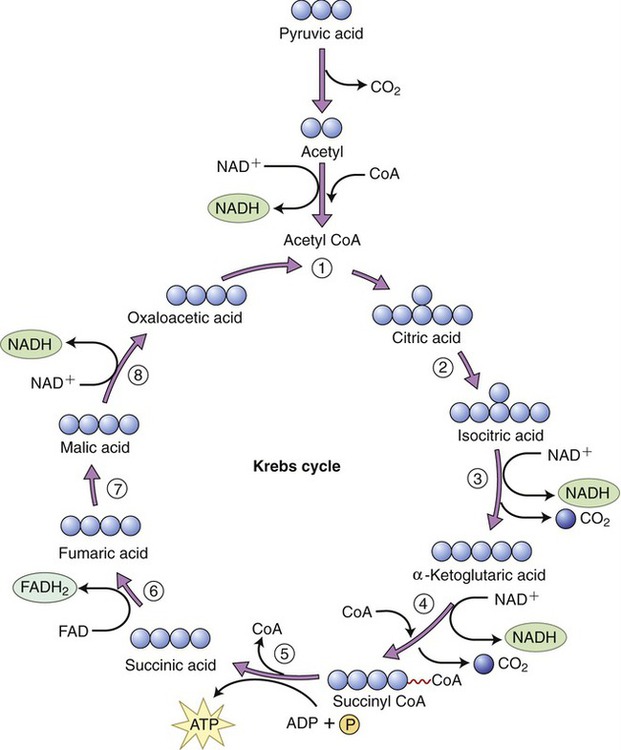
Acetyl-CoA enters the Krebs cycle and combines with oxaloacetic acid to form citric acid. Throughout the steps of the cycle citric acid is finally reconverted into oxaloacetic acid and another cycle can begin.
Steps in the Krebs cycle include the following:
• The production of a guanosine triphosphate (GTP) molecule in step 5, which donates a phosphate group to ADP to form one ATP molecule
• The release of CO2 in steps 3 and 4 for use in endergonic pathways (excess CO2 is released as a gas into the environment)
• The reduction of three molecules of NAD → NADH in steps 4, 5, and 8
• The reduction of one molecule of flavin adenine dinucleotide (FAD) → FADH2 in step 6
The electron transport chain, the last step in aerobic cellular respiration, takes place in the cristae of the inner mitochondrial membrane. In order to regenerate NAD+ and FAD+, electrons and hydrogen ions are transferred from reduced NAD and FAD molecules to carrier molecules in the electron transport chain. These molecules include flavoproteins, coenzyme Q, iron–sulfur enzymes, and cytochromes. The last carrier transfers the electrons to oxygen, the final electron acceptor of aerobic respiration. As the hydrogen ions and electrons are transferred in a stepwise fashion from NADH to the chain of carriers, energy is released. This energy is captured by ADP to generate many ATP molecules from each glucose molecule (Figure 3.34).
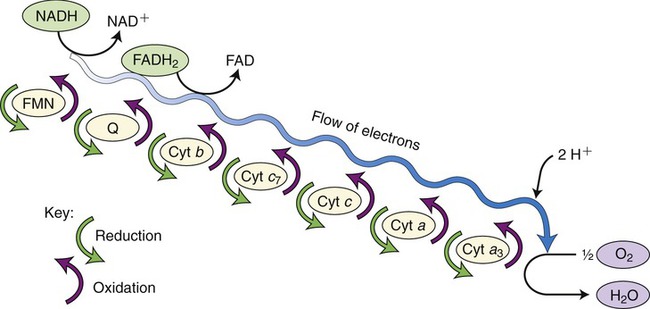
This is the final reaction in aerobic cellular respiration and consists of a series of carrier molecules capable of oxidation–reduction reactions. Electrons are passed down the chain and energy is released in this stepwise reaction and then used to form ATP. The final electron acceptor is oxygen.
The breakdown of one glucose molecule in aerobic respiration is summarized in Table 3.4:
TABLE 3.4
Summary of Aerobic Cellular Respiration per Glucose Molecule
Produced | Glycolysis | Krebs Cycle | Respiratory Chain | Net Total |
ATP | 4 (2 used) | 2 | 34 | 38 |
NADH | 2 | 8 | 0 | 10 |
FADH | 0 | 2 | 0 | 2 |
CO2 | 6 | 0 | 0 | 6 |
H2O | 2 | 2 used | 6 | 6 |
• NADH and FADH entering the respiratory chain produce an estimated 36 ATP molecules plus 4 ATP from glycolysis to create 40 molecules of ATP. But, 2 ATP are consumed during glycolysis, which leaves a maximum of 38 ATP molecules generated per glucose molecule.
• Six carbon dioxide molecules are generated during the Krebs cycle.
• Six oxygen molecules are used in the electron transport chain.
• A net six water molecules are produced in the electron transport chain. A total of eight water molecules are produced, including two in glycolysis, but two of this total are used in the Krebs cycle. Thus, a net number of six water molecules is generated.
The phosphogluconate pathway (also called the pentose phosphate pathway or hexose monophosphate shunt) is an alternative catabolic pathway followed by some bacteria (Figure 3.35). This pathway generates NADPH and synthesizes pentose sugars in two distinct phases. The first is the oxidative phase, which generates NADPH, and the second phase synthesizes pentose sugars. This is a common pathway for heterolactic fermentative bacteria. It yields various end products including lactic acid, ethanol, and carbon dioxide. In addition, this pathway is a significant source of pentose sugars during nucleic acid synthesis.
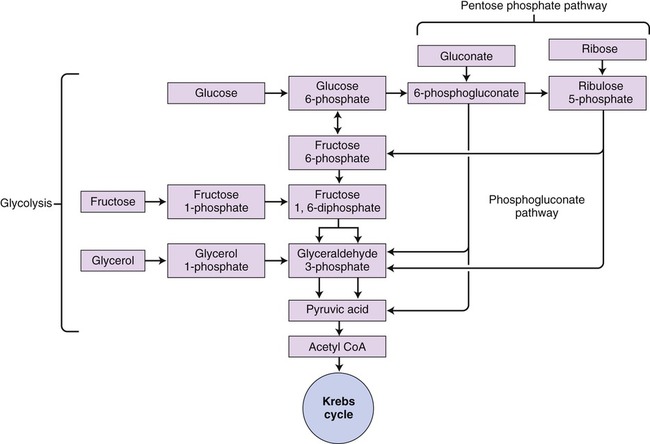
This pathway is an alternative pathway for glycolysis used by heterolactic fermentative bacteria. The diagram shows how different molecules can be utilized to produce NADPH.
• Streptococcus and Lactobacillus: Lactic acid, ethyl alcohol, and acidic acid
• Escherichia coli: Acetic acid, lactic acid, succinic acid, ethyl alcohol, CO2, and H2
• Clostridium: Butyric acid, butyl alcohol, acetone, acetic acid, isopropyl alcohol, CO2, and H2
• Enterobacter: Formic acid, ethyl alcohol, 2,3-butanediol, lactic acid, CO2, and H2
• Actinomyces: Formic acid, acetic acid, and ethyl alcohol
• Saccharomyces (yeast): Ethyl alcohol, acetaldehyde, and CO2
Photosynthesis
Photosynthesis is a fundamental biochemical process by which plants, most algae, cyanobacteria, and some phototrophic bacteria convert light energy into chemical energy via ATP. Chlorophyll, a pigment found in chloroplasts (Figure 3.36, A) of plants and algae, is the site of photosynthesis. Photosynthetic bacteria do not have chloroplasts and photosynthesis takes place directly within the cell. Cyanobacteria contain thylakoid membranes (Figure 3.36, B) similar to those in chloroplasts and these are the only prokaryotes that perform oxygen-generating photosynthesis. Other photosynthetic bacteria contain a variety of different pigments, called bacteriochlorophylls, but they do not produce oxygen. Photosynthesis requires water for oxidation but some bacteria oxidize hydrogen sulfide instead and produce sulfur as a waste product. The rate of photosynthesis is affected by carbon dioxide, light intensity, and temperature.


The first stage of photosynthesis is a light-dependent reaction that requires solar energy that is converted to chemical energy. Chlorophyll absorbs light and drives a transfer of electrons and hydrogen from water to an acceptor called NADP+ (nicotinamide adenine dinucleotide phosphate), which stores the energized electrons for a short period of time. During this process water is split and oxygen gas is given off. The process of producing ATP from sunlight is called photophosphorylation. There are two forms of photophosphorylation: noncyclic photophosphorylation (Figure 3.37, A) and cyclic photophosphorylation (Figure 3.37, B).
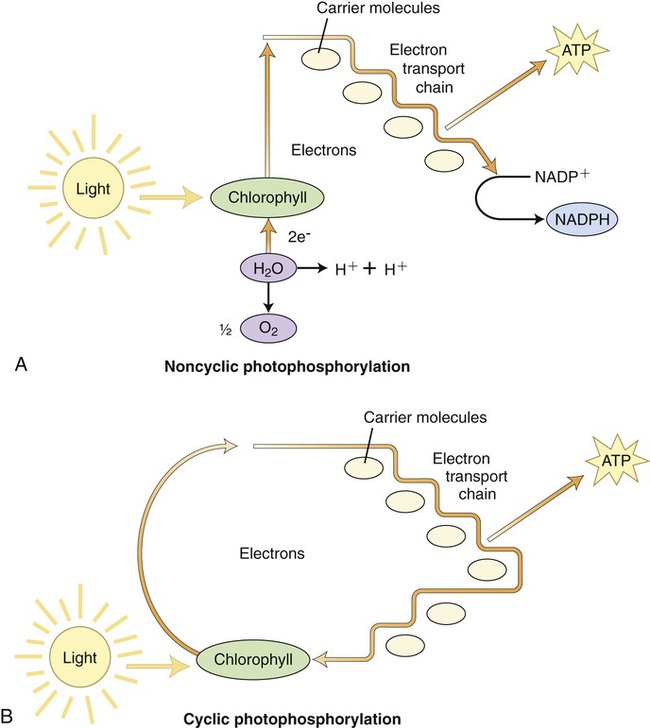
A, Noncyclic photophosphorylation, the more common route by which the electrons released by chlorophyll are replaced by electrons from water. B, In cyclic photophosphorylation the released electrons return to the chlorophyll after going through the electron transport chain. Both pathways utilize the energy released through the electron transport chain to produce ATP molecules.
1. Oxidation of chlorophyll in PSII by light causes an electron to rise to a higher energy level. This electron is detained by the primary electron acceptor. This oxidized P680 chlorophyll now has a “hole” that needs filling.
2. Electrons are enzymatically removed from water and transferred to P680 to replace the electrons lost when light was absorbed by chlorophyll. A water molecule is then split into two hydrogen ions and an oxygen atom. The oxygen atom combines with another one to form O2.
3. Each excited electron from the primary electron acceptor of PSII moves to PSI through the electron transport chain.
4. The energy released by electrons during the cascade down the electron transport chain is captured by the thylakoid membrane. This energy is used to produce ATP for synthesis of sugar during the second stage of photosynthesis—the Calvin cycle.
5. The electron “hole” in P700 of PSI, created by light energy driving an electron to the primary acceptor of PSI, is filled.
6. Electrons are passed by the primary acceptor to the iron-containing protein ferredoxin (Fd) in a second electron transport chain. The enzyme NADP+ reductase transfers the electrons from Fd to NADP+. This reduction reaction stores the electrons in NADPH, which is the molecule that will also provide energy for the synthesis of sugar in the Calvin cycle.
The second stage of photosynthesis, the light-independent reactions or dark reactions, takes place in the stroma within the chloroplast. The enzyme RuBisCO (ribulose-1,5-biphosphate carboxylase/oxygenase) captures CO2 from the air and releases three-carbon sugars in a complex process called the Calvin-Benson cycle. The Calvin-Benson cycle is similar to the Krebs cycle because the starting material regenerates after molecules enter and leave the cycle. Carbon enters the cycle in the form of CO2 and leaves in the form of sugar. The energy source for the cycle is ATP, and NADPH is the reducing power for adding high-energy electrons to make three-carbon sugars (glyceraldehyde 3-phosphate) that later combine to form glucose. The Calvin-Benson cycle is organized into three phases: carbon fixation, reduction, and regeneration of the CO2 receptor (Figure 3.38).
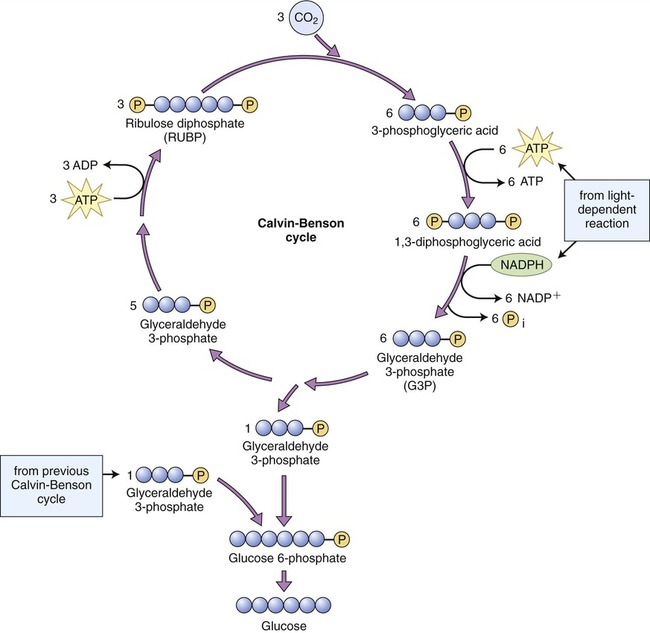
Three carbon dioxide molecules from the atmosphere are fixed and combine with three molecules of ribulose 1,5-biphosphate (RuBP). The resulting molecules are then reduced to form six molecules of glyceraldehyde 3-phosphate (G3P). Five of the G3P molecules are converted to three RuBP to complete the cycle. The other G3P molecule remains and combines with another G3P molecule to form glucose 6-phosphate, which then can be converted to glucose. Therefore, it takes two turns of the Calvin-Benson cycle to synthesize one glucose 6-phosphate molecule.
Protein Synthesis
Cellular structure and function are dependent on proteins (see Chapter 2, Chemistry of Life). Amino acids are the monomers of proteins and 20 amino acids are the building blocks in the synthesis of the different polypeptide chains. The codes for the amino acid sequence in polypeptides and proteins lie in the genes of DNA and must be copied to direct their synthesis. In order for a gene to be expressed a copy must be made from the DNA template. This copying process is called transcription and the subsequent production of a particular amino acid sequence in a polypeptide chain is called translation.
Transcription
Prokaryotic transcription occurs in the cytoplasm and eukaryotic transcription takes place in the nucleus. The description or central dogma of the flow of information from genetic material was first introduced by Francis Crick in 1958 (Figure 3.39). It states that DNA transfers information to RNA and RNA then controls protein synthesis. Or, DNA makes RNA and RNA makes protein. DNA also controls its own replication (see DNA Replication, later in this chapter). The structures of DNA and RNA are discussed in Chapter 2.
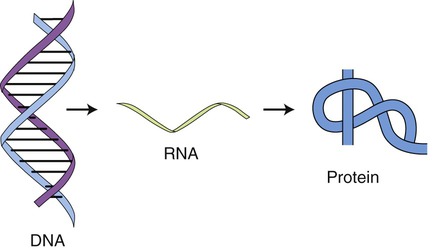
This drawing illustrates the basis of the flow of information in protein synthesis. The genetic information of the DNA is translated and transcribed by RNA to form the protein.
The initiation of transcription is controlled by the enzyme RNA polymerase, which recognizes the beginning of a gene so that it can start mRNA synthesis at a particular DNA sequence that appears at the beginning of genes. This sequence is called a promoter. The promoter is a unidirectional sequence on one strand of the DNA and tells the RNA polymerase where to start and in which direction to continue synthesis. RNA polymerase stretches open the double helix at that point and then begins the synthesis of mRNA. This synthesis of mRNA follows the principle of complementary base pairing (see Chapter 2). Termination of transcription occurs when the polymerase recognizes a DNA sequence known as a terminator sequence. In prokaryotic cells ribosomes can begin protein synthesis with the mRNA immediately, whereas in eukaryotic cells the mRNA must leave the nucleus before it combines with the ribosomes in the cytoplasm. As stated earlier in this chapter, eukaryotic ribosomes may be free in the cytoplasm, or attached to rER.
Each mRNA molecule contains several hundred or more nucleotides complementary to the DNA template. Every three bases form a base triplet or codon and each codon is the code for a specific amino acid. DNA triplets, RNA codons, and their amino acid translation are shown in Table 3.5. However, some amino acids can be translated by different codon sequences as indicated in Table 3.6. As mRNA moves through the ribosome, the sequence of codons translates into a sequence of amino acids.
TABLE 3.5
DNA Base Triplets, mRNA Codons, and Their Amino Acids
DNA Triplet | mRNA Codon | Amino Acid |
TAC | AUG | “Start” (methionine) |
ATC | UAG | “Stop” |
CGT | GCA | Alanine |
GCT | CGA | Arginine |
ACA | UGU | Cysteine |
CCG | GGC | Glycine |
CTC | GAG | Glutamic acid |
GTG | CAC | Histidine |
GAA | CUU | Leucine |
AAA | UUU | Phenylalanine |
GGG | CCC | Proline |
AGG | UCC | Serine |
ACC | UGG | Tryptophan |
TABLE 3.6
Amino Acids Encoded by Multiple mRNA Codons
Amino Acid | mRNA Codons |
Alanine | GCA GCC GCG GCU |
Arginine | AGA AGG CGA CGC CGG CGU |
Asparagine | AAC AAU |
Aspartic acid | GAC GAU |
Cysteine | UGC UGU |
Glutamic acid | GAA GAG |
Glutamine | CAA CAG |
Glycine | GGA GGC GGG GGU |
Histidine | CAC CAU |
Isoleucine | AUA AUC AUU |
Leucine | UUA UUG CUA CUC CUG CUU |
Lysine | AAA AAG |
Methionine | AUG |
Phenylalanine | UUC UUU |
Proline | CCA CCC CCG CCU |
Serine | AGC AGU UCA UCC UCG UCU |
Threonine | ACA ACC ACG ACU |
Tryptophan | UGG |
Tyrosine | UAC UAU |
Valine | GUA GUC GUG GUU |
Translation
Specific enzymes and tRNA translate codons. Like other RNA molecules, tRNA is a single-stranded molecule; but it bends back in on itself to form a cloverleaf structure with an anticodon on one end (Figure 3.40). An anticodon consists of three nucleotides complementary to a specific codon on the mRNA molecule. Specific enzymes (aminoacyl-tRNA synthetase enzymes) in the cytoplasm bind specific amino acids to the ends of tRNA; and a tRNA molecule with a given anticodon can bind to a specific amino acid. Each tRNA molecule is therefore bound to one specific amino acid.
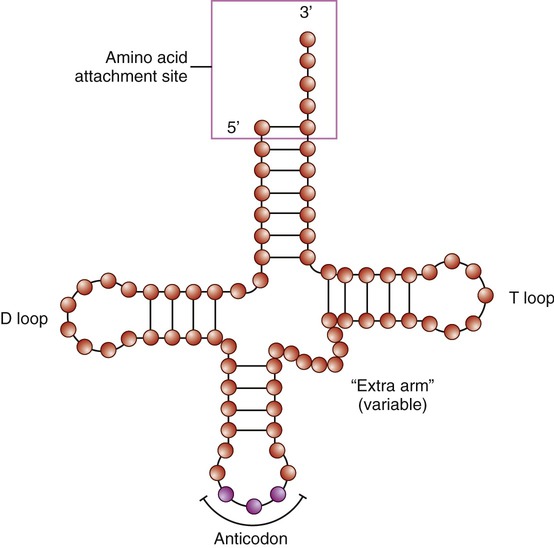
The transfer RNA (tRNA) is a small, cloverleaf-shaped RNA that is the transporting molecule for a particular amino acid. The molecule has an amino acid attachment site (acceptor stem) and a three-base region, the anticodon, that can base pair with the corresponding codon region of messenger RNA (mRNA).
• Initiation: The ribosome assembles on the identified start codon (AUG—methionine) in mRNA and then each sequential base pair forms the next codon. The position of the start codon determines the open reading frame or order of codons that will be read to form a protein.
• Elongation: The first and second tRNAs bring the first and second amino acids close together. The first amino acid detaches from its tRNA and forms a peptide bond with the amino acid of the neighboring second tRNA, forming a dipeptide. When the third tRNA binds to the third codon, its amino acid binds to the second amino acid, which then detaches from its tRNA. The polypeptide chain grows as new amino acids are added to this tripeptide by the same process. This growing polypeptide chain remains attached by only one tRNA to the strand of mRNA (Figure 3.41).
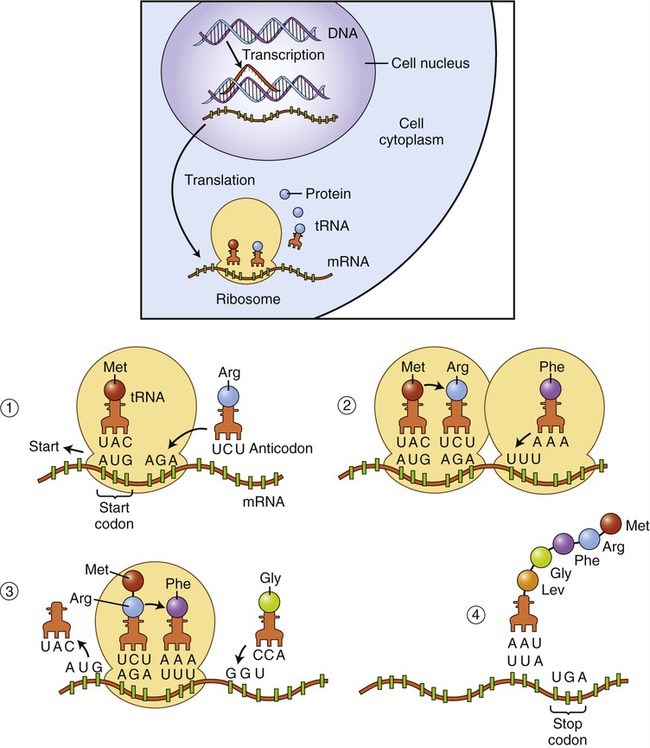
(1) On the ribosome a tRNA molecule carrying the first amino acid and the anticodon combine with the start codon on the mRNA. tRNA carrying the second amino acid is approaching its codon. (2) The ribosome moves along the mRNA so that the second tRNA can attach. When the second tRNA is attached a peptide bond is formed between the first two amino acids and a third tRNA is approaching. (3) Once the peptide bond has formed the first tRNA detaches, allowing the third tRNA to bind to its codon, the adjacent amino acids form another peptide bond, and a fourth tRNA is approaching. (4) This process of forming a peptide chain continues until the appearance of a stop codon.
• Termination: Elongation of the designated protein continues until a “stop” codon (UAA, UGA, or UAG) signals the end of the process. The building of the protein stops because there is no tRNA molecule that is complementary to the stop codon. A releasing enzyme frees the newly formed polypeptide chain from the last tRNA and the mRNA is released from the ribosome.
DNA Replication and Cell Division
DNA Replication
The unwinding of DNA occurs through the action of the enzyme helicase, which separates the two strands of DNA. The site of separation is the replication fork (Figure 3.42). DNA synthesis begins when a short complementary strand of RNA (primer RNA) binds to each parent strand of DNA. The primer RNA serves as the point of attachment for the enzyme DNA polymerase III, which initiates the synthesis of complementary DNA. As the DNA chain lengthens, DNA polymerase I replaces the primer RNA. When the complementary nucleotides are in position opposite each of the nucleotides on the parent strand, covalent bonds are formed between the sugar and phosphate groups of adjacent deoxyribonucleotides on the new chain. Hydrogen bonds form, joining the complementary base pairs between the parental DNA strand and new DNA strand. DNA polymerase can synthesize DNA only in the 5′ → 3′ direction. It is a bidirectional process during which one strand is copied continuously and the other discontinuously. The continuous strand of DNA is the leading strand and the discontinuous strand is the lagging strand. The lagging strand of DNA consists of short stretches of RNA primer plus newly synthesized DNA approximately 100 to 1000 bases long. These DNA fragments are Okazaki fragments that attach to the lagging strand by the action of the enzyme DNA ligase. Replication continues until the entire chromosome is duplicated, forming two identical DNA molecules.
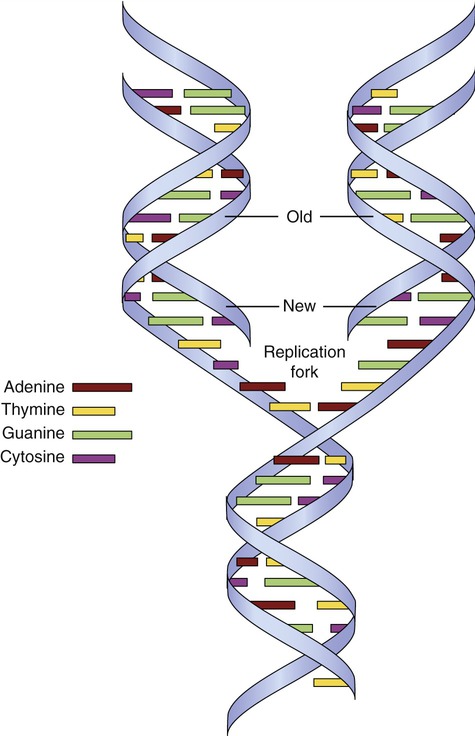
The DNA double helix unwinds, separating the two strands of DNA at the replication fork. New strands of DNA are formed on each old strand by complementary base pairing. Each new DNA molecule therefore contains an old strand and a new strand of DNA.
Cell Division
Binary Fission
The form of asexual reproduction by which all bacteria and most protists reproduce is binary fission. A single cell separates into two identical daughter cells, each containing an identical copy of the parental DNA. This process occurs in a stepwise fashion starting with elongation of the bacterium. Next, the bacterial chromosomal DNA, which is a single circular molecule, replicates. After this, a central transverse septum forms that divides the cell into two daughter cells (Figure 3.43). This type of reproduction normally results in two identical cells. However, bacterial DNA has a relatively high mutation rate, which is a rapid rate of genetic change that is the underlying reason for the development of antibiotic-resistant bacterial strains.
Cell Cycle and Mitosis
Eukaryotic cells undergo a cell cycle or a sequential series of events between cell divisions. The frequency of cell divisions varies between different cell types, and some cells never divide after they differentiate (i.e., nerve cells and muscle cells). The cell cycle consists of four distinct phases: the G1 phase, S phase, G2 phase, and M phase. The G1 phase, S phase, and G2 phase are collectively known as the interphase or the nondividing stage of the cell cycle (Figure 3.44). The M phase is composed of two tightly coupled processes: mitosis and cytokinesis.
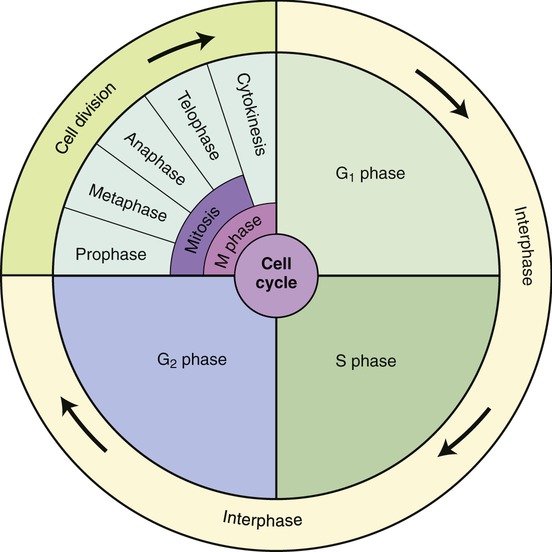
The cell cycle consists of four distinct phases: the G1 phase, S phase, G2 phase, and M phase. The G1 phase, S phase, and G2 phase are collectively known as the interphase or the nondividing stage of the cell cycle. The M phase is composed of two coupled processes: mitosis and cytokinesis (division of the cytoplasm).
• During the G1 phase, cells carry out the metabolic activities characteristic of the tissue to which they belong. In preparation for cell division, a cell might increase the amount of cytoplasm and the number of cell organelles. Chromosomes are in their extended form, and their genes actively direct RNA synthesis. At the end of G1, the cell commits to dividing and has reached the “point of no return.” In yeast it is called START and in multicellular eukaryotes, it is the restriction point.
• During the S phase DNA duplicates.
• During the G2 phase the cell continues to grow and its metabolic activities prepare for mitosis. The quantity of DNA increases and the chromatin condenses to form short, thick structures that indicate the end of the phase. Now, each chromosome consists of two strands called chromatids joined together by a centromere (Figure 3.45). The two chromatids contain identical DNA base sequences because each is produced by semiconservative replication of DNA. Each chromatid becomes a separate chromosome after mitotic cell division.
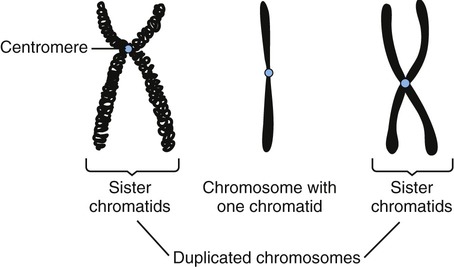
During the G2 phase the duplicated DNA condenses into chromosomes and each chromosome consists of two strands, called chromatids, held together by a centromere. After mitosis each chromatid becomes a separate chromosome.
• During the M phase, mitosis and cytokinesis occur. Mitosis is divided into four stages: prophase, metaphase, anaphase, and telophase (Figure 3.46). The visible characteristics of these phases are as follows:
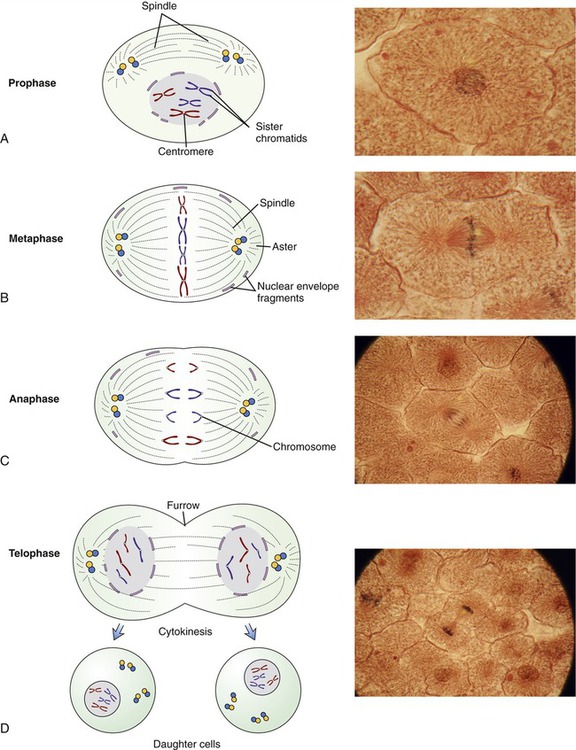
This illustration provides drawings and micrographs of the stages of mitotic cell division of eukaryotic cells. A, Prophase—chromosomes become clearly visible, centrioles migrate to the opposite poles of the cell, and spindle fibers form. B, Metaphase—the chromosomes line up at the equator of the cell, and the nuclear envelope is dissolved. C, Anaphase—the chromosomes split and the sister chromatids separate and are pulled to opposite poles. D, Telophase—the chromosomes are at the opposite poles, a nuclear membrane is formed around them, and the cell membrane forms a distinct furrowing at the cell midline just before cytokinesis.
• Prophase: The chromosomes become clearly visible by light microscopy. The centrioles migrate toward the opposite poles of the cell and each centrosome has spindle fibers extending from it. The nuclear membrane starts to disappear and the nucleolus is no longer visible.
• Metaphase: The chromosomes line up at the equator of the cell. Spindle fibers from each centriole attach to the centromeres of the chromosomes. The nuclear membrane is now absent.
• Anaphase: The centrosomes split and the sister chromatids separate as each is pulled to an opposite pole.
• Telophase: The centrosomes become longer, thinner, and less distinct. New nuclear membranes form and the nucleolus reappears. The cell membrane develops a distinct furrowing at the cell midline. Two separate nuclei are now apparent within the same cell.
• Cytokinesis: The cytoplasm divides to complete cell division.
Meiosis
In meiosis, a single duplication of chromosomes occurs and two cell divisions follow. This type of cell division is diploid → haploid in nature, and occurs only during the production of gametes, that is, sex cells. The diploid cells in the sex organs undergo two cell divisions to produce haploid sex cells. The stages of meiosis subdivide depending on whether they occur in the first or in the second meiotic cell division. These stages are prophase I, metaphase I, anaphase I, and telophase I; and prophase II, metaphase II, anaphase II, and telophase II (Figure 3.47).
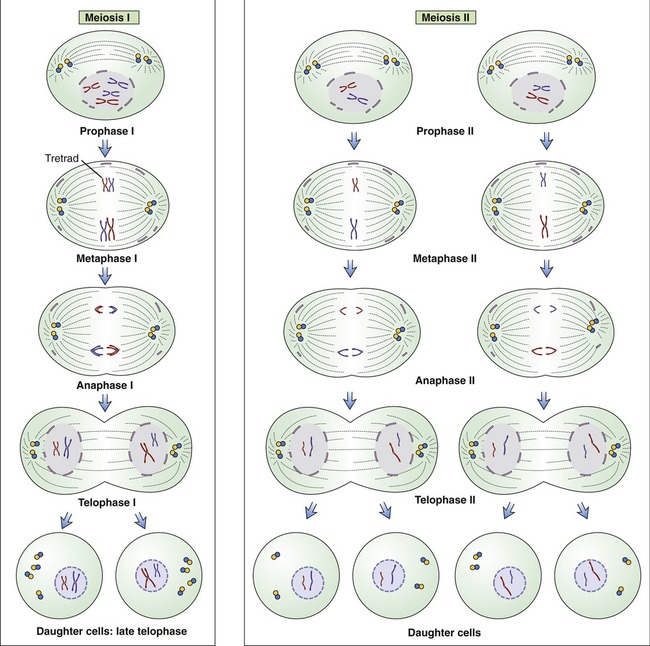
Meiosis occurs in a series of two divisions called meiosis I and meiosis II. During the first meiotic division in metaphase I, the pairs of homologous chromosomes of a diploid parent cell line up with each member facing a given pole of the cell. Maternal and paternal members of the homologous chromosomes randomly shuffle and each daughter cell obtains one complement from the homologous chromosome pair. During the second meiotic division, with each of the chromosomes containing duplicate strands (chromatids), the strands split and each daughter cell receives a haploid number of chromosomes. Note that each parental cell undergoing meiotic division produces four daughter cells.
• During the first meiotic division in metaphase I, the pairs of homologous chromosomes of a diploid parent cell line up with each member facing a given pole of the cell. Maternal and paternal members of the homologous chromosomes randomly shuffle and each daughter cell obtains one complement from the homologous chromosome pair.
• During the second meiotic division, with each of the chromosomes containing duplicate strands (chromatids), the strands split and each daughter cell receives a haploid number of chromosomes.
Summary
• The cell is the basic structural and functional unit of all living organisms. Two main categories of cells exist: prokaryotes, the cells without a nucleus, and eukaryotes, cells with a membrane-bound nucleus.
• All cells are surrounded by a plasma membrane composed of a phospholipid bilayer and embedded proteins. Functionally, the plasma membrane is selectively permeable and controls the movement of particles in and out of the cell. Many cells have a matrix surrounding the plasma membrane, the multifunctional glycocalyx.
• A cell wall is present around the plasma membrane of many types of cells. The bacterial cell wall is a unique structure, which maintains the rigid shape of bacteria and protects them from hostile environments. On the basis of the composition of their cell wall, most bacteria when stained with the Gram stain, are either gram-positive or gram-negative. This classification provides a major tool for the identification of bacteria.
• Surface appendages are present in both prokaryotic and eukaryotic cells. Prokaryotic cells can have pili or flagella, whereas cilia, flagella, and microvilli are common in eukaryotic cells.
• Biofilms are collections of surface-associated microbes enclosed by an extracellular matrix. Biofilms can form on a wide variety of surfaces and can be the source of product contamination and microbial infections.
• Enclosed by the plasma membrane is the cytoplasm, a gelatinous matrix and the site for biochemical activities of a cell. Within the cytoplasm are the nucleus, other cell organelles, inclusions, and the supportive cytoskeleton.
• Prokaryotic cells and unicellular organisms are in direct contact with the external environment, whereas the tissues of multicellular organisms are in contact with extracellular fluid (ECF) collectively referred to as the extracellular fluid compartment. The fluid inside the cells is the intracellular fluid or ICF, which collectively is the intracellular fluid compartment.
• Cells exhibit passive and active transport mechanisms by which they can move materials across the plasma membrane. These mechanisms include diffusion, facilitated diffusion, osmosis, filtration, pump transport, endocytosis, and exocytosis.
• Cellular metabolism includes all chemical reactions in a cell and is organized into metabolic pathways. Metabolic pathways that break down molecules and release energy are part of catabolism, and pathways that produce larger molecules from smaller ones, using energy, are anabolic pathways.
• All metabolic pathways require biological catalysts, called enzymes, to initiate biochemical reactions. Enzymes are proteins that often require nonprotein compounds such as coenzymes or cofactors for action.
• Prokaryotic and eukaryotic cells metabolize nutrients to generate ATP by respiration, fermentation, and photosynthesis. Cellular respiration in the presence of oxygen is aerobic cellular respiration with glycolysis, the Krebs cycle, and the respiratory chain. In the absence of oxygen, cells utilize anaerobic cellular respiration including fermentation. Some cells and organisms can convert light energy into chemical energy via ATP, in a process called photosynthesis.
• Cellular structure and function depend on many different types of proteins. Proteins are composed of different sequences of amino acids and the amino acid sequence of a given protein is encoded in the cell’s DNA. The process of protein synthesis requires RNA and involves two processes: transcription and translation.
• Before a cell divides, it duplicates its DNA to ensure that each cell after division contains identical DNA. This process is called replication or DNA synthesis.
• The different classes of cells use different processes for cell division. Prokaryotic cells, that is, bacteria, divide by binary fission and eukaryotic cells divide by mitosis and meiosis.
Review Questions
1. Which of the following is not found in all bacterial cells?
2. Bacterial capsules are important in:
3. The cell organelles responsible for the packaging of proteins are the:
4. The cell organelle found only in algae and plant cells is the:
5. The intracellular fluid has a high concentration of:
6. Which of the following is an active transport mechanism?
7. Which of the following is an enzyme?
8. The compound that enters the Krebs cycle and combines with oxaloacetic acid is:
9. The anticodons are located in:
10. In which phase of mitosis do the chromatids separate?
11. Cells without a nucleus are __________ cells.
12. The sterol-like molecules in bacterial plasma membranes are __________.
13. A cell organelle that contains digestive enzymes is a(n) __________.
14. The allosteric site is present in __________.
15. The organelle necessary for photosynthesis is a(n) __________.
16. Compare and contrast prokaryotic and eukaryotic cells.
17. Describe the cell wall of gram-positive and gram-negative cells.
18. Discuss the regulation of enzyme activity.
19. Name and describe the pathways of aerobic cellular respiration.