Chapter 88 Anterior Horn Cell and Cranial Motor Neuron Disease
Hereditary Motor Neuron Diseases
The Anterior Horn Cells of Spinal Cord
Anterior horn cells (α-motor neurons), located in the anterior gray matter of the spinal cord, are found at every segment and are concentrated in the cervical and lumbosacral enlargements. Morphologic differentiation of the anterior horn cells is most evident from 12 to 14 weeks’ gestation [Vassilopoulos and Emery, 1977]. There is a period of normal differentiation, followed by programmed cell death [Yachnis et al., 1998; Fidzianska and Rafalowska, 2002]. Anterior horn cells are clustered into medial and lateral cell divisions (Figure 88-1). The medial group is subdivided into ventromedial and dorsomedial components. The ventromedial component innervates the superficial larger muscles, and the dorsomedial component innervates the small, deep muscles adjacent to the spine.
The lateral cell mass is also subdivided into groups. The ventrolateral group innervates extensor muscles, and the centrodorsal group innervates flexor muscles [Romanes, 1951]. Since these groups of neurons are located in a relatively small region, deleterious influences harm cells from more than one group and weakness may be widespread.
Autosomal-Recessive Spinal Muscular Atrophy Linked to the SMN1 Gene
Clinical Features of Spinal Muscular Atrophy
Spinal muscular atrophy (SMA) is the most common autosomal-recessive motor neuron disease of childhood, characterized by degeneration of the spinal cord and brainstem motor neurons, resulting in hypotonia and muscle weakness. Previously, SMA was diagnosed by electromyography (EMG) and muscle biopsy. EMG characteristics of SMA include spontaneous activity with positive sharp waves, fasciculation, and fibrillation. Compound muscle action potential demonstrates high amplitudes and long durations coupled with decreased recruitment. Histopathology of skeletal muscle documents atrophic fibers with islands of group hypertrophy, and the spinal cord documents severe motor neuron loss in the anterior horn region (Figure 88-2).
SMA is divided into three clinical types, based on age of onset and motor function achieved: the most severe type I, the intermediate type II, and the mild type III [Munsat and Davies, 1992]. Recently, an adult-onset type IV SMA was added to include very mild SMA (Table 88-1) [Russman, 2007; Wang et al., 2007].
Type i spinal muscular atrophy
Type I SMA (Werdnig–Hoffman disease) is the most severe subtype, which accounts for approximately 50 percent of patients diagnosed with SMA [Markowitz et al., 2004]. Disease onset is usually before 6 months of age, and death occurs within the first 2 years of life. Profound hypotonia, symmetric flaccid paralysis, and poor head control are common clinical features of these patients. They are unable to sit without support (Figure 88-3). The spared diaphragm, combined with weakened intercostal muscles, results in paradoxical breathing (inward thorax movement with outward abdominal movement during inspiration) and a bell-shaped upper torso. Bulbar involvement results in tongue fasciculation and poor suck and swallow. It also leads to decreased airway protection and increased risk of aspiration pneumonia. Death is usually caused by airway complications.
Type iii spinal muscular atrophy
Type III SMA (Kugelberg–Welander disease) patients exhibit extreme symptom heterogeneity. These patients all achieve independent walking, but some may require wheelchair assistance in childhood, while others continue to walk and live productive adult lives with minor muscular weakness (Figure 88-4). Scoliosis often develops in these patients but later than type II SMA. Additionally, joint overuse symptoms are frequently seen.
Molecular Genetics and Pathogenesis of Spinal Muscular Atrophy
In 1990, researchers used linkage analysis to identify the SMA gene locus on chromosome 5q13 [Brzustowicz et al., 1990; Melki et al., 1990]. The initial10-centimorgan (cM) interval was later narrowed to a 1- to 2-cM region using recombinant mapping [Brzustowicz et al., 1992; Clermont et al., 1994; Wang et al., 1995]. In 1994, Melki and colleagues uncovered a small 11-kb fragment that is uniquely missing in SMA patients, thereby further narrowing the candidate genetic region. A human fetal brain complementary deoxyribonucleic acid (cDNA) library was probed by genomic DNA from this region, and the “survival motor neuron” (SMN) gene was identified as the disease-causing gene in 1995 [Lefebvre et al., 1995]. The SMN gene is present in multiple copies in the human genome: one SMN1 (SMNT, telomeric) and several SMN2 (SMNC, centromeric) (Figure 88-5). The two genes differ by only five nucleotides, two of which are within the 1.7-kb coding region, but none affects the predicted amino acid sequence [Melki et al., 1994; Schmutz et al., 2004]. Both genes contain nine exons and eight introns that span an approximately 20-kb genomic region. The SMN1 gene encodes a 38-kDa, 294-amino acid protein. The SMN protein is ubiquitously expressed in all somatic tissues and is highly conserved from yeast to humans [Lefebvre et al., 1995; Burglen et al., 1996; Schrank et al., 1997]. The SMN gene duplication found in primates occurred after the split of rodents from primates, as mice have only one copy denoted Smn. The SMN2 gene is unique to humans because nonhuman primates (e.g., chimpanzee) do not possess this gene signature, despite having multiple SMN gene copies in the genome [Rochette et al., 2001].
Over 98 percent of SMA patients possess a homozygous deletion or mutation of the SMN1 gene [Hahnen et al., 1995; Lefebvre et al., 1995]. All SMA patients, however, retain at least one copy of SMN2. SMN2 undergoes alternative splicing and produces a truncated messenger ribonucleic acid (mRNA) isoform lacking exon 7. A C-to-T nucleotide transition at position 6 of exon 7 (Ex7+6) is responsible for the alternatively spliced isoform lacking exon 7 (Figure 88-6) [Lorson et al., 1999; Lorson and Androphy, 2000]. The resultant SMNΔ7 protein is nonfunctional and thought to be rapidly degraded [Lorson and Androphy, 2000; Chang et al., 2004; Vitte et al., 2007]. Approximately 25 percent of SMN2 pre-mRNA is properly spliced and subsequently translated into full-length SMN protein. This low level of SMN protein permits embryonic development but is not sufficient to sustain the survival of spinal cord motor neurons; thus, the occurrence of SMA.
Cellular Function of SMN Protein
SMN protein is expressed in the cytoplasm and nucleus in all somatic cells, with high levels in motor neurons of the spinal cord [Battaglia et al., 1997; Coovert et al., 1997]. Within the cytoplasm, SMN protein localizes to several small discrete structures associated with coiled (Cajal) bodies named “gems” (“gemini of coiled bodies”) [Liu and Dreyfuss, 1996; Carvalho et al., 1999]. While the exact cellular function of gems remains unknown, cells from SMA patients contain significantly fewer gems compared with those in gene carriers and non-SMA controls [Coovert et al., 1997; Grzeschik et al., 2005]. In eukaryotic cells, the spliceosome is the cellular organelle that executes post-transcriptional pre-mRNA splicing. Uridine-rich small nuclear ribonuclear proteins (U snRNPs), the principal components of spliceosomes, recognize highly conserved sequences and ligate exons together. SMN protein, part of a highly stable complex with at least eight other proteins (reviewed in Otter et al. [2007]), is necessary and sufficient for proper assembly of Sm (Smith class) core proteins in the U snRNP [Meister et al., 2001; Pellizzoni et al., 2002]. A point mutation (E134K) in the SMN tudor domain, the region that mediates Sm protein assembly [Buhler et al., 1999], can lead to SMA disease by affecting the charge distribution in the SMN-Sm binding site.
While SMN protein is ubiquitously expressed in all somatic cells, it is puzzling why the spinal cord motor neurons are specifically vulnerable in SMA. Recent studies suggest that SMN protein may play an important role in certain cellular functions that are unique to motor neurons. SMN protein was recently found to localize in RNP granules in the neurites and growth cones of primary motor neurons and in embryonic stem cell-derived motor neurons [Fan and Simard, 2002; Zhang et al., 2006]. Active bidirectional transport of these granules to neuronal processes and growth cones was observed by live-cell fluorescence microscopy [Zhang et al., 2006]. SMN protein also interacts with heterogeneous nuclear ribonucleoprotein (hnRNP) R and Q [Mourelatos et al., 2001; Rossoll et al., 2003]. The former interacts with the 3′ untranslated region (UTR) of β-actin mRNA [Rossoll et al., 2002]. Low levels of SMN protein resulted in low β-actin mRNA and protein levels in axons and growth cones [Rossoll et al., 2003], further suggesting that SMN protein may be involved in transportation of RNP complexes containing β-actin. Beta-actin deficiency results in motor neuron axon outgrowth and pathfinding defects in two neuronal culture models [Zhang et al., 2003] and in a zebrafish model of SMA [McWhorter et al., 2003]. Profilin, an actin-binding protein, regulates actin dynamics through sequestration and release of actin monomers. SMN protein co-localizes with profilin IIa (the predominant isoform in neuronal tissue [Lambrechts et al., 2000]) in distinct granules in neurite-like extensions and growth cones. Mutant SMN protein fails to interact with profilin IIa, and knocking down profilin IIa alone inhibits neurite outgrowth [Sharma et al., 2005].
Other Hereditary Diseases Affecting Spinal Motor Neurons
Other children with proximal weakness onset at birth or within the first year of life also manifest anatomic and electrodiagnostic evidence of motor neuron disease not linked to the SMN gene [Guillot et al., 2008]. Additional or atypical features are present in these children. Several of these conditions are now well characterized and genes have been identified (Table 88-2). Pontocerebellar hypoplasia (PCH1) with SMA was first described by Norman in an infant who experienced cognitive decline and died at 6 months [Norman, 1961]. The gene responsible for this autosomal-recessive disease was subsequently mapped to 14q32. In 2009, the responsible gene (vaccinia-related kinase 1) was determined by Renbaum et al. [Renbaum et al., 2009]. Scapuloperoneal SMA was first described clinically by Emery prior to the identification of the SMN gene, and Mawatari recognized sporadic and X-linked cases [Emery et al., 1968; Mawatari and Katayama, 1973]. More recently, a clinically similar syndrome with onset in the first decade of life and slow progression has been linked to chromosome 12 with autosomal-dominant inheritance [DeLong and Siddique, 1992; Isozumi et al., 1996].
Table 88-2 Rare and Atypical Forms of Proximal or Bulbar Spinal Muscular Atrophy Not Linked to the SMN Gene
Arthrogryposis Multiplex Congenita and Motor Neuron Diseases
Arthrogryposis multiplex congenita, a syndrome manifesting at birth, is characterized by multiple, fixed contractures of large joints. The pathologic features of arthrogryposis cover a broad spectrum [Banker, 1985, 1986, 1994]. General and genetic classifications have been proposed [Hall, 1997]. Fetal hypokinesia or restricted movement in utero of any cause will result in congenital contractures. Congenital anomalies of the brain are common in this group of children (e.g., hydrocephalus, hydranencephaly, microcephaly) [Hageman et al., 1987; Hennekam et al., 1991], and may be accompanied by anomalies of other organs [Vlaanderen et al., 1991]. Inheritance of anterior horn cell arthrogryposis, when determinable, is most often autosomal-recessive. However, X-linked and autosomal-dominant modes of transmission have been reported [Fleury and Hageman, 1985].
Anterior horn cell arthrogryposis results from a marked decrease of the anterior horn cell population in the cervical and lumbar spinal cord enlargements. More than 90 percent of patients with arthrogryposis have anterior horn cell-related disease [Banker, 1985; Adams et al., 1988]. Most affected infants hold their arms rotated inwardly; elbow position varies. The forearms are held pronated, with ulnar deviation at the wrists. The hands are flexed, and the fingers are curled tightly. The thighs are usually externally rotated and flexed at the hips. There is either flexion or extension of the knees, but the feet are almost always positioned in an equinovarus deformity (Figure 88-7). The limbs are slender (Figure 88-8); conversely, the joints appear greatly enlarged (Figure 88-9). The patellae may be very small or absent. Other abnormalities may include inguinal hernias, cleft palate, scoliosis, ankylosis of the temporomandibular joint, and cardiac malformations [Drachman and Banker, 1961].
Passive movement of the affected joints is possible, but only a small range of motion is present. Deep tendon reflexes are not elicitable, and electromyographic examination confirms electrical abnormalities consistent with anterior horn cell impairment. The available pathologic studies of the involved muscles revealed markedly decreased bulk [Drachman and Banker, 1961] or even absence [Adams et al., 1988]. Hip contractures develop from unopposed contraction of the iliopsoas muscles. Muscle spindles are normal. Few pathologic alterations are evident in endomysial connective tissue, but perimysial connective tissue may be decidedly increased. One affected member each in two sets of identical twins has been reported [Drachman and Banker, 1961]. Children with arthrogryposis associated with anterior horn cell disease present in infancy may demonstrate autosomal-recessive, sporadic, or X-linked inheritance. The genetically defined syndromes are described in Table 88-2. Pathological features of arthrogryposis multiplex congenita associated with anterior horn cell dysfunction include infants from two families who had flexed elbows, extended wrists, acute flexion of the knee and hip joints, and bilateral pes equinovarus [Pena et al., 1968; Hooshmand et al., 1971]. Postmortem examination documented nodular fibrosis of the anterior spinal roots along the entire length of the spinal cord and absence of myelin and axis cylinders. Central chromatolysis was evident in a few anterior cells; the morphologic changes were believed to be secondary to the anterior root lesions. The skeletal muscle displayed neurogenic muscular atrophy. Identification of muscle tissue in some areas was impossible because of extensive replacement by fat [Pena et al., 1968].
A rare form of lethal arthrogryposis multiplex congenita related to abnormal Schwann cell development has been reported [Charnas et al., 1988]. Contractures have accompanied neonatal myasthenia gravis, although anticholinesterase therapy does not improve them [Holmes et al., 1980; Smit and Barth, 1980].
Prenatal diagnosis using ultrasound [Stoll et al., 1991; Bonilla-Musoles et al., 2002] is now common. Newborns with arthrogryposis may also have myotonic dystrophy [Sarnat et al., 1976]. The lower extremities are predominantly involved. These infants often have loose, wrinkled skin and a paucity of subcutaneous tissue. Neck muscle weakness precludes normal neck flexion. Serum creatine kinase activity may be elevated. Diagnosis can be confirmed by blood DNA analysis.
Considering the differential diagnosis, it is not surprising that muscle pathology varies greatly in arthrogryposis patients. All of the congenital myopathies have been associated with arthrogryposis, including central core disease, nemaline myopathy, and centronuclear myopathy, as well as congenital muscular dystrophy [Moerman et al., 1985; Schmalbruch et al., 1987; Quinn et al., 1991]. Selective distal arthrogryposis is more likely to be caused by specific muscle mutations [Sung et al., 2003a, b]. Administration of anesthesia during surgical intervention may be complicated by malignant hyperthermia, especially in children with muscular dystrophy or central core disease [Baines et al., 1986].
Arthrogryposis has been reported in Alaskan Eskimos, named Kuskokwim disease for the river delta on which the original cases were diagnosed [Petajan et al., 1969]. Multiple contractures of the joints, commonly of the knees and ankles, characterize the disease. The primary pathologic process is associated with the connective tissue. There is no indication of myopathy, neuropathy, or motor cell disease. The disease manifests in the first decade of life and is transmitted as an autosomal-recessive trait. Contractures at the elbows and knees may be seen during the first few months of life. Accompanying internal tibial torsion and profound planovalgus occur. Flexion hip contractures are also present. The deep tendon reflexes are usually elicitable in the biceps and Achilles tendons, but are frequently absent in the triceps and quadriceps tendons. Although one infant had a small cataract at 3 years of age, cataracts are uncommon in adults. Radiographic examinations have revealed cysts in the proximal long bones of several patients. Other patients have had pigmented nevi and diminished corneal reflexes. This form of arthrogryposis may be associated with webbed-neck or Klippel–Feil deformity, and with anomalies of the genitourinary system [Beckerman and Buchino, 1978].
New information describing genes that control development of limb buds suggests that abnormalities in transcription factors may be theoretically responsible for some cases of arthrogryposis [Roberts and Tabin, 1994; Nelson et al., 1996]. In such cases, there would be no evidence of muscle or nerve disease but muscle development in the limbs may be severely defective or absent (amyoplasia) [Sells et al., 1996]. To date, no such mutations have been found. On the other hand, mutations in contractile proteins, such as tropomyosin 2 and troponin I, have been documented to cause amyoplasia or distal arthrogryposis [Sung et al., 2003a, b]. Mutations in HOXD10 and homeobox PITX1 have also been described in isolated distal vertical talus [Dobbs et al., 2006] and clubfoot, respectively [Gurnett et al., 2008].
Orthopedic procedures performed on patients with arthrogryposis often provided sufficient alignment and stability of the legs to allow independent ambulation [Fisher et al., 1970; Bennett et al., 1985; Guidera and Drennan, 1985]. Talectomy before walking ability is acquired may prove beneficial [Solund et al., 1991]. Scoliosis may be a prominent problem and may require surgical correction [Daher et al., 1985]. Some improvement in arm and hand function is usually possible with physical therapy and exercise. Anesthesia for these patients requires special consideration [Baines et al., 1986; Oberoi et al., 1987]. Otolaryngologic management may be necessary for poor suck reflex, omega-shaped epiglottis, airway compromise, achalasia, and micrognathia [Laureano and Rybak, 1990].
Motor Neuron Diseases Involving Bulbar Functions
In progressive bulbar paralysis of childhood, Fazio–Londe disease, motor neurons in cranial nerve nuclei may be progressively impaired and decreased in number, with resultant progressive bulbar paralysis and little or no accompanying anterior spinal cord involvement [Gomez et al., 1962]. While the condition may be genetically transmitted [Fazio, 1892; Londe, 1893, 1894], sporadic cases are more common [McShane et al., 1992; Suresh and Deepa, 2004] and there has been no gene identified. Age of onset varies and may be as early as 3 years. Bulbar paralysis is only one facet of progressive motor neuron disease in many of these patients, and pathologic study confirms widespread degenerative changes in the brainstem. Morphologic involvement of anterior horn cells has been demonstrated, despite the absence of clinical signs [Alexander et al., 1976]. Involvement of the anterior horn cells in the cervical and upper thoracic cord segments is often associated with demonstrable loss of neurons in other locations, including decreased neuronal population in the dentate nucleus. Of the cranial nerves, cranial nerve VII is almost always affected. Cranial nerve XII is usually affected pathologically, and clinical manifestations are apparent in the early-onset patients. The nuclei of cranial nerves III, IV, VI, and X may also be involved. Clinical impairment of extraocular movement is rare.
Juvenile-onset bulbospinal muscular atrophy associated with deafness (Brown–Vialetto–Van Laere syndrome) manifests late in the first decade or in the second decade [Brown, 1894; Vialetto, 1936; Van Laere, 1966]. Bulbar impairment is indicated by dysarthria, dysphagia, and facial diplegia [Gallai et al., 1981; Summers et al., 1987].
Spinal and bulbar muscular atrophy (kennedy disease)
Kennedy disease or spinal and bulbar muscular atrophy (SBMA) was first described in 1968 [Kennedy et al., 1968]. It is a rare X-linked motor neuron disease that causes proximal weakness, bulbar weakness with dysphagia and aspiration, asymmetric or symmetric facial weakness, and gynecomastia. Age of onset is variable, and diagnosis may not be possible until gynecomastia develops after the fourth decade [Atsuta et al., 2006]. Fischbeck et al. localized the gene in seven families to Xq12–22 [Fischbeck et al., 1986]. Subsequently, the human androgenic receptor gene (hAR) was localized to Xq11–12, and examination of hAR in SBMA patients demonstrated that there was an abnormal expansion of exon 1 to more than twice normal size [Choi et al., 1993]. The expansion was found to be caused by CAG repeats, normally numbering 15–31 in hAR, but numbering more than 40 in the SBMA mutation [La Spada et al., 1991; Belsham et al., 1992; La Spada et al., 1994].
While the age of genetic diagnosis is usually in the fourth of fifth decade of life when weakness is present, the earliest symptoms may be present in the second decade of life and include gynecomastia, muscle cramps, and fatigue [Sperfeld et al., 2002]. This weakness is slowly progressive and involves bulbar musculature, including a weak, atrophic tongue. There are no signs of spinal cortical tract dysfunction; deep tendon reflexes are diminished or normal. Fasciculations and cramps in limb muscles are common. Hypesthesia and decreased vibratory sense are present in about half of cases. There may be mild elevation of muscle enzymes, but electrodiagnostic study and muscle biopsy are indicative of motor neuron disease. Electrodiagnostic studies indicate that motor involvement correlates directly with CAG repeat length, but sensory involvement corresponds inversely with CAG repeat length [Suzuki et al., 2008]. Once the clinical phenotype is recognized in a patient, diagnosis may be confirmed by mutation analysis in a blood sample.
Möbius’ syndrome
First described in 1898 by Thomas, Möbius’ syndrome is a congenital, static condition manifested by facial palsy (often bilateral) and involvement (usually bilateral) of cranial nerve VI [Thomas, 1898]. The lower face is usually less involved than the upper face. Impaired lateral rectus muscle contraction results in unopposed medial rectus muscle action, with resultant convergence (Figure 88-10). Conjugate eye movements in the vertical plane are usually present, although ptosis may also occur. Dysfunction of other cranial nerves, including cranial nerves V, X, XI, and XII, often accompanies the abnormalities of cranial nerves VI and VII. Associated absence of the pectoral muscles may occur. The clinical findings are correlated with failure of development or degeneration of the involved cranial nerve motor cells. When three different gene loci were identified, there was a change in nomenclature to reflect this (Möbius’ syndromes 1, 2, and 3 (Table 88-3). However, additional pathological studies suggested that Möbius’ syndrome 1 demonstrates more hypoplasia of the entire brainstem. Thus, the nomenclature concurrently used often refers to Möbius types 2 and 3 as hereditary facial nerve palsy (HFNP) types 1 and 2 [Verzijl et al., 2005]. Acquired Möbius’ syndrome has also been associated with exposure to cocaine or misoprol in utero [Kankirawatana et al., 1993; Shepard, 1995; Leong and Ashwell, 1997; Pastuszak et al., 1998; de Muelenaere, 1999; Vargas et al., 2000; Goldberg et al., 2001; Marques-Dias et al., 2003]. While the differential diagnosis includes non-motor neuron involvement, such as supranuclear or myopathic conditions like facial scapulohumeral dystrophy, myotubular myopathy, or myotonic dystrophy, other clinical features usually will distinguish Möbius’ syndrome from these.
Amyotrophic lateral sclerosis
While most ALS occurs in a sporadic fashion, multiple genes or gene loci have now been identified in familial ALS, which is present in about 10 percent of all patients (Table 88-4). In 1991, Siddique et al., in international collaboration, reported the linkage of one form of familial amyotrophic lateral sclerosis to 21q22.1–22.2 in 23 families. SOD, a gene that encodes a cytosolic Cu/Zn-binding superoxide dismutase, is causative in ALS1 [Deng et al., 1993; Rosen et al., 1993]. The role of superoxide dismutase in controlling free radical tissue levels made it a logical choice as a candidate gene in familial ALS. Superoxide is known to be cytotoxic, but the accumulation of superoxide by an inhibition of superoxide dismutase may be exacerbated by activation of excitatory neurotransmitters, such as glutamate [Coyle and Puttfarcken, 1993]. Transgenic mouse models have helped understanding of novel specific pathogenic mechanisms. While SOD1 knockout mouse has no weakness, severity of weakness in this model is related to transgene copy number. Mice with high copy numbers develop progressive weakness and motor neuron loss, resulting in death [Borchelt et al., 1998; Shibata, 2001].
In addition to ALS 1 caused by SOD, other specific genes or gene linkages are associated with adult-onset disease (see Table 88-4). Sporadic and familial types of ALS are clinically indistinguishable. Familial ALS has been studied to understand pathogenesis. There are three well-recognized ALS syndromes with onset in the first two decades of life. ALS 2 is an autosomal-recessive disease due to mutations in the Alsin gene [Hadano et al., 2001; Yang et al., 2001]. Alsin is a member of the guanine nucleotide exchange factors for the small guanosine triphosphatase, RAB5, and plays a role in endosomal trafficking [Hadano et al., 2006]. Children present in the first decade of life with spasticity involving face and limbs, and may develop pseudobulbar affects. Symptoms are very slowly progressive, with difficulty walking after age 40. An allelic condition, progressive lateral sclerosis with onset in infancy, has also been found to be associated with mutations in this gene [Eymard-Pierre et al., 2002; Gros-Louis et al., 2003; Verschuuren-Bemelmans et al., 2008]. Alsin-deficient mice experience progressive axonal degeneration [Yamanaka et al., 2006].
The second juvenile-onset form of ALS is ALS 4, an autosomal-dominantly inherited distal motor neuronopathy with pyramidal symptoms [Chance et al., 1998]. Symptoms begin in the second decade of life with difficulty walking, and examination indicates both upper and lower motor neuron involvement. Bulbar symptoms are infrequent. Electrophysiology confirms localization to the level of the anterior horn cell [Rabin et al., 1999]. The clinical course is one of slow progression, with use of wheelchair by fifth or sixth decade and a normal life span. Recently, causative mutations in the Senataxin gene (SETX) have been demonstrated. While the precise function is unknown, the senataxin gene has homology to genes involved in RNA processing [Chen et al., 2004]. Allelic variants include autosomal-recessive ataxia oculomotor apraxia type 2 [Moreira et al., 2004]. The third form of ALS with childhood onset is ALS 5, localized to chromosome 15q15.1–q21.1; this type is also recessively inherited. No specific gene has been identified. This form of juvenile ALS has been described in multiple ethnic groups from North Africa, South Asia, and Europe. There are two clinical subtypes. Onset for both is in the second decade of life. Type 1 manifests arms more involved than legs and bulbar symptoms developing late. Type 2 is characterized by much more spasticity and much more prominent involvement of the legs, and may be a form of familial spastic paraparesis [Hentati et al., 1998].
Distal Spinal Muscular Atrophy and Hereditary Motor Neuropathy
Informative families and molecular genetics have allowed researchers to identify multiple distal forms of SMA (neuronopathy) that may be difficult to distinguish clinically from hereditary motor neuropathies. Since positional cloning has been developed, several disease-associated genes have been identified for autosomal-dominant, autosomal-recessive, and X-linked distal hereditary motor neuropathy (reviewed by Irobi et al. [Irobi et al., 2006]). Chromosomal linkage is present for many others (Table 88-5). Many of these identified genes code for housekeeping functions, including RNA processing, apoptosis, and stress response, suggesting that the motor neuron is particularly vulnerable to these defects [Irobi et al., 2006]. Localization to the motor neuron is suspected when the clinical picture demonstrates distal weakness and atrophy with normal sensation. It is confirmed with electrodiagnostic studies that show normal or near-normal sensory studies, normal conduction velocities, but chronic denervation on electromyography testing.
Motor Neuron Diseases of Unknown Etiology
Monomelic Amyotrophy (Hirayama’s Disease)
In 1959, Hirayama et al. described a form of monomelic atrophy in young Japanese patients [Hirayama et al., 1963]. This disorder has also been called O’Sullivan–McLeod syndrome for description of similar patients several years later [O’Sullivan and McLeod, 1977]. This form of sporadic disease occurs predominantly in young men (10:1 ratio) and involves the hand and forearm. While it is usually unilateral, it can be bilateral, with progressive weakness over 1–4 years, followed by a plateau. A few familial cases have been described [Schlegel et al., 1987; Tandan et al., 1990; Nalini et al., 2004] but no causative gene is known. The precise pathogenesis is not known but recent imaging evidence suggests neck flexion may induce cervical myelopathy (Figure 88-11), and pathology of the dura mater obtained at surgery suggests that elastic fibers are decreased in patients [Yoshiyama et al., 2010].
Benign Congenital Hypotonia
When tone is examined in infants and young children, both axillary and appendicular tone should be assessed. Infants with central nervous system injury often demonstrate decreased axial tone but increased appendicular tone after the first months of life. However, in the first months, tone in both areas may be decreased in these infants. In contrast, children with hypotonia secondary to disorders of spinal cord (SMA), nerve, neuromuscular junction, or myopathy usually maintain low axial and appendicular tone and have clear weakness throughout infancy and childhood. The initial reports of children with “benign congenital hypotonia” cited 17 infants with hypotonia at birth or in the first months of life. Eight improved completely by 10 years, and the other 9 improved but had definite muscle weakness or severe ligamentous laxity. All 8 children who recovered had deep tendon reflexes when initially examined, although 3 had reflexes that were difficult to elicit [Walton, 1957]. While, there was no clinical evidence of muscular dystrophy, infantile spinal muscular atrophy, mental deficiency, or upper motor neuron disease in these children, diagnostic studies were limited. Just 2 had muscle biopsies with hematoxylin and eosin staining only [Walton, 1957]. Children with “benign hypotonia” may have various conditions, including nonprogressive congenital myopathies identified with more detailed testing [Spagnoli et al., 1985; Thompson, 2002]. Some of these disorders have clinical or hereditary implications, making these specific diagnoses important. Other children with hypotonia in infancy without weakness may have cognitive difficulties later [Dubowitz, 1968; Zellweger, 1983; Shuper et al., 1987]. Thus, some suggest that this diagnosis be eliminated or be replaced with the term central hypotonia because the prognosis is not necessarily benign. The cause in those children without a neuromuscular diagnosis is likely related to improper modulatory influences on the gamma loop from the central nervous system. As the brain matures, typical motor deficits may develop that are consistent with a diagnosis of cerebral palsy. The condition may be accompanied by cognitive deficit [Dubowitz, 1968]. In short-term follow-up to age 3 years, 44 percent of 36 children still had hypotonia and gross motor delay, and a small number had speech delay [Shuper et al., 1987]. One recent follow-up of 25 children with a clinical diagnosis of benign congenital hypotonia demonstrated that, by age 6–8 years, these children still had inferior gross motor performance for coordination and strength, compared to 26 healthy controls [Parush et al., 1998]. Thus, the clinician must be wary of making the diagnosis of benign congenital hypotonia because of the large differential diagnosis, which includes infantile spinal muscular atrophy and congenital myopathies [Zellweger, 1983; Thompson, 2002]. Follow-up examination to determine if the appendicular tone increases or stays low is critical to deciding which children should have more detailed diagnostic studies [Thompson, 2002]. It is also possible that some children with congenital hypotonia without persistent weakness have variable degrees of ligament laxity [McGrory et al., 1997; Carboni et al., 2002].
Spinal Cord Anomalies Affecting Motor Neurons
Syringomyelia
Syringomyelia is a slowly progressive cavity formation within the spinal cord, medulla oblongata, or both, which is associated with gliosis. When the medulla is involved, the condition is called syringobulbia. Cavity formation usually occurs in the cervical and lumbar cord segments. On cross-section, the destruction occurs in the area of the anterior white commissure, interrupting crossing fibers of the lateral spinothalamic tracts, with resultant bilateral loss of temperature and pain sensation (Figure 88-12). Superficial sensation is normal because fiber tracts associated with this function are located dorsally and do not cross the cord. Dissociation of sensation occurs when there is concurrent preservation of light touch perception and decrease or absence of pain and temperature perception. The anterior horn cells are affected only after the lesion has reached significant proportions.
Chiari I malformations are often accompanied by syringomyelia or hydromyelia [Gillespie et al., 1986; Isu et al., 1990; Isu et al., 1992; Inoue et al., 2003]. The association of Chiari I malformation with syringomyelia is poorly understood [Gillespie et al., 1986; Iqbal et al., 1992]. After surgery for Chiari malformation, syringomyelia may persist [Tubbs et al., 2004]. In one set of monozygotic twins, both had syringomyelia but they were discordant for Chiari I malformation [Murphy et al., 2006]. Other congenital structural abnormalities, including basilar impression, Klippel–Feil syndrome, Dandy–Walker malformation, cervical ribs, and scoliosis, may be associated with syringomyelia [Berke and Magee, 1976; Isu et al., 1990, 1992; Jaeger et al., 1997; Kasliwal et al., 2008]. Trauma may also cause syringomyelia [Reed et al., 2005]. Growth hormone deficiency is also recognized to be associated with syringomyelia [Murphy et al., 2007] and resolution may occur with growth hormone replacement [Gupta et al., 2008].
The earliest clinical presentation of syringomyelia may be isolated congenital scoliosis or kyphosis. Magnetic resonance imaging (MRI) studies of these infants indicate that 20–30 percent have associated spinal cord abnormalities, including syringomyelia, diastematomyelia, and tethered cord [Suh et al., 2001; Dobbs et al., 2002].
Incomplete closure of the neural tube during the fourth week of gestation is the likely cause of congenital syringomyelia. The normal pattern of cell differentiation causes an inner clustering of spongioblastic cells, which subsequently form glial tissue, and an outer clustering of neuroblastic cells, which subsequently form neurons and their processes. Congenital syringomyelia may result from delayed differentiation of the spongioblastic cells, with ensuing cavitation and gliosis [Leyden, 1876].
Another theory cites a possible congenital structural defect of the hindbrain reminiscent of the one that leads to myelomeningocele and the Chiari deformity. Gardner reported many instances in which the foramen of Magendie was sealed by a membrane [Gardner, 1960]. Clear fluid appeared to accumulate between the syrinx and the fourth ventricle. Failure of the foramen to form an adequate passageway for the fluid during gestation may cause distention of the syrinx and fourth ventricle. Although these findings occasionally may reflect hydromyelia, it is more likely that the pathophysiologic defect is an abnormal intramedullary vascular pattern. The vessels appear to be susceptible to occlusion; thus, infarction or hemorrhage is likely to occur and cause cavitation [Netsky, 1957]. This vascular compromise may come as a result of intermittent compression from increased pressure [Levine, 2004; Brodbelt et al., 2003a].
Another explanation for syringomyelia evolved from a questionnaire designed to determine whether a high number of mothers of children with syringomyelia had experienced difficult labor [Williams, 1977]. An unexpectedly high number of patients had undergone forceps delivery. The number of instances in which the patient with syringomyelia was the firstborn was also significantly increased. It was suggested that, as a result of birth injury, the tonsils descend through the foramen magnum and cause arachnoiditis. This mechanism may only be relevant for infants who already have a Chiari malformation [Newman et al., 1981]. The difference in fluid pressure between cranial and spinal compartments may lead to on-going insidious descent of the tonsils, resulting in communicating syringomyelia.
Recent animal models to study syringomyelia have focused on post-traumatic models. These models have very high syrinx formation when an excitotoxic agent is added to the traumatic injury. In the rat model, fluid enters the syrinx from the subarachnoid space via perivascular spaces. There is more flow in the perivascular spaces at the levels of the syrinx [Yang et al., 2001; Brodbelt et al., 2003a, b, c].
Patients with syringomyelia have limited abilities to monitor pain and temperature. Trophic ulcers of the fingers are often present. Weakness of the limbs is often profound because the cervical and lumbosacral enlargements bear the brunt of the cavitation and gliosis. Muscle atrophy is often first appreciated in the distal small hand muscles. Deep tendon reflexes are either difficult to elicit or absent. Fasciculations are visible in muscles of the shoulder, elbow, and hip. Cervical syringomyelia has been accompanied by papilledema. Elevated intracranial pressure may be present and results from hydrocephalus, mass lesions, or obstruction of the foramina of Luschka and Magendie [Alpers and Comroe, 1931]. Rarely, syringomyelia may be acquired from tumors, or infections of the spinal cord. These acquired types are unusual in infants and young children. Trauma may be a more common cause of acquired syringomyelia in older children and adults [Schurch et al., 1996; Silver, 2001].
Intramedullary cord tumors may cause signs and symptoms similar to those of syringomyelia and prove confounding [Williams, 1977; Gillespie et al., 1986; Madsen et al., 1994]. Differentiation between syringomyelia and intramedullary tumors is possible with modern imaging techniques.
Spinal fluid dynamics and constituents are usually normal in syringomyelia, although the fluid may be xanthochromic and flow may be obstructed. The Valsalva maneuver, which accompanies coughing, straining, and postural changes, may increase pressure and grossly exaggerate findings associated with cysts in the cord. The transient increased pressure may enhance the formation of syringomyelia and syringobulbia [Bertrand, 1973; Levine, 2004].
MRI appears to be the most sensitive method in the detection of syringomyelia [Gillespie et al., 1986; Iqbal et al., 1992]. Management of syringomyelia accompanied by Chiari I malformation consists of posterior fossa decompression, which is beneficial in 50–75 percent of such patients [Vaquero et al., 1990; Attenello et al., 2008]. Motor symptoms may improve more than sensory symptoms, and clinical improvement precedes radiographic improvement [Attenello et al., 2008]. Iskandar et al. demonstrated normalization of cerebrospinal fluid flow postoperatively in children with Chiari I malformation, with or without associated syringomyelia [Iskandar et al., 2004]. Children with syringobulbia also benefit significantly from posterior fossa decompression [Greenlee et al., 2005].
Hydromyelia
Hydromyelia is a congenital deformity resulting from distention of the central canal, which in turn compresses the surrounding cord structures, including the anterior horn cells. The clinical findings are similar to those in syringomyelia. Hydromyelia or syringomyelia is found in more than 50 percent of patients with Chiari I malformations [Menezes, 1991]. Hydromyelia is found most frequently in the cervical and lumbar cord enlargements. The pathologic distention may involve the cord throughout its entire length. The hydromyelic cavity is lined with ependymal cells, in contrast to the glial cell lining of the syrinx [Levine, 2004].
While the cause of hydromyelia is unknown, its association with Chiari I malformation is well established [Wisoff, 1988]. The lack of patency in the foraminae of the fourth ventricle may underlie the distention of the central canal [Gardner, 1965; Lassman et al., 1968]. However, cerebrospinal fluid protein content differs from that of the hydromyelic segment and the measured pressure in the syrinx is higher than that of cerebrospinal fluid at rest. As with syringomyelia, erect position, cough, or strain may transiently elevate the cerebrospinal fluid pressure, causing an increase in fluid in the syrinx [Levine, 2004]. Hydromyelia develops in about one-quarter of patients with post-traumatic cord injuries, and is more likely to develop if there is more than 14 degrees of kyphosis or more than 25 percent stenosis [Abel et al., 1999]. Hydromyelia may also develop or increase postoperatively in patients undergoing surgery for resection of dorsal or terminal lipomas [Scatliff et al., 2005]. Current therapeutic approaches in patients with Chiari I malformations are similar to those used in patients with syringomyelia, and include posterior fossa decompression, with or without duraplasty [Munshi et al., 2000].
Diastematomyelia
Diastematomyelia is an abnormal division of the spinal cord, usually almost equally, by a septum of variable caudal–rostral length (Figure 88-13). The mesodermal septum is composed of fibrous tissue, bone, cartilage, or a mixture of these tissues. The septum traverses the neural canal, thus splitting the spinal cord, and is usually anchored posteriorly on the dura or bony neural arch [Moes and Hendrick, 1963]. The neural tube forms a closed cavity when normal maturation occurs during the first fetal month. The tube becomes distended, the dorsal surface (roof) becomes permeable, and fluid flows into the adjacent space; this space develops into the subarachnoid space. The point of failure of the developmental process that results in diastematomyelia is still not completely understood.
Surgical manipulation of the chick embryo documents that it is possible to create a chick with diastematomyelia by intraspinal grafting of a somite. Transplanted quail cells may form a septum between the dorsal surface (roof) and floor, dividing the neural tube into two parts. However, in some chicks, the same experiment leads to the chick host eliminating quail cells and, in some, spina bifida is produced. This suggests that spontaneous diastematomyelia may be the consequence of abnormal gastrulation, the process by which the two early germ layers convert to three distinctive germ layers [Klessinger and Christ, 1996].
When diastematomyelia is present, the cartilaginous or bony septum may compromise the anterior horn cell column and cause muscle atrophy, decreased or absent deep tendon reflexes, and moderate to severe weakness of the distal muscles of the legs and feet. This weakness is often the patient’s first complaint. Involvement is usually bilateral. Deformities of the feet, particularly talipes equinovarus, commonly occur. Atonic bladder, with urinary retention or incontinence, may occur. Cutaneous lesions, including hairy patch, dimple, hemangioma, teratoma, or subcutaneous mass, are present in more than half of patients [James and Lassman, 1964; Miller et al., 1993]. Congenital scoliosis is present in 79 percent, and musculoskeletal anomalies, including spinal dysraphism, clubfoot, or cavus foot, are present in 98 percent [Miller et al., 1993].
Radiography, computed tomography (CT), or MRI usually demonstrates the septum; the deposition of calcium, which may be delayed for years, facilitates identification [Thron and Schroth, 1986; Bruhl et al., 1990; Harwood-Nash and McHugh, 1990]. A large retrospective study of 17 children reported that the mean age at diagnosis was 3.4 years, with a range of 5 days to 12 years [Gan et al., 2007]. Two distinct types are now recognized [Leung and Buxton, 2005]. Type I is most common and consists of two hemicords, each with its own dural sac separated by the cartilaginous or boney spur. In type II, the two hemicords are within a single dural tube [Leung and Buxton, 2005] (Figure 88-14). Increasingly, diastematomyelia is diagnosed prenatally, with ultrasound between 13 and 23 weeks demonstrating widening of the spinal canal and the echogenic midline spur [Has et al., 2007]. Prenatal diagnosis with ultrasound and MRI (Figure 88-15) may allow earlier intervention [Allen and Silverman, 2000; Sonigo-Cohen et al., 2003; Has et al., 2007]. The septum is usually located in the lower thoracic and lumbar areas, but may be present as high as the cervical vertebra [Cowie, 1951; Balci et al., 2002]. One patient with involvement of the basicranium has been described [Pfeifer, 1991]. Spina bifida occulta or tethered cord may be associated [Guthkelch and Hoffmann, 1981]. Kyphoscoliosis or scoliosis may accompany the other characteristic findings. Intersegmental laminar fusion occurring with spina bifida, at or adjacent to the level of fusion, should suggest the diagnosis of diastematomyelia [Hilal et al., 1974]. Diastematomyelia has been associated with epidermoid cysts and syrinx [Sheehan et al., 2002] or teratoma [Hader et al., 1999]. As these may not occur at the same level, imaging of all levels is indicated.
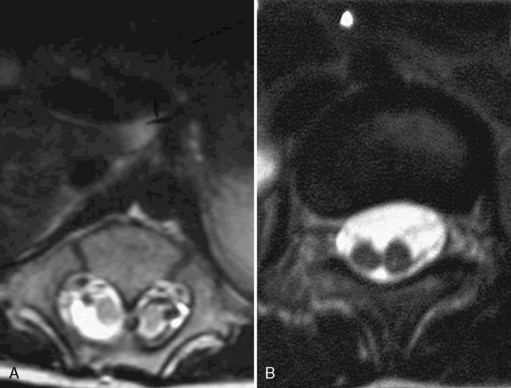
Fig. 88-14 Two forms of diastematomyelia are depicted.
A, In type I, two hemicords, each with its own dual tube, are separated by a rigid, dural, sheathed, osseocartilaginous septum. B, In type II, the two hemicords are within a single dural tube [Leung and Buxton, 2005].
Clinical and radiographic correlation suggest three subsets of patients with diastematomyelia: those with associated tethered cord, those with midline cutaneous stigmata, and those with meningocele or meningomyelocele [Schijman, 2003]. The natural history without surgical intervention is not clear but does depend on these associated findings. In the largest reported series by Miller et al., it is clear that some children have a nonprogressive course [Miller et al., 1993]. Has et al. reported their experience and review of all published children diagnosed prenatally. Those children with no additional sonographic findings and normal α-fetoprotein levels were asymptomatic at birth [Has et al., 2007]. Symptoms, particularly pain, may be prevented or significantly ameliorated by surgery [Meacham, 1967; Kim et al., 1994; Schijman, 2003]. The goals of surgery are to prevent progressive deformity and neurological deficit. However, neurologic symptoms may persist [Kim et al., 1994] and, in some cases, worsen [Goldberg et al., 1984]. Excision of the mesodermal septum, including all portions attached to the anterior dural surface, and subsequent laminectomy are indicated in those with progression of neurological symptoms [Miller et al., 1993; Gan et al., 2007]. While there is some evidence that prophylactic surgery may prevent deficits [Gan et al., 2007], there are no randomized trials in this population.
Infection and Autoimmune Diseases Affecting Motor Neurons of the Spinal Cord
Poliomyelitis
Poliomyelitis is a viral disease of motor neurons caused by polio virus. Nonparalytic poliomyelitis is the most common form of the disease. From a clinical standpoint, it is largely indistinguishable from other meningoencephalitides. About 25 percent of patients reported in one series had nonparalytic poliomyelitis, 50 percent had spinal poliomyelitis, about 12 percent had bulbar poliomyelitis, and 10 percent had bulbospinal involvement [Auld et al., 1960; Lepow and Spence, 1965].
Onset is indicated by signs of malaise, which are usually accompanied by muscular pains or stiffness. Headaches occur frequently, and symptoms of upper respiratory tract infection are common in children. The patient may experience some degree of nuchal rigidity and a low-grade fever as the disease progresses. Muscular tightness then becomes noticeable, primarily in the hamstring, thigh, and neck. Poliomyelitis characterized by a triad of fever, nuchal rigidity, and spasm of the back muscles may affect infants who are younger than 1 year of age [Abramson and Greenberg, 1955]. Spinal involvement is more common than bulbar involvement in infants. The course of the disease may range from slowly progressive to fulminating; the more rapid the progression, the more severe the eventual involvement.
The anterior horn cells are the prime target of the virus, and motor weakness is the hallmark of the clinical pattern. In most cases, the weakness is asymmetric. The incidence of quadriplegia and trunk muscle compromise is greater after the first year of life. Bulbar involvement is life-threatening, resulting in embarrassment of the respiratory, autonomic, and circulatory centers of the brainstem and in profound weakness of the muscles of respiration and swallowing [Baker et al., 1950; Auld et al., 1960]. Poliomyelitis has occurred in utero on numerous occasions and can result in death during the neonatal period [Pugh and Dudgeon, 1954]. Evidence of pleocytosis with mononuclear cells and a normal or slightly elevated protein concentration can usually be obtained through spinal fluid examination. Polymorphonuclear cells may become prevalent early in the course of the disease. The spinal fluid glucose content is usually normal. Direct analysis of cerebrospinal fluid for virus DNA makes diagnosis rapid and accurate. Treatment is supportive. Mechanical ventilation and support of circulation may be required in the presence of bulbar symptoms. Prolonged rehabilitation is generally the rule after patients have recovered from the acute stage of polio, and permanent motor deficits are common. Postpolio syndrome may begin two or more decades after the illness, and is associated with progressive loss of strength and atrophy of an already affected limb. Its cause is unknown [Munsat, 1991].
West Nile Poliomyelitis
While poliovirus immunization has led to marked reduction of this form of anterior horn cell disease, it is clear that many other viruses, including Epstein–Barr [Wong et al., 1999], enterovirus 71 [Hayward et al., 1989], echo 4 virus [Kopel et al., 1965], and coxsackie [Grist, 1962; Jarcho et al., 1963], are capable of causing a “polio-like syndrome.” Most recently, West Nile virus (WNV) has also been demonstrated to cause flaccid paralysis that localizes by examination, imaging, and electrophysiologically to the anterior horn cell [Solomon and Willison, 2003].
WNV is a single-strand RNA flavivirus that is transmitted between Culex mosquitoes and birds, with incidental infections developing in humans. It is endemic to Africa, the Middle East, and Southwest Asia. An epidemic in New York City in 1999 has led to the virus spreading across North America [Asnis et al., 2000, 2001]. There is a 3- to 15-day incubation period, and infection through blood products been documented [Pealer et al., 2003]. Most infections are asymptomatic, with 20 percent of patients developing a mild febrile illness.
Symptomatic infections are more common in adults over 50 but have been described in children. Systemic features include fever, nausea, vomiting, and headache, and a meningoencephalitis is present in 63 percent of cases. Cerebrospinal fluid studies indicate elevated protein, normal glucose, and pleocytosis [Petersen and Marfin, 2002]. Children with WNV infection have less severe illnesses [Hayes and O’Leary, 2004].
Neurologic involvement develops in between 1 in 140 and 1 in 320 infections [Horga and Fine, 2001]. Weakness over days may be associated with encephalopathy. The weakness may involve the face and may be asymmetric, involving a single limb. Sensory examination is normal, but electrophysiologic studies demonstrate normal conduction velocities but small compound muscle action potentials and asymmetrical denervation changes [Leis et al., 2002; Flaherty et al., 2003; Al-Shekhlee and Katirji, 2004]. MRI changes are not uniformly present but may include cauda equina enhancement, spinal cord parenchymal signal abnormalities, and parenchymal or leptomeningeal signal changes in the brain [Jeha et al., 2003; Heresi et al., 2004]. While most patients who have been well studied electrophysiologically have localization to the anterior horn cells, a demyelinating, Guillain–Barré-like picture has also been described [Ahmed et al., 2000].
While neuroinvasive disease was believed to be less common in children, encephalitis and aseptic meningitis developed in two hospitalized children in the original outbreak [Horga and Fine, 2001], while 3 in a series of 11 children in the breakout in Los Angeles County had meningitis (2) or encephalitis (1) [Civen et al., 2006]. Polio-like illness was first described by Heresi et al. [Heresi et al., 2004]. The most extensive study of children reported to the Centers for Disease Control and Prevention from 1999 to 2007 reported that, of the 1875 children reported, 68 percent were classified as having West Nile fever and 30 percent (443) as having West Nile neuroinvasive disease [Lindsey et al., 2009]. Of these 443 children with West Nile neuroinvasive disease, 47 percent had meningitis, 37 percent with encephalitis, and 1 percent developed acute flaccid paralysis without concurrent encephalitis or meningitis [Lindsey et al., 2009]. Three fatal cases were reported [Lindsey et al., 2009]. The most detailed study of a child with polio secondary to WNV confirmed localization to the anterior horn cell both by MRI and electrophysiology [Hainline et al., 2008].
Pathologic features include perivascular and leptomeningeal inflammation, microglial nodules, and neuronophagia, involving temporal lobes and brainstem in patients with meningoencephalitis [Kelley et al., 2003]. In patients with polio-like illnesses, similar findings are present in spinal cord [Kelley et al., 2003; Sampson et al., 2003].
Diagnosis is confirmed by demonstrating rising immunoglobulin M titer to the virus. While this test is sensitive, antibodies to WNV may cross-react with dengue or St. Louis encephalitis, and so positive results should be confirmed using reverse transcriptase polymerase chain reaction [Horga and Fine, 2001].
Transverse Myelitis
The thoracic area of the cord is most often affected. The illness begins acutely and progresses quickly for 1–2 days. Early manifestations of spinal cord dysfunction may follow or be accompanied by fever, lethargy, malaise, and myalgia. Paresthesias of the legs are common. Back pain at the segmental level of the involved cord frequently occurs (Figure 88-16). Anterior horn cell disruption in the cervical or lumbar enlargements causes symptoms and signs of lower motor unit dysfunction in the arms and legs. The permanency of the weakness is not predictable from its characteristics. The sequence of involvement most often exhibited is weakness and even flaccidity of the legs, with subsequent loss of rectal and bladder sphincter control. Sensory impairment usually extends to the segmental level of motor impairment. The transitional zone between the involved and uninvolved areas may be the site of dysesthesias and hyperalgesia. Deprivation of autonomic innervation below the cord lesion causes loss of sweating.
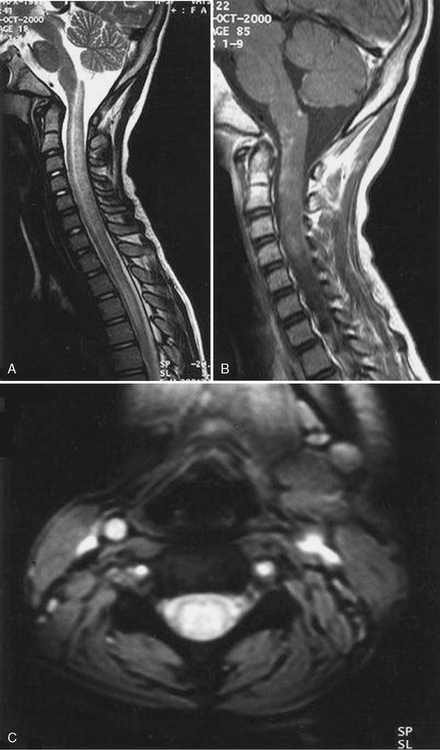
Fig. 88-16 Magnetic resonance imaging findings in a child with transverse myelitis.
(From Andronikou S, et al: MRI findings in acute idiopathic transverse myelopathy in children, Pediatr Radiol 33:624, 2003.)
Recovery may be evident within a week of onset; more commonly, improvement may not occur for several weeks or months, if it occurs at all. Unfortunately, flaccidity in limbs innervated caudal to the spinal segment interruption may slowly transform into spasticity after several weeks. Consonant with the appearance of spasticity, hyperreflexia, associated with ankle clonus and extensor toe signs, may occur. In a large retrospective single-site study of 47 children, 43 percent were still unable to walk at a median of 3.2 years after the acute illness, 54 percent had persistent dysesthesias, and 68 percent required bladder catheterization [Pidcock et al., 2007]. In this series, age at onset of less than 3 years predicted worse functional outcomes [Pidcock et al., 2007]. Aggressive corticosteroid therapy may be beneficial.
Examination of spinal fluid usually reveals pleocytosis with a high percentage of lymphocytes. Protein content is usually normal, but a slight increase may occur when the rootlets or peripheral nerves are compromised. Spinal fluid glucose concentration is usually normal. Electromyographic examination may be normal or may demonstrate anterior horn cell dysfunction in the involved cord segments. MRI may depict the degree and extent of the lesion [Awerbuch et al., 1987; Andronikou et al., 2003].
Numerous viral and other infections, as well as other agents and processes, including immune-mediated phenomena that are associated with inflammation, have been implicated in transverse myelitis and accompanying anterior horn cell involvement. They include acquired immune deficiency syndrome [Barakos et al., 1990], infectious mononucleosis [Grose and Feorino, 1973; Grose et al., 1975; Junker et al., 1991; Tsutsumi et al., 1994; Clevenbergh et al., 1997], mumps [Benady et al., 1973; Nussinovitch et al., 1992; Venketasubramanian, 1997; Caksen and Ustunbas, 2003], Mycoplasma pneumoniae infection [Francis et al., 1988; Parisi and Filice, 2001; Candler and Dale, 2004], rabies vaccination [Harrington and Olin, 1971; Label and Batts, 1982], smallpox vaccination [Shyamalan et al., 1964], rubella, rubeola, smallpox [Booss and Davis, 2003], hepatitis E [Mandal and Chopra, 2006], and varicella.
Vascular Diseases of the Spinal Cord
Anterior Spinal Artery Occlusion
The anterior spinal artery originates where the vertebral arteries meet and traverses the anterior median sulcus over the entire course of the spinal cord. The radicular arteries are divided into anterior and posterior radicular arteries. Blood from the anterior radicular arteries that enter with the nerve roots between the third cervical and third lumbar segments flows into the anterior spinal artery (Figure 88-17). The anterior spinal artery then is separated further into several sulcal branches that pierce the central gray matter, perfuse the anterior horn cells, and form numerous small penetrating vessels that supply the white matter of the anterior and lateral columns. As the penetrating branches of the anterior spinal artery are end arteries, their occlusion results in infarction of the anterior half of the spinal cord. The anatomic relationship between the two posterior spinal arteries provides a very different vascular pattern for the posterior cord than the anterior spinal arteries do for the anterior cord. The posterior circulation enjoys a more extensive collateral system. Therefore, occlusion of the posterior spinal arteries usually does not cause severe clinical dysfunction.
In 1952, Steegman reported the clinical effects of obstruction of anterior spinal artery circulation [Steegman, 1952]. Occlusion of the anterior spinal artery often results from arteriosclerotic vascular disease in adults, a condition uncommon in children. However, certain childhood pathologic conditions may lead to similar obstruction of blood flow. Trauma and infectious conditions are among the most common causes of anterior spinal artery insufficiency in children [Goebel et al., 1977; Blennow and Starck, 1987]. Other causes include embolism from cardiac disease and thrombosis from hypercoagulable states [Oller-Navarro et al., 1979]. Compromise of blood circulation in rhesus monkeys occurring inferior to the first lumbar vertebra causes the development of a lesion that expands from the central gray matter toward the periphery [Fried and Aparicio, 1973]. The most devastated areas of infarction are found in the third and sixth lumbar segments. The region superior to the experimental ligation is relatively unaffected. The central gray matter is entirely infarcted. In the lesions that are associated with the greatest degree of destruction, only a thin rim of the peripheral white matter is preserved. In lesions that are less extensive, the neighboring circumferential white matter is disrupted. This occasional survival of the peripheral white matter and the lateral area of the anterior horn may result from perfusion from pial plexus vessels and vasa corona, both receiving blood supplies from the posterior spinal arteries. As much as 50 percent of the outer rim of white matter receives its circulation from these sources [Fried and Aparicio, 1973].
Clinical findings associated with anterior spinal artery occlusion depend on the level at which the artery is occluded [Steegman, 1952]. Obstruction of the anterior spinal artery near its inception from the two vertebral arteries initiates brainstem compromise. Clinical findings may be unilateral but are usually bilateral. Loss of pain and temperature sensation and spastic quadriplegia occur, and are commonly associated with some loss of superficial sensation inferior to the involved segmental level. Ischemia or infarction of the cervical spinal cord causes flaccid paralysis of the arms because of disruption of the anterior horn cells in the cervical enlargement. Weakness, muscle atrophy, and absence of deep tendon reflexes in the legs follow occlusion of the anterior spinal artery in the region of the lumbar enlargement. Temperature and pain perception are often affected in the legs.
Tumors and congenital vascular malformations are among the conditions that must be considered in the differential diagnosis of anterior spinal artery interruption in children. Vascular syndromes of the spinal cord may be seen in association with coarctation of the aorta [Owens and Swan, 1963; Darwish et al., 1979; Servais et al., 2001]. During corrective surgery, blood flow must be maintained from the proximal to the distal portions of the aorta. Surgical anastomosis requires removal of the stenotic section of the aorta and establishment of an adequate anastomosis. One and occasionally two radicular arteries in the thoracic region originate in the aorta; they, in turn, provide blood flow for the anterior spinal artery through radicular branches. If the radicular artery is cut or clamped for a significant period during surgery, clinical findings of anterior spinal artery compromise may develop [Weenink and Smilde, 1964].
Sensory impairment may initially be severe after ischemia induced during surgery but tends to relent in the first few postoperative weeks; unfortunately, the motor deficits often remain. The deficits appear to mirror anterior horn cell dysfunction. This pattern may reflect the fact that the anterior horn cells are in the region of greatest oxidative metabolic dependency in the spinal cord [Dodson and Landau, 1973]. The anterior spinal artery syndrome has been reported with coarctation of the aorta in children who have not had surgical correction [Weenink and Smilde, 1964].
Spinal Cord Vascular Anomalies
While arteriovenous malformations of the spinal cord are rare, symptoms during the first two decades of life develop in 20 percent of patients [Rodesch et al., 2004]. Of the 30 children in one large series, 21 presented with hemorrhage and 5 had hereditary hemorrhagic telangiectasia [Rodesch et al., 2004]. Infarction is accompanied by pain in the back or abdomen; the loss of strength and sensation in the legs may develop gradually or suddenly. The lower extremities become flaccid over the next 12–24 hours, and bladder control ceases [Buchanan, 2004]. During the next few weeks, the leg flaccidity gradually relents, only to be replaced by spasticity. Priapism, which may be intractable, may occur. Imaging studies and surgical exploration demonstrate a large mass of pulsating vessels in the spinal canal.
Despite anterior horn cell compromise, concurrent impairment of the corticospinal and spinothalamic tracts results in a clinical picture primarily of loss of temperature and pain perception, and of upper motor unit dysfunction manifested by acute flaccidity that evolves into spasticity after several weeks. Lumbar puncture reveals normal cerebrospinal fluid pressure. The cerebrospinal fluid may be xanthochromic. Normal cerebrospinal fluid flow is often obstructed, with resultant marked elevation of protein content. Myelography may not depict the extent of the lesion faithfully. Angiography or MR angiogram may be necessary for adequate visualization of the arteriovenous malformation [Tobin and Layton, 1976; Pattany et al., 2003]. Based on angiography, three types of lesions have been described: single coiled vessel, glomus, and juvenile. CT and MRI may be most useful in delineating the vascular lesions. The relative benefit of surgery is determined by such factors as residual damage, number of feeding vessels, and extent and pattern of the abnormal vessels within the mass. Less invasive endosaccular coil therapy may have promise in these rare malformations [Lavoie et al., 2007].
Trauma-Related Anterior Horn Cell Disease
Incomplete or total transection of the spinal cord may follow birth trauma, falls, motor vehicle accidents, or blows to the spinal column. Neurologic dysfunction, reflected in diverse functional impairment, may result. Fractures or dislocations of the vertebrae are often present. The pattern of clinical involvement is determined by the areas of anatomic disruption. Therefore, signs and symptoms of corticospinal tract, posterior column, and lateral spinothalamic tract interruption may be present. Limb weakness occurs consonant with the segmental level of injury. Losses of deep and superficial sensations below that level are present. Compromise of bladder function is common. Findings characteristic of anterior horn cell dysfunction are evident in the limbs as a sequel to spinal cord trauma in the cervical or lumbosacral enlargements. Neurologic impairment resulting from trauma localized to the anterior horn cell column is unlikely, unless the anterior spinal artery is the primary site of injury. Trauma accompanying delivery, particularly in a breech presentation, usually affects the middle and lower cervical segments of the spinal cord [Abroms et al., 1973]. Radiographic documentation of dislocation or fracture of the spinal column is rare. Although flaccidity is present at birth, spasticity of the legs may gradually develop. The distribution of weakness in the arms and hands is variable. The pattern may be such that bilateral Erb’s paralysis is erroneously diagnosed. The combination of compromise of the small muscles of the hands and paresis of the legs should suggest spinal cord involvement rather than brachial plexus injury. Development of pectus excavatum in these patients results from unopposed diaphragmatic respiration by the intercostal muscles. The abdominal muscles are frequently weak, and protrusion of the abdomen occurs. Impairment of bladder function, with resultant urinary retention followed by infection, may become a major problem. Depending on the level of involvement, Horner’s syndrome may be present. Decreased or absent perspiration below the segmental levels innervated by the upper thoracic nerves may alter temperature homeostasis, resulting in hyperthermia that may prove life-threatening if the ambient temperature is high. Provision of an air-conditioned environment may be obligatory for these infants.
Many reports corroborate the relatively high risk of middle or lower cervical cord trauma during breech delivery when there is hyperextension of the head. In one report, complete transection of the cervical cord occurred in 21 percent of the 88 patients reviewed. None of the infants delivered by cesarean section sustained spinal cord injury [Abroms et al., 1973]. Unfortunately, no specific treatment exists, although physical and occupational therapy may be helpful.
References
The complete list of references for this chapter is available online at www.expertconsult.com.
Abel R., Gerner H.J., et al. Residual deformity of the spinal canal in patients with traumatic paraplegia and secondary changes of the spinal cord. Spinal Cord. 1999;37(1):14-19.
Abramson H., Greenberg M. Acute poliomyelitis in infants under one year of age: epidemiological and clinical features. Pediatrics. 1955;16(4):478-488.
Abroms I.F., Bresnan M.J., et al. Cervical cord injuries secondary to hyperextension of the head in breech presentations. Obstet Gynecol. 1973;41(3):369-378.
Adams C., Becker L.E., et al. Neurogenic arthrogryposis multiplex congenita: clinical and muscle biopsy findings. Pediatr Neurosci. 1988;14(2):97-102.
Ahmed S., Libman R., et al. Guillain-Barre syndrome: An unusual presentation of West Nile virus infection. Neurology. 2000;55(1):144-146.
Al-Chalabi A., Andersen P.M., et al. Deletions of the heavy neurofilament subunit tail in amyotrophic lateral sclerosis. Hum Mol Genet. 1999;8(2):157-164.
Alexander M.P., Emery E.S.3rd, et al. Progressive bulbar paresis in childhood. Arch Neurol. 1976;33(1):66-68.
Allen L.M., Silverman R.K. Prenatal ultrasound evaluation of fetal diastematomyelia: two cases of type I split cord malformation. Ultrasound Obstet Gynecol. 2000;15(1):78-82.
Alpers B., Comroe B. Syringomyelia with choked disk. J Nerv Ment Dis. 1931;73:577.
Al-Shekhlee A., Katirji B. Electrodiagnostic features of acute paralytic poliomyelitis associated with West Nile virus infection. Muscle Nerve. 2004;29(3):376-380.
Andronikou S., Albuquerque-Jonathan G., et al. MRI findings in acute idiopathic transverse myelopathy in children. Pediatr Radiol. 2003;33(9):624-629.
Asnis D.S., Conetta R., et al. The West Nile virus encephalitis outbreak in the United States (1999–2000): from Flushing, New York, to beyond its borders. Ann N Y Acad Sci. 2001;951:161-171.
Asnis D.S., Conetta R., et al. The West Nile Virus outbreak of 1999 in New York: the Flushing Hospital experience. Clin Infect Dis. 2000;30(3):413-418.
Atsuta N., Watanabe H., et al. Natural history of spinal and bulbar muscular atrophy (SBMA): a study of 223 Japanese patients. Brain. 2006;129(Pt 6):1446-1455.
Attenello F.J., McGirt M.J., et al. Outcome of Chiari-associated syringomyelia after hindbrain decompression in children: analysis of 49 consecutive cases. Neurosurgery. 2008;62(6):1307-1313. discussion 1313
Auld P.A., Kevy S.V., et al. Poliomyelitis in children. Experience with 956 cases in the 1955 Massachusetts epidemic. N Engl J Med. 1960;263:1093-1100.
Awerbuch G., Feinberg W.M., et al. Demonstration of acute post-viral myelitis with magnetic resonance imaging. Pediatr Neurol. 1987;3(6):367-369.
Baines D.B., Douglas I.D., et al. Anaesthesia for patients with arthrogryposis multiplex congenita: what is the risk of malignant hyperthermia? Anaesth Intensive Care. 1986;14(4):370-372.
Baker A.B., Matzke H.A., et al. Bulbar poliomyelitis; a study of medullary function. Arch Neurol Psychiatry. 1950;63(2):257-281.
Balci S., Oguz K.K., et al. Cervical diastematomyelia in cervico-oculo-acoustic (Wildervanck) syndrome: MRI findings. Clin Dysmorphol. 2002;11(2):125-128.
Banker B.Q. Arthrogryposis multiplex congenita: spectrum of pathologic changes. Hum Pathol. 1986;17(7):656-672.
Banker B.Q.. Neuropathologic aspects of arthrogryposis multiplex congenita. Clin Orthop Relat Res. 1985(194):30-43.
Banker B.Q. The congenital muscular dystrophies. In: Engel AGaCF-A, editor. Myology. McGraw-Hill, Inc.; 1994:1275-1289.
Barakos J.A., Mark A.S., et al. MR imaging of acute transverse myelitis and AIDS myelopathy. J Comput Assist Tomogr. 1990;14(1):45-50.
Barth P.G. Pontocerebellar hypoplasias. An overview of a group of inherited neurodegenerative disorders with fetal onset. Brain and Development. 1993;15(6):411-422.
Battaglia G., Princivalle A., et al. Expression of the SMN gene, the spinal muscular atrophy determining gene, in the mammalian central nervous system. Hum Mol Genet. 1997;6(11):1961-1971.
Baumer D., Hilton D., et al. Juvenile ALS with basophilic inclusions is a FUS proteinopathy with FUS mutations. Neurology. 2010;Vol 75(7):611-618.
Beckerman R.C., Buchino J.J. Arthrogryposis multiplex congenita as part of an inherited symptom complex: two case reports and a review of the literature. Pediatrics. 1978;61(3):417-422.
Belsham D.D., Yee W.C., et al. Analysis of the CAG repeat region of the androgen receptor gene in a kindred with X-linked spinal and bulbar muscular atrophy. J Neurol Sci. 1992;112(1–2):133-138.
Benady S., Zvi A.B., et al. Transverse myelitis following mumps. Acta Paediatr Scand. 1973;62(2):205-206.
Bennett J.B., Hansen P.E., et al. Surgical management of arthrogryposis in the upper extremity. J Pediatr Orthop. 1985;5(3):281-286.
Berke J.P., Magee K.R. Craniofacial dysostosis with syringomyelia and associated anomalies. Arch Neurol. 1976;33(1):63-65.
Bertrand G. Chapter 26. Dynamic factors in the evolution of syringomyelia and syringobulbia. Clin Neurosurg. 1973;20:322-333.
Blair I.P., Bennett C.L., et al. A gene for autosomal dominant juvenile amyotrophic lateral sclerosis (ALS4) localizes to a 500-kb interval on chromosome 9q34. Neurogenetics. 2000;3(1):1-6.
Blennow G., Starck L. Anterior spinal artery syndrome. Report of seven cases in childhood. Pediatr Neurosci. 1987;13(1):32-37.
Boltshauser E., Lang W., et al. Hereditary distal muscular atrophy with vocal cord paralysis and sensorineural hearing loss: a dominant form of spinal muscular atrophy? J Med Genet. 1989;26(2):105-108.
Bonilla-Musoles F., Machado L.E., et al. Multiple congenital contractures (congenital multiple arthrogryposis). J Perinat Med. 2002;30(1):99-104.
Booss J., Davis L.E. Smallpox and smallpox vaccination: neurological implications. Neurology. 2003;60(8):1241-1245.
Borchelt D.R., Wong P.C., et al. Transgenic mouse models of Alzheimer’s disease and amyotrophic lateral sclerosis. Brain Pathol. 1998;8(4):735-757.
Borochowitz Z., Glick B., et al. Infantile spinal muscular atrophy (SMA) and multiple congenital bone fractures in sibs: a lethal new syndrome. J Med Genet. 1991;28(5):345-348.
Brodbelt A.R., Stoodley M.A., et al. Altered subarachnoid space compliance and fluid flow in an animal model of posttraumatic syringomyelia. Spine (Phila Pa 1976). 2003;28(20):E413-E419.
Brodbelt A.R., Stoodley M.A., et al. Fluid flow in an animal model of post-traumatic syringomyelia. Eur Spine J. 2003;12(3):300-306.
Brodbelt A.R., Stoodley M.A., et al. The role of excitotoxic injury in post-traumatic syringomyelia. J Neurotrauma. 2003;20(9):883-893.
Brown C.H. Infantile amyotrophic lateral sclerosis of the family type. J Nerv Ment Dis. 1894;21:707-716.
Brown J., Ashworth A., et al. Familial non-specific dementia maps to chromosome 3. Hum Mol Genet. 1995;4(9):1625-1628.
Bruhl K., Schwarz M., et al. Congenital diastematomyelia in the upper thoracic spine. Diagnostic comparison of CT, CT-myelography, MRI, and US. Neurosurg Rev. 1990;13(1):77-82.
Brzustowicz L.M., Kleyn P.W., et al. Fine-mapping of the spinal muscular atrophy locus to a region flanked by MAP1B and D5S6. Genomics. 1992;13(4):991-998.
Brzustowicz L.M., Lehner T., et al. Genetic mapping of chronic childhood-onset spinal muscular atrophy to chromosome 5q11.2-13.3. Nature. 1990;344(6266):540-541.
Buchanan J.W. Tracheal signs and associated vascular anomalies in dogs with persistent right aortic arch. J Vet Intern Med. 2004;18(4):510-514.
Buhler D., Raker V., et al. Essential role for the tudor domain of SMN in spliceosomal U snRNP assembly: implications for spinal muscular atrophy. Hum Mol Genet. 1999;8(13):2351-2357.
Burglen L., Lefebvre S., et al. Structure and organization of the human survival motor neurone (SMN) gene. Genomics. 1996;32(3):479-482.
Caksen H., Ustunbas H.B. A fatal case of acute transverse myelitis associated with mumps. J Emerg Med. 2003;24(3):341-342.
Candler P.M., Dale R.C. Three cases of central nervous system complications associated with Mycoplasma pneumoniae. Pediatr Neurol. 2004;31(2):133-138.
Carboni P., Pisani F., et al. Congenital hypotonia with favorable outcome. Pediatr Neurol. 2002;26(5):383-386.
Carvalho T., Almeida F., et al. The spinal muscular atrophy disease gene product, SMN: A link between snRNP biogenesis and the Cajal (coiled) body. J Cell Biol. 1999;147(4):715-728.
Chance P.F., Rabin B.A., et al. Linkage of the gene for an autosomal dominant form of juvenile amyotrophic lateral sclerosis to chromosome 9q34. Am J Hum Genet. 1998;62(3):633-640.
Chang H.C., Hung W.C., et al. Degradation of survival motor neuron (SMN) protein is mediated via the ubiquitin/proteasome pathway. Neurochem Int. 2004;45(7):1107-1112.
Charnas L., Trapp B., et al. Congenital absence of peripheral myelin: abnormal Schwann cell development causes lethal arthrogryposis multiplex congenita. Neurology. 1988;38(6):966-974.
Chen Y.Z., Bennett C.L., et al. DNA/RNA helicase gene mutations in a form of juvenile amyotrophic lateral sclerosis (ALS4). Am J Hum Genet. 2004;74(6):1128-1135.
Choi W.T., MacLean H.E., et al. Kennedy’s disease: genetic diagnosis of an inherited form of motor neuron disease. Aust N Z J Med. 1993;23(2):187-192.
Chou S.M. Controversy over Werdnig-Hoffmann disease and multiple system atrophy. Curr Opin Neurol. 1993;6(6):861-864.
Chou S.M., Gilbert E.F., et al. Infantile olivopontocerebellar atrophy with spinal muscular atrophy (infantile OPCA + SMA). Clin Neuropathol. 1990;9(1):21-32.
Christodoulou K., Kyriakides T., et al. Mapping of a distal form of spinal muscular atrophy with upper limb predominance to chromosome 7p. Hum Mol Genet. 1995;4(9):1629-1632.
Christodoulou K., Zamba E., et al. A novel form of distal hereditary motor neuronopathy maps to chromosome 9p21.1-p12. Ann Neurol. 2000;48(6):877-884.
Civen R., Villacorte F., et al. West Nile virus infection in the pediatric population. Pediatr Infect Dis J. 2006;25(1):75-78.
Clermont O., Burlet P., et al. Use of genetic and physical mapping to locate the spinal muscular atrophy locus between two new highly polymorphic DNA markers. Am J Hum Genet. 1994;54(4):687-694.
Clevenbergh P., Brohee P., et al. Infectious mononucleosis complicated by transverse myelitis: detection of the viral genome by polymerase chain reaction in the cerebrospinal fluid. J Neurol. 1997;244(9):592-594.
Coovert D.D., Le T.T., et al. The survival motor neuron protein in spinal muscular atrophy. Hum Mol Genet. 1997;6(8):1205-1214.
Courtens W., Johansson A.B., et al. Infantile spinal muscular atrophy variant with congenital fractures in a female neonate: evidence for autosomal recessive inheritance. J Med Genet. 2002;39(1):74-77.
Cowie T.N. Diastematomyelia with vertebral column defects; observations on its radiological diagnosis. Br J Radiol. 1951;24(279):156-160.
Coyle J.T., Puttfarcken P. Oxidative stress, glutamate, and neurodegenerative disorders. Science. 1993;262(5134):689-695.
Daher Y.H., Lonstein J.E., et al. Spinal surgery in spinal muscular atrophy. J Pediatr Orthop. 1985;5(4):391-395.
Darwish H., Archer C., et al. The anterior spinal artery collateral in coarctation of the aorta. A clinical angiographic correlation. Arch Neurol. 1979;36(4):240-243.
Davis C.J., Bradley W.G., et al. The peroneal muscular atrophy syndrome: clinical, genetic, electrophysiological and nerve biopsy studies. I. Clinical, genetic and electrophysiological findings and classification. J Genet Hum. 1978;26(4):311-349.
DeLong R., Siddique T. A large New England kindred with autosomal dominant neurogenic scapuloperoneal amyotrophy with unique features. Arch Neurol. 1992;49(9):905-908.
de Muelenaere C. Misoprostol and Mobius syndrome. S Afr Med J. 1999;89(1):12. 14
Deng H.X., Hentati A., et al. Amyotrophic lateral sclerosis and structural defects in Cu,Zn superoxide dismutase. Science. 1993;261(5124):1047-1051.
Dobbs M.B., Gurnett C.A., et al. HOXD10 M319K mutation in a family with isolated congenital vertical talus. J Orthop Res. 2006;24(3):448-453.
Dobbs M.B., Lenke L.G., et al. Prevalence of neural axis abnormalities in patients with infantile idiopathic scoliosis. J Bone Joint Surg Am. 2002;84-A(12):2230-2234.
Dodson W.E., Landau W.M. Motor neuron loss due to aortic clamping in repair of coarctation. Neurology. 1973;23(5):539-542.
Drachman D.B., Banker B.Q. Arthrogryposis multiplex congenita. Case due to disease of the anterior horn cells. Arch Neurol. 1961;5:77-93.
Dubowitz V. The floppy infant – a practical approach to classification. Dev Med Child Neurol. 1968;10(6):706-710.
Ellsworth R.E., Ionasescu V., et al. The CMT2D locus: refined genetic position and construction of a bacterial clone-based physical map. Genome Res. 1999;9(6):568-574.
Emery E.S., Fenichel G.M., et al. A spinal muscular atrophy with scapuloperoneal distribution. Arch Neurol. 1968;18(2):129-133.
Eymard-Pierre E., Lesca G., et al. Infantile-onset ascending hereditary spastic paralysis is associated with mutations in the alsin gene. Am J Hum Genet. 2002;71(3):518-527.
Fan L., Simard L.R. Survival motor neuron (SMN) protein: role in neurite outgrowth and neuromuscular maturation during neuronal differentiation and development. Hum Mol Genet. 2002;11(14):1605-1614.
Fazio M. Ereditarieta della paralisi bulbare progressiva. Riforma Med. 1892;8:327.
Felderhoff-Mueser U., Grohmann K., et al. Severe spinal muscular atrophy variant associated with congenital bone fractures. J Child Neurol. 2002;17(9):718-721.
Fidzianska A., Rafalowska J. Motoneuron death in normal and spinal muscular atrophy-affected human fetuses. Acta Neuropathol (Berl). 2002;104(4):363-368.
Fischbeck K.H., Ionasescu V., et al. Localization of the gene for X-linked spinal muscular atrophy. Neurology. 1986;36(12):1595-1598.
Fisher R.L., Johnstone W.T., et al. Arthrogryposis multiplex congenita: a clinical investigation. J Pediatr. 1970;76(2):255-261.
Flaherty M.L., Wijdicks E.F., et al. Clinical and electrophysiologic patterns of flaccid paralysis due to West Nile virus. Mayo Clin Proc. 2003;78(10):1245-1248.
Fleury P., Hageman G. A dominantly inherited lower motor neuron disorder presenting at birth with associated arthrogryposis. J Neurol Neurosurg Psychiatry. 1985;48(10):1037-1048.
Francis D.A., Brown A., et al. MRI appearances of the CNS manifestations of Mycoplasma pneumoniae: a report of two cases. J Neurol. 1988;235(7):441-443.
Fried L.C., Aparicio O. Experimental ischemia of the spinal cord. Histologic studies after anterior spinal artery occlusion. Neurology. 1973;23(3):289-293.
Gallai V., Hockaday J.M., et al. Ponto-bulbar palsy with deafness (Brown-Vialetto-Van Laere syndrome). J Neurol Sci. 1981;50(2):259-275.
Gan Y.C., Sgouros S., et al. Diastematomyelia in children: treatment outcome and natural history of associated syringomyelia. Childs Nerv Syst. 2007;23(5):515-519.
Gardner W.J. Hydrodynamic Mechanism of Syringomyelia: Its Relationship to Myelocele. J Neurol Neurosurg Psychiatry. 1965;28:247-259.
Gardner W.J. Myelomeningocele, the result of rupture of the embryonic neural tube. Cleve Clin Q. 1960;27:88-100.
Gillespie J.E., Jenkins J.P., et al. Magnetic resonance imaging in syringomyelia. Acta Radiol Suppl. 1986;369:239-241.
Goebel H.H., Lenard H.G., et al. Fibre type disproportion in the rigid spine syndrome. Neuropadiatrie. 1977;8(4):467-477.
Goldberg A.B., Greenberg M.B., et al. Misoprostol and pregnancy. N Engl J Med. 2001;344(1):38-47.
Goldberg C., Fenelon G., et al. Diastematomyelia: a critical review of the natural history and treatment. Spine (Phila Pa 1976). 1984;9(4):367-372.
Gomez M.R., Clermont V., et al. Progressive bulbar paralysis in childhood (Fazio-Londe’s disease). Report of a case with pathologic evidence of nuclear atrophy. Arch Neurol. 1962;6:317-323.
Gorgen-Pauly U., Sperner J., et al. Familial pontocerebellar hypoplasia type I with anterior horn cell disease. Eur J Paediatr Neurol. 1999;3(1):33-38.
Greenberg F., Fenolio K.R., et al. X-linked infantile spinal muscular atrophy. Am J Dis Child. 1988;142(2):217-219.
Greenlee J.D., Menezes A.H., et al. Syringobulbia in a pediatric population. Neurosurgery. 2005;57(6):1147-1153. discussion 1147–1153
Greenway M.J., Andersen P.M., et al. ANG mutations segregate with familial and ‘sporadic’ amyotrophic lateral sclerosis. Nat Genet. 2006;38(4):411-413.
Grist N.R. Type A7 Coxsackie (type 4 poliomyelitis) virus infection in Scotland. J Hyg (Lond). 1962;60:323-332.
Grohmann K., Varon R., et al. Infantile spinal muscular atrophy with respiratory distress type 1 (SMARD1). Ann Neurol. 2003;54(6):719-724.
Grose C., Feorino P.M. Epstein-Barr virus and transverse myelitis. Lancet. 1973;1(7808):892.
Grose C., Henle W., et al. Primary Epstein-Barr-virus infections in acute neurologic diseases. N Engl J Med. 1975;292(8):392-395.
Gros-Louis F., Lariviere R., et al. A frameshift deletion in peripherin gene associated with amyotrophic lateral sclerosis. J Biol Chem. 2004;279(44):45951-45956.
Gros-Louis F., Meijer I.A., et al. An ALS2 gene mutation causes hereditary spastic paraplegia in a Pakistani kindred. Ann Neurol. 2003;53(1):144-145.
Grzeschik S.M., Ganta M., et al. Hydroxyurea enhances SMN2 gene expression in spinal muscular atrophy cells. Ann Neurol. 2005;58(2):194-202.
Guidera K.J., Drennan J.C.. Foot and ankle deformities in arthrogryposis multiplex congenita. Clin Orthop Relat Res. 1985(194):93-98.
Guillot N., Cuisset J.M., et al. Unusual clinical features in infantile Spinal Muscular Atrophies. Brain and Development. 2008;30(3):169-178.
Gupta A., Vitali A.M., et al. Resolution of syringomyelia and Chiari malformation after growth hormone therapy. Childs Nerv Syst. 2008;24(11):1345-1348.
Gurnett C.A., Alaee F., et al. Asymmetric lower-limb malformations in individuals with homeobox PITX1 gene mutation. Am J Hum Genet. 2008;83(5):616-622.
Guthkelch A.N., Hoffmann G.T. Tethered spinal cord in association with diastematomyelia. Surg Neurol. 1981;15(5):352-354.
Gydesen S., Brown J.M., et al. Chromosome 3 linked frontotemporal dementia (FTD-3). Neurology. 2002;59(10):1585-1594.
Hadano S., Benn S.C., et al. Mice deficient in the Rab5 guanine nucleotide exchange factor ALS2/alsin exhibit age-dependent neurological deficits and altered endosome trafficking. Hum Mol Genet. 2006;15(2):233-250.
Hadano S., Hand C.K., et al. A gene encoding a putative GTPase regulator is mutated in familial amyotrophic lateral sclerosis 2. Nat Genet. 2001;29(2):166-173.
Hader W.J., Steinbok P., et al. Intramedullary spinal teratoma and diastematomyelia. Case report and review of the literature. Pediatr Neurosurg. 1999;30(3):140-145.
Hageman G., Willemse J., et al. The pathogenesis of fetal hypokinesia. A neurological study of 75 cases of congenital contractures with emphasis on cerebral lesions. Neuropediatrics. 1987;18(1):22-33.
Hahnen E., Forkert R., et al. Molecular analysis of candidate genes on chromosome 5q13 in autosomal recessive spinal muscular atrophy: evidence of homozygous deletions of the SMN gene in unaffected individuals. Hum Mol Genet. 1995;4(10):1927-1933.
Hainline M.L., Kincaid J.C., et al. West nile poliomyelitis in a 7-year-old child. Pediatr Neurol. 2008;39(5):350-354.
Haliloglu G., Chattopadhyay A., et al. Spinal muscular atrophy with progressive myoclonic epilepsy: report of new cases and review of the literature. Neuropediatrics. 2002;33(6):314-319.
Hall J.G. Arthrogryposis multiplex congenita: etiology, genetics, classification, diagnostic approach, and general aspects. J Pediatr Orthop B. 1997;6(3):159-166.
Hand C.K., Khoris J., et al. A novel locus for familial amyotrophic lateral sclerosis, on chromosome 18q. Am J Hum Genet. 2002;70(1):251-256.
Harrington R.B., Olin R. Incomplete transverse myelitis following rabies duck embryo vaccination. JAMA. 1971;216(13):2137-2138.
Harwood-Nash D.C., McHugh K. Diastematomyelia in 172 children: the impact of modern neuroradiology. Pediatr Neurosurg. 1990;16(4–5):247-251.
Has R., Yuksel A., et al. Prenatal diagnosis of diastematomyelia: presentation of eight cases and review of the literature. Ultrasound Obstet Gynecol. 2007;30(6):845-849.
Hayes E.B., O’Leary D.R. West Nile virus infection: a pediatric perspective. Pediatrics. 2004;113(5):1375-1381.
Hayward J.C., Gillespie S.M., et al. Outbreak of poliomyelitis-like paralysis associated with enterovirus 71. Pediatr Infect Dis J. 1989;8(9):611-616.
Hennekam R.C., Barth P.G., et al. A family with severe X-linked arthrogryposis. Eur J Pediatr. 1991;150(9):656-660.
Hentati A., Ouahchi K., et al. Linkage of a commoner form of recessive amyotrophic lateral sclerosis to chromosome 15q15-q22 markers. Neurogenetics. 1998;2(1):55-60.
Heresi G.P., Mancias P., et al. Poliomyelitis-like syndrome in a child with West Nile virus infection. Pediatr Infect Dis J. 2004;23(8):788-789.
Hilal S.K., Marton D., et al. Diastematomyelia in children. Radiographic study of 34 cases. Radiology. 1974;112(3):609-621.
Hirayama K., Toyokura Y., Tsubaki T. Juvenile muscular atrophy of unilateral upper extremity; a new clinical entity. Psychiatr Neurol Japan. 1959;61:2190-2197.
Hirayama K., Tsubaki T., et al. Juvenile muscular atrophy of unilateral upper extremity. Neurology. 1963;13:373-380.
Holmes L.B., Driscoll S.G., et al. Contractures in a newborn infant of a mother with myasthenia gravis. J Pediatr. 1980;96(6):1067-1069.
Hooshmand H., Martinez A.J., et al. Arthrogryposis multiplex congenita. Simultaneous involvement of peripheral nerve and skeletal muscle. Arch Neurol. 1971;24(6):561-572.
Horga M.A., Fine A. West Nile virus. Pediatr Infect Dis J. 2001;20(8):801-802.
Hosler B.A., Siddique T., et al. Linkage of familial amyotrophic lateral sclerosis with frontotemporal dementia to chromosome 9q21-q22. JAMA. 2000;284(13):1664-1669.
Inoue M., Nakata Y., et al. Idiopathic scoliosis as a presenting sign of familial neurologic abnormalities. Spine (Phila Pa 1976). 2003;28(1):40-45.
Iqbal J.B., Bradey N., et al. Syringomyelia in children: six case reports and review of the literature. Br J Neurosurg. 1992;6(1):13-20.
Irobi J., Dierick I., et al. Unraveling the genetics of distal hereditary motor neuronopathies. Neuromolecular Med. 2006;8(1–2):131-146.
Irobi J., Van den Bergh P., et al. The phenotype of motor neuropathies associated with BSCL2 mutations is broader than Silver syndrome and distal HMN type V. Brain. 2004;127(Pt 9):2124-2130.
Irobi J., Van Impe K., et al. Hot-spot residue in small heat-shock protein 22 causes distal motor neuropathy. Nat Genet. 2004;36(6):597-601.
Iskandar B.J., Quigley M., et al. Foramen magnum cerebrospinal fluid flow characteristics in children with Chiari I malformation before and after craniocervical decompression. J Neurosurg. 2004;101(Suppl 2):169-178.
Isozumi K., DeLong R., et al. Linkage of scapuloperoneal spinal muscular atrophy to chromosome 12q24.1-q24.31. Hum Mol Genet. 1996;5(9):1377-1382.
Isu T., Chono Y., et al. Scoliosis associated with syringomyelia presenting in children. Childs Nerv Syst. 1992;8(2):97-100.
Isu T., Iwasaki Y., et al. Hydrosyringomyelia associated with a Chiari I malformation in children and adolescents. Neurosurgery. 1990;26(4):591-596. discussion 596–597
Jaeger H.J., Schmitz-Stolbrink A., et al. Cervical diastematomyelia and syringohydromyelia in a myelomeningocele patient. Eur Radiol. 1997;7(4):477-479.
James C.C., Lassman L.P. Diastematomyelia. A Critical Survey of 24 Cases Submitted to Laminectomy. Arch Dis Child. 1964;39:125-130.
Jarcho L.W., Fred H.L., et al. Encephalitis and polio-myelitis in the adult due to Coxsackie virus group B, type 5. N Engl J Med. 1963;268:235-238.
Jeha L.E., Sila C.A., et al. West Nile virus infection: a new acute paralytic illness. Neurology. 2003;61(1):55-59.
Joseph S., Robb S.A., et al. Interfamilial phenotypic heterogeneity in SMARD1. Neuromuscul Disord. 2009;19(3):193-195.
Junker A.K., Roland E.H., et al. Transverse myelitis and Epstein-Barr virus infection with delayed antibody responses. Neurology. 1991;41(9):1523-1524.
Kankirawatana P., Tennison M.B., et al. Mobius syndrome in infant exposed to cocaine in utero. Pediatr Neurol. 1993;9(1):71-72.
Kasliwal M.K., Suri A., et al. Dandy Walker malformation associated with syringomyelia. Clin Neurol Neurosurg. 2008;110(3):317-319.
Kelley T.W., Prayson R.A., et al. The neuropathology of West Nile virus meningoencephalitis. A report of two cases and review of the literature. Am J Clin Pathol. 2003;119(5):749-753.
Kelly T.E., Amoroso K., et al. Spinal muscular atrophy variant with congenital fractures. Am J Med Genet. 1999;87(1):65-68.
Kennedy W.R., Alter M., et al. Progressive proximal spinal and bulbar muscular atrophy of late onset. A sex-linked recessive trait. Neurology. 1968;18(7):671-680.
Kim S.K., Chung Y.S., et al. Diastematomyelia – clinical manifestation and treatment outcome. J Korean Med Sci. 1994;9(2):135-144.
Kizilates S.U., Talim B., et al. Severe lethal spinal muscular atrophy variant with arthrogryposis. Pediatr Neurol. 2005;32(3):201-204.
Klessinger S., Christ B. Diastematomyelia and spina bifida can be caused by the intraspinal grafting of somites in early avian embryos. Neurosurgery. 1996;39(6):1215-1223.
Kobayashi H., Baumbach L., et al. A gene for a severe lethal form of X-linked arthrogryposis (X-linked infantile spinal muscular atrophy) maps to human chromosome Xp11.3-q11.2. Hum Mol Genet. 1995;4(7):1213-1216.
Kopel F.B., Shore B., et al. Nonfatal bulbospinal paralysis due to ECHO 4 virus. J Pediatr. 1965;67(4):588-594.
Kremer H., Kuyt L.P., et al. Localization of a gene for Mobius syndrome to chromosome 3q by linkage analysis in a Dutch family. Hum Mol Genet. 1996;5(9):1367-1371.
Kwiatkowski T.J.Jr, Bosco D.A., et al. Mutations in the FUS/TLS gene on chromosome 16 cause familial amyotrophic lateral sclerosis. Science. 2009;323(5918):1205-1208.
Label L.S., Batts D.H. Transverse myelitis caused by duck embryo rabies vaccine. Arch Neurol. 1982;39(7):426-430.
Lambrechts A., Braun A., et al. Profilin II is alternatively spliced, resulting in profilin isoforms that are differentially expressed and have distinct biochemical properties. Mol Cell Biol. 2000;20(21):8209-8219.
Lance J.W., Evans W.A. Progressive myoclonic epilepsy, nerve deafness and spinal muscular atrophy. Clin Exp Neurol. 1984;20:141-151.
Landers J.E., Leclerc A.L., et al. New VAPB deletion variant and exclusion of VAPB mutations in familial ALS. Neurology. 2008;70(14):1179-1185.
La Spada A.R., Paulson H.L., et al. Trinucleotide repeat expansion in neurological disease. Ann Neurol. 1994;36(6):814-822.
La Spada A.R., Wilson E.M., et al. Androgen receptor gene mutations in X-linked spinal and bulbar muscular atrophy. Nature. 1991;352(6330):77-79.
Lassman L.P., James C.C., et al. Hydromyelia. J Neurol Sci. 1968;7(1):149-155.
Laureano A.N., Rybak L.P. Severe otolaryngologic manifestations of arthrogryposis multiplex congenita. Ann Otol Rhinol Laryngol. 1990;99(2 Pt 1):94-97.
Lavoie P., Raymond J., et al. Selective treatment of an anterior spinal artery aneurysm with endosaccular coil therapy. Case report. J Neurosurg Spine. 2007;6(5):460-464.
Lefebvre S., Burglen L., et al. Identification and characterization of a spinal muscular atrophy-determining gene. Cell. 1995;80(1):155-165.
Leis A.A., Stokic D.S., et al. A poliomyelitis-like syndrome from West Nile virus infection. N Engl J Med. 2002;347(16):1279-1280.
Leong S., Ashwell K.W. Is there a zone of vascular vulnerability in the fetal brain stem? Neurotoxicol Teratol. 1997;19(4):265-275.
Lepow M.L., Spence D.A. Effect of Trivalent Oral Poliovirus Vaccine in an Institutionalized Population with Varying Natural and Acquired Immunity to Poliomyelitis. Pediatrics. 1965;35:236-246.
Leung Y.L., Buxton N. Combined diastematomyelia and hemivertebra: a review of the management at a single centre. J Bone Joint Surg Br. 2005;87(10):1380-1384.
Levine D.N. The pathogenesis of syringomyelia associated with lesions at the foramen magnum: a critical review of existing theories and proposal of a new hypothesis. J Neurol Sci. 2004;220(1–2):3-21.
Leyden E. Ueber hydromyelus und Syringomyelie. Virchows Arch A Pathol Anat Histopathol. 1876;68:1.
Lindsey N.P., Hayes E.B., et al. West Nile virus disease in children, United States, 1999–2007. Pediatrics. 2009;123(6):e1084-e1089.
Liu Q., Dreyfuss G. A novel nuclear structure containing the survival of motor neurons protein. EMBO J. 1996;15(14):3555-3565.
Londe P. Paralysie bulbaire progressive infantile et familiale. Rev Med. 1893;13:1020.
Londe P. Paralysie bulbaire progressive infantile et familiale. Rev Med. 1894;14:212-254.
Lorson C.L., Androphy E.J. An exonic enhancer is required for inclusion of an essential exon in the SMA-determining gene SMN. Hum Mol Genet. 2000;9(2):259-265.
Lorson C.L., Hahnen E., et al. A single nucleotide in the SMN gene regulates splicing and is responsible for spinal muscular atrophy. Proc Natl Acad Sci USA. 1999;96(11):6307-6311.
Madsen P.W.3rd, Yezierski R.P., et al. Syringomyelia: clinical observations and experimental studies. J Neurotrauma. 1994;11(3):241-254.
Mandal K., Chopra N. Acute transverse myelitis following hepatitis E virus infection. Indian Pediatr. 2006;43(4):365-366.
Marjanovic B., Todorovic S., et al. Association of progressive myoclonic epilepsy and spinal muscular atrophy. Pediatr Neurol. 1993;9(2):147-150.
Markowitz J.A., Tinkle M.B., et al. Spinal muscular atrophy in the neonate. J Obstet Gynecol Neonatal Nurs. 2004;33(1):12-20.
Marques-Dias M.J., Gonzalez C.H., et al. Mobius sequence in children exposed in utero to misoprostol: neuropathological study of three cases. Birth Defects Res A Clin Mol Teratol. 2003;67(12):1002-1007.
Mawatari S., Katayama K. Scapuloperoneal muscular atrophy with cardiopathy. An X-linked recessive trait. Arch Neurol. 1973;28(1):55-59.
McEntagart M., Dunstan M., et al. Clinical and genetic heterogeneity in peroneal muscular atrophy associated with vocal cord weakness. J Neurol Neurosurg Psychiatry. 2002;73(6):762-765.
McGrory B.J., Burke D.W., et al. Posterior instability of the hip in an adult. A case report. Clin Orthop Relat Res. 1997(341):151-154.
McShane M.A., Boyd S., et al. Progressive bulbar paralysis of childhood. A reappraisal of Fazio-Londe disease. Brain. 1992;115(Pt 6):1889-1900.
McWhorter M.L., Monani U.R., et al. Knockdown of the survival motor neuron (Smn) protein in zebrafish causes defects in motor axon outgrowth and pathfinding. J Cell Biol. 2003;162(5):919-931.
Meacham W.F. Surgical treatment of diastematomyelia. J Neurosurg. 1967;27(1):78-85.
Meister G., Buhler D., et al. A multiprotein complex mediates the ATP-dependent assembly of spliceosomal U snRNPs. Nat Cell Biol. 2001;3(11):945-949.
Melki J., Abdelhak S., et al. Gene for chronic proximal spinal muscular atrophies maps to chromosome 5q. Nature. 1990;344(6268):767-768.
Melki J., Lefebvre S., et al. De novo and inherited deletions of the 5q13 region in spinal muscular atrophies. Science. 1994;264(5164):1474-1477.
Mellins R.B., Hays A.P., et al. Respiratory distress as the initial manifestation of Werdnig-Hoffmann disease. Pediatrics. 1974;53(1):33-40.
Menezes A.H. Chiari I malformations and hydromyelia – complications. Pediatr Neurosurg. 1991;17(3):146-154.
Middleton L.T., Christodoulou K., et al. Distal hereditary motor neuronopathy of the Jerash type. Ann N Y Acad Sci. 1999;883:439-442.
Miller A., Guille J.T., et al. Evaluation and treatment of diastematomyelia. J Bone Joint Surg Am. 1993;75(9):1308-1317.
Moerman P., Fryns J.P., et al. Congenital muscular dystrophy associated with lethal arthrogryposis multiplex congenita. Virchows Arch A Pathol Anat Histopathol. 1985;408(1):43-48.
Moes C.A., Hendrick E.B. Diastematomyelia. J Pediatr. 1963;63:238-248.
Moreira M.C., Klur S., et al. Senataxin, the ortholog of a yeast RNA helicase, is mutant in ataxia-ocular apraxia 2. Nat Genet. 2004;36(3):225-227.
Morita M., Al-Chalabi A., et al. A locus on chromosome 9p confers susceptibility to ALS and frontotemporal dementia. Neurology. 2006;66(6):839-844.
Mourelatos Z., Abel L., et al. SMN interacts with a novel family of hnRNP and spliceosomal proteins. EMBO J. 2001;20(19):5443-5452.
Munsat T.L. Poliomyelitis – new problems with an old disease. N Engl J Med. 1991;324(17):1206-1207.
Munsat T.L., Davies K.E. International SMA consortium meeting. (26–28 June 1992, Bonn, Germany). Neuromuscul Disord. 1992;2(5–6):423-428.
Munshi I., Frim D., et al. Effects of posterior fossa decompression with and without duraplasty on Chiari malformation-associated hydromyelia. Neurosurgery. 2000;46(6):1384-1389. discussion 1389–1390
Murphy R.L., Tubbs R.S., et al. Chiari I malformation and idiopathic growth hormone deficiency in siblings. Childs Nerv Syst. 2006;22(6):632-634.
Murphy R.L., Tubbs R.S., et al. Chiari I malformation and idiopathic growth hormone deficiency in siblings: report of three cases. Childs Nerv Syst. 2007;23(10):1221-1223.
Nalini A., Lokesh L., et al. Familial monomelic amyotrophy: a case report from India. J Neurol Sci. 2004;220(1–2):95-98.
Nelson C.E., Morgan B.A., et al. Analysis of Hox gene expression in the chick limb bud. Development. 1996;122(5):1449-1466.
Netsky M.G. Diffuse meningiomatosis, arachnoidal fibrosis, and syringomyelia. AMA Arch Neurol Psychiatry. 1957;78(6):553-561.
Nevo Y., Kramer U., et al. SMA type 2 unrelated to chromosome 5q13. Am J Med Genet. 1998;75(2):193-195.
Newman P.K., Terenty T.R., et al. Some observations on the pathogenesis of syringomyelia. J Neurol Neurosurg Psychiatry. 1981;44(11):964-969.
Nishimura A.L., Mitne-Neto M., et al. A mutation in the vesicle-trafficking protein VAPB causes late-onset spinal muscular atrophy and amyotrophic lateral sclerosis. Am J Hum Genet. 2004;75(5):822-831.
Norman R.M. Cerebellar hypoplasia in Werdnig-Hoffmann disease. Arch Dis Child. 1961;36:96-101.
Nussinovitch M., Brand N., et al. Transverse myelitis following mumps in children. Acta Paediatr. 1992;81(2):183-184.
O’Sullivan D.J., McLeod J.G. Distal chronic spinal muscular atrophy involving the hands. Clin Exp Neurol. 1977;14:256-259.
Oberoi G.S., Kaul H.L., et al. Anaesthesia in arthrogryposis multiplex congenita: case report. Can J Anaesth. 1987;34(3 Pt 1):288-290.
Oller-Navarro J.L., Rivera-Reyes L., et al. Juvenile diabetic myelopathy. A 14-year-old girl with juvenile diabetes mellitus complicated by acute transverse myelopathy. Clin Pediatr (Phila). 1979;18(1):60-61.
Otter S., Grimmler M., et al. A comprehensive interaction map of the human survival of motor neuron (SMN) complex. J Biol Chem. 2007;282(8):5825-5833.
Owens J.C., Swan H. Complications in the Repair of Coarctation of the Aorta. J Cardiovasc Surg (Torino). 1963;4:816-825.
Parisi A., Filice G. Transverse myelitis associated with Mycoplasma pneumoniae pneumonitis: a report of two cases. Infez Med. 2001;9(1):39-42.
Parkes J.D. Genetic factors in human sleep disorders with special reference to Norrie disease, Prader-Willi syndrome and Moebius syndrome. J Sleep Res. 1999;8(Suppl 1):14-22.
Parush S., Yehezkehel I., et al. Developmental correlates of school-age children with a history of benign congenital hypotonia. Dev Med Child Neurol. 1998;40(7):448-452.
Pastuszak A.L., Schuler L., et al. Use of misoprostol during pregnancy and Mobius’ syndrome in infants. N Engl J Med. 1998;338(26):1881-1885.
Pattany P.M., Saraf-Lavi E., et al. MR angiography of the spine and spinal cord. Top Magn Reson Imaging. 2003;14(6):444-460.
Pealer L.N., Marfin A.A., et al. Transmission of West Nile virus through blood transfusion in the United States in 2002. N Engl J Med. 2003;349(13):1236-1245.
Pellizzoni L., Yong J., et al. Essential role for the SMN complex in the specificity of snRNP assembly. Science. 2002;298(5599):1775-1779.
Pena C.E., Miller F., et al. Arthrogryposis multiplex congenita. Report of two cases of a radicular type with familial incidence. Neurology. 1968;18(9):926-930.
Petajan J.H., Momberger G.L., et al. Arthrogryposis syndrome (Kuskokwim disease) in the Eskimo. JAMA. 1969;209(10):1481-1486.
Petersen L.R., Marfin A.A. West Nile virus: a primer for the clinician. Ann Intern Med. 2002;137(3):173-179.
Pfeifer J.D. Basicranial diastematomyelia: a case report. Clin Neuropathol. 1991;10(5):232-236.
Pidcock F.S., Krishnan C., et al. Acute transverse myelitis in childhood: center-based analysis of 47 cases. Neurology. 2007;68(18):1474-1480.
Pugh R.C., Dudgeon J.A. Fatal neonatal poliomyelitis. Arch Dis Child. 1954;29(147):381-384.
Puls I., Jonnakuty C., et al. Mutant dynactin in motor neuron disease. Nat Genet. 2003;33(4):455-456.
Puls I., Oh S.J., et al. Distal spinal and bulbar muscular atrophy caused by dynactin mutation. Ann Neurol. 2005;57(5):687-694.
Quinn C.M., Wigglesworth J.S., et al. Lethal arthrogryposis multiplex congenita: a pathological study of 21 cases. Histopathology. 1991;19(2):155-162.
Rabin B.A., Griffin J.W., et al. Autosomal dominant juvenile amyotrophic lateral sclerosis. Brain. 1999;122(Pt 8):1539-1550.
Ramser J., Ahearn M.E., et al. Rare missense and synonymous variants in UBE1 are associated with X-linked infantile spinal muscular atrophy. Am J Hum Genet. 2008;82(1):188-193.
Reed C.M., Campbell S.E., et al. Atlanto-occipital dislocation with traumatic pseudomeningocele formation and post-traumatic syringomyelia. Spine (Phila Pa 1976). 2005;30(5):E128-E133.
Renbaum P., Kellerman E., et al. Spinal muscular atrophy with pontocerebellar hypoplasia is caused by a mutation in the VRK1 gene. Am J Hum Genet. 2009;85(2):281-289.
Roberts D.J., Tabin C. The genetics of human limb development. Am J Hum Genet. 1994;55(1):1-6.
Rochette C.F., Gilbert N., et al. SMN gene duplication and the emergence of the SMN2 gene occurred in distinct hominids: SMN2 is unique to Homo sapiens. Hum Genet. 2001;108(3):255-266.
Rodesch G., Hurth M., et al. Angio-architecture of spinal cord arteriovenous shunts at presentation. Clinical correlations in adults and children. The Bicetre experience on 155 consecutive patients seen between 1981–1999. Acta Neurochir (Wien). 2004;146(3):217-226. discussion 226–217
Romanes G.J. The motor cell columns of the lumbo-sacral spinal cord of the cat. J Comp Neurol. 1951;94(2):313-363.
Rosen D.R., Siddique T., et al. Mutations in Cu/Zn superoxide dismutase gene are associated with familial amyotrophic lateral sclerosis. Nature. 1993;362(6415):59-62.
Rossoll W., Jablonka S., et al. Smn, the spinal muscular atrophy-determining gene product, modulates axon growth and localization of beta-actin mRNA in growth cones of motoneurons. J Cell Biol. 2003;163(4):801-812.
Rossoll W., Kroning A.K., et al. Specific interaction of Smn, the spinal muscular atrophy determining gene product, with hnRNP-R and gry-rbp/hnRNP-Q: a role for Smn in RNA processing in motor axons? Hum Mol Genet. 2002;11(1):93-105.
Ruddy D.M., Parton M.J., et al. Two families with familial amyotrophic lateral sclerosis are linked to a novel locus on chromosome 16q. Am J Hum Genet. 2003;73(2):390-396.
Rudnik-Schoneborn S., Stolz P., et al. Long-term observations of patients with infantile spinal muscular atrophy with respiratory distress type 1 (SMARD1). Neuropediatrics. 2004;35(3):174-182.
Rudnik-Schoneborn S., Sztriha L., et al. Extended phenotype of pontocerebellar hypoplasia with infantile spinal muscular atrophy. Am J Med Genet A. 2003;117A(1):10-17.
Russman B.S. Spinal muscular atrophy: clinical classification and disease heterogeneity. J Child Neurol. 2007;22(8):946-951.
Ryan M.M., Cooke-Yarborough C.M., et al. Anterior horn cell disease and olivopontocerebellar hypoplasia. Pediatr Neurol. 2000;23(2):180-184.
Sampson B.A., Nields H., et al. Muscle weakness in West Nile encephalitis is due to destruction of motor neurons. Hum Pathol. 2003;34(6):628-629.
Sapp P.C., Hosler B.A., et al. Identification of two novel loci for dominantly inherited familial amyotrophic lateral sclerosis. Am J Hum Genet. 2003;73(2):397-403.
Sarnat H.B., O’Connor T., et al. Clinical effects of myotonic dystrophy on pregnancy and the neonate. Arch Neurol. 1976;33(7):459-465.
Scatliff J.H., Hayward R., et al. Pre- and post-operative hydromyelia in spinal dysraphism. Pediatr Radiol. 2005;35(3):282-289.
Schijman E. Split spinal cord malformations: report of 22 cases and review of the literature. Childs Nerv Syst. 2003;19(2):96-103.
Schlegel U., Jerusalem F., et al. Benign juvenile focal muscular atrophy of upper extremities – a familial case. J Neurol Sci. 1987;80(2–3):351-353.
Schmalbruch H., Kamieniecka Z., et al. Early fatal nemaline myopathy: case report and review. Dev Med Child Neurol. 1987;29(6):800-804.
Schmutz J., Martin J., et al. The DNA sequence and comparative analysis of human chromosome 5. Nature. 2004;431(7006):268-274.
Schrank B., Gotz R., et al. Inactivation of the survival motor neuron gene, a candidate gene for human spinal muscular atrophy, leads to massive cell death in early mouse embryos. Proc Natl Acad Sci USA. 1997;94(18):9920-9925.
Schurch B., Wichmann W., et al. Post-traumatic syringomyelia (cystic myelopathy): a prospective study of 449 patients with spinal cord injury. J Neurol Neurosurg Psychiatry. 1996;60(1):61-67.
Sells J.M., Jaffe K.M., et al. Amyoplasia, the most common type of arthrogryposis: the potential for good outcome. Pediatrics. 1996;97(2):225-231.
Servais L.J., Rivelli S.K., et al. Anterior spinal artery syndrome after aortic surgery in a child. Pediatr Neurol. 2001;24(4):310-312.
Sharma A., Lambrechts A., et al. A role for complexes of survival of motor neurons (SMN) protein with gemins and profilin in neurite-like cytoplasmic extensions of cultured nerve cells. Exp Cell Res. 2005;309(1):185-197.
Sheehan J.P., Sheehan J.M., et al. Thoracic diastematomyelia with concurrent intradural epidermoid spinal cord tumor and cervical syrinx in an adult. Case report. J Neurosurg. 2002;97(Suppl 2):231-234.
Shepard T.H. Mobius syndrome after misoprostol: a possible teratogenic mechanism. Lancet. 1995;346(8977):780.
Shibata N. Transgenic mouse model for familial amyotrophic lateral sclerosis with superoxide dismutase-1 mutation. Neuropathology. 2001;21(1):82-92.
Shuper A., Weitz R., et al. Benign congenital hypotonia. A clinical study in 43 children. Eur J Pediatr. 1987;146(4):360-364.
Shyamalan N.C., Singh S.S., et al. Transverse Myelitis after Vaccination. Br Med J. 1964;1(5380):434-435.
Siddique T., Figlewicz D.A., et al. Linkage of a gene causing familial amyotrophic lateral sclerosis to chromosome 21 and evidence of genetic – locus heterogeneity. N Engl J Med. 1991;324:1381-1384.
Silver J.R. History of post-traumatic syringomyelia: post traumatic syringomyelia prior to 1920. Spinal Cord. 2001;39(3):176-183.
Skibinski G., Parkinson N.J., et al. Mutations in the endosomal ESCRTIII-complex subunit CHMP2B in frontotemporal dementia. Nat Genet. 2005;37(8):806-808.
Slee J.J., Smart R.D., et al. Deletion of chromosome 13 in Moebius syndrome. J Med Genet. 1991;28(6):413-414.
Smit L.M., Barth P.G. Arthrogryposis multiplex congenita due to congenital myasthenia. Dev Med Child Neurol. 1980;22(3):371-374.
Solomon T., Willison H. Infectious causes of acute flaccid paralysis. Curr Opin Infect Dis. 2003;16(5):375-381.
Solund K., Sonne-Holm S., et al. Talectomy for equinovarus deformity in arthrogryposis. A 13 (2–20) year review of 17 feet. Acta Orthop Scand. 1991;62(4):372-374.
Sonigo-Cohen P., Schmit P., et al. Prenatal diagnosis of diastematomyelia. Childs Nerv Syst. 2003;19(7–8):555-560.
Spagnoli L.G., Palmieri G., et al. Benign congenital hypotonia with uniform type 1 fibers and aspecific ultrastructural changes in the muscle: a case with esophagus involvement. Ital J Neurol Sci. 1985;6(3):317-321.
Sperfeld A.D., Karitzky J., et al. X-linked bulbospinal neuronopathy: Kennedy disease. Arch Neurol. 2002;59(12):1921-1926.
Sreedharan J., Blair I.P., et al. TDP-43 mutations in familial and sporadic amyotrophic lateral sclerosis. Science. 2008;319(5870):1668-1672.
Steegman A.T. Syndrome of the anterior spinal artery. Neurology. 1952;2(1):15-35.
Stoll C., Ehret-Mentre M.C., et al. Prenatal diagnosis of congenital myasthenia with arthrogryposis in a myasthenic mother. Prenat Diagn. 1991;11(1):17-22.
Striano P., Boccella P., et al. Spinal muscular atrophy and progressive myoclonic epilepsy: one case report and characteristics of the epileptic syndrome. Seizure. 2004;13(8):582-586.
Suh S.W., Sarwark J.F., et al. Evaluating congenital spine deformities for intraspinal anomalies with magnetic resonance imaging. J Pediatr Orthop. 2001;21(4):525-531.
Summers B.A., Swash M., et al. Juvenile-onset bulbospinal muscular atrophy with deafness: Vialetta-van Laere syndrome or Madras-type motor neuron disease? J Neurol. 1987;234(6):440-442.
Sung S.S., Brassington A.M., et al. Mutations in genes encoding fast-twitch contractile proteins cause distal arthrogryposis syndromes. Am J Hum Genet. 2003;72(3):681-690.
Sung S.S., Brassington A.M., et al. Mutations in TNNT3 cause multiple congenital contractures: a second locus for distal arthrogryposis type 2B. Am J Hum Genet. 2003;73(1):212-214.
Suresh P.A., Deepa C. Congenital suprabulbar palsy: a distinct clinical syndrome of heterogeneous aetiology. Dev Med Child Neurol. 2004;46(9):617-625.
Suzuki K., Katsuno M., et al. CAG repeat size correlates to electrophysiological motor and sensory phenotypes in SBMA. Brain. 2008;131(Pt 1):229-239.
Tagawa A., Tan C.F., et al. Familial amyotrophic lateral sclerosis: a SOD1-unrelated Japanese family of bulbar type with Bunina bodies and ubiquitin-positive skein-like inclusions in lower motor neurons. Acta Neuropathol (Berl). 2007;113(2):205-211.
Takata R.I., Speck Martins C.E., et al. A new locus for recessive distal spinal muscular atrophy at Xq13.1-q21. J Med Genet. 2004;41(3):224-229.
Tandan R., Sharma K.R., et al. Chronic segmental spinal muscular atrophy of upper extremities in identical twins. Neurology. 1990;40(2):236-239.
Tang B.S., Luo W., et al. A new locus for autosomal dominant Charcot-Marie-Tooth disease type 2 (CMT2L) maps to chromosome 12q24. Hum Genet. 2004;114(6):527-533.
Thomas H.M. Congenital facial paralysis. J Nerv Ment Dis. 1898;25:571-593.
Thompson C.E. Benign congenital hypotonia is not a diagnosis. Dev Med Child Neurol. 2002;44(4):283-284.
Thron A., Schroth G. Magnetic resonance imaging (MRI) of diastematomyelia. Neuroradiology. 1986;28(4):371-372.
Timmerman V., Raeymaekers P., et al. Linkage analysis of distal hereditary motor neuropathy type II (distal HMN II) in a single pedigree. J Neurol Sci. 1992;109(1):41-48.
Tobin W.D., Layton D.D. The diagnosis and natural history of spinal cord arteriovenous malformations. Mayo Clin Proc. 1976;51(10):637-646.
Tsutsumi H., Kamazaki H., et al. Sequential development of acute meningoencephalitis and transverse myelitis caused by Epstein-Barr virus during infectious mononucleosis. Pediatr Infect Dis J. 1994;13(7):665-667.
Tubbs R.S., Iskandar B.J., et al. A critical analysis of the Chiari 1.5 malformation. J Neurosurg. 2004;101(Suppl 2):179-183.
Vance C., Al-Chalabi A., et al. Familial amyotrophic lateral sclerosis with frontotemporal dementia is linked to a locus on chromosome 9p13.2-21.3. Brain. 2006;129(Pt 4):868-876.
Vance C., Rogelj B., et al. Mutations in FUS, an RNA processing protein, cause familial amyotrophic lateral sclerosis type 6. Science. 2009;323(5918):1208-1211.
van der Vleuten A.J., van Ravenswaaij-Arts C.M., et al. Localisation of the gene for a dominant congenital spinal muscular atrophy predominantly affecting the lower limbs to chromosome 12q23-q24. Eur J Hum Genet. 1998;6(4):376-382.
van der Zee J., Urwin H., et al. CHMP2B C-truncating mutations in frontotemporal lobar degeneration are associated with an aberrant endosomal phenotype in vitro. Hum Mol Genet. 2008;17(2):313-322.
van de Warrenburg B.P., Scheffer H., et al. BSCL2 mutations in two Dutch families with overlapping Silver syndrome-distal hereditary motor neuropathy. Neuromuscul Disord. 2006;16(2):122-125.
Van Laere J. Paralysie bulbo-pontine chronique frogressive familial avec surdite: un cas de syndrome de Klippel-Trenaunay das la meme fratrie (problems diagnostiques et gentiques). Rev Neurol (Paris). 1966;115:289-295.
Vaquero J., Martinez R., et al. Syringomyelia-Chiari complex: magnetic resonance imaging and clinical evaluation of surgical treatment. J Neurosurg. 1990;73(1):64-68.
Vargas F.R., Schuler-Faccini L., et al. Prenatal exposure to misoprostol and vascular disruption defects: a case-control study. Am J Med Genet. 2000;95(4):302-306.
Vassilopoulos D., Emery A.E. Quantitative histochemistry of the spinal motor neurone nucleus during human fetal development. J Neurol Sci. 1977;32(2):275-281.
Venketasubramanian N. Transverse myelitis following mumps in an adult — a case report with MRI correlation. Acta Neurol Scand. 1997;96(5):328-331.
Verschuuren-Bemelmans C.C., Winter P., et al. Novel homozygous ALS2 nonsense mutation (p.Gln715X) in sibs with infantile-onset ascending spastic paralysis: the first cases from northwestern Europe. Eur J Hum Genet. 2008;16(11):1407-1411.
Verzijl H.T., van den Helm B., et al. A second gene for autosomal dominant Mobius syndrome is localized to chromosome 10q, in a Dutch family. Am J Hum Genet. 1999;65(3):752-756.
Verzijl H.T., van der Zwaag B., et al. The neuropathology of hereditary congenital facial palsy vs Mobius syndrome. Neurology. 2005;64(4):649-653.
Vialetto E. Contibuto alla forma ereditaria della paralisi bulbare progressiva. Riv Sper Frenait. 1936;40:1-24.
Vitte J., Fassier C., et al. Refined characterization of the expression and stability of the SMN gene products. Am J Pathol. 2007;171(4):1269-1280.
Vlaanderen W., Manschot T.A., et al. A dominant-hereditary variation of the Pena-Shokeir syndrome; a case report. Eur J Obstet Gynecol Reprod Biol. 1991;40(2):163-165.
Walton J.N. The limp child. J Neurol Neurosurg Psychiatry. 1957;20(2):144-154.
Wang C.H., Finkel R.S., et al. Consensus statement for standard of care in spinal muscular atrophy. J Child Neurol. 2007;22(8):1027-1049.
Wang C.H., Kleyn P.W., et al. Refinement of the spinal muscular atrophy locus by genetic and physical mapping. Am J Hum Genet. 1995;56(1):202-209.
Weenink H.R., Smilde J. Spinal Cord Lesions Due to Coarctatio Aortae. Psychiatr Neurol Neurochir. 1964;67:259-269.
Williams B. Difficult labour as a cause of communicating syringomyelia. Lancet. 1977;2(8028):51-53.
Windpassinger C., Auer-Grumbach M., et al. Heterozygous missense mutations in BSCL2 are associated with distal hereditary motor neuropathy and Silver syndrome. Nat Genet. 2004;36(3):271-276.
Wisoff J.H. Hydromyelia: a critical review. Childs Nerv Syst. 1988;4(1):1-8.
Wong M., Connolly A.M., et al. Poliomyelitis-like syndrome associated with Epstein-Barr virus infection. Pediatr Neurol. 1999;20(3):235-237.
Yachnis A.T., Giovanini M.A., et al. Developmental patterns of BCL-2 and BCL-X polypeptide expression in the human spinal cord. Exp Neurol. 1998;150(1):82-97.
Yamanaka K., Miller T.M., et al. Progressive spinal axonal degeneration and slowness in ALS2-deficient mice. Ann Neurol. 2006;60(1):95-104.
Yang L., Jones N.R., et al. Excitotoxic model of post-traumatic syringomyelia in the rat. Spine (Phila Pa 1976). 2001;26(17):1842-1849.
Yang Y., Hentati A., et al. The gene encoding alsin, a protein with three guanine-nucleotide exchange factor domains, is mutated in a form of recessive amyotrophic lateral sclerosis. Nat Genet. 2001;29(2):160-165.
Yoshiyama Y., Tokumaru Y., et al. Flexion-induced cervical myelopathy associated with fewer elastic fibers and thickening in the posterior dura mater. J Neurol. 2010;257(1):149-151.
Young I.D., Harper P.S. Hereditary distal spinal muscular atrophy with vocal cord paralysis. J Neurol Neurosurg Psychiatry. 1980;43(5):413-418.
Zellweger H. The floppy infant: a practical approach. Helv Paediatr Acta. 1983;38(4):301-306.
Zhang F.F., Tang B.S., et al. Mutation analysis of small heat-shock protein 22 gene in Chinese patients with Charcot-Marie-Tooth disease. Zhonghua Yi Xue Yi Chuan Xue Za Zhi. 2005;22(4):361-363.
Zhang H.L., Pan F., et al. Active transport of the survival motor neuron protein and the role of exon-7 in cytoplasmic localization. J Neurosci. 2003;23(16):6627-6637.
Zhang H., Xing L., et al. Multiprotein complexes of the survival of motor neuron protein SMN with Gemins traffic to neuronal processes and growth cones of motor neurons. J Neurosci. 2006;26(33):8622-8632.