CHAPTER 28 Anesthesia for Organ Transplantation
Since the inception of the United Network of Organ Sharing (UNOS) in 1987, nearly half a million solid organ transplants have been performed in the United States. Of these, 375,000 have been from deceased donors, or donation after cardiac death (DCD), and 103,000 have been from living donors (UNOS 4-2010) (Tables 28-1 and 28-2). The total number of pediatric transplants has reached nearly 37,000, and the total number of living donors is 9200 for all pediatric patients. In 2009, the yearly totals for pediatric organ transplants increased significantly for the first time in 6 years, to a total of 2019, from a low of 1964 transplants in both of the years 2005 and 2008. Unfortunately, the yearly total of pediatric living donors decreased from a decade high of 560 in 2001, to 387 in 2009. The incidence of end-stage organ disease increases with age, and children still account for only a small fraction (7.60%) of the total number of transplant recipients.
With the new changes, Organ Procurement Organization (OPO) members must submit data to the Organ Procurement and Transplantation Network (OPTN) under the directorship of the U.S. Department of Health and Human Services through the use of standardized forms and electronic databases. There are 11 OPTNs in the United States and Puerto Rico (Fig. 28-1). In addition to providing data on all deceased donors, living donors, potential transplant recipients, and actual transplant recipients, all OPOs must also submit of the total number of reported deaths by donor hospital.
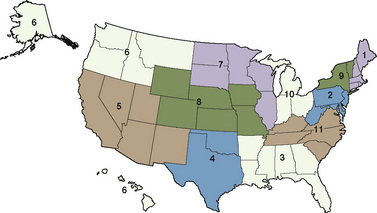
FIGURE 28-1 A map of the 11 organ procurement and transplantation networks in the United States in 2010.
(From the United Network for Organ Sharing (UNOS) and Organ Procurement and Transplantation Network (OPTN) data, 2010. Available at http://optn.transplant.hrsa.gov/data.)
As of January 2010, there are 486 transplant centers or hospitals in the United States that operate one or more organ transplant programs. These centers house 1136 types of solid organ transplantation programs, managed by the 11 OPTNs (UNOS-OPTN, April 2010), as reported by the Health Resources and Services Administration of Health and Human Services. Data extracted from the UNOS database suggest that there are currently over 100,000 candidates awaiting transplantation. Table 28-3 demonstrates the numbers of pediatric and adult candidates awaiting organ transplantation by organ type. Pediatric patients outnumber these adults only in the category of intestinal transplantation.
The number of pediatric candidates awaiting organ transplantation pales in comparison with the total number of available organs. In fact, for the past 5 years, the total number of patients awaiting transplantation (~100,000) is one fifth of the total number of patients transplanted since 1985 (~500,000). Although organ transplantation procedures are relatively infrequent compared with the over 50 million surgical procedures performed per year in the United States, perioperative care for these patients has become highly specialized. Maintenance of physiologic homeostasis during the removal of a failed native organ and the subsequent allograft reperfusion period are challenging for both anesthesiologists and surgeons. This chapter focuses on the current body of knowledge for each type of major solid organ transplantation, with a specific focus on special considerations for the management of children. In addition, cellular transplantation, stem-cell transplantation, immunosuppression, complications, graft-versus-host disease, and posttransplant lymphoproliferative disease will be addressed. Information on artificial assist devices being used in the treatment of end-organ failure or as a bridge to transplantation, and information on xenotransplantation. See related video online at www.expertconsult.com.
Determination of brain death in children
The pediatric central nervous system (CNS) may be more resilient to certain forms of injury, a fact that should be considered when interpreting diagnostic evidence and confirming brain death in infants and children. Brain death is defined as the absence of cortical and cerebral function without preservation of brainstem function. When measured in this setting, cerebral blood flow (CBF) is absent. Publication of brain death criteria specifically addressing findings in infants and children has clarified most of the ambiguities created by age-related neurologic differences. The Task Force for the Uniform Determination of Brain Death in Children was assembled in 1987 (Report of Special Task Force, Pediatrics, 1988). Box 28-1 summarizes the currently accepted guidelines for determination of brain death in neonates, infants, and children.
Box 28-1 Guidelines for Brain Death in Children
If hypoxic encephalopathy is present, observation for 24 hours is recommended. This time may be reduced if an electroencephalogram shows electrocortical silence, or if a radionuclide study is negative for CBF. In patients with electrocortical silence, metabolic coma must be excluded. Detailed reviews of these guidelines and their applications have been published (Ashwal and Schneider, 1991; Ashwal, 1993; Koszer and Moshe, 2010). Prospective donors must fully meet the age-appropriate criteria and be declared legally brain dead before attempting to obtain consent for organ donation from their family. A physician must record the official time of death in the chart before transferring the donor to the operating room for the organ procurement procedure. Although the donor is officially dead, the care of an anesthesiologist is needed during these procedures to continue efforts to maintain cardiac output, at least until the viscera have been flushed with the cold preservative solution. At this point, ventilation can be discontinued and the responsibility of the anesthesiologist is concluded.
One area that remains particularly controversial is the appropriateness of organ donation from anencephalic newborns. This developmental anomaly results in absence of the cerebral cortex and upper brainstem, so these infants possess no potential for normal neurologic development. Despite the uniformly fatal outcome in these cases, such infants never meet the usual criteria for brain death (Baird and Sadovnick, 1984).
Donor strategies
Organ donation in the pediatric population has decreased by almost 25% in the past 15 years, from 1201 in 1995, to 919 in 2009, with a low in 2008 of 879, the lowest number of pediatric donors since the inception of UNOS data collection (Table 28-4). Additionally, living donation has also decreased significantly in the past 5 years, a result of the ethics controversy and the complications that are now apparent with respect to living donation. The distribution of pediatric donor ages has remained consistent, with close to 60% being between the ages of 11 and 17 (Table 28-5). Although the number of adult donors is significantly greater than the number of pediatric donors, the percentage of recovered organs in the pediatric age range is generally greater than the percent of adult transplanted organs. For deceased kidney donors, 94.7% of the pediatric kidneys recovered were transplanted compared with 91.6% of the kidneys recovered from donors aged 18 to 49 years. There was a similar trend for pancreas donors (81.4% versus 72.2%), liver donors (96.0% versus 91.4%), and intestine donors (94.7% versus 85.7%). For heart and lung donors, the percentage of recovered organs transplanted was high, and it was similar for both pediatric donors and adult donors aged 18 to 49 years (98.9% versus 99.0% for heart and 98.1% versus 96.4% for lung) (OPTN/SRTR, 2010).
TABLE 28-4 Donors of Organs Recovered in the United States between January 1, 2001 and January 31, 2010
Recovery of organs from deceased donors requires continuing care of the patient’s preexisting condition, with a shift in primary emphasis from minimizing neurologic injury and preserving life to protection of specific organs. Principles of pediatric donor care are comparable with those of adults, but meticulous adjustments are necessary because of the special physiologic needs of children. The leading causes of death in children are asphyxia and trauma, so a history of cardiac resuscitation, hypotension, and hypoxemia is frequent in this donor population (Fischer-Froehlich et al., 2002).
Pediatric donation management goals and dosing guidelines are available for reference from NATCO (Nakagawa, 2008b), the UNOS Critical Pathway for the Pediatric Organ Donor (www.unos.org), and the Canadian Council for Donation and Transplantation (www.ccdt.ca) (Shemie et al., 2006). In 2001, a Consensus Conference made specific recommendations for management of cardiac donors (Zaroff et al., 2002). See also Kutsogiannis (2006), Braunfeld (2004), Wood et al. (2004), and Ullah et al. (2006).
Ultimately, the management of the pediatric organ donation is dictated by regional standards of care and the physicians caring for the child. The primary responsibilities of the pediatric intensive care specialist and anesthesiologist are to anticipate the normal physiologic sequelae of brain death (Table 28-6), and to direct therapy toward normalizing gross alterations in physiologic and biochemical parameters.
Sequela | Cause | Management |
Hypotension | Neurogenic shock, hypovolemia, hypothermia, electrolyte disorders, endocrine abnormalities, myocardial dysfunction | Mean arterial pressure > 60 mm Hg CVP 4 to 12 mm Hg PCWP 8 to 12 mm Hg SVR 800 to 1200 dyne/sec per cm5 Cardiac index > 2.4 L/min per m2 Inotropic support in order of preference (in mcg/kg per min): |
Steroids: methylprednisolone (15 mg/kg)
T3: 4-mcg bolus and 3 mcg/hr infusion
Arginine vasopressin: 1-U bolus, then continuous infusion at 0.5 to 4 U/hr, titrated to a systemic vascular resistance of 800 to 1200 dyne/sec per cm5
Insulin: 1 U/hr minimum. Titrate to maintain blood sugar 120 to 180 mg/dL
At <30° C, may be unresponsive
to ACLS, drug therapy, defibrillation, or pacing
ACLS, Advanced cardiac life support; CVP, cerebrovascular pressure; DIC, disseminated intravascular coagulation; PCWP, pulmonary capillary wedge pressure; SVR, systemic vascular resistance.
From Rosengard B, Feng S, Alfrey E, et al: Report of the Crystal City meeting to maximize the use of organs recovered from the cadaver donor, Am J Transplant 2:701, 2002.
Organs from deceased donors may be injured or damaged from many things: the preexisting comorbid factors; hemodynamic instability (autonomic surge, hypotension, cardiac ischemia, metabolic acidosis, and use of vasopressors); endocrine, metabolic and electrolyte imbalances; hypoxemia (acute respiratory distress syndrome [ARDS]); coagulopathy; thrombocytopenia; renal failure; endotoxin release; and immune activation inherent in the brain death process (Jawan et al., 2002; Zaroff et al., 2002). The presence of brain death–related complications has been reported to have no effect on the number of organs donated if an aggressive donation management protocol is instituted (DuBose and Salim, 2008).
Organ Retrieval
Organ procurement is usually completed in 4 hours or less, depending on the operating team’s experience, the number of organs intended for retrieval, and the presence of variations in the vascular anatomy. In the face of worsening hypoxemia, coagulopathy, or refractory hypotension, the organ recovery surgery should be expedited to prevent warm ischemic injury to transplantable organs. The specific organs to be retrieved determine the type of fluid management, the ideal central venous pressure (CVP), the fraction of inspired oxygen (Fio2), and the choice of inotropic or vasopressor support and maximum allowable dosages. Management decisions depend on the organs being considered; for example, hemodynamic goals preferred by abdominal transplant surgeons occasionally differ from those of cardiothoracic transplant surgeons. Pediatric donation management goals are summarized in (Table 28-7) (Nakagawa, 2008b).
Strict asepsis is observed, prophylactic antibiotics are administered, and the donor is positioned and prepared surgically. If the corneas are to be harvested, the eyelids are taped shut and covered with cold saline compresses or ice packs for corneal protection. After 300 U/kg of heparin is administered and cardioplegia is achieved, the liver, intestines, pancreas, and kidneys are flushed with cold preservation solution, and sequential removal of organs can proceed. The spleen and omental lymph nodes are removed for tissue typing, and the aorta, inferior vena cava, and common carotid and iliac vessels are taken for vascular grafts. Anesthesia support of the organ donor is necessary until the proximal aorta is surgically occluded and in situ flushing of organs has begun. Subsequently, the ventilator should be disconnected and monitoring discontinued, as electrocardiographic (ECG) activity may persist for up to 70 minutes after cardiac arrest (Oaknine, 1975; Logigian and Ropper, 1985).
Because brain death results in the loss of central mechanisms that control the endocrine and autonomic nervous systems, cardiac death usually occurs within 48 to 72 hours despite maximal physiologic support. Up to 25% of potential brain-dead organ donors are lost each year in North America because of cardiovascular collapse (Jenkins et al., 1999). Hypotension and hemodynamic instability secondary to neurogenic shock, dysrhythmias, and hypovolemia, all resulting from the absence of brainstem function, should be anticipated and treated in all donors.
Intravascular Fluid Management
Brainstem injury produces a sequence of hemodynamic events, beginning with an increase in parasympathetic tone and evolving to a massive autonomic surge with hypertension (systolic blood pressure > 200 mm Hg) and tachycardia (>140 beats/min) (Audibert et al., 2006). The pathophysiology of neurocardiogenic injury involves an initial neural phase (catecholamine surge and mixed venous oxygen saturation [MVo2] supply-demand imbalance) within hours, followed by a humoral phase and release of inflammatory cytokines. Depletion of thyroid hormones and cortisol, and decreased coronary perfusion pressure may also impair myocardial function. Coronary vasoconstriction, subendocardial ischemia, focal myocardial necrosis, and endothelial injury are factors that contribute to accelerated cardiac allograft vasculopathy in the recipient (Segel et al., 2002; Szabo et al., 2002; Mehra et al., 2004). Systolic and diastolic dysfunction has been reported in 10% to 28% of pediatric donors and 71% of adult donors. It is reversible in the majority of cases (Banki and Zaroff, 2003). Despite normal or increased perfusion pressure, the resulting vasoconstriction may cause tissue ischemia that disrupts the production of ATP, generates oxygen free radicals, increases cytosolic calcium concentration, and activates various enzymatic cascades such as endonucleases and nitric oxide syntheses (Kunzendorf et al., 2002). A subsequent hypotensive phase caused by loss of autonomic regulation of the peripheral vasculature and unopposed vasodilation may further reduce oxygen supply to the tissues. Hypovolemia is seen with diuresis and fluid restriction during brain resuscitation, inadequate volume replacement after trauma, and diuresis resulting from diabetes insipidus or hyperglycemia.
Brain death is a dynamic inflammatory process. The activation of inflammatory mediators leads to a nonspecific immune response, which may be associated with accelerated acute graft rejection and poor long-term outcomes (Kunzendorf et al., 2002). In addition, a significant factor in organ injury after storage and cold ischemia is caused by reperfusion, initiated by leukocyte adhesion to endothelial cells and the production of oxygen-derived free radicals and peroxides.
If the initial two-dimensional echocardiographic evaluation reveals that the left ventricular ejection fraction is less than 45%, management with invasive monitoring, fluids, inotropes, vasopressors, hormonal, replacement therapy (with hydrocortisone, levothyroxine, or triiodothyronine), and treatment of diabetes insipidus (with desmopressin [DDAVP] or vasopressin infusion) is strongly recommended (Table 28-8).
TABLE 28-8 Maintaining Mean Arterial Pressure in the Pediatric Organ Donor
Hemodynamically Stable | Hemodynamically Unstable |
From Nakagawa TA: Updated pediatric donor management and dosing guidelines. NATCO, Organization for Transplant Professionals, 2008. Available at www.natco1.org/prof_development/index.htm#guidelines; accessed September 2008.
Hourly maintenance fluids are calculated according to the 4-2-1 rule; that is, for the first 10 kg, use 4 mL/kg; for the second 10 kg, use 2 mL/kg; when the weight is greater than 20 kg, use the weight in kilograms plus 40. Guidelines for transfusion therapy are listed in Table 28-9. Packed red blood cells (RBCs) are transfused to maintain the hemoglobin at 10 g/dL for unstable donors, with the lowest acceptable being 70 g/L. Platelets, International Normalized Ratio (INR), and partial thromboplastin time (PTT) have no predefined targets, with transfusion indicated in cases of clinically evident bleeding or coagulopathy. The recommended hemodynamic endpoints are mean arterial pressure (MAP), 60 mm Hg or greater; CVP and pulmonary capillary wedge pressure (PCWP), 12 mm Hg or less; systemic vascular resistance (SVR), 800 to 1200; cardiac index, 2.5 or greater; left ventricular stroke work index, 15 or greater; and dopamine dosage, 12 mcg/kg per minute or less (UNOS Critical Pathway for the Pediatric Organ Donor).
TABLE 28-9 Transfusion Therapy for the Organ Donor
Component | Quantity (mL/kg) | Administration |
Packed red blood cells | 10-15 | Administer over 2-3 hours. May be administered faster if hypotension or bleeding requires more aggressive correction of anemia. |
Fresh frozen plasma | 10-15 | Administer over 1-2 hours. May be administered faster if correction of coagulopathy is associated with volume depletion or hypotension. |
Cryoprecipitate | 5-10 or 1 unit for every 10 kg of body weight | Administer for hypofibrinogenemia. |
Platelets | <15 kg: 10-20 >15 kg: single unit of platelets | Administer slowly over 2-3 hours. |
From Nakagawa TA: Updated pediatric donor management and dosing guidelines. NATCO, Organization for Transplant Professionals, 2008. Available at www.natco1.org/prof_development/index.htm#guidelines; accessed September 2008.
Cardiovascular Management
Hypotension in the potential organ donor most commonly results from hypovolemia (absolute or effective with a reduction in venous return), cardiac dysfunction, or arterial vasodilation from neurogenic shock (Table 28-10). The goals of hemodynamic support are to correct hypovolemia, to adjust vasoconstrictors and vasodilators to maintain a normal afterload, and to optimize cardiac output and maintain perfusion pressure gradients without relying on high dosages of β-agonists or other inotropes. Proposed adverse biochemical, histopathologic, and functional effects of catecholamine infusions on the myocardium include high-energy substrate depletion, intramyocardial noradrenaline depletion, and myocardial β-adrenoceptor down-regulation (Sakagoshi et al., 1992; Pinelli et al., 1995; Zaroff et al., 2002). With persistent hypotension despite volume replacement, vasopressor therapy should be initiated.
TABLE 28-10 Causes of Hypotension in the Potential Organ Donor
Hypovolemia | Cardiac Dysfunction | Vasodilation |
Initial injury Inadequate resuscitation Third spacing Decreased intravascular oncotic pressure after crystalloid resuscitation Dehydration from treatment of intracranial pressure Fluid restriction Urea Diuretics Mannitol Hyperglycemia-induced osmotic dieresis Diabetes insipidus Hypothermic “cold” diuresis Venodilation Loss of vasomotor tone and pooling in venous capacitance bed Rewarming of hypothermia |
Preexisting disease Initial injury Myocardial contusion Pericardial tamponade Myocardial ischemia/infarct Brain death process Catecholamine damage Ischemia-reperfusion injury Metabolic depression Acidosis Hypothermia Hypophosphatemia Hypocalcemia Hypoxia Endocrinopathy of brain death Volume overload congestive heart failure Arrhythmias Catecholamines Ischemia Hypokalemia Hypomagnesemia |
Spinal shock Catecholamine depletion Loss of vasomotor control and autoregulation “Relative” adrenal insufficiency of trauma or critical illness Endocrinopathy of brain death Acquired sepsis |
From Jalili M: Organ donor management: intensivist’s role. Pediatric Summit Powerpoint presentation, Los Angeles, March 2008. Available at www.onelegacy.org.
Dopamine up to 10 mcg/kg per minute is the drug of choice because the glomerular filtration rate (GFR) is increased as well as cardiac output while dilating the renal, mesenteric, and coronary vasculature. Inotropic agents should be titrated to maintain a normal blood pressure for the patient’s age (normal systolic blood pressure mm Hg = 80 + 2 × age in years). Blood pressure alone does not indicate adequate tissue perfusion. Therefore, serum biomarkers such as base deficit and lactate should be followed as inotropic support is titrated (Table 28-11).
TABLE 28-11 Inotropic Infusions
Drug | Dosage |
Milrinone (Primacor) | 0.25-0.75 mcg/kg per min IV Loading dose: 50 mcg/kg |
Dopamine | 2-20 mcg/kg per min IV |
Dobutamine (Dobutrex) | 2-20 mcg/kg per min IV |
Epinephrine | 0.1-1 mcg/kg per min IV |
Norepinephrine (Levophed) | 0.05-2 mcg/kg per min IV |
Phenylephrine (Neosynephrine) | 0.1-0.5 mcg/kg per min IV Bolus: 5-20 mcg/kg |
Vasopressin (Pitressin) | 0.3-2 mU/kg per min IV Note: Dosage is different for treatment of patients with diabetes insipidus |
From Nakagawa TA: Updated pediatric donor management and dosing guidelines. NATCO, Organization for Transplant Professionals, 2008. Available at www.natco1.org/prof_development/index.htm#guidelines; accessed September 2008.
Echocardiography should be performed early, followed by insertion of a pulmonary artery catheter, especially if the ejection fraction is less than 40% or the donor requires high-dosage inotropes. According to Zaroff (2004), ejection fraction is the most significant predictor of nonuse of potential donor hearts, with an odds ratio of 1.48 per 5% decrease in ejection fraction.
When dopamine is used for inotropic support in infants, dosages significantly higher than those needed in the adult may be required, presumably because of catecholamine receptor immaturity or deficiency (Kelly et al., 1984). At these higher dosages (15 mcg/kg per minute), no adverse effects on GFR or urine output are apparent (Outwater and Rockoff, 1984). Dopamine at α-agonist dosages has not been shown to influence outcomes in liver, kidney, or heart in pediatric donors (Finfer et al., 1996). There is evidence that dopamine may reduce allograft rejection, not only through support of blood pressure but possibly also through more complex immunomodulatory effects that inhibit expression of adhesion molecules, which are required for leukocyte migration into the graft to produce acute rejection (Carlos et al., 1997; Schnuelle et al., 1999).
An infusion of an α-adrenoceptor vasoconstrictor, epinephrine or phenylephrine, may be useful to increase peripheral vascular tone, but they have the inherent risk of causing marked peripheral vasoconstriction and/or an increase in pulmonary artery pressure (PAP) or oxygen consumption. Jhanji and colleagues (2009) have shown norepinephrine to be protective of global oxygen consumption, without affecting the splanchnic circulation or producing significant deleterious renal effects. An infusion of vasopressin starting at 0.3 mU/kg per minute and titrated to the desired blood pressure, with a maximal infusion rate of 2 mU/kg per minute, may be efficacious in the setting of hypotension and low SVR, especially in combination with an inotropic infusion such as epinephrine. Vasopressin is not recommended as first-line therapy, as there are limited data available in children. Recent studies, however, suggest it is most efficacious when coupled with corticosteroids (Büchele et al., 2009). Dobutamine (IV, at 2 to 20 mcg/kg per minute) should be used when cardiac output is decreased because of reduced myocardial contractility and pulmonary hypertension. ECG abnormalities are common during the brain-death process, manifested as marked ST-T wave abnormalities, ischemic changes, inverted T waves, widened QRS complexes, and prolonged QT interval. In addition, atrial and ventricular arrhythmias and varying degrees of conduction abnormalities may occur. These arrhythmias usually result from autonomic instability (catecholamine storm and loss of the vagal motor nucleus) compounded by oxygenation, acid-base, temperature, and electrolyte (e.g., low magnesium and potassium) disturbances or increased intracranial pressure (ICP) (Cushing’s reflex) (Powner and Allison, 2006). The difficulty lies in differentiating these transient findings from those of catecholamine-induced myocardial injury or irreversible ####ischemia, which can produce global myocardial dysfunction. Bradycardia is not a problem unless it contributes to hypotension. It may be treated with any inotropic agent or temporary transthoracic or venous pacing.
Eventually, the heart stops. Despite all therapeutic efforts, the arrhythmias encountered are usually resistant to therapy (Table 28-12). Bradyarrhythmias leading to asystole rather than ventricular fibrillation (as seen in adults) are the terminal cardiac rhythms in pediatric patients. The propensity for this particular dysrhythmia may be related to the immature autonomic nervous system and small muscle mass in the pediatric donor (Walsh and Krongrad, 1983). In the event of a sudden cardiac arrest during the procurement procedure, cardiopulmonary resuscitation should be started to facilitate organ perfusion, and liver, pancreas, intestines, and kidney procurement should proceed rapidly with cross-clamping of the aorta at the diaphragm and infusion of cold preservation solution into the distal aorta and portal vein.
Pulmonary Management
All potential organ donors require mechanical ventilation, usually for a period of several days. Progressive pulmonary dysfunction may result from pulmonary contusions resulting from trauma, aspiration pneumonitis, fat emboli, and pulmonary edema (cardiogenic and neurogenic). Any of these may result in hypoxia, placing all perfuseable organs at risk for ischemia. In addition, infectious complications, oxygen toxicity, barotrauma or volutrauma, pulmonary embolism, and atelectasis may contribute to an increase in the alveolar-arterial oxygen gradient. High-dosage methylprednisolone administration has been shown to significantly improve oxygenation and increase donor lung recovery (Follette et al., 1998). The beneficial effects of steroids probably result from attenuation of the effects of proinflammatory cytokines released as a consequence of brain death (Glasser et al., 2001). Interleukin (IL)-8 expression and neutrophil infiltration of the donor lungs may result in impairment of graft oxygenation, development of severe early graft dysfunction, and early recipient mortality (Fisher et al., 2001). As the total lung water increases from a combination of pulmonary capillary leakage and disruption of the Starling forces in the lungs, pulmonary compliance decreases, with resultant impedance of alveolar gas exchange. Neurogenic pulmonary edema is best managed with positive end-expiratory pressure (PEEP), diuresis, and small volume fluid resuscitation. Lung-protective strategies include maintenance of an arterial saturation of 95% or a Pao2 greater than 100 mm Hg, with the lowest possible Fio2 setting preferably being no higher than 40%, and with Pao2/Fio2 greater than 300. Limiting high distention pressures in the lung during volume-controlled modes of ventilation using tidal volumes of 6 to 8 mL/kg, low peak inspiratory pressure (28 to 30 cm H2O), and avoiding PEEP in excess of 5 cm H2O is desirable. Paco2 should be normalized to 35 to 45 mm Hg, and serum pH to 7.35 to 7.45. The maintenance of a mild to moderate alkalemia has been reported by some to reduce the likelihood of ventricular fibrillation (programmed ventilator sigh) (Becker et al., 1981). Pulmonary hygiene, bronchodilators, and lung-expansion techniques every hour or two may help in preventing atelectasis and improve the quality of donor lungs. Recruitment maneuvers for oxygenation impairment include periodic increases in PEEP (up to 15 cm H2O) and sustained inflations (peak inspiratory pressure at 30 cm H2O for 30 to 60 seconds).
Endocrine Management
Once the diagnosis is confirmed, therapeutic intervention should consist of pharmacologic management to decrease urine output to 3 mL/kg per hour or less. Replacement of urine output with 0.2% one-fourth or 0.45% normal saline should be used in conjunction with desmopressin (intermittent IV DDAVP, 0.25 to 1 mcg every 6 hours) or vasopressin (0.5 to 0.7 mU/kg per minute IV to a maximum dosage of 2.4 U/hour) infusion to maintain serum sodium levels between 130 and 150 mEq/L. DDAVP is an analogue of arginine vasopressin, which is highly selective for the vasopressin V2 receptor subtype found in the renal collecting duct, and without the vasopressor activity in humans that is mediated by V1 receptors on vascular smooth muscle. DDAVP has a long half-life of 75 to 120 minutes, so an IV infusion may be discontinued 2 to 3 hours before organ recovery. Several mechanisms regulate the release of arginine vasopressin (AVP). Hypovolemia resulting from hemorrhage or any other cause results in a decrease in atrial pressure. Specialized stretch receptors in the atrial walls and large veins (cardiopulmonary baroreceptors) entering the atria decrease their firing rate when there is a fall in atrial pressure. Afferent nerve fibers from these receptors synapse in the nucleus tractus solitarii of the medulla, which sends fibers to the hypothalamus, a region of the brain that controls AVP release by the pituitary gland. Atrial receptor firing normally inhibits the release of AVP by the posterior pituitary. With hypovolemia or decreased AVP, the decreased firing of atrial stretch receptors leads to an increase in AVP release. Hypothalamic osmoreceptors sense extracellular osmolarity and stimulate AVP release when osmolarity rises, as occurs with dehydration. Finally, angiotensin II receptors located in a region of the hypothalamus regulate AVP. Therapy should always be guided by serum electrolyte and osmolality measurements made every 2 to 4 hours. Several investigators have demonstrated prolonged hemodynamic stability in donors, with the addition of an infusion of AVP to existing pressor support. Donors without clinically apparent DI may demonstrate a baroreflex-mediated defect of vasopressin secretion and pressor hypersensitivity to exogenous hormones. In these patients, low-dosage vasopressin significantly increases blood pressure (Chen et al., 1999). Absence of AVP may be a predominant contributing factor to the eventual cardiac arrest in all brain-dead patients.
Endocrine and Metabolic Functions
Despite disruption of the hypothalamic-pituitary-adrenal axis, hormone production from the anterior lobe of the pituitary gland persists in most brain-dead patients. The prevalence of anterior pituitary dysfunction is estimated at 30% (Schneider et al., 2005), with evidence of at least one abnormal hormonal function in 53% (Dimopoulou et al., 2004). More recently, Nicolas-Robin and colleagues (2010) noted in 31 brain-dead donors older than 18 years that the incidence of adrenal insufficiency was 87% and that the administration of 50 mg of hydrocortisone enhanced cardiovascular stability and decreased the requirement for norepinephrine. The incidences of hormonal reduction are estimated as follows: adrenal, 15%; thyroid, 5% to 15%; growth hormone, 18%; gonadal, 25% to 80%; and vasopressin, 3% to 37%. Endocrine failure is associated with basilar skull fracture, hypothalamic edema, prolonged unresponsiveness, hyponatremia, and hypotension (Powner and Allison, 2006).
Rapid depletion of vasopressin, cortisol, insulin, thyroxine (T4) and free triiodothyronine (T3) occurs in experimental animal models of brain death (Novitzky et al., 1984, 2006), but endocrine dysfunction in humans is usually manifested solely as DI (Wijdicks, 2001). Novitzky and others (1987) claim that true hypothyroidism exists, as evidenced by a decrease in free T3. They have shown that hormonal replacement therapy (T3, cortisol, and insulin with glucose) improves cardiac output and reduces the need for pressor support. Brain death is also associated with global mitochondrial dysfunction with a change to anaerobic metabolism and lactic acidosis. It leads to depletion of myocardial high-energy phosphates and cellular dysfunction. Thus, many organ procurement groups administer thyroid hormones to hemodynamically “rescue” unstable donors who show evidence of anaerobic metabolism or profound hypotension refractory to volume resuscitation and therapy with multiple vasopressors. In a landmark paper published in 1995, Wheeldon and colleagues (1995) showed that by using invasive hemodynamic monitoring and a standard donation management protocol (steroids, insulin, vasopressin, and T3), the Papworth Hospital Group in Cambridge, United Kingdom, was able to functionally resuscitate 92% of initially unacceptable donors into acceptable donors. Hormonal replacement therapy (methylprednisolone, T4) has been shown to decrease the need for inotropic support and improve metabolic stability in critically ill children with cessation of neurologic function and hemodynamic instability (Powner, 2001; Salim et al., 2001; Zuppa et al., 2004). Rosendale and colleagues (2003) compared non–hormone replacement with a three–hormone replacement protocol (methylprednisolone bolus and infusions of vasopressin and T3 or T4) in brain-dead donors, with 30-day mortality and early graft dysfunction used as measures of outcome. Multivariate results demonstrated a 46% reduced odds of death within 30 days and a 48% reduced odds of early graft dysfunction in the three–hormone replacement donor group.
An infusion of nitroglycerine or nitroprusside is occasionally recommended to prevent myocardial ischemia and the potential untoward renal effects of diminished renal perfusion with the use of vasopressin. Vasopressin supplementation (2 to 10 mcg/kg per minute continuous infusion) in a porcine model of brain-dead potential organ donors resulted in physiologic levels of the hormone, with normal plasma osmolarity and serum sodium levels and decreased urine output, potassium needs, and fluid requirements, without effects on peripheral vascular resistance and without microscopic evidence of organ ischemia (Blaine et al., 1984).
North American Transplant Coordinators Organization (Nakagawa, 2008a) currently recommends that hormonal replacement therapy (T4 or T3, steroids, insulin) be considered early in the course of pediatric donation management to improve donor heart function. Levothyroxine (Synthroid) is administered as a bolus dosage of 1 to 5 mcg/kg followed by an IV infusion of 0.8 to 1.4 mcg/kg per hour, with infants and smaller children requiring a larger bolus and infusion dosage. IV T4 has the disadvantage of a slower and unpredictable onset of action. Alternatively, T3 may be replaced as an infusion 0.05 to 0.2 mcg/kg per hour IV. Although T3 may rescue the donor heart, it may have detrimental effects, including tachycardia, arrhythmia, metabolic acidosis, and profound hypotension. Methylprednisolone (Solu-Medrol), 20 to 30 mg/kg IV bolus, is administered and may be repeated in 8 to 12 hours. An IV insulin infusion (0.05 to 0.1 units/kg per hour) is titrated to control blood glucose levels to 60 to 150 mg/dL, with monitoring for hypoglycemia (Table 28-13).
Electrolyte disturbances are routine, most being iatrogenic, resulting from treatment of the original injury or in response to physiologic perturbations during the brain-death process. Hypernatremia is inevitable as a result of DI, and a variety of strategies are used to treat elevated ICP. The liver is exquisitely sensitive to hypernatremia (>155 mEq/L) with increased levels correlating with primary graft loss after transplantation (Figueras et al., 1996). Serum potassium, magnesium, calcium, and phosphate levels are often depleted and require prompt replacement therapy. Hyperglycemia may be caused by glucose intolerance (steroids) or administration of large amounts of a dextrose-containing solution for fluid resuscitation.
Hypothermia
Although hyperthermia is common in the early phase of the sympathetic storm, the donor rapidly becomes hypothermic as a result of vasodilation and the loss of thermal regulation by the CNS. The patient becomes poikilothermic, with the body temperature determined by the environmental temperature. Although a mild degree of hypothermia may be beneficial to organ protection and preservation, the undesirable consequences of hypothermia, which are clinically significant, include diuresis and hypovolemia, hyperglycemia, coagulopathy, arrhythmias, myocardial depression, pulmonary hypertension, and eventually cardiac arrest (Reuler, 1978). Hypothermia further complicates the process of certification of brain death by causing the pupils to appear fixed and dilated. Active rewarming measures to maintain a core body temperature 36° to 38° C should be initiated early.
Organ Preservation
The field of organ preservation includes in situ core cooling, organ preservation fluids, and machine perfusion techniques. Traditionally, optimal organ preservation has been achieved by the combination of maximizing donor hemodynamics, using improved surgical procurement techniques with minimal dissection of vascular structures, cannulating the abdominal aorta for rapid in situ core cooling, removing abdominal organs en bloc with separation of graft components on the back table, employing cold storage techniques with a hyperkalemic hyperosmolar solution at a temperature of 4° C, and treating the donor or recipient (or both) pharmacologically. Most centers use static cold storage to preserve organs, and this is useful for younger donors with good-quality organs (Southard and Belzer, 1995; Marshall, 1997). With the introduction of extended donor criteria, the limitations of cold storage have probably been reached. Kidneys can be preserved for up to 72 hours but seldom do well in the recipient after 36 to 48 hours with cold ischemia. The heart and lungs should be transplanted within 4 to 6 hours, and the pancreas preferably within 6 to 12 hours.
The preservation solution introduced by the University of Wisconsin (UW solution) in 1987 allowed extension of the safe preservation time of the donor livers from 8 to 24 hours (Jamieson et al., 1988) and kidneys for up to 72 hours. Iced storage and core cooling result in a slowing of cellular metabolism and energy consumption; glucose or hydrogen ion buffers prevent cellular acidosis; donor heparinization (30,000 U, or 300 U/kg) prevents microvascular thrombosis and promotes a more even organ flushing and reperfusion. Effective impermeants are saccharides and nonsaccharide anions. Molecular weight determines the effectiveness of saccharides to prevent cell swelling, with larger saccharides being more effective (Sumimoto et al., 1990; Hart et al., 2002). The UW solution, histidine-tryptophan-ketoglutarate (HTK), and Celsior are the primary solutions used currently for organ preservation (Table 28-14).
HTK, originally introduced for cardioplegia in 1980, has gained popularity in clinical transplantation for cold perfusion and organ storage since the U.S. Food and Drug Administration (FDA) approved its use for kidney and liver preservation (Lam et al., 1989). Early experiences with HTK have demonstrated that it compares favorably with the UW solution in kidney and liver organ preservation. Growing clinical experience with living donor liver transplantation has shown comparable clinical performances of UW solution and HTK (Lange et al., 1997; Testa et al., 2003). UW solution and HTK also show clinical equivalence for deceased donor liver transplants, although UW solution remains the gold standard for longer preservation times (Guarrera and Karim, 2008).
In the early 1970s, hypothermic machine perfusion (HMP) was used by many centers in the United States and Europe to preserve kidneys, allowing transportation to distant transplantation centers. Although modern HMP systems are smaller, lighter, and more sophisticated than the original machine used by Belzer and coworkers, the principles of HMP have not changed. Machine perfusion generates a controlled continuous or pulsatile recirculating flow of preservation solution at 0° to 4° C. This continuous flow allows complete perfusion of the organ, promoting a thorough washout of blood and subsequent tissue equilibration with the preservation solution. Beneficial effects of machine perfusion are a low incidence of delayed graft function, the possibility of real-time organ viability assessment, the ability to provide metabolic support during perfusion, and the potential to add pharmacologic agents to the perfusate. A meta-analysis reported by Wight and coworkers noted that HMP produced a 20% reduction in delayed graft function compared with cold storage (Guarrera and Karim, 2008). Most experience with HMP involves the kidneys (Henry, 1997), and clinical application of HMP in human liver transplantation has been limited to the recent pioneering work of Guarrera and colleagues (2004).
Living related organ donor
Over the past several years, a consistently widening gap has become evident between the demand for and supply of transplantable organs. At the end of 2009, there were 1907 pediatric transplant candidates on the waiting lists for various organs, out of a total of 113,702 candidates of all ages awaiting all organs. Of the pediatric candidates, approximately 50% were from 11 to 17 years old. This reflects a decline in the past 10 years and a decrease in the size of the liver and lung waiting lists, with the majority of children wait-listed for liver and kidney organs. The number of pediatric deceased donors over the past decade has steadily decreased (OPTN/UNOS, January 1988 to June 2010). Compared with adult deceased donors, pediatric deceased donors are more likely to donate a specific organ, as evident in data reported up to 2010: kidneys (92%), livers (87%), lungs (16%), heart (50%), pancreas (40%), and intestine (9%) (OPTN/UNOS, December 2009). With this serious shortage of donor organs of appropriate size and suitability for infants and small children, the waiting times for some organs has increased dramatically. For example, with only 20% of lungs from deceased donors meeting stringent donor criteria, the waiting period varies from 42% of pediatric patients waiting less than 1 year, to 15% listed for 5 or more years. This chronic shortage has prompted the development of programs for living related organ donation. The original concept was developed for renal transplantation in the early 1950s, and, currently in the United States, 25.2% of pediatric transplants are from living donors (OPTN/UNOS, 2009) (Table 28-15). Justification was relatively easy, as healthy relatives could “safely” donate one of their two kidneys without jeopardizing renal function in their remaining solitary kidney, and the results from living related pediatric renal transplantation have, until recently, been superior to deceased donor transplantation, at all ages. Later, techniques were developed to use one or more left-lateral segments of a parent’s liver, which would be suitable for a small child with chronic liver disease (Broelsch et al., 1991; Lang et al., 2004). Lessons learned from split or reduced-size deceased adult livers for implantation into children were used in the development of living related liver transplantation programs, which can accommodate large children and small adults using the right lobe of the liver. From 1987 to 2009 in the United States, 1264 of the total 3967 pediatric liver transplants were from living donors (see Table 28-15). With similar methods, living donor transplantation has been successfully developed for pancreas, lung (unilateral and bilateral lower lobes), and small bowel transplantation.
Living-related organ donation has, for the most part, been performed from parent to child. The use of children for living kidney donation remains highly controversial, and in general, most transplant programs do not use a donor younger than 18 years except in very limited circumstances, such as identical twins or an emancipated minor for his or her own child (Abecassis et al., 2000). Living-related donor procedures have several advantages, including the fact that they can be electively scheduled when the recipient is in optimal condition. However, with the exception of living related renal transplantation (which has excellent outcomes, with 1-year graft survival in children older than 1 year ranging from 94% to 96%), the long-term functional outcome of living-donor lung, pancreas, and small intestine transplants remains to be established because of the relatively small number of transplants performed. Currently, the risk for mortality and graft loss is less for children younger than 2 years if they receive a liver allograft from a living donor compared with a deceased donor (Reding et al., 2003). In addition, many troubling ethical issues arise, such as the risk to the donor and recipient, the validity of informed consent, and concerns about donor privacy and confidentiality. Uncomplicated unilateral nephrectomy has an exceedingly low mortality (less than 0.1%), but the same is not true for right lobe liver donors (0.2% to 1%, or 4 to 10 of 2000 cases), left segment/lobe liver donors (0.06% to 0.2%, or 2 to 6 of 3300), and right lobectomy of the lung, less than 5% mortality (Hayashi and Trotter, 2002; Trotter et al., 2002). Additionally, Hashikura and colleagues (2009) reported an 8.4% complication rate in 299 donors undergoing living related liver donation. Postoperative donor complications included biliary complications (3.0%), reoperation (1.3%), severe aftereffects in two cases (0.06%), and death (apparently related to donor surgery) in one donor (0.03%). The incidences of postoperative complications in left- and right-lobe donors were 8.7% and 9.4%, respectively.
In addition, there have been increasing interest and use of DCD, with the hope of expanding this pool from 200 to 1000 donors per year in the United States. Although pediatric donors constitute approximately 20% of the total DCD donor pool, very few of the kidneys recovered are allocated to pediatric recipients (OPTN/UNOS, 2009). This practice may reflect concerns about long-term graft function, given the limited outcomes data available.
Immunosuppression
Immunosuppression Medications
Corticosteroids
Corticosteroids were the initial mainstay of therapy for organ transplantation, but their use is on the decline. They are particularly effective in both the prevention and treatment of acute rejection episodes. Their predominant mechanisms of action include the inhibition of IL-1 and IL-2 production, suppression of helper and suppressor T cells, suppression of cytotoxic T cells, and reduction in the migration and activity of neutrophils (Cohen, 2002). The long-term use of corticosteroids has been questioned because of their adverse side effects, particularly in the pediatric population. The trend during the past decade and a half is to use fewer steroids for maintenance therapy (Margarit et al., 1989; Ascher, 1995). Many centers do not now use steroids as part of their long-term regimens. The first report of steroid withdrawal after transplantation was published in 1989 (Margarit et al., 1989). Everson and associates (1999) published a literature review, which demonstrated that greater than 50% to 85% of patients can be withdrawn from steroids without changes in acute rejection episodes, patient survival, or graft survival rates (Stegall et al., 1997). This percentile difference depends on the antirejection agent used for maintenance therapy (e.g., tacrolimus versus cyclosporine or serolimus). Steroid withdrawal is preferable, as it is associated with significantly less hypertension, hypercholesterolemia, and diabetes mellitus, and, moreover, it is less likely to result in cushingoid features and delayed development and obesity in the pediatric patient. Despite controversy over their use in maintenance therapy, corticosteroids remain the first-line agents for the treatment of acute rejection. The response rates vary depending on the organ system and whether the patient is maintained on cyclosporine or tacrolimus (U.S. Multicenter FK506 Liver Study Group, 1994).
Azathioprine
Azathioprine, the imidazole derivative of 6-mercaptopurine, was introduced in the late 1950s and early 1960s. Its mechanism of action is the inhibition of differentiation and proliferation of T and B lymphocytes by blocking DNA and RNA synthesis. The effect is a reduction in the numbers of circulating white cells, both lymphocytes and granulocytes. One notable advantage of this agent is its steroid-sparing effect, allowing lower dosages of steroids to be used with equal efficacy and fewer side effects. The use of this agent is limited, however, by side effects, particularly hematologic and gastrointestinal (GI). Its use may result in as many as 50% of patients exhibiting bone marrow suppression and a profound leukopenia or thrombocytopenia (Cattral et al., 2000). Gastrointestinal side effects include pancreatitis, nausea, vomiting, and hepatotoxicity. Patients are at increased risk for opportunistic infections. An increased risk for malignancy, especially lymphoma, however, remains controversial. The primary pathway of metabolism is inactivation by xanthine oxidase, an enzyme blocked by allopurinol. The recommended dosage of azathioprine is 1 to 2 mg/kg per day. Plasma levels are not monitored for this agent.
Mycophenolate Mofetil
Mycophenolate mofetil (MMF) (CellCept) is a morpholinoethyl ester of mycophenolate. It is well absorbed orally and hydrolyzed to its active form, mycophenolic acid, which acts by competitively inhibiting inosine monophosphate dehydrogenase and hence blocks de novo synthesis of purines, primarily guanine. Lymphocytes, unlike other rapidly replicating cells, depend entirely on this pathway for purine synthesis. Hence, the primary mechanism of action of MMF is the selective inhibition of lymphocyte proliferation. This drug is one of the newer agents introduced in the past 10 years and has been shown to be more efficacious than azathioprine in combination therapy (Fisher et al., 1998). There is also some experimental evidence to suggest that MMF may reduce the risk for chronic allograft rejection, which has been attributed to its antiproliferative activity against B lymphocytes and arterial smooth-muscle cells (Azuma et al., 1995; Schmid et al., 1995). The most common side effects are GI, including nausea, abdominal pain, anorexia, gastritis, and diarrhea. Diarrhea affects as many as 30% of patients but usually responds to a decrease in dosage. MMF also causes leukopenia and thus should be avoided in combination with azathioprine. The usual dosage is 1 to 3 g/day orally in two divided doses. Like azathioprine, blood levels are not monitored with this agent. The antivirals acyclovir and ganciclovir increase MMF levels, and antacid decrease the absorption of MMF (Cohen, 2002).
Calcineurin Inhibitors
Cyclosporine
Cyclosporine is a neutral lipophilic cyclic endecapeptide extracted from the fungus Tolypocladium inflatum in the early 1970s. Cyclosporine is poorly absorbed from the GI tract, and there is considerable variation in its bioavailability, as biliary output is essential for its absorption. Neoral (Novartis Pharmaceutical Corp., East Hanover, NJ), the microemulsion formula of cyclosporine, is much less dependent on bile production and flow than Sandimmune (Novartis Pharmaceuticals Corp.), the standard preparation. Neoral gives a more consistent level of cyclosporine and is associated with less evidence of rejection (Graziadei et al., 1997; Pinson et al., 1998), so it has replaced Sandimmune in most major transplant centers worldwide.
Cyclosporine is metabolized by the cytochrome P450 3A system (Cantarovich et al., 1998). Agents that induce these enzymes increase the metabolism of cyclosporine, resulting in lower blood levels, whereas agents that inhibit P450 activity result in higher circulating cyclosporine levels. In addition, cyclosporine can interfere with the metabolism of other medications. Digoxin, lovastatin, and prednisolone can have significantly decreased clearance with resultant toxicity. The side effects associated with cyclosporine are numerous. Nephrotoxicity is one of the most significant, and it may involve acute or chronic renal pathologic alterations. The acute nephrotoxicity, which often resolves on reducing or discontinuing the medication, probably results from afferent arteriolar vasoconstriction with resulting decreased GFR. The chronic nephrotoxicity, which does not appear to be reversible, is associated with arteriolar hyalinosis, tubular vacuolization, interstitial nephritis, and cortical atrophy. Neurotoxicity is another common side effect, seen in as many as 50% of patients. This can range from headaches and tremors to seizures and coma. Because of variations in absorption and metabolism, cyclosporine levels tend to fluctuate. Dosages should be determined on the basis of the formulation used (i.e., Neoral versus Sandimmune) and serum or blood levels. Techniques to measure cyclosporine levels include radioimmunoassay and high-pressure liquid chromatography. The levels can be measured in whole blood, serum, or plasma. Most centers use whole blood trough levels by the radioimmunoassay technique. A level of 100 to 200 ng/mL is generally desirable in the first 3 to 6 months after transplantation, depending on the organ transplanted. The level can usually be maintained at lower levels thereafter, depending on the organ transplanted.
Tacrolimus
The calcineurin inhibitor tacrolimus, initially researched and brought to the market under the name FK-506, is a novel immunosuppressant that was isolated from Streptomyces tsukubaensis in 1985 (Kino et al., 1987; Starzl et al., 1989). Tacrolimus (FK506, Prograf) shares many similarities with cyclosporine, including its basic mechanism of action and metabolism by cytochrome P450 3A, in addition to its side-effect profiles. However, significant differences between these drugs have been elucidated in the past decade. Tacrolimus is 10 to 100 times more potent than cyclosporine, it possesses hepatotrophic properties, and it does not rely on bile for its absorption. Renal impairment, neurotoxicity, and hypertension occur with similar frequencies in both cyclosporine- and tacrolimus-based regimens. Tacrolimus is associated with more hyperglycemia. Reportedly, as many as 20% of tacrolimus recipients become insulin-dependent diabetics because of the agent’s direct effect on pancreatic beta cells and peripheral insulin receptors. Tacrolimus appears to have a higher incidence of posttransplant lymphoproliferative disease. Considering that accelerated atherosclerosis is an important issue after solid organ transplantation, it is important to note that tacrolimus produces less hyperlipidemia and thus a less adverse cardiovascular risk profile than cyclosporine. Statistically significant improvements have been seen in total cholesterol, low-density lipoprotein cholesterol, and triglyceride levels when patients were switched from cyclosporine to tacrolimus (Manzarbeitia et al., 2001). Tacrolimus levels are usually measured in whole blood specimens by enzyme immunoassay techniques. Drug levels of 5 to 15 ng/mL are desirable in the first few months after transplantation, depending on the organ system transplanted.
Efficacy of Cyclosporine and Tacrolimus
After its introduction, cyclosporine became the drug of choice for the prevention of allograft rejection. Tacrolimus, initially reserved for use as rescue therapy in refractory rejection, has now become a first-line immunosuppressive agent in many centers worldwide. Large multicenter U.S. and European randomized clinical trials have been performed to compare tacrolimus and cyclosporine (Sandimmune) for primary and maintenance immunosuppression (European FK506 Multicenter Liver Study Group, 1994; U.S. Multicenter FK506 Liver Study Group, 1994; Wiesner, 1998). In the European trial, patient and graft survival rates were similar; however, significantly lower rates of acute rejection were seen in the tacrolimus group in some organ systems. More episodes of acute and steroid-resistant rejection were seen in the cyclosporine group. The tacrolimus group had a lower cumulative steroid exposure and fewer requirements for monoclonal antibody therapy (European FK506 Multicenter Liver Study Group). Patients on tacrolimus, however, had significantly more medication-related adverse effects. At the 5-year follow-up, tacrolimus showed less rejection but no overall improvement in patient or graft survival for all solid organ transplants. Many of these large trials used Sandimmune as the cyclosporine preparation, hence confounding some of the data to date. Neoral has been shown to be superior to Sandimmune (Freeman et al., 1995; Mirza et al., 1997). Tacrolimus appears to be superior to cyclosporine for the treatment of periodic rejection. These episodes of acute rejection, even steroid-resistant events, may resolve when patients are switched from cyclosporine- to tacrolimus-based therapy (Jonas et al., 1996; Millis et al., 1996a, 1996b, 1998; Doria et al., 2003; Mazariegos, 2010). Additionally, chronic rejection was responsive in more than half of patients converted from cyclosporine to tacrolimus for some solid organ transplants (Klintmalm et al., 1993). Hence, the choice of calcineurin inhibitor tends to be a matter of institutional preference. Many institutions are now using tacrolimus-based therapy, citing less rejection, less steroid use, reduced metabolic derangements (including reduced rates of hyperlipidemia), diminished neurologic complications, and less OKT3 needed for immunosuppression. However, many centers still advocate the use of cyclosporine (usually Neoral) based on equivalent patient or graft survival rates and a lower incidence of diabetes mellitus and posttransplant lymphoproliferative disease.
Rapamycin
Rapamycin (Sirolimus) is a macrolide antibiotic isolated from the fungus Streptomyces hygroscopicus. It effectively inhibits both B- and T-cell activity (Poon et al., 1996; Poston et al., 1999). Rapamycin is structurally similar to tacrolimus. It uses the same intracellular binding protein as tacrolimus but blocks B- and T-cell activation at a later stage than the calcineurin inhibitors. Rapamycin and cyclosporine appear to act synergistically to inhibit lymphocyte proliferation (Kimball et al., 1991). The oral bioavailability of rapamycin is variable. Like the calcineurin inhibitors, it is metabolized by the cytochrome P450 3A system. The efficacy of rapamycin in solid organ transplantation was initially shown in the renal transplant population (Groth et al., 1999; Kahan et al., 1999; McAlister et al., 2000). Small studies in liver transplant patients have shown rapamycin to be an effective agent when combined with a calcineurin inhibitor (Watson et al., 1999; Trotter et al., 2001). Most of these patients could be maintained on steroid-free regimens. Acute rejection episodes were significantly decreased compared with historical controls (30% versus 70%) (Kahan for the Rapamune U.S. Study Group 2000). A primary role of rapamycin is to facilitate a dosage reduction of calcineurin inhibitor in those patients with evidence of tacrolimus or cyclosporine toxicity (Brattstrom et al., 1998; Groth et al., 1999). The most frequent dosage-related adverse effects include hyperlipidemia, leukopenia, thrombocytopenia, oral ulcerations, and joint pains. Both cholesterol and triglyceride levels can significantly increase and should be monitored while on therapy. Calcineurin inhibitor levels, especially those of cyclosporine, have been shown to significantly increase while on rapamycin therapy and should be closely monitored.
Antilymphocyte and Antithymocyte Globulins
Antilymphocyte globulin (ALG) and antithymocyte globulin (ATG) are produced by extracting immunoglobulins from animals (usually horse or rabbit) that have been immunized with human lymphocytes or thymocytes, respectively. The IV administration of these polyclonal antibodies causes rapid and profound depletion of peripheral lymphocytes. A major limitation of all polyclonal antilymphocyte preparations is batch-to-batch heterogeneity, which results in unpredictable side effects and more importantly, variable efficacy. ALG and ATG are not typically used as first-line agents for immunosuppression. They appear to delay the onset of the first episodes of organ rejection, but the overall rates of rejection are similar to those seen with calcineurin inhibitors (Neuhaus et al., 2000). The focus of this form of therapy is as the primary immunosuppression in patients unable to tolerate calcineurin inhibitors (i.e., because of significant pretransplant renal insufficiency) and in the treatment of steroid-resistant (and possibly OKT3-resistant) rejection. Commonly observed side effects include allergic reactions, serum sickness, fever, and thrombocytopenia, as with OKT3 and other systemically infused immunoglobulins. Cytokine release syndrome (cardiovascular collapse with a hemodynamic profile similar to septic shock, noncardiogenic pulmonary edema, seizures, hyperpyrexia and renal insufficiency) may be seen with the administration of these agents, which is similar to that sometimes seen with OKT3 or any other immunoglobulin. The incidence of lymphoproliferative disease is also increased among patients who have received ALG and ATG.
OKT3
OKT3 (Orthoclone OKT3, muromonab-CD3) is a monoclonal antibody directed against the CD3 complex of the cell membrane of lymphocytes (Fung et al., 1987); it is an antibody specifically directed at the T3 antigen of human T cells—the cells that directly attack the transplanted organ—and it is unlike the polyclonal antibody preparations. IV infusion results in tremendous lymphocyte depletion. Induction with OKT3 has not shown any significant benefit over the calcineurin inhibitors (McDiarmid et al., 1991). Because of its toxicity and the availability of less toxic agents, OKT3 is generally reserved for patients with severe steroid-resistant rejection (Portela et al., 1995; Wall and Adams, 1995). OKT3, ALG, and ATG provide 60% to 90% graft salvage rates for acute rejection in various organ system transplants. The side-effect profile of OKT3 is similar to that of ALG and ATG, including the cytokine release syndrome, infectious complications, and malignancy potential. This protein, like ALG and ATG, should be administered to a patient who is monitered. Lymphocyte and platelet counts must be closely monitored. CMV infection should be anticipated in all patients treated with OKT3 if they have not received CMV prophylaxis.
Interleukin-2 Receptor Antagonists
As discussed earlier, IL-2 is the most noteworthy cytokine known to be involved in the rejection of transplanted solid organs. Although calcineurin inhibitors decrease the amount of IL-2 produced, competitive inhibition by IL-2 receptor antagonists prevents the protein itself from binding to the active lymphocytes. Unlike ALG, ATG, and OKT3, which target the entire lymphocyte population, IL-2 receptor antagonists specifically target the actively dividing cells by binding to the IL-2 receptor’s CD25 moiety, which is expressed only in active cells (Langrehr et al., 1998). The two available IL-2 receptor antagonists are basiliximab and daclizumab. Early studies indicated that these agents need to be combined with calcineurin inhibitors to be effective (Vincenti et al., 1998). These agents should probably be used for induction of immunosuppression (Eckhoff et al., 2000) in those patients at high risk for calcineurin inhibitor–related toxicity. Additionally, they appear to be well tolerated without significant adverse effects. Dosages and timing of administration of these agents vary, depending on the organ system to be transplanted. Moreover, it appears that IL-2 receptor antagonists are needed immediately at the time of the transplantation (immediately before or after reperfusion of the transplanted organ), with a second dose at approximately 4 days in the immediate immunosuppressive induction period.
Alemtuzumab (Campath-1H)
The use of alemtuzumab (Campath-1H) in organ transplantation goes back to 1986, when Hale and colleagues (1986) in Europe reported on the preliminary results of a pilot study in renal, liver, and pancreas transplant recipients. Alemtuzumab is a humanized immunoglobulin IgG1 monoclonal antibody directed against CD52, a cell-surface glycoprotein expressed on circulating T and B cells and to a lesser extent on natural killer cells, monocytes, and macrophages. It has been suggested that after binding to its target, alemtuzumab causes cell death through complement-mediated cell lysis and antibody-mediated cellular cytotoxicity (Hale et al., 1986; Nuckel et al., 2005; Weaver and Kirk, 2007). Alemtuzumab was approved by the FDA for the treatment of lymphoid malignancies in 1999. It has been used off-label in bone marrow transplantation to prevent graft-versus-host disease and to treat various autoimmune diseases such as rheumatoid arthritis, scleroderma, and multiple sclerosis. Because of its rapid and profound lymphocyte-depleting effects, alemtuzumab was initially used off-label as induction therapy in renal transplantation to allow safe avoidance or minimization of steroid or calcineurin-inhibitor therapy. Retrospective analysis of the OPTN/UNOS database revealed that alemtuzumab induction was associated with a lower rate of acute rejection during the first 6 months after transplantation compared with no induction. Other studies have shown that antibody preconditioning with alemtuzumab without maintenance immunosuppression failed to achieve tolerance in clinical transplantation. Campath-1H also has an associated cytokine-release syndrome, which reportedly has been prevented completely by coadministration of solumedrol (2 mg/kg), Benadryl (2 mg/kg), and other antiinflammatory agents. Most patients who are pretreated still become febrile with the induction dose of the medication. The treatment for this hyperpyrexia is discontinuation of the agent. The usual pediatric induction dose is 0.3 to 0.4 mg/kg infused over 8 hours. Opportunistic infections and malignancies are of grave concern with a potent lymphocyte-depleting agent such as this, but an excessive frequency has not been reported.
Liver transplantation
The first successful pediatric liver transplantation was performed in 1967 (Starzl et al., 1968). Before 1980, with the use of the immunosuppressive agents azathioprine and prednisone, the 5-year survival rate in the pediatric patient after liver transplantation was 20% (Gordon and Bismuth, 1991; Gordon et al., 1991). After the introduction of cyclosporine in 1980, long-term survival after liver transplantation became a reality. For the first time, 5-year patient survival began to exceed that of the life expectancy related to the specific disease process. Graft survival has progressively improved since 1992, with, for example, a 1-year graft survival of 81% in the 6- to 10-year-old recipient, compared with 68% a decade ago (UNOS, 2009). Patient survival for this age group is estimated to be 90.5% at 1 year, 85.9% at 3 years, and 83.8% at 5 years. Patient survival in the first year after transplantation is similar for all age groups except children younger than 1 year, who have the highest annual death rate. For these infant recipients transplanted between 2003 and 2004, there was a marked decline in 1-year death rate, a trend also seen for children ages 1 to 5 years and those ages 11 to 17 years. Improvements in patient and graft survival rates have been attributed to new immunosuppressive regimens consisting of tacrolimus Campath, mycophenolate, and rapamycin, with diminished use of cyclosporine, azathioprine, and corticosteroids and improved access to the donor pool using reduced grafts, living donors, and a new UNOS pediatric end-stage liver disease (PELD) scoring system for estimating medical urgency.
The majority of pediatric liver transplantations are performed in children less than 5 years of age. In children, 28% of grafts have been transplanted in patients less than 1 year of age, 37% in patients 1 to 5 years of age, and 35% in children 6 to 17 years of age. From 1988 to 2010, a total of 11,958 pediatric liver transplantations have been performed in the United States, of which 1264 (approximately 10%) have been living related (UNOS, 2009) (see Table 28-15).
General Indications and Contraindications
Pathophysiology of End-Stage Liver Disease
Child-Pugh Classification
The Child-Pugh classification is a universal scoring system of the degree of liver failure in patients with cirrhosis. Traditionally, the Child-Pugh class (A, B, or C) has been used as a predictive index for operative mortality rate in adult patients undergoing portosystemic shunting procedures. The estimated 1- and 5-year survival rates are 95% and 75% for patients with Child-Pugh class B, and 85% and 50% for patients with Child-Pugh class C. After the onset of the first major medical complication (ascites, variceal bleeding, jaundice, or encephalopathy), survival rates for these patients are significantly reduced. Variables measured by this system include ascites, encephalopathy, serum albumin, bilirubin, and prothrombin time (PT). Points are then assigned to different degrees of each variable, and the total points are used to assign a grade in the Child-Pugh scoring system (Table 28-16). Although a liver biopsy is often helpful in assessing histologic activity and the amount of fibrosis in patients with chronic hepatitis, it is not essential for the determination of the Child-Pugh class. Until February 2002, the Child-Pugh score was used by transplantation centers to group patients into one of four medical urgency categories. Blood type, patient size, medical urgency and waiting time determined liver allocation.
Systemic Manifestations of End-Stage Liver Disease
ESLD is associated with unique systemic physiologic alterations (Box 28-3) (Robertson, 1998).
Box 28-3 Cardiovascular, Pulmonary, and Renal Complications of Advanced Cirrhosis
Cardiovascular
Pulmonary
Cardiovascular System
Systemic vascular resistance is low because of peripheral vasodilation and shunting (cutaneous, splanchnic, intrapulmonary, portopulmonary, lumbrical, and pleural). This profound vasodilation may result from abnormal levels of vasodilator substances, possibly originating in the splanchnic circulation, which would otherwise be cleared by the liver, or from the lack of a substance produced by the liver. The most likely mediators include nitric oxide, tumor necrosis factor-α, and endothelium-derived relaxing factor. Activation of the renin-angiotensin-aldosterone system causes an increase in extracellular fluid volume through salt and water retention, and release of AVP results in a decrease in free-water excretion. Similarly, the sympathetic nervous system is activated in an attempt to cause peripheral vasoconstriction to maintain an adequate MAP. Mixed venous oxygen saturation (Svo2) measured in the pulmonary artery outflow tract is markedly elevated (usually ≥85%). The increase in Svo2 presumably results from poor oxygen extraction capacity and correlates somewhat with cardiac index (Jugan et al., 1992; Steib et al., 1993). Yet there is no evidence of tissue dysoxia or increased serum lactate with the patient at rest.
Respiratory System
Cirrhotic patients are predisposed to arterial hypoxemia from intrapulmonary shunting because of capillary vasodilation, restrictive lung disease caused by ascites or pleural effusions, impaired hypoxic pulmonary vasoconstriction, increased pulmonary blood flow, and a rightward shift in the oxygen-dissociation curve resulting from decreased levels of 2, 3-diphosphoglycerate. Respiratory compromise may also result from the hepatopulmonary syndrome (HPS), portopulmonary hypertension, defects in alveolar oxygen diffusion, or pulmonary manifestations of systemic disease (e.g., cystic fibrosis, autoimmune disease, α1-antitrypsin deficiency). These defects are seemingly compensated for by an increase in Svo2 and resting cardiac output (Schott et al., 1999; Teramoto et al., 2000). Arterial hypoxemia usually responds to supplemental oxygen and positive pressure ventilation suggestive of a V/Q mismatch. Depressed airway reflexes, delayed gastric emptying, hiatus hernia, and massive ascites increase the risk for aspiration. Pulmonary edema, atelectasis, and pneumonia are also common findings in patients with ESLD.
The frequency of HPS (chronic liver disease, increased alveolar-arterial gradient while breathing room air, and intrapulmonary vasodilation) is reported to be between 4% and 29% (Naeije, 2003; Mazzeo et al., 2004). Patients with liver disease may develop progressive and refractory hypoxemia when abnormal intrapulmonary vascular dilation causes anatomic shunting and diffusion-perfusion abnormalities (Hoeper et al., 2004). The prognosis for patients with HPS is poor, and a mortality rate of 41% within 2 to 5 years has been reported (Krowka et al., 1993). In contrast, up to 20% of cirrhotic patients are at risk of developing portopulmonary hypertension (portal hypertension and increased pulmonary vascular resistance). Severe pulmonary hypertension may cause acute right ventricular failure and sudden cardiac death (Scott et al., 1999). Preoperative therapy with epoprostenol and nitric oxide may improve outcome in this group if right ventricular function is preserved and treatment results in a decrease in pulmonary pressures and vascular remodeling.
Renal System
Renal dysfunction is common in patients with ESLD, and the kidneys are very susceptible to injury and prone to failure. Fluid and electrolyte imbalances are secondary to diuretic therapy, hypoalbuminemia, and portal hypertension causing generalized ascites, progressive edema, hypovolemia, dilutional hyponatremia, and hypokalemic metabolic alkalosis resulting from renin-aldosterone activation. Three main mechanisms, singularly or in combination, contribute to renal insufficiency: prerenal causes, acute tubular necrosis, and hepatorenal syndrome. Renal insufficiency not only complicates the management of liver failure but, more importantly, may increase patient mortality. Thus, it is important to evaluate and treat reversible causes of renal insufficiency (Box 28-4). Renal function is often difficult to assess in these patients because reduced muscle mass and hepatic synthesis of creatine reduces the serum creatinine level. Creatinine clearance will overestimate the GFR.
Box 28-4 Strategy for Optimizing Renal Function and Preventing Hepatorenal Syndrome
Initial management
Adapted from Gines P, Guevara M, Arroyo V, Rodes J: Hepatorenal syndrome, Lancet 362:1819, 2003.
The kidneys in patients with liver failure are also at increased risk for developing acute tubular necrosis (ATN) as a result of decreased renal perfusion. This decreased perfusion is a consequence of a relative decrease in central blood volume caused by splanchnic pooling, which activates secretion of vasopressin, in combination with other compensatory mechanisms attempting to restore MAP, such as increased sympathetic tone and renin-angiotensin activity. Renal perfusion is further compromised because prostaglandin synthesis is reduced in advanced liver disease. Prostaglandins are potent renal arteriolar vasodilators (Govindarajan et al., 1987; Claria and Arroyo, 2003). Hence, tubular function is much closer to an ischemic threshold in patients with liver failure. This low ischemic threshold renders the kidneys more susceptible to nephrotoxic drugs such as IV contrast dye or aminoglycosides. Additionally, in the patient with tense ascites, renal cortical perfusion may also be diminished as a result of elevated intraabdominal pressure. Urine sodium in this setting is usually greater than 20 mEq/L, with a FENa of greater than 1% (Epstein, 1985). Treatment should be directed at minimizing additional renal injury, optimizing renal perfusion, and maintaining urine output, with some form of dialysis possibly introduced until the ATN resolves. This usually occurs within 10 to 14 days. It is not uncommon, however, for the hepatorenal syndrome to become superimposed once the patient develops ATN.
The most consequential form of renal dysfunction is the hepatorenal syndrome, and it has a very poor prognosis. It is characterized by a rapid deterioration in renal function associated with profound sodium retention and low urinary sodium excretion. It is usually precipitated by a major physiologic event, such as GI hemorrhage, sepsis, or surgery. It is differentiated from prerenal azotemia by the lack of responsiveness to volume expansion. The pathogenesis is severe renal vasoconstriction with absence of renal cortical blood flow. Interestingly, this vasoconstriction is reversible if the kidney is transplanted into a host with normal hepatic function (Koppel et al., 1969). This form of renal failure requires dialysis to sustain life, but it may be reversible with liver transplantation (Iwatsuki et al., 1973).
Hematologic Complications
Anemia, thrombocytopenia, and coagulopathy are the expected findings in the patient with liver failure. Anemia is usually a result of bone marrow suppression, vitamin deficiency, hemorrhage, and diminished erythropoietin production caused by renal insufficiency. Thrombocytopenia (platelet count < 100,000/mm3) is seen in 70% of patients with liver disease (Kang et al., 1985) and is primarily a result of diminished thrombopoietin production and portal hypertension with platelet sequestration in the spleen (Kaushansky, 1995; Martin et al., 1997). However, bone marrow suppression, abnormalities in platelet metabolism or autoimmune causes may also be contributing factors (Peck-Radosavljevic, 2000). Impaired platelet aggregation and clot retraction caused by qualitative platelet defects are also seen in patients with liver disease and renal failure.
The tissue factor pathway of coagulation is classically assessed by measuring PTT and PT. The liver is the main site of synthesis of all coagulation factors except von Willebrand’s factor, which is produced primarily in the vascular endothelium. Failure of bile salt secretion results in poor absorption of vitamin K, which is a cofactor necessary for the posttranscriptional γ-carboxylation and activation of factors II, VII, IX, and X (Table 28-17). In cirrhotic patients, the levels of fibrinogen and factor VIII are usually supranormal, and the production of all other clotting factors is diminished. Additionally, approximately 80% of patients with liver failure produce an abnormal fibrinogen molecule (dysfibrinogenemia) (Martinez et al., 1978; Cunningham et al., 2002). Control of coagulation therefore depends on the balance of hepatic synthesis of clotting factors and the clearance of activated clotting factors, plasminogen activators, and fibrinolytic proteins.
Neurologic Complications
Hepatic encephalopathy is a frequent metabolic complication of acute or chronic liver disease. The neuropsychiatric abnormalities are often reversible, with the clinical presentation ranging from subtle personality changes to frank coma. Neuromuscular symptoms include tremor, hyperreflexia, and decerebrate posturing. The three theories regarding the pathogenesis of hepatic encephalopathy are (1) ammonia toxicity with accumulation of toxins in the brain, (2) an alteration in plasma amino acid composition with accumulation of false neurotransmitters, and (3) an increase in neuroinhibitory substances, such as manganese, monoamines, and endogenous opiates. Various studies have supported aspects of each hypothesis, yet none has been conclusively established. In patients who have died in hepatic coma, neuropathologic findings occur in the astrocytes rather than in the neurons. Positron emission tomography studies show decreased glucose use in the cerebral cortex, which may explain some neuropsychiatric abnormalities (Butterworth, 1996).
Gamma-aminobutyric acid (GABA), a major inhibitory neurotransmitter in the CNS, regulates the chloride channel. It has been suggested that elevated ammonia levels enhance GABA-ergic neurotransmission and synergistically augment the action of benzodiazepine receptor agonists (Basile and Jones, 1997). This theory is supported by the fact that hepatic encephalopathy can at times be improved by the benzodiazepine receptor-antagonist flumazenil.
Among the multiple precipitating factors of hepatic encephalopathy are azotemia, drugs (sedatives, tranquilizers, analgesics), GI bleeding, excess dietary protein, metabolic alkalosis, infection, and constipation (Abou-Assi et al., 2001). Treatment consists of reducing dietary protein, avoiding sedatives, administering antibiotics such as neomycin or Bactrim, administering lactulose (which converts ammonia to nonabsorbable ammonium and modifies the colonic flora), correction of hypokalemia, discontinuation of diuretics, treating infection, and volume expansion.
Pathophysiology of a Few Uncommon Genetic Disorders
Urea-Cycle Disorders
Urea-cycle disorders are one of the common inborn errors of metabolism that are clinically cured by liver transplantation (Morioka et al., 2005). As the urea cycle is the final pathway for the elimination of nitrogen. Enzymatic defects or errors in the pathway lead to the accumulation of nitrogen as ammonia, alanine, glutamate, and other intermediate metabolites. The urea cycle contains five enzyme systems, and the clinical manifestations depend on the enzyme that is deficient (Fig. 28-2). These enzyme deficiency syndromes are carbamoyl phosphate synthetase I deficiency, ornithine transcarbamylase deficiency (OTCD), argininosuccinate synthetase deficiency (citrullinemia), argininosuccinate lyase deficiency, and N-acetylglutamate synthetase deficiency. OTCD is the most common urea-cycle disorder, with a prevalence of 1 in 40,000 live births, and it is inherited as an X-linked, partially dominant chromosomal defect. Ornithine transcarbamylase is a mitochondrial enzyme that catalyzes the conversion of ornithine (the product of carbamoyl phosphate synthetase–catalyzed ammonia and bicarbonate) and carbamylphosphate to citrulline. OTCD thus results in the accumulation of ammonia, carbamylphosphate, and glutamate, with diminished production of citrulline, arginine, and urea. Because of the X-linked, partially dominant inheritance of OTCD, the phenotypical expression of OTCD is varied. In the severe form, which affects homozygous males, hypothermia, lethargy, and vomiting follow a 24- to 72-hour asymptomatic period. Symptoms are progressive and severe and are related to accumulation of glutamine and ammonia and secondary cerebral edema. In heterozygous females, the expression is more varied and can range from neonatal expression to clinically imperceptible disease. Accumulation of ammonia is exacerbated by protein breakdown at times of stress (surgery, sepsis, dehydration, anesthesia) or fasting, and also with the ingestion of high-protein foods or parenteral nutrition. Anesthesia management in these children focuses on avoidance of catabolic states through infusion of physiologic fluids containing at least 10% dextrose. Avoidance of emotional stress is imperative, with liberal use of perioperative benzodiazepines and analgesics. Hypothermia should be prevented with the use of warm room temperatures, irrigation, and warming blankets. Some centers also use continuous intraoperative IV infusions of sodium benzoate (300 to 500 mg/kg per day) for nitrogen binding. OTCD may also be independently associated with a hypercoagulable state, with documented thrombotic complications in four children (Venkateswaran, 2009).
Maple Syrup Urine Disease
Maple syrup urine disease (MSUD) is an inborn error of amino acid metabolism caused by the autosomal recessive–mediated deficiency in branched-chain α-ketoacid dehydrogenase (BCKD) activity. BCKD is the second enzyme in the pathway used in the degradation of the branched-chain amino acids leucine, isoleucine, and valine (Morton et al., 2002) (see Fig. 28-2). The pathophysiology of MSUD is primarily explained by the accumulation of leucine in the plasma and in the organs. Similar to the urea-cycle disorders, severe metabolic decompensation is associated with catabolism of tissue proteins at times of starvation and stress. The disease usually manifests within 48 hours of birth with ketonuria, irritability, and dystonia that progresses to seizures and coma by the fourth day of life (Felber et al., 1993). Diagnosis is confirmed by testing for the presence of branched-chain 2-ketoacids in the urine and by quantification of branched-chain amino acids in the plasma. Children are treated with dietary restriction of branched-chain amino acids and avoidance of catabolic states. Despite strict dietary control, severe metabolic crises can still develop suddenly during intercurrent illnesses and can be associated with life-threatening cerebral edema. In addition to facing the dangers of acute metabolic decompensation, patients with MSUD often suffer from neurocognitive disorders, probably from imbalances of branched-chain amino acids in the brain. Because of their protein-restricted diet, MSUD patients can also develop essential-amino-acid deficiencies, resulting in immunodeficiency, growth failure, and global developmental delays. Patients undergoing liver transplantation for MSUD are carefully selected, and successful outcomes depend on a multidisciplinary approach to their management in the perioperative period (Strauss, 2006). A careful neurologic examination should be documented before sedation to serve as a baseline, and a careful history of past metabolic crises and neurologic deficits should be obtained. Baseline levels of branched-chain amino acids should be recorded, and, if they are excessive, transplantation should be deferred and medical treatment initiated. Like patients with urea-cycle disorders, patients with MSUD cannot be fasted without catabolic consequence. In addition, specialized total parenteral nutrition (TPN) should be used that include concentrated dextrose infusions. MSUD patients should never receive hypotonic infusions, and plasma osmolarity should be carefully monitored and kept in the normal range. Perioperative sedation and analgesia should be optimized to avoid catabolism, and physiologic stress should be kept to a minimum (Kahraman, 1996; Fuentes-Garcia, 2009). Leucine levels are generally followed after reperfusion of the liver and daily after the transplantation. Patients are immediately fed an unrestricted diet or are given enteral feedings with standard protein compositions.
Domino Liver Transplantation
Domino liver transplantation is a procedure in which an index patient (e.g., one with MSUD) with a genetic defect receives a hepatic allograft, and that index patient’s liver is then carefully resected and removed, maintaining anatomic integrity, and it is then flushed and used as an allograft for a second patient (Khanna, 2006; Wilczek, 2008). The hepatectomy of the index donor must preserve adequate vascular and biliary cuffs for use in implantation into the second recipient. Because of the anatomy of the hepatic veins, this often requires the hepatectomy to be performed in the standard manner, which involves removal of the retrohepatic vena cava together with the hepatectomy specimen. As the index patients do not have portal hypertension or parenchymal liver disease, portal and caval clamping is not well tolerated and venovenous bypass (VVB) is often required in older patients to avoid significant mesenteric venous hypertension, associated visceral edema, and hemodynamic instability.
Alagille’s Syndrome
Alagille’s syndrome (AS) is an autosomal disorder characterized by bile duct paucity, cholestasis, and progression to cirrhosis, requiring transplantation in 30% of patients. The syndrome is also associated with characteristic facial features, ocular manifestations, vertebral anomalies, and pancreatic insufficiency. Renal disease is another important feature of this syndrome with structural and functional disorders in up to 50% of patients including renal artery stenosis, dysplastic or ectopic kidneys, and tubulointerstitial nephropathy. Idiopathic intracranial hemorrhage occurs in up to 15% of patients and is often fatal. Patients with AS should be screened with magnetic resonance angiography (MRA) of the head prior to transplantation to identify any cerebral vascular malformations. Cardiac anomalies are also a component of AS, with the most common manifestation being central or peripheral pulmonary arterial stenoses. Up to 24% of patients also have associated intracardiac anomalies. Tetralogy of Fallot, aortic coarctation, septal defects, patent ductus arteriosus, pulmonary atresia and pulmonary stenosis have also been reported with AS (Perlmutter and Shepherd, 2002). In patients with right ventricular outflow obstruction, the severity of the outflow gradient is a major factor in the perioperative morbidity and mortality.
Wilson’s Disease
Wilson’s disease (hepatolenticular degeneration) is an autosomal recessive disorder characterized by progressive or acute liver decompensation in the first 2 decades of life, although its appearance later with neuropsychiatric symptoms is also common. Wilson’s disease represents a mutation in the ATP7B gene, which causes a progressive accumulation of copper in the liver as a result of impaired copper excretion into the bile. As time passes, the capacity of the liver to accumulate copper is exceeded, and copper is released into the circulation. In addition to liver decompensation, copper accumulation also affects the CNS, with a myriad of neuropsychiatric symptoms. Severe Coombs-negative (non-immune-mediated) intravascular hemolysis is frequent and is the presenting symptom in up to 15% of patients. Arrhythmias, autonomic dysfunction, and LV hypertrophy are seen in up to 25% of patients (Hlubocká et al., 2002).
α1-Antitrypsin Deficiency
α1-Antitrypsin deficiency (A1AT) is a relatively common autosomal recessive disorder. It is a serine protease inhibitor that inhibits destructive neutrophil proteases, and it limits the destructive effects of neutrophil effectors during times of inflammation. In the homozygous form, there is a 90% reduction in circulating concentrations of A1AT. α1-Antitrypsin has a reported incidence of liver involvement in 10% to 20% of patients, primarily those who are PIZZ homozygotic (Psacharopoulos et al., 1983). The incidence of this phenotype is 1 in 7000 in the United States and 1 in 2000 in Scandinavia (Schwarzenberg and Sharp, 1990). Males are affected more frequently than females. Some 10% to 20% of PIZZ individuals develop neonatal cholestasis, and jaundice is often the first sign of this disease. The liver disease associated with A1AT deficiency can become clinically apparent at any time from infancy to late adulthood. Of note for anesthesiologists, patients with homozygous A1AT deficiency develop progressive emphysematous lung disease; however, these changes are generally not severe until well into adulthood.
Fulminant Hepatic Failure
Fulminant hepatic failure (FHF) is the most severe, life-threatening complication of liver failure. See related video online at www.expertconsult.com. Differentiating acute fulminant liver failure from an acute exacerbation of chronic liver disease has important therapeutic and prognostic implications, as not all patients with acute liver failure are the same. Fulminant hepatic failure is usually defined as rapidly progressive liver failure, with the onset of encephalopathy within 8 weeks of the onset of jaundice in patients without a previous history of liver disease. However, no uniform definition of FHF in children has been established yet. One definition that is widely accepted is the sudden onset of liver failure with altered mental status and coagulopathy in an otherwise healthy child (Nazer and Nazer, 2004). FHF was the primary diagnosis in 15% of pediatric patients transplanted from 1995 to 2000. Viral hepatitis and drug-induced hepatotoxity are the two most common causes, but in most cases, the etiology is unidentified. For the majority of patients with fulminant failure, survival ultimately depends on medical stabilization and urgent liver transplantation, as mortality rates may reach 80%.
Cerebral edema appears to be the major cause of morbidity in patients with FHF and contributes to the high mortality. Early signs and symptoms include increased muscle tone, arterial hypertension, seizures, agitation, and sluggish papillary response to light. Infants may present with poor feeding, irritability, and altered sleep patterns. The mechanism of cerebral edema is unknown, although vasogenic and cytotoxic etiologies have been proposed. Whatever the cause, cerebral edema ultimately leads to intracranial hypertension, impairment of cerebral perfusion, irreversible neurologic brain injury, uncal herniation, and death (Hanid et al., 1979). Encephalopathy may be classified according to the scheme in Table 28-18. This is useful to judge the effects of treatment and assess the progression of the disease process.
Medical treatment is generally supportive until either recovery with hepatocyte regeneration, liver transplantation (deceased, split, or living related donor), hepatocyte transplantation, or death. See related video online at www.expertconsult.com. Experimental approaches have been attempted using liver-assist devices. Supportive measures are directed at minimizing morbidity and mortality from serious complications. This includes a critical care unit, monitoring, endotracheal intubation for airway protection, infusions of 10% to 20% dextrose to prevent hypoglycemia, replacement of calcium phosphorous and magnesium, antibiotic treatment of infections, maintenance of adequate urine output, avoidance of nephrotoxic drugs with surveillance for renal insufficiency, and H2-blockers for stress ulcer prophylaxis. Correction of coagulopathy is with vitamin K and plasmapheresis. In general, platelets and fresh frozen plasma are reserved for patients with active bleeding. Normalization of the patient’s PT is often used as a prognostic indicator for recovery of synthetic function. Avoiding volume overload and management of intracranial hypertension are crucial in the management of FHF. Protein intake is restricted. Lactulose enemas and oral neomycin are administered to decrease enteric bacteria that produce ammonia If a causative agent is identified, specific treatment should be initiated early (e.g., N-acetylcysteine for acetaminophen overdose).
Initial management of cerebral edema should begin with a computed tomography (CT) scan of the brain to assess cerebral blood flow with xenon (Aggarwal et al., 1994). If there is evidence of cerebral edema and compromise of cerebral blood flow without CO2 responsiveness, continuous monitoring of intracranial pressure is vital, especially in stage 3 or 4 encephalopathy. Therapeutic measures to reduce ICP include head-up positioning, ventilatory support with moderate hyperventilation, and mannitol for a documented ICP greater than 30 mm Hg and for progressive edema. It may be efficacious to place a jugular bulb catheter so that adequate assessment of cerebral metabolism is possible (Lassen and Lane, 1961). The important parameters that aid in management are the measured arteriovenous oxygen content difference (AVDo2), glucose, and lactate differences across the brain (Aggarwal et al., 1993, 1994). Mild hypothermia (to 34° C) and barbiturate coma remain controversial topics with respect to the management of hepatic coma. Barbiturate coma should be reserved until all therapeutic interventions have failed to reduce ICP. However, hypothermia should be initiated early in stage III coma. Continuous EEG monitoring is essential, as the goal is to achieve EEG silence with IV barbiturates.
Prognostic criteria include the patient’s age, cause of liver disease, onset and degree of encephalopathy relative to the appearance of jaundice, serum bilirubin level, PT and INR, serum creatinine, factor V level, and arterial pH. A sensitive predictor of outcome is the INR value. With an INR of 4 or more, the mortality rate reaches 86%; with an INR of less than 4, it may be as low as 27% (Nazer and Nazer, 2004).
Cardiovascular Evaluation
Cardiac function in adults with cirrhosis has been extensively investigated, but there is little information available on children. A study of 22 children with cirrhosis compared with a control group of healthy age- and gender-matched children (mean age, 4.1 ± 3.5 years) reported that pediatric OLT candidates have normal LV systolic function unless their heart was primarily involved in the underlying disease. In advanced liver failure, LV systolic function may be impaired. These children also had increased systolic LV posterior wall thickness, which may reflect LV hypertrophy and impaired diastolic function. LV ejection fraction is usually normal or increased at rest in adults with cirrhosis unaccompanied by ascites (Grose et al., 1995; Laffi et al., 1997). The presence of ascites adversely affects cardiac function in adults (Valeriano et al., 2000). In contrast, most pediatric patients with ascites have normal LV systolic function. Cyanosis is not a reliable sign of hypoxemia in children because of changes caused by crying, anemia, and jaundice. Clubbing in patients with chronic liver disease is a common finding, with a prevalence ranging from 23% to 32% (Ozcay et al., 2002). Given the physiologic stresses inherent in liver transplantation surgery, cardiac problems may emerge perioperatively that contribute to significant morbidity and mortality in patients with cirrhosis and mild or latent cardiomyopathy (Myers and Lee, 2000).
Pulmonary Evaluation
Cirrhosis affects the pulmonary circulation, lung parenchyma, and pleural spaces. Reduced alveolar-capillary diffusion (DLco) may be seen in the absence of significant hypoxemia, especially in patients with hepatitis C virus (HCV) cirrhosis. This anatomic derangement of the alveolar-capillary membrane worsens with disease progression, with the measured DLco becoming abnormal at less than 80% of predicted value. A reduced diffusion capacity may persist up to 15 months after transplantation (Ewert et al., 1999). Of interest, the Hepatitis C Association supports a no-smoking policy for teens and adults, as smoking has been determined to be an independent risk factor associated with elevated ALT levels among anti-HCV-seropositive patients (Wang et al., 2002; Hezode et al., 2003).
Mild Arterial Hypoxemia
Mild arterial hypoxemia is common in patients with cirrhosis and primarily caused by a decrease in functional residual capacity (FRC) and total lung capacity (ascites, pleural effusions), impaired diffusion capacity, and pulmonary arteriovenous shunting (Liu and Lee, 1999). In advanced liver diseases, ventilation-perfusion () defect is an important cause of hypoxemia. The arterial blood gas analysis with the patient standing and breathing 100% Fio2 is of particular importance, because severely hypoxemic patients with at least a moderate response to breathing 100% oxygen on standing (Pao2 > 150 mm Hg) are thought to have an adequate pulmonary reserve and may be safely oxygenated intraoperatively (Krowka et al., 1997; Mohamed et al., 2002). Unresponsive patients should be suspected of having a fixed shunt, and transplantation should be delayed pending further evaluation.
Hepatopulmonary Syndrome
Hepatopulmonary syndrome was once considered a contraindication to transplantation, but now it is considered an indication for early liver transplantation, as there is no successful long-term medical treatment. Patients typically present with progressive exertional dyspnea and hypoxemia. HPS is characterized by the triad of chronic liver disease, hypoxemia, and intrapulmonary shunting in the absence of primary cardiac or pulmonary disease. The incidence in the pediatric patient population is unknown. The pathologic defects causing arterial hypoxemia include intrapulmonary vascular dilation resulting from dilated precapillaries, direct arteriovenous communications, portopulmonary communication, and pleural arteriovenous malformations. This results in decreased oxygen diffusion into the dilated vessels, along with decreased intrapulmonary transit time and decreased hypoxic pulmonary vasoconstriction. This is not a true anatomic shunt, as the patient with the more common type I lesion demonstrates a significant Pao2 response to 100% oxygen. Appropriate preoperative evaluation should include a chest radiograph, an arterial blood gas measurement with the patient breathing room air and then 100% Fio2, and one of several imaging techniques: perfusion lung scanning, contrast-enhanced (microbubble) transthoracic echocardiography, lung scintiscan, and, rarely, pulmonary angiography (which identifies type I O2 reactive versus type II O2 nonreactive lesions). With liver transplantation, HPS is reversible (Liang et al., 2001), but regression of vascular abnormalities in patients with true anatomic shunts and those with marked precapillary dilation and evidence of poor response to supplemental oxygenation is not predictable (Lange and Stoller, 1995). Treatment of HPS type II presents a dilemma, although liver transplantation with concomitant lung transplantation is a possible choice (Yuan et al., 2003).
Portopulmonary Hypertension
Pulmonary arterial hypertension as a consequence of liver dysfunction is termed portopulmonary hypertension (PPH). This is a pulmonary vasoproliferative and vasoconstrictive process leading to pulmonary hypertension (increased peripheral vascular resistance and normal PCWP or LV end-diastolic pressure) and right heart failure frequently not reversible by liver transplantation. This disorder is uncommon (20% of patients with cirrhosis of the liver), and its existence in the pediatric population is not well described. How rapidly PPH can develop varies, as reports indicate anywhere from 3 weeks to 5 years. Remarkably, a review of published PPH cases through 1999 documented that 65% of diagnoses were first recognized during the liver transplantation procedure (Krowka et al., 2004). The clinical presentation is subtle and includes exertional dyspnea, fatigue, ankle edema, chest pain, and syncope. Arterial hypoxemia is reported in 80% of patients with moderate to severe disease, with an increased alveolar-arterial oxygen gradient, reduced diffusion capacity, and accentuated respiratory alkalosis (Kuo et al., 1997; Cotton et al., 2002). Transthoracic contrast-enhanced echocardiography is the screening procedure of choice, with right heart catheterization the gold standard for making the diagnosis and assessing right ventricular function. The best prognosis is in patients with mild symptoms, preserved right heart function, and pulmonary arteries responsive to vasodilator therapy. Treatment options include inhaled nitric oxide, sildenafil (Viagra), calcium channel blockers, anticoagulation, digoxin, diuretics, supplemental oxygen, and IV prostacyclin. The significance of this disease entity is its high perioperative morbidity and mortality in patients undergoing OLT. The available data indicate a perioperative mortality of greater than 70% with a mean pulmonary artery pressure of 45 mm Hg or higher, and up to 100% if the mean pressure is greater than 50 mm Hg at the time of transplantation. The national liver transplantation database reports an overall mortality perioperatively of 36%. The key to survival is preserved right ventricular function. Even after OLT, however, the pulmonary vascular abnormalities may progress unless long-term pulmonary vasodilator therapy is instituted.
Renal Evaluation
Renal dysfunction in conjunction with worsening hepatic function is common. Acute renal failure requiring hemodialysis is a strong predictor of mortality in these patients. Bartosh and colleagues (1997) reported abnormal renal function in one third of children after liver transplantation, with acute renal failure requiring dialysis (in 6.2%) being a predictor of a high mortality rate (85%). The etiology of renal insufficiency in the patient with ESLD is usually multifactorial. Possible causes include hepatorenal syndrome, disturbances of salt and water clearance, acute tubular necrosis, renal pathology associated with the underlying liver diseases, and diminished intravascular volume causing prerenal azotemia. Exposure to nephrotoxic drugs such as IV contrast dyes, aminoglycosides, or nonsteroidal antiinflammatory agents may also contribute to ATN.
Urine sodium handling is an important variable during evaluations. Both prerenal azotemia and hepatorenal syndrome are characterized by normal urinary sediment, very low urine sodium concentrations (<10 mEq/L), azotemia, and oliguria; it is therefore important to exclude hypovolemia. Specifically, hepatorenal syndrome does not respond to a fluid challenge with diuresis, whereas the expected response in the patient with prerenal azotemia is diuresis and a subsequent decrease in the serum creatinine and urea nitrogen levels. ATN tends to be salt wasting at its initial presentation, with urine sodium concentrations characteristically greater than 20 mEq/L. An important diagnostic test is the FENa, which also aids in the distinction between these two disease processes (FENa ≤ 1% for HRS and prerenal azotemia, and greater than 1% for ATN). Obtaining an accurate GFR using creatinine clearance rate values in patients with cirrhosis and ascites may give spurious results, as GFR measurements may range from high values to those diagnostic of end-stage renal disease despite the presence of a normal serum creatinine level (Papadakis and Arieff, 1987).
Prerenal azotemia caused by hypovolemia usually resolves with judicious volume replacement. ATN is usually self-limited, with resolution in 4 to 6 weeks with appropriate support. However, the hepatorenal syndrome is usually not reversible without liver transplantation (Gonwa et al., 1989).
Surgical Technique
The technique used for liver transplantation in children has evolved over the past two decades and varies greatly between different centers, but the physiologic changes that occur during the procedure can be divided into four distinct stages. Here, we review the operative considerations during each stage, and a little later, the anesthesia management during each stage will be discussed. In general, open communication between the surgical and anesthesia teams is essential to avoid difficulty in the progression between the different stages. See related video online at www.expertconsult.com.
Stage I: Preanhepatic Stage
The second portion of stage I entails the dissection of the inferior vena cava (IVC), culminating in the hepatectomy. This can be performed in two ways. The standard complete hepatectomy (Starzl et al., 1984; Starzl and Iwatsuki, 1987) (Fig. 28-3) is performed with complete excision of the retrohepatic vena cava along with the liver. The alternative approach is the one primarily used for the pediatric patient: the “piggyback” hepatectomy (Tzakis et al., 1989). With the standard technique, during stage I, the infrahepatic cava is circumferentially dissected with careful control of adrenal vasculature, and the suprarenal cava is prepared for clamping. Similarly, the suprahepatic cava is isolated. The standard procedure requires careful preparation for complete caval occlusion. IV infusions should be limited to the tributaries of the superior vena cava (SVC), as those infused below the diaphragm encounter upstream obstruction once the IVC is clamped. Most children tolerate complete caval occlusion well; however, the surgical team should always perform a brief test clamp before structures are divided to allow proper fluid resuscitation to begin. If hemodynamic stability is not achieved with clamping, the use of VVB to augment preload during caval clamping should be considered (see later). Although the standard hepatectomy generally affords some usefulness in tumor cases and can be done relatively quickly, excision of the native cava requires a separate, lower caval anastomosis to be performed prior to reperfusion, possibly extending the warm ischemic time and adding an additional site of potential complication.
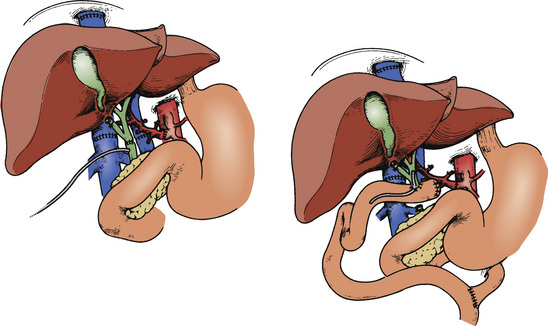
FIGURE 28-3 Biliary tract reconstruction with choledochojejunostomy, using a Roux-en-Y limb.
(From Starzl TE, Iwatsuki S, VanThiel DH, et al: Evolution of liver transplantation, Hepatology 2:614, 1982.)
The piggyback hepatectomy (Tzakis et al., 1989) (Fig. 28-4) involves careful dissection of the liver from the retrohepatic vena cava with preservation of the native vena cava. Briefly, the hepatic veins connecting the posterior liver and the cava are isolated and divided, allowing the liver to be lifted off the vena cava. The main hepatic veins are also circumferentially dissected and readied for clamping. Although the piggyback procedure can take some additional time, avoidance of caval division and the need for one less anastomosis makes it an attractive option in children. Hemodynamic instability is usually insignificant because of the retroperitoneal collateralization of vessels with the azygous system. However significant blood loss can occur with accidental injury to the vena cava, the short hepatic veins, or the main hepatic veins. Although complete caval occlusion is not needed, partial obstruction of the cava generally occurs and can result in some instability (Moreno-Gonzalez et al., 2003).
Venovenous Bypass
The routine use of VVB during the anhepatic phase of OLT, with or without preservation of the cava, remains controversial. In early experiments of OLT in a noncirrhotic dog model, it was discovered that clamping of the portal vein or IVC resulted in death of the animal within 30 minutes (Shaw et al., 1985). Although it was later found that most patients can tolerate caval and portal vein clamping remarkably well, Shaw and colleagues (1984) reported a 10% intraoperative mortality due to hemodynamic instability in adults during the anhepatic phase. Therefore, a bypass system was developed consisting of heparin-bonded tubing and a centripetal-vortex pump, allowing cannulation of the femoral and portal veins and diversion of mesenteric and IVC blood to the SVC through the subclavian or axillary veins (Griffith et al., 1985) (Fig. 28-5). There are no published randomized clinical trials of specific outcomes to evaluate its potential benefits.
It is difficult to use VVB in patients weighing less than 40 or 45 pounds (~20 kg) (Shaw et al., 1984, 1985). The prerequisite VVB blood flow is usually 20% to 40% of the cardiac output, or greater than 1 L/min (Griffith et al., 1985). As patients are not heparinized, the extremely low-flow states (<500 to 700 mL/min) that result in children weighing less than 20 kg would predispose to almost certain formation of emboli. Despite these findings, VVB still has some specific value in pediatric liver transplantation—notably in larger children with FHF or in older pediatric recipients who are being used as donors for living-donor domino transplantations.
Anesthesia Management
Anesthesia management can be categorized into four distinct stages corresponding to the four surgical stages previously described. Thus, an understanding of the pathophysiologic changes that occur in each stage of the surgery allows the anesthesiologist to anticipate and appropriately diagnose, manage, and treat the cardiovascular, hematologic, metabolic, and other derangements encountered throughout the surgery (Borland et al., 1985; Carlier et al., 1987; Kang and Gelman, 1987; Lindop and Farman, 1987; Carton et al., 1994a, 1994b). Liver transplantation in the pediatric patient is very similar to that in adults from a technical point of view, but there are distinct differences in transfusion requirements and strategies for minimizing blood loss, perioperative coagulation, hemodynamic consequences of caval clamping and reperfusion of the liver, monitoring, vascular access, incidence of thrombosis of the hepatic artery, retransplantation rate, and postoperative pain relief.
Induction and Maintenance of Anesthesia
Succinylcholine has traditionally been used for intubation in pediatric patients at risk for aspiration. Today, this practice is controversial because of its black-box warning. Because it is difficult to identify which patients are at risk, it is recommended that the use of succinylcholine in children should be reserved for emergency intubation or for when immediate securing of an airway is necessary, as succinylcholine has a faster onset (30 to 60 seconds) than other muscle relaxants. Liver disease, especially if chronic and severe, may be associated with decreased plasma levels of pseudocholinesterases, which will result in prolongation of the duration of action of succinylcholine or drugs requiring ester hydrolysis for degradation (Khoury et al., 1987). However, this effect is of little clinical significance when one considers the duration of perioperative ventilation and the dilutional effect of blood replacement during massive intraoperative bleeding.
Alternatively, using a relatively large dosage of a nondepolarizing muscle relaxant (e.g., rocuronium) permits securing the airway rapidly without placing the patient at risk for hyperkalemia. Cisatracurium is the ideal drug for patients with end-stage liver or kidney disease, as it undergoes spontaneous breakdown at physiologic temperature and pH (Hoffmann elimination), as well as ester hydrolysis. However, the dosage required for a rapid onset of action may result in hypotension. In patients with hepatorenal syndrome, nondepolarizing muscle relaxants should be administered cautiously and titrated to effect. Muscle relaxants requiring significant renal excretion include pancuronium (40%), doxacurium (30%), and pipecuronium (38%). Vecuronium and pancuronium also have 3-hydroxy metabolites, which accumulate in renal failure. The 3-hydroxy metabolite of vecuronium is 50% as potent as the parent compound, and that of pancuronium has two-thirds the potency of the parent compound. Muscle relaxants that are metabolized in the liver include pancuronium, vecuronium, rocuronium, and pipecuronium. Vecuronium and rocuronium also have significant biliary excretion. Pancuronium has an increased volume of distribution, prolonged elimination half-life, and decreased plasma clearance in patients with cirrhosis. Therefore, these patients may need more pancuronium initially to achieve muscle relaxation, and they have a prolonged recovery of blockade between doses (Duvaldestin et al., 1978). In patients with biliary obstruction, the initial dosage of pancuronium required is unchanged, but the duration of action of each dose is similarly prolonged (Duvaldestin et al., 1978). This may be advantageous because the operative procedure is lengthy and ventilation is usually required for at least 24 hours postoperatively.
Following induction, the patient is positioned on the operating table supine with the arms abducted and elbows flexed. It is essential to keep the patient covered, warm, and dry. Strategies include the use of a warming blanket, heated humidified gases, or IV fluid warmers; wrapping the extremities; mylar foil wrapping; and use of a thick cotton mattress pad below with a U-shaped surround Bair Hugger forced-air warming device. Care should be taken to avoid stretch injury of the brachial plexus or pressure necrosis of the occiput, ears, heels, sacrum, or elbows. After the induction of anesthesia, additional monitoring is established, using end-tidal CO2, rectal and esophageal temperature probes, an esophageal stethoscope, an indwelling urinary catheter, a peripheral nerve stimulator, and systemic arterial and CVP cannulas. Access to arterial pressure monitoring above the diaphragm is ideal because cross-clamping of the abdominal aorta may be necessary during the hepatic arterial anastomosis. A femoral arterial catheter can also be used during surgery if there is concern about the accuracy of the radial arterial blood pressure (Kamath et al., 2001). One or two additional large IV cannulas (preferably 20- or 18-gauge) are then inserted in the upper extremities, either percutaneously or by surgical cutdown. The use of VVB in larger children may preclude use of the left arm for venous access. If vascular access in the arms is difficult to obtain, the lower extremities may be used, with the understanding that during the anhepatic stage, venous return to the heart depends on collateral flow or VVB. A central venous catheter is placed in the external or internal jugular vein. In smaller children, the surgeon will often insert a Hickman catheter (large-bore, double-lumen central venous line). The use of a sheath-type catheter in larger children allows the rapid infusion of large volumes of blood components and, in rare circumstances, the insertion of a flow-directed, balloon-tipped pulmonary artery catheter. Technical difficulties in placing a pulmonary artery catheter and subsequent displacement or migration by surgical maneuvers generally preclude its routine use in pediatric patients.
During anesthesia, all factors inducing arterial hypotension should be avoided. General anesthesia and surgery decrease hepatic blood flow and jeopardize oxygen supply to the liver. Intraoperative reductions in the arterial blood pressure and cardiac output decrease portal blood flow. Contributing factors include anesthesia drugs (inhalational anesthetics, vasodilators, β-blockers, α-1-agonists, histamine receptor-2 (H2) blockers, and vasopressin), hypovolemia, ventilatory mode, hypoxemia, hypercarbia, and acidosis. Surgical manipulation in the right upper quadrant can reduce hepatic blood flow up to 60% from sympathetic activation or direct compression of the vena cava and splanchnic vessels. Compensatory vasodilation of the hepatic artery in response to decreased portal inflow is diminished by volatile anesthetic agents in a dosage-related manner (and absent in a denervated liver), and consequently blood flow becomes pressure dependent. Isoflurane has the least detrimental effect on hepatic blood flow. A simultaneous decrease in the liver’s metabolic demand tends to balance the oxygen supply-uptake ratio. A study of hepatic circulation in pigs during surgical stress and anesthesia suggested that fentanyl and light isoflurane provided adequate hepatic oxygen supply, whereas anesthesia with concentrations of isoflurane that decreased blood pressure more than 30%, or with halothane in any concentrations studied, resulted in inadequate hepatic oxygen supply (Gelman et al., 1987). Matsumoto and colleagues (1987) examined the effects of various anesthetic agents on hepatic oxygen supply and hepatic oxygen consumption in the presence of hypoxia. Their results showed that during exposure to mild hypoxia, a dosage of isoflurane less than the minimum alveolar concentration (sub-MAC) maintained the relation of hepatic oxygen supply to hepatic oxygen consumption better than thiopental, halothane, or enflurane.
When administering drugs to patients with chronic liver disease, all dosages should be decreased and carefully titrated until the desired effect is achieved. Current practice is to administer isoflurane alone or in combination with small dosages of fentanyl. The anesthesia management protocol used at the Children’s Hospital of Pittsburgh, and the anesthesia management problems encountered during the four stages of the surgery, can be found online at www.expertconsult.com.
Physiologic Alterations during Liver Transplantation
Cardiovascular Changes
During stage I, hypotension results from major fluid shifts, bleeding, and ionized hypocalcemia (Jawan et al., 2003). Transient decreases in MAP are not unusual in this stage and result from surgical manipulation of the liver and compression of the IVC, which transiently preclude venous return to the heart. Drainage of ascites may result in hypotension if the patient is not adequately hydrated prior to incision of the peritoneum. Factors contributing to blood loss include adhesions from prior operations, coagulopathy (factor deficiency, low platelet count, abnormal fibrinogen, or disseminated intravascular coagulation), portal hypertension and collateral venous circulation, and lack of surgical hemostasis (especially a laceration to the IVC). Placement of the suprahepatic caval clamp may be associated with arrhythmia and hypoxemia because of acute right ventricular outflow tract obstruction, as the pericardium and a portion of the RV may be included in the clamp. Hypotension that is unresponsive to IV pressors should raise suspicions of absolute or relative hypovolemia (limited preload caused by vascular clotting or torsion of the liver on its vascular pedicle), acidosis, sepsis, or vasoparesis.
During stage II, classic OLT requires a trial of portal vein and IVC clamping. The hemodynamic response is characterized by a decrease in cardiac output, CVP, and PAP and compensatory increase in heart rate and SVR (Eyraud et al., 2002). Hemodynamic instability may result from decreased venous return (modified by VVB or piggyback technique) and insufficient physiologic compensation by the patient for this acute change. Therapeutic strategies include colloid or crystalloid boluses, and vasopressor support with dopamine or norepinephrine (phenylephrine or epinephrine). The goal is to maintain the lowest filling pressures compatible with an acceptable MAP in anticipation of an increase in PAP with removal of the caval and portal vein clamps. Pulmonary hypertension may result because of an increase in venous return from the gut and lower extremities and reactive changes in PVR with the release of vasoactive hormones that are recirculated systemically. The donor liver is flushed with crystalloid or colloid, or by backwashing (backflushing) the liver. The procedure for backwashing involves insertion of a red rubber cannula into the recipient/donor IVC, and then removal of the clamp from the portal vein allows washing of the liver with autologous blood retrograde from the portal vein through the donor liver, exiting through the cannula into a stainless steel graduated cylinder. If the patient become immediately and dramatically hypotensive, the surgeon must be notified immediately. Hypotension associated with this maneuver can be ameliorated with the prophylactic administration of phenylephrine or epinephrine boluses, gentle fluid administration, or transfusion of packed RBCs if the hematocrit is low. The elimination of air bubbles, hyperkalemic preservation solution, hormones, or other “evil humors” has been credited for decreasing the incidence, in adult patients, of subsequent reperfusion syndrome that may occur with reperfusion of the liver (Fukuzawa et al., 1994). The goal for warm ischemia time is usually less than 60 minutes, so managing hypotension and preparing for reperfusion occurs over a relatively short period. Reactivation of HCV has been shown to be less if the warm ischemia time can be kept under 35 minutes, which shortens the target time for completing this portion of the operation even further (Clavien et al., 2004).
During stage III, profound hemodynamic instability may occur immediately after graft reperfusion. This instability includes severe hypotension, bradycardia, supraventricular and ventricular arrhythmias, variable cardiac output, and occasionally cardiac arrest (0% to 0.5%). These changes are caused by recirculation of the residual cold, acidotic, hyperkalemic preservation solution from the donor liver directly into the recipient’s heart. The incidence of this postreperfusion syndrome (defined as a decrease in MAP and heart rate > 30% from baseline for at least 1 minute within 5 minutes of reperfusion) in adults may be as high as 30%, and epinephrine boluses are usually required to prevent cardiovascular collapse (Aggarwal et al., 1987). Immediately after reperfusion, LV function may be impaired, and PCWP, CVP, and PAP usually increase with a major decrease in SVR, and transesophageal echocardiogram (TEE) monitoring shows a stable or even decreased LV end-diastolic volume. These contradictory findings may be result from a period of deteriorated LV compliance, or cardioplegia, on reperfusion (Suriani et al., 1996; De Wolf, 1999). The etiology of PRS remains unclear, but it may be caused by the release of vasoactive mediators from the ischemic liver or decompressed portal circulation, changes in the rate of venous return (volume overload), and perhaps an increase in serum potassium. Possible mediators that are highly suspect include nitric oxide and tumor necrosis factor-α, with demonstrably increased levels after graft reperfusion (Nishimura et al., 1993), and xanthine oxidase, a generator of cytotoxic oxygen radicals, which may produce myocardial dysfunction and cellular damage. Vasoactive drugs for pressor support (norepinephrine, epinephrine, dopamine) may be required, and a balance is reached between a lowered MAP and often a very low SVR permitting increased flow to perfusable organs at a lower perfusion pressure. High filling pressures should be avoided, as they may cause congestion of the donor liver, bleeding from surgical sites, and biventricular dysfunction with pulmonary edema. Therapies for reducing filling pressures include limiting IV infusions, furosemide-induced diuresis, vasodilation (IV nitroglycerine or morphine), nitric oxide, or prostaglandin E1.
Blood Loss, Coagulation, and Hemostasis
During liver transplantation surgery, the effects of fibrinolysis, thrombocytopenia, decreased coagulation factors, and fibrinogen deficiency on clinical bleeding are not always predictable, and transfusion requirements are variable. Portal hypertension with fragile venous collaterals, adhesions from prior operations, and lack of surgical hemostasis contribute to blood loss. Independent predictors of increased transfusion requirements include the severity of liver disease or Child-Pugh classification (especially for those hospitalized for inpatient support), preoperative PT, history of abdominal operations, preoperative hematocrit, and factor V levels. In addition, portal vein hypoplasia, the use of a reduced-size liver graft, and increased operative time make surgery technically challenging (Ozier et al., 1995; Maurer and Spence, 2004). Usually, the greatest operative blood loss occurs during vascular dissection and the hepatectomy phase (stage II). During this stage, there is a progressive degradation of the coagulation cascade, and a dilutional coagulopathy with a progressive thrombocytopenia. The blood loss can range from 0.5 to 25 times the patient’s blood volume (Borland et al., 1985).
Alterations of coagulation in the pediatric patient during OLT have been studied (Kang et al., 1989). Classic hourly monitoring of the PT, activated PTT (aPTT), and platelet counts demonstrates a progressive prolongation of the PT and PTT, as well as a significant decrease of all clotting factors. On graft reperfusion, there may be profound prolongation of the PTT, usually to greater than 100 seconds. Besides the standard coagulation tests (PT, aPTT, fibrinogen, platelets, and D-dimer), the thromboelastogram (TEG) and Sonoclot (coagulation and platelet function analyzer) are used in the evaluation of coagulation. The TEG is performed using whole blood; it assesses clot formation until an endpoint of clot lysis or retraction is determined. TEG findings have correlated with clinical bleeding and can assist in treating intraoperative hemorrhage by identifying the cause of the bleeding diathesis (factor deficiency, fibrinolysis, heparin effect, thrombocytopenia, or platelet dysfunction) (Zuckerman et al., 1981; Kang, 1986) (Fig. 28-6). Abnormalities of the reaction time (r), the alpha angle (a), or the maximum amplitude (MA) may indicate decreased clotting factors, diminished factor VIII and fibrinogen, or diminished platelet function or number, respectively (Kang et al., 1989). In contrast, a short reaction time is indicative of a hypercoagulable state. This has occasionally been observed in patients who have developed thrombosis after graft reperfusion (Kang, 1995a, 1995b; Gologorsky et al., 2001; Planinsic et al., 2004).
Increased fibrinolytic activity is observed in patients with ESLD as a result of increased tissue plasminogen activator activity and reduced synthesis of fibrinolysis inhibitors. Tissue plasminogen activator further increases during the anhepatic stages and peaks immediately after graft reperfusion. Various antifibrinolytic agents have been used to counter this accelerated fibrinolysis (evident immediately on graft reperfusion in up to 80% of patients), but their precise role remains undefined. These include aprotinin, ε-aminocaproic acid (EACA), and tranexamic acid. Aprotinin, a serine protease inhibitor that prevents the lysis of fibrinogen by inhibiting plasmin, kallikrein, and leukocyte elastase, is no longer available. EACA (Kang et al., 1987) and tranexamic acid prevent fibrinolysis by inhibiting plasminogen and plasmin, thus preventing the eventual degradation of fibrin. In addition, a significant heparin effect may be seen immediately on graft reperfusion for up to 30 minutes. This effect is caused by the release of endogenous heparinoids from the liver, as well as residual heparin from the preservation solution. Calcium is an important coenzyme in the coagulation cascade. During the dissection and anhepatic phases of liver transplantation, hypocalcemia may develop, especially when large amounts of fresh frozen plasma have been given. In many transplantation centers, continuous calcium infusions and magnesium supplements are routine therapy.
The approach to blood product replacement in children differs from that in adults because of two important concerns: thrombosis of the hepatic artery and postoperative hypercoagulability. Thrombosis of the hepatic artery is the most common serious complication after liver transplantation in children, so less fresh frozen plasma and platelets are routinely administered. After reperfusion, the hematocrit should ideally be maintained at 20% to 30% to minimize the increase in blood viscosity and to limit the risk for thrombotic complications (Tisone et al., 1988). Children may be at greater risk for hepatic artery thrombosis than adults because their arteries are smaller, and because of their postoperative hypercoagulable state (Heffron et al., 2003). The incidence of hepatic artery thrombosis has been reported in 15% of the mixed-age; pediatric series (Otte et al., 1990); however, it appears to be directly related to the size of the hepatic artery (smaller arteries and livers from neonates or infants having a higher incidence of this complication) (Mazzaferro et al., 1989; Jurim et al., 1995; Mas et al., 2003; Martin et al., 2004) and to technical complications during the vascular reconstruction. When a split liver is used, thrombosis is relatively rare (5% to 8%) and is similar to the adult rate (Gridelli et al., 2003). Thus, in some institutions, aggressive correction of coagulation is pursued only when there is diffuse bleeding after graft reperfusion or if monitoring reveals fibrinolysis that was not reversed by the new liver. Many transplantation centers use normalization of the PT and platelet count as indicators of recovery of donor liver graft function. Persistent hypothermia, coagulopathy with bleeding, increased serum lactate, hypocapnia, hyperkalemia, acidosis, absence of bile production, hyperglycemia, renal insufficiency, and hemodynamic instability may suggest suboptimal graft function.
After liver transplantation in children, levels of protein C and antithrombin III decrease to below 50% of normal and stay there for 10 days. These prolonged decreases are not seen in adults. Immediately after surgery, a 10-fold increase in plasminogen activator inhibitor occurs, with a further increase 6 to 9 days later. Therefore, in the immediate postoperative period, between days 4 and 10, children are at increased risk for thrombosis (Harper et al., 1988). To minimize this, anticoagulation therapy has been administered (IV heparin, dextran 40, aspirin, and antithrombin III) (Abengochea et al., 1995). There are also differences in coagulation between the infant and adult. In the normal neonate, there is a deficiency of vitamin K–dependent clotting factors (II, VII, IX, X) for several weeks. Protein C is significantly reduced for at least 6 months (Andrew et al., 1987), and protein S concentrations do not increase to within the normal adult range until 3 months of age (Donaldson et al., 1991). Protein C inhibits the function of factors VIII and V and enhances fibrinolysis, which is enhanced by protein S.
Hypothermia and hypocalcemia are known to contribute to coagulopathy (Kang, 1995a), and they affect platelet function and increase the incidence of fibrinolysis. Thus, temperatures below 35° C are best avoided. Finally, cell salvage may be beneficial in the patient undergoing OLT. Blood recovery of up to 30% is expected, but the need for rapid volume replacement limits its use. In addition, the use of the cell saver is contraindicated in patients with viral or bacterial infections, tumors, or FHF without an identifiable cause of the liver failure. Massive blood transfusion has an associated morbidity of more frequent bouts of sepsis, a prolonged stay in the intensive care unit (ICU), a higher rate of severe CMV infection, and higher rates of graft failure and patient mortality (Maurer and Spence, 2004).
Metabolic Function
Altered Glucose Metabolism
Hypoglycemia is rarely seen except in patients who present with acute FHF or have exhausted their liver glycogen stores. Patients may also be at risk during catecholamine infusions or if they require insulin for the management of hyperkalemia. During the anhepatic phase, several sources of glucose are available to the patient, such as packed RBCs (which contain a significant amount of free glucose: 84 mg/dL by day 35 of storage) (Miller, 2000), 5% dextrose in water (D5W, used as a carrier for drug infusions), and IV methylprednisolone (used for induction of immunosuppression during the portal anastomosis). Hyperglycemia after graft reperfusion is common because of glucose release from ischemic hepatocytes, steroid-induced insulin resistance, and decreased glucose metabolism in hypothermic patients (DeWolf et al., 1987). Thus, hourly monitoring of blood glucose levels is essential (Fig. 28-7).
Acute Ionized Hypocalcemia and Hypomagnesemia
Ionized hypocalcemia results from the rapid transfusion of large volumes of blood products containing citrate, especially during periods of hypothermia and acidosis. Citrate chelates calcium and magnesium and other divalent cations. Hypotension is the usual consequence and becomes significant at an ionized calcium level of 0.56 mmol/L, at which point the patient may develop cardiovascular collapse (Marquez et al., 1986; Martin et al., 1990).
The consequence of acute ionized hypomagnesemia (Scott et al., 1996) is not yet apparent, but it may result in a higher incidence of atrial arrhythmias and decreased myocardial contractility (Fig. 28-8).
Hypokalemia
Hypokalemia is the norm in chronic liver failure. It results from the use of diuretics, altered aldosterone metabolism, and metabolic alkalosis. If ventricular arrhythmias are noted during stages I and II, magnesium replacement is recommended. Potassium replacement is avoided because there is an expected transient twofold to threefold increase in potassium concentration immediately on graft reperfusion. Hypokalemia is common after graft reperfusion, usually as a result of diuresis, receptor stimulation with epinephrine, and uptake of potassium by the new liver (Xia et al., 2006). Cautious replacement during the biliary reconstruction phase is appropriate if the patient has no preexisting renal insufficiency and has evidence of adequate urine output.
Hyperkalemia should be treated aggressively at any point during the surgery. This electrolyte disturbance usually results from massive transfusion of RBCs, which have an estimated potassium content of 76 mEq/L by day 35 of cold storage, or from renal failure (Miller, 2000). The judicious use of diuretics as well as an infusion of glucose and insulin may be of benefit in reducing the potassium load (De Wolf et al., 1993). This is most crucial before revascularization of the donor liver. Postreperfusion hyperkalemia (7 to 12 mEq/L) is transitory, with redistribution occurring in 1 to 2 minutes, which seldom needs pharmacologic intervention other than prophylactic sodium bicarbonate and calcium intravenously (Nakasuji and Bookallil, 2000). Washed RBCs prevent the further development of this problem. When hyperkalemia is life threatening, the use of venovenous hemofiltration or removal of part of the patient’s blood volume and washing by the cell saver may be beneficial.
Hyponatremia and Hyperosmolarity
Dilutional hyponatremia is common and worrisome at serum sodium levels less than 125 mEq/L. Central pontine myelinolysis (CPM) is a frequently symmetric, noninflammatory demyelinating disorder in the brainstem pons. In at least 10% of patients, demyelination also occurs in extrapontine areas. Clinical manifestations are postoperative confusion or weakness, or a “locked-in” syndrome, after transplantation (Estol et al., 1989; Wszolek et al., 1989). The most frequent findings are delirium, pseudobulbar palsy, and spastic quadriplegia, which may result in permanent neurologic deficits. CPM occurs inconsistently as a complication of severe and prolonged hyponatremia, particularly when the sodium is corrected too rapidly. A study by Singh and coworkers (1994) demonstrated that CPM was present in 29% of postmortem examinations of adults after liver transplantation. Risk factors included serum sodium less than 120 mEq/L for more than 48 hours, aggressive IV fluid therapy with hypertonic saline solutions, and hypernatremia during treatment.
Empirical data show that CPM is likely to occur when the total perioperative increase in sodium concentration is above 15 to 20 mEq/L (Estol et al., 1989; Yu et al., 2004). In these patients, the choice of crystalloid solution should be Plasmalyte or 0.45% NaCl. Hyperosmolality has also been considered a contributor to the development of CPM. In the presence of either of these metabolic derangements, the judicious use of tris(hydroxymethyl)aminomethane (THAM) is recommended because its osmolality is 308 mOsm/L, whereas that of sodium bicarbonate is 2000 mOsm/L. In addition, the sodium content of bicarbonate buffer is 8.4% compared with 0.9% NaCl in IV solutions.
Pulmonary Function
Factors that may worsen oxygenation during the surgical procedure include clot or air emboli, progression of ARDS, transfusion-related acute lung injury, HPS, and pulmonary edema from excessive fluid administration. Finally, extubation of the patient at the end of the procedure is not recommended, no matter how short the duration of the surgery, because 50% to 55% (McAlister et al., 1993) of patients develop right diaphragmatic paralysis postoperatively and 25% will have pleural effusions, which compromise pulmonary function. The fast-track approach to anesthesia care may reduce the requirement for postoperative mechanical ventilation, but it does not reduce the length of stay in the ICU after liver transplantation (Findlay et al., 2002).
Renal Function
Renal insufficiency, a common finding in patients undergoing OLT, contributes significantly to postoperative morbidity, and it is an independent predictor of postoperative mortality (Gonwa, 2005). The patient who comes to the operating room with hepatorenal syndrome or ATN should not be expected to show improvement of renal function until after the graft functions (McCauley et al., 1990). Information collected by the National Institute of Diabetes and Digestive and Kidney Diseases Liver Transplantation Database support the conclusion that renal insufficiency in patients with FHF and in those requiring preoperative dialysis or liver-kidney transplantation for cirrhosis predicts lower posttransplantation patient and graft survival rates (Brown et al., 1996).
A progressive decline in urine output is expected, with little or none during the anhepatic portion of the surgery without preservation of the IVC or use of VVB. This physiologic alteration has been thought to be secondary to congestion of the kidneys from increased renal venous pressures with total cross-clamping of the infrahepatic IVC (Gunning et al., 1991). Whether the incidence of postoperative renal insufficiency is as frequent when the piggy-back technique is used remains unclear. Thus, the judicious use of the diuretics (mannitol and furosemide) is recommended in the patient who develops oliguria (Polson et al., 1987). It should be noted that furosemide may be ineffective in the patient with a low albumin level (average albumin in the patient with liver failure for OLT is 2.5 to 1.8 mg/dL). Although a renal dosage of dopamine not exceeding 1.5 mcg/kg per min is often recommended, there is no clear benefit from its use as a renal protective agent (Polson et al., 1987; Gray et al., 1991; Swygert et al., 1991; Kellum and Decker, 2001). The incidence of renal complications postoperatively in all patients undergoing OLT remains the same with or without the use of VVB or diuretic therapy (Schwarz et al., 2001; Cabezuelo et al., 2003). Acute renal insufficiency is estimated to occur after liver transplantation in up to 67% of recipients (Rimola et al., 1987). Contributing factors include preexisting renal insufficiency, intraoperative complications (suboptimal renal perfusion associated with hypotension, massive transfusion, increased caval pressures), early graft dysfunction, sepsis, and administration of cyclosporine or tacrolimus and other nephrotoxic drugs in the immediate posttransplantation period.
Graft Reduction and Split-Liver Transplantation
With the plateau in organ availability in the past 5 years and the increased number of indications for OLT, creative techniques have been developed to increase organ availability to pediatric patients. Three techniques, living related (see Living Related Organ Donor, p. 900), split livers, and graft reduction, now account for up to 30% of all pediatric OLTs in some centers. See related video online at www.expertconsult.com.
Reduced-size liver transplantation (graft reduction) was first performed by Bismuth and Houssin (1984; Broelsch et al., 1988). This procedure involves the dissection of the graft at the back table with preparation of either the right lobe, the left lobe, or the left-lateral segment graft, depending on the size of the recipient. With a recipient-to-donor size ratio of 1:2, a right lobe graft is chosen. Similarly, if the recipient-to-donor size ratio is 1:4 or 1:8 (Thistlethwaite et al., 1991), a left lobe graft or a left-lateral segment graft is chosen, respectively. Because this technique, as with split livers, requires the preparation of the graft at the back table, it lends itself to an increased cold ischemic time of the liver. Vascular anastomoses for this procedure are usually of the end-to-end type, with the occasional need for a piggy-back technique or the direct anastomosis of the hepatic veins to the vena cava.
In split-liver transplantation, the liver is divided into left and right lobes and transplanted into two recipients (Emond et al., 1990; Otte et al., 1990; Renz et al., 2004) (Fig. 28-9). As a result, the right lobe contains the portal vein, hepatic artery, IVC, and common bile duct, and the left lobe includes the left lobar branches of the vessels and the left hepatic duct. Transplantation of the left lobe may necessitate the use of interposition vascular grafts, whereas the right lobe is transplanted in a fashion similar to that used for the whole organ. Most frequently, biliary reconstruction is by the Roux-en-Y technique because of the size discrepancy of the donor and recipient bile ducts.
Living related liver transplantation from parent to child, described in 1989 by Broelsch and coworkers (1991), has the potential advantages of increasing the availability of organs, reducing the waiting times and deaths on the recipient waiting list, allowing medical optimization of the recipient while minimizing the risk for clinical deterioration, and limiting immunosuppressive therapy by decreasing the immunogenicity of the transplanted organ. In addition, societies whose criteria for death differ from those previously described (Tanaka et al., 1993) are now able to offer organ transplantation in a socially acceptable manner. Until 1998, the majority of all living donor grafts in the United States went to children under 1 year of age. Living donation is not without risk. Mortality among healthy donors is low, but complications in the donor are relatively common. These findings then raise the issues of safety and ethics. Potential risks to the donor include transfusion complications, biliary complications, negative psychosocial aspects (out-of-pocket expenses, loss of income, stress on the donor and donor family, change in body image, inability to work for at least 4 to 8 weeks after donation, and poor recipient outcome), and death. Up to 20% of donors experience complications related to major abdominal surgery, including pneumonia, atelectasis, wound infection, small-bowel obstruction, incisional hernia, pressure ulcers, phlebitis, neuropraxia or peroneal nerve palsy, and reoperation. Until 1994, all living-donor liver transplantations were performed with a left-lateral segmentectomy of one or more lobes, or with a left lobectomy (20% of liver) from parent to child, or adult to small adult. Adult-to-adult transplantation of the right hepatic lobe (60% of liver) was first reported in Japan in 1994. An estimated 5% of patients on the transplant waiting list would be able to identify a suitable donor, resulting in approximately 750 living-donor liver cases in the United States each year. Adult-sized pediatric patients undergoing adult-to-adult living-donor liver transplantation may require a right hepatic lobe to provide sufficient liver mass for the recipient. The donor is left with approximately half or less of their hepatic mass after hepatectomy, but because of the large functional reserve of the liver and its regenerative capacity, clinical evidence of hepatic insufficiency is rare. Prolonged PT, elevated serum aminotransferase levels, and increased bilirubin levels normalize after 1 week. The donor’s native liver can regenerate to its original size within several weeks. The greatest concern for donor safety is the risk for donor death, which has been estimated to be between 0.28% and 2% to 3%, with ten reported cases caused by technical errors, sepsis, and pulmonary embolism (Hayashi and Trotter, 2002; Trotter et al., 2002). The actual mortality rates are probably higher than those reported.
All three of these techniques have associated complications, with split-liver grafts having the highest rates of associated complications and mortality. Because the integrity of the transected surface of the liver cannot be fully assessed until after reperfusion of the graft, these procedures have a greater associated blood loss than whole organ transplantation (Moreno et al., 1991). In addition, bile duct leaks and seroma formations are not uncommon in the transected liver. Although this specific complication was initially associated with split-liver transplantations, alterations of the surgical techniques have resulted in bile leak complications similar to those seen with reduced-size liver transplantation.
The incidence of hepatic artery and portal vein thrombosis is clearly reduced with the use of these three techniques. Survival of grafts and of patients at 1 year is better after living related liver transplantation (81%) (Epstein, 1985) than after split-liver transplantation (67%) (Broelsch et al., 1991). However, overall 5-year survival rates with all these procedures are similar.
Outcomes
In the 2006 report from Studies of Pediatric Liver Transplantation, Ng and colleagues (2008) reported on a cohort of 5-year survivors for whom the 1- and 5-year Kaplan Meier estimates of survival rates were 89.8% and 84.8%, respectively. Among the 5-year survivors, first allograft survival rates at 1, 3, and 5 years were 93%, 90%, and 88%, respectively. There was no difference in graft survival by donor type (Figs. 28-10 and 28-11). However, Diamond and colleagues (2007) reported that graft survival in 2192 children undergoing liver transplantation was decreased, and the relative risk for graft loss was increased in all of the technical variants compared with whole-organ recipients (Table 28-19, and Figs. 28-12 and 28-13). Survival also appears to be influenced by the underlying disease (Fig. 28-14).
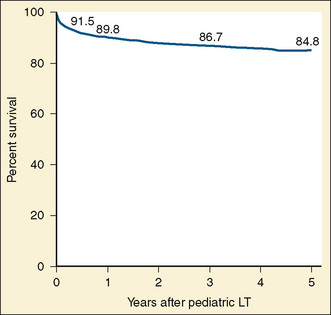
FIGURE 28-10 Kaplan-Meier probability of patient survival after pediatric liver transplantation.
(From Ng VL, Fecteau A, Shepherd R, et al: Outcomes of 5-year survivors of pediatric liver transplantation: report on 461 children from a North American multicenter registry, Pediatrics 122:e1128, 2008.)
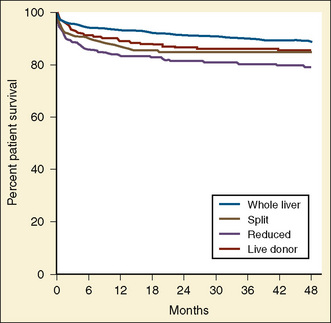
FIGURE 28-12 Kaplan-Meier probability of patient survival after pediatric liver transplantation.
(From Diamond ER, Fecteau A, Millis JM, et al: Impact of graft type on outcome in pediatric liver transplantation, Ann Surg 246:305, 2007.)
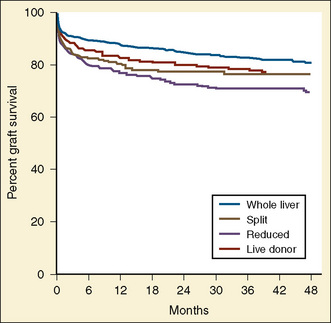
FIGURE 28-13 Kaplan-Meier probability of graft survival pediatric liver transplantation.
(From Diamond ER, Fecteau A, Millis JM, et al: Impact of graft type on outcome in pediatric liver transplantation, Ann Surg 246:305, 2007.)
Early studies in children undergoing OLT for a variety of conditions reported that infants less than 1 year of age had lower graft and patient survival rates than older children. However, recent trends suggest that survival rates for younger patients now approach those for older children. In children undergoing OLT for metabolic reasons, 1-, 5-, and 10-year survival rates for children older than 1 year were better than for children less than 1 year of age (Sze et al., 2009).
In a single-center study of 638 children receiving 745 grafts, Bourdeaux and coworkers (2009) noted in their 21-year experience that single liver transplantations had 5- and 10-year patient survival rates of 86% and 85%, respectively, versus 66% and 61% for recipients who needed to be retransplanted. Not surprisingly, these authors also noted that the era in which the child received the transplant also affected survival. Patients recently transplanted had better survival rates and less need to be retransplanted than patients who received their transplant in an earlier era (Fig. 28-15). The rate of retransplantation was less (3%) for patients who received a living-donor graft than for children who received a whole (15%), reduced-size (20%), or split (12%) liver at first transplantation (Bourdeaux et al., 2009).
With improved outcomes, the population of survivors has grown, and the number of survivors is more than 10-fold greater than the number of children and adolescents who undergo liver transplantation each year (Ryckman et al., 2008). Thus, the study of outcomes should focus on the side effects of immunosuppression and on quality of life issues, in addition to patient and graft survival and surgical technical issues.
Hepatic artery thrombosis (HAT) and portal vein thrombosis (PVT) are the most common vascular complications after liver transplantation, reported to occur after 4% to 15% of liver transplantations, and more frequently after pediatric liver transplantations. Factors associated with HAT include dissection of the hepatic arterial wall, technical imperfections, celiac stenosis, aberrant donor or recipient arterial anatomy, complex back-table arterial reconstruction, and organ rejection (Duffy et al., 2009). In pediatrics, type of organ graft, whole versus split, and deceased versus living donor, as well as age less than 1 year, also affect the incidence of HAT. Overall, HAT decreases both patient and graft survival.
PVT occurs about 2% of the time but is usually more detrimental to patient and graft survival. As with HAT, pediatric patients are at greater risk than adult patients. Risk factors for PVT include small portal vein size, portal vein redundancy, pretransplantation PVT, and prior splenectomy (Duffy et al., 2009). Type of graft may also influence the incidence (Diamond et al., 2007). Life-long immunosuppression with reduction in therapy remains the practice. Thus, immunosuppression balances the preservation of graft function with its side effects. Posttransplant lymphoproliferative disease occurs in 3% to 28% of children after liver transplantation. Nephrotoxicity is a well-known complication of the immunosuppressive agents. Chronic renal disease increases with increased survival. Renal insufficiency was reported as 33% and 77% in 3- and 10-year survivors, respectively (Starzl and Kaupp, 1960).
Growth is also adversely affected by both disease and immunosuppressive therapy. In children, approximately 73% were below average in height and 29% below the 10th percentile (Ng et al., 2008). Quality-of-life issues for children frequently focus on school performance. Ng reported that almost 18% of 5-year survivors had either repeated a grade or been held back more than one school year. Learning disabilities were reported in 21% of 5-year survivors.
Multivisceral and intestinal transplantations
The technical feasibility of intestinal transplantation was first described in 1905 by Alexis Carrel (1905). After early successes with kidney transplantation in identical twins during the early 1960s, Lillehei and colleagues (1967) described experimental transplantation of the stomach, intestine, and pancreas. Simultaneously, Starzl and colleagues described transplantation of multiple abdominal viscera in dogs and, many years later, in humans (Starzl et al., 1989). Until the late 1980s, there were no long-term (>6 months) survivors of small bowel transplantation (SBT). With the introduction of cyclosporine and tacrolimus, and with an improved understanding of graft rejection in pigs, successful long-term survival became possible (Grant et al., 1990). This procedure has a high rate of morbidity and mortality. The major cause of graft failure is acute or chronic rejection (SBT, 79%; small bowel in combination with liver transplantation [SB/LT], 71%; multivisceral transplantation, 56%). Infection, CMV enteritis, lymphoproliferative disease, and multisystem organ failure are major causes of death. The rate of graft-versus-host disease after intestinal transplantation is 0% to 16% (Abu-Elmagd et al., 1998; Reyes et al., 1998; Sudan et al., 2000; Pirenne et al., 2007). Patient and graft survival rates are lower for intestinal transplantation than for solid organ transplantation, with approximately half of the patients receiving intestinal transplants surviving 5 years.
Multivisceral transplantation may include SBT in isolation, in combination with liver transplantation, or in combination with multiple abdominal organs (Starzl et al., 1991a, 1991b). An isolated SBT is typically recommended for patients on home parenteral nutrition (HPN) who have exhausted their venous access. Combined SB/LT is recommended for patients with irreversible cirrhosis when HPN has failed, or those who have intestinal failure associated with a hypercoagulable state that can be corrected by a simultaneous liver transplantation. Multivisceral transplantations are for patients with locally aggressive tumors that necessitate evisceration of the abdominal organs (duodenal fistulae, locally aggressive tumors, multiorgan failure with a nonreconstructible GI tract) or for the patient with benign conditions involving the liver, pancreas, or stomach (Tzakis et al., 1989).
General Indications
In the pediatric population, chronic intestinal failure results from massive bowel resection (short bowel syndrome) or functional impairment of the bowel caused by disturbances in motility or extensive parenchymal disorders (Okada et al., 1994). Necrotizing enterocolitis, intestinal atresia (Todo et al., 1994a, 1994b), midgut volvulus, gastroschisis, microvillous inclusion disease, Hirschsprung’s disease, and intestine pseudoobstruction are the primary causes of isolated small intestinal failure. This is in marked contrast to the adult population, in which intestinal failure is caused primarily by Crohn’s disease and thrombotic episodes involving the major splanchnic vessels. If the length of the small bowel is less than 60 or 70 cm, parenteral nutrition is required (normal length of a small bowel in the infant being 200 to 250 cm). For the patient to survive, HPN is essential. HPN has significant metabolic sequelae, the foremost being liver failure and bone disease (Bowyer et al., 1985; Colomb et al., 1994; Sondheimer et al., 1998; Chan et al., 1999; Wasa et al., 1999; Cavicchi et al., 2000). The hepatic dysfunction in the patient on long-term TPN most commonly includes hepatic steatosis, as well as cholestasis, phospholipidosis, or cirrhosis, which is observed in 15% to 40% of patients after 3 years. Up to 100% of patients have biliary sludge or gallstones after 6 weeks. In the pediatric population, this is a reversible form of liver dysfunction (Grosfeld et al., 1986) if the bilirubin level is less than 30 mg/dL and the patient is returned to total enteral feeding. Patients are followed through the North American Home Parenteral and Enteral Nutrition Patient Registry supported by the Oley Foundation (formerly the OASIS registry, started in 1984) (Howard et al., 1991). This registry reports survival for patients on long-term HPN to be 87% to 96% at 1 year, and 70% to 90 % at 3 years. The majority of deaths are related to progression of the underlying disease.
Preoperative Assessment
The patient should be evaluated specifically for progressive liver disease and evidence of portal hypertension. Extrahepatic manifestation of the primary disease causing the patient’s intestinal failure should be identified. Complications of TPN should be elucidated (infection, catheter occlusion, hepatic disease). Comorbid conditions such as infection, renal disease, electrolyte abnormalities, and gastroparesis predisposing to regurgitation and aspiration should be considered. Appropriate evaluation of patency of the large vessels of the neck (subclavian and internal jugular veins) is essential, because repeated central vein cannulation or long-term indwelling catheter use for the administration of HPN may lead to loss of vascular access resulting from venous thrombosis (Grosfeld et al., 1986). Doppler ultrasonography is sometimes useful to find central venous access, but the gold standard is a venogram.
Surgical Technique: Recipient Surgery
The final decision to proceed with either a multivisceral or an intestinal transplantation is made after a laparotomy and a meticulous inspection of the native vessels and abdominal organs. Thus, continuous communication between the donor and recipient teams is essential. In addition, procurement of the donor iliac artery and vein (Starzl et al., 1991a, 1991b), as well as the thoracic aorta, is essential for vascular reconstruction of the graft. The graft is usually preserved with cold UW solution. The length of time the organ is kept in the preservation solution should be minimized because intestinal mucosal damage occurs in UW solution as a result of increased lipid peroxidation (Takeyoshi et al., 2001).
The operation begins with a midline incision, which is extended to either a unilateral or bilateral transverse subcostal incision. The choice of incision depends on the planned operation, with a bilateral incision used preferentially in the multivisceral transplantation. The procedure continues as described by Todo and coworkers (1992) (Fig. 28-16). In the patient receiving an isolated intestinal transplantation, the superior mesenteric artery is anastomosed exclusively to the infrarenal aorta. Venous blood from the isolated small bowel graft is drained into the mesenteric venous system at one of three sites: the donor SMV to the distal end of the recipient SMV, SMV to the hilar portion of the main portal vein, or donor SMV to the confluence of the SMV and splenic vein (Todo et al., 1994a, 1994b).
In the recipient of liver-intestine or multivisceral graft, the use of VVB is usually impossible because of vascular thromboses of the major vessels seen with the long-term use of HPN. The liver is usually placed in a piggy-back fashion, and occasionally a temporary portacaval shunt is used to decompress the abdominal viscera. Vascular anastomoses are performed as illustrated in Figure 28-16.
The arterial and venous anastomoses are performed before reperfusion of the graft. Arterial anastomoses in this instance are usually with a Carrel patch (Fig. 28-17), containing both the celiac and superior mesenteric arteries for the combined intestinal and multivisceral graft to the infrarenal abdominal aorta. In some instances, an aortic conduit may be used to facilitate the anastomoses of the superior mesenteric artery and celiac axis to the abdominal aorta. If a portacaval shunt is performed, it is subsequently converted to a portaportal anastomosis to facilitate perfusion of the liver with the splanchnic hepatotropic factors.
Anesthesia Management
Multivisceral Transplantation
Anesthesia considerations for the patient scheduled for multivisceral transplantation are identical to those for the patient undergoing OLT. There are, however, unique and specific considerations with respect to planned vascular anastomoses (Starzl et al., 1993; Todo et al., 1995) (see Fig. 28-16). The liver is usually placed in piggy-back fashion in this instance. A partial cross-clamping of the abdominal aorta should be anticipated for the placement of the arterial anastomosis using a Carrel patch (see Fig. 28-17). The surgical procedure is divided into three stages, similar to those with solitary liver transplantation. Completion of the abdominal viscera exenteration during the preanhepatic stage is the period of greatest blood loss (De Wolf, 1991). Hemodynamic alterations during this period are related to complications of massive transfusion and manipulation of the abdominal viscera. Ionized hypocalcemia, lactic acidosis, and progressive hypothermia should be anticipated. In addition, a progressive coagulopathy, as seen in patients undergoing OLT, is expected. Thus, monitoring of coagulation is essential. The anhepatic stage of the surgery is usually performed without the use of VVB. The liver is placed in piggy-back fashion, and vascular anastomosis of the intestine follows. A significant difference with respect to reperfusion of the multivisceral graft from that of the liver is that the arterial anastomoses must be performed before graft reperfusion. The graft is flushed with 50 mL/kg of cold saline or lactated Ringer’s solution before reperfusion, to reduce the potassium load from the preservation solution.