Chapter 13 Visual Pathway
The visual pathway consists of the series of cells and synapses that carry visual information from the environment to the brain for processing. It includes the retina, optic nerve, optic chiasm, optic tract, lateral geniculate nucleus (LGN), optic radiations, and striate cortex (Figure 13-1). The first cell in the pathway—a special sensory cell, the photoreceptor—converts light energy into a neuronal signal that is passed to the bipolar cell and the amacrine cell and then to the ganglion cell; all these cells and synapses lie within the retina. The axons of the ganglion cells exit the retina via the optic nerve, with the nasal fibers from each eye crossing in the optic chiasm and terminating in the opposite side of the brain. The optic tract carries these fibers from the chiasm to the LGN, where the next synapse occurs. The fibers leave the LGN as the optic radiations that terminate in the visual cortex of the occipital lobe. From various points in this pathway, information about the visual environment is transferred to related neurologic centers and to visual association areas.
This chapter discusses the structures of the visual pathway and orientation of the fibers within each structure, then briefly reviews characteristic field defects associated with specific locations in the visual pathway. Most of the current knowledge of the visual pathway is based on degeneration studies using laboratory animals, particularly monkeys and cats.1–3 This type of investigation is based on the finding that damage to a neuron causes the cell and its processes to degenerate. After a small area of nerve tissue is damaged, researchers make serial sections of the tissue through which the neuronal processes are believed to pass. By examining these sections under the microscope, they identify the pathway by determining the location of the degenerating processes. In some studies, small lesions were made in the retina, and the degeneration was followed through the optic nerve, chiasm, and tract into the LGN.1,3 In other studies, lesions were made in the striate cortex, and the degeneration was followed through the optic radiations toward the LGN.2 Whenever possible, reference to studies on the human pathway are cited.
Anatomy of Visual Pathway Structures
The anatomy of the retina and optic disc are discussed in Chapter 4.
Optic Nerve
The retinal nerve fibers make a 90-degree turn at the optic disc and exit as the optic nerve. This nerve consists of visual fibers, 90% of which will terminate in the LGN. Approximately 10% project to areas controlling pupil responses or the circadian rhythm.4 Various counts of the optic nerve fibers range from 1 million to 2.22 million, with their size ranging from small-diameter macular fibers to larger-caliber extramacular fibers.1,2,5–7
The nerve is 5 to 6 cm long and can be divided into four segments on the basis of location: intraocular (0.7 to 1 mm), intraorbital (30 mm), intracanalicular (6 to 10 mm), and intracranial (10 to 16 mm).6,8,9
The intraocular section of the optic nerve can be divided into prelaminar and laminar sections on the basis of association with the lamina cribrosa. In the prelaminar optic nerve, a glial tissue network provides structural support for the delicate nerve fibers; sheaths of astrocytes bundle the nerve fibers into fascicles, containing approximately 1000 fibers each.8 The optic nerve fibers are separated from the retinal layers by a ring of glial tissue, the intermediary tissue (of Kuhnt). The continuation of this glial tissue, the border tissue (of Jacoby), separates the choroid from the optic nerve fibers, and a ring of collagenous tissue of scleral derivation, the marginal (or border) tissue (of Elschnig), lies outer to the glial sheaths.8 Tight junctions within the glial border tissue may prevent leakage from adjacent choriocapillaris into the optic nerve head.10 These layers are shown in Figure 13-2.
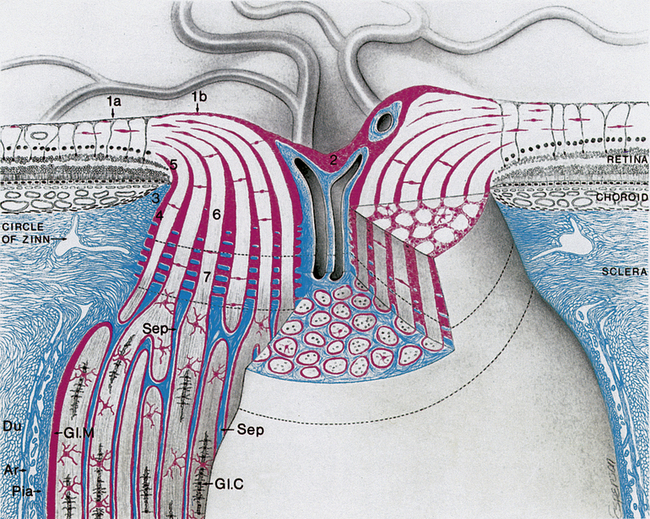
FIGURE 13-2 Intraocular and part of orbital optic nerve.
(From Anderson D, Hoyt W: Ultrastructure of interorbital portion of human and monkey optic nerve, Arch Ophthalmol 82:506, 1969.)
The intraorbital (postlaminar) length exceeds the distance from the globe to the apex of the orbit, giving the nerve a slight sine wave-shaped curve, allowing for full eye excursions without stretching the nerve.6,11 Within the orbit, the nerve is surrounded by the rectus muscles; the sheaths of the superior and medial rectus muscles are adherent to the sheath of the optic nerve (which explains the pain associated with eye movements in optic neuritis).8
The optic nerve is surrounded by three meningeal sheaths continuous with the meningeal coverings of the cranial contents. The outermost sheath, the dura mater, is tough, dense connective tissue containing numerous elastic fibers.8 Next to it, the thin collagenous membrane of the arachnoid sends a fine network of trabeculae through the subarachnoid space and connects to the innermost layer, the pia mater. The subarachnoid space around the optic nerve is continuous with the intracranial subarachnoid space and contains cerebrospinal fluid. The loose, vascular connective tissue of the pia mater branches, sending blood vessels and connective tissue septa into the nerve (see Figure 13-2). All three of these layers fuse and become continuous with the sclera and with the periorbita.8 Of these sheaths, only the pia continues along the intracranial optic nerve.10
As the unmyelinated retinal fibers pass through the scleral perforations of the lamina cribrosa, they become myelinated by oligodendrocytes because no Schwann cells exist in the central nervous system. It is postulated that the lamina cribrosa is a barrier to oligodendrocytes because these cells are not located in retinal tissue and myelination does not normally occur in the retina.10 The sheath of connective tissue branching from and continuous with the pia mater meningeal covering is added to the glial sheath of each fascicle posterior to the lamina (Figure 13-3). These additional tissues double the diameter of the optic nerve as it leaves the eye; the nerve is approximately 1.5 mm in diameter at the level of the retina and 3 mm after its exit from the globe. The septa that separate the fiber fascicles end near the chiasm.8 Astrocytes present in the optic nerve probably function similar to Müller cells of the retina; they provide structure, store glycogen, and regulate the extracellular concentration of certain ions.10
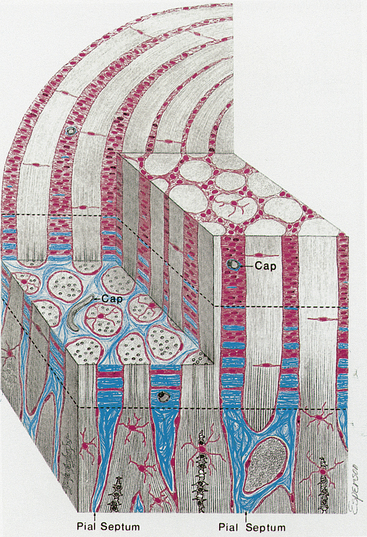
FIGURE 13-3 Prelaminar, laminar, and postlaminar optic nerve.
(From Anderson D: Ultrastructure of human and monkey lamina cribrosa and optic nerve head, Arch Ophthalmol 82:800, 1969.)
The anterior perforated substance, the root of the olfactory tract, and the anterior cerebral artery lie superior to the optic nerve in its intracranial path. The sphenoid sinus is medial, with only a thin plate of bone separating it from the nerve.8 The internal carotid artery is below and then lateral to the nerve, and the ophthalmic artery enters the dural sheath of the optic nerve as it passes through the optic canal.
Optic Chiasm
The optic chiasm is roughly rectangular, approximately 15 mm in its horizontal diameter, 8 mm anterior to posterior, and 4 mm high.6,9,12 As with the optic nerve, the optic chiasm is surrounded by the meningeal sheaths and cerebrospinal fluid.
The chiasm lies within the circle of Willis, a circle of blood vessels that is a common location for aneurysms.6 The circle of Willis is an anastomotic group of anterior and posterior arteries that join the anterior circulation of the internal carotid arteries with the posterior circulation of the basilar artery (Figure 13-4). The internal carotid arteries supply the anterior cranial regions, including most of the cerebral hemispheres and orbital and ocular structures. The vertebral branches of the basilar artery supply the posterior regions, including the brainstem, occipital lobes, and inferomedial temporal lobes, thus supplying most of the ocular motor centers and the cortical visual areas.13 If the circle is complete, the anterior cerebral arteries are joined via the anterior communicating artery, and each internal carotid artery is joined to the ipsilateral posterior cerebral artery by a posterior communicating artery. The anterior cerebral and anterior communicating arteries are anterior to the chiasm, and an internal carotid artery lies on each lateral side of the chiasm.
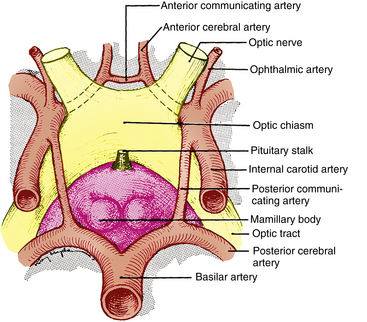
FIGURE 13-4 Relationship of optic chiasm to vessels of circle of Willis.
(From Harrington DO: The visual fields, ed 5, St Louis, 1981, Mosby.)
Above the optic chiasm is the floor of the third ventricle, and approximately 1 cm below the chiasm is the pituitary gland (Figure 13-5). The position of the optic chiasm above the sella turcica (the fossa in which the pituitary gland sits) can vary from being directly above it (in 75% of the population) to a position referred to as prefixed (if the optic nerves are short and the gland lies below the posterior part of the chiasm) or postfixed (if the optic nerves are long and the gland is situated toward the anterior of the chiasm).12 The chiasm is anteriorly displaced in approximately 10% of individuals and posteriorly displaced in 15%.10
Optic Tract
The optic tract is a cylindric, slightly flattened band of fibers approximately 3.5 mm high and 5.1 mm long that runs from the posterolateral corner of the optic chiasm to the LGN.9 Most of the fibers (which are still the axons of retinal ganglion cells) terminate in the LGN. Fibers from the retinal ganglion cells may branch so that the same cell sends fibers to various target structures or some axons may be destined for a specific structure. The afferent fibers of the pupillomotor reflex leave the optic tract before reaching the LGN and pass by way of the superior brachium to the pretectal nucleus in the midbrain. Other fibers project to areas in the hypothalamus involved with the circadian rhythm, and others terminate in the superior colliculus. The rather poorly defined accessory optic system, including the nucleus of the optic tract, is involved in the optokinetic nystagmus response and receives information generated by retinal ganglion cells.4
The optic tract lies along the upper anterior and then the lateral surface of the cerebral peduncle and is parallel to the posterior cerebral artery. The globus pallidus is above, the internal capsule is medial, and the hippocampus is below the optic tract.8
Lateral Geniculate Nucleus
Information from all the sensory systems except the olfactory pass through the thalamus before being transferred to the cerebral cortex; visual information is processed in the LGN and then is relayed to higher cortical centers.14 The lateral geniculate nucleus (LGN, lateral geniculate body) is located on the dorsolateral aspect of the thalamus and resembles an asymmetric cone, the rounded apex of which is oriented laterally. The retinal axons terminate here. Most of the fibers that leave the LGN project to the visual cortex.
The LGN is a layered structure; the layers are piled on each other, with the larger ones draping over smaller ones, and some layers becoming fragmented and irregular. The cells within a layer are all of the same type, and three types have been identified according to size. Magnocellular layers contain large cells, parvocellular layers contain medium-sized cells, and koniocellular layers contain small cells. The number of layers present depends on the location of the plane through the structure. In the classic textbook presentation of the LGN, six layers are seen. Two magnocellular layers are located inferiorly and numbered 1 and 2, and four parvocellular layers are above them and numbered 3, 4, 5, and 6 (Figure 13-6). Below each of these six layers lies a koniocellular layer (Figure 13-7). The retinal ganglion cells that project to each of these layers differ in a number of their characteristics.15
The LGN is not a simple relay station; it also receives input from cortical and subcortical centers and reciprocal innervation from the visual cortex and is a center of complex processing.6,16 It regulates the flow of visual information, ensuring that the most important information is sent to the cortex.17 The optic tract enters the LGN anteriorly; the internal capsule is lateral, the medial geniculate nucleus is medial, and the inferior horn of the lateral ventricle is posterolateral to the LGN.8 The axons leave the LGN as the optic radiations.
Optic Radiations (Geniculocalcarine Tract)
The optic radiations spread out fanwise as they leave the LGN, deep in the white matter of the cerebral hemispheres, sweeping laterally and inferiorly around the anterior tip of the temporal horn of the lateral ventricle (Figure 13-8). Some fibers loop into the temporal lobe en route to the occipital lobe. The fibers within the parietal lobe pass lateral to the occipital horn of the lateral ventricle before terminating in the striate cortex.8,18
Primary Visual Cortex (Striate Cortex)
The primary visual cortex (Brodmann area 17 or, according to more recent nomenclature, V1), is located almost entirely on the medial surface of the occipital lobe; just a small portion (perhaps 1 cm long) extends around the posterior pole onto the lateral surface. The visual cortex also is called the striate cortex because a white myelinated fiber layer, the white stria of Gennari, is characteristic of this area.6 The calcarine fissure extends from the parieto-occipital sulcus to the posterior pole, dividing the visual cortex into an upper portion (the cuneus gyrus) and a lower part (the lingual gyrus) (Figure 13-9); most of the primary visual cortex is buried in the tissue within the calcarine fissure.19
The primary visual cortex has a thickness of about 2 mm and is organized into horizontal layers and vertical columns. Layer I, the most superficial layer, contains a few scattered neurons. Layer II contains neurons that send axons only to deeper cortical layers. Layer III contains neurons that communicate with both near and far cortical locations. Layer IV contains the stria of Gennari and is subdivided into strata, one of which receives information from the magnocellular layers and another that receives information from the parvocellular layers.20,21 Layer IV sends axons to more superficial visual cortex, as well as other visual cortical areas. Layer V sends axons to the superior colliculus and other areas in the brainstem. Layer VI sends projections back to the LGN.17
Certain cortical regions are active during motion stimulation, whereas others are active during color vision.22 The magnocellular areas probably mediate movement detection and low-spatial-frequency contrast sensitivity, and the parvocellular areas likely mediate color and high-spatial-frequency contrast sensitivity, although this generalization oversimplifies the properties.23–26
Cells are also distributed in a vertical organization, according to the eye of origin, forming alternating parallel ocular dominance columns.21,27,28 These columns are lacking in the area of the cortex that represents the physiologic blind spot because this region receives information exclusively from one eye.14 A second system of columns, specific for stimulus orientation, responds on the basis of the direction of a light slit or edge.21 Contour analysis and binocular vision are two functions of the visual cortex, and such processing is a function of both its horizontal and its vertical organization. The cells within the striate cortex are activated only by input from the LGN, although other cortical areas have input into the striate cortex.16,29,30 The striate cortex communicates with the superior colliculus and the frontal eye fields.
The superior colliculus, which has a complete retinotopic map of the contralateral field of vision, also receives communication from fibers exiting the posterior optic tract. It does not analyze sensory information for perception but is important for visual orientation, foveation, and the control of saccadic eye movements with input from the frontal eye fields.14,31 The frontal eye fields, in the frontal lobe, receive fibers from the striate cortex that contribute to the control of conjugate eye movements. Both voluntary and reflex ocular movements are mediated in this area, as are pupillary responses to near objects (see Chapter 14).8
The striate cortex combines and analyzes the visual information relayed from the LGN and transmits this information to the higher visual association areas (the extrastriate cortex), which provide further interpretation.14 These areas surround the striate cortex and are located on the lateral aspects of the occipital cortex. Historically called Brodmann areas 18 and 19 (Figure 13-10), these areas now are known to contain several distinct cortical areas (designated V2, V3, V4, and V5) in which visual processing occurs. A study involving the macaque monkey has identified 32 such areas associated with visual processing.14 The visual and visual association areas in one hemisphere are connected to the corresponding areas in the other hemisphere through the posterior portion of the corpus callosum.6 Magnetic resonance imaging (MRI) techniques that are sensitive to changes in blood flow and oxygenation occurring with neuronal activity can be used to study the human visual system in vivo. Innovative studies are attempting (1) to identify the areas of visual cortex and associated visual areas activated during visual stimulation and visual processing, (2) to detect the storage areas for learned visual patterns, and (3) to establish the pathway of activation in the cortex for recall and recognition of a visual pattern.32–38
Blood Supply to the Visual Pathway
The structures of the visual pathway have an extensive blood supply. Figure 13-11 shows many of the involved vessels. The outer retinal layers receive nutrition from the choroid, whereas the inner retina is supplied by the central retinal artery. The circle of Zinn, the anastomotic ring of branches of the short ciliary arteries, and peripapillary vessels supply the optic disc.6 Capillaries within the optic nerve are composed of nonfenestrated endothelium joined by zonula occludens, thus the vessels perfusing the nerve head are part of the blood-brain barrier.10,39 Pial vessels supply the optic nerve throughout its length; the intraorbital portion is supplied by branches from the ophthalmic artery, and the intracranial optic nerve is nourished by branches of the ophthalmic, anterior cerebral, anterior communicating, and internal carotid arteries.6,8
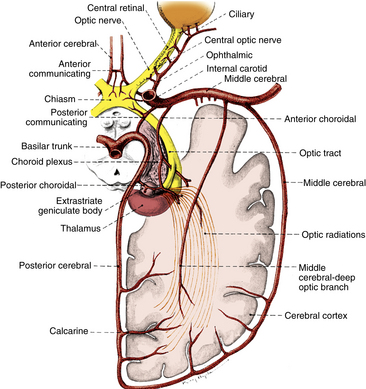
FIGURE 13-11 Vascular supply of visual pathway from retina to occipital cortex.
(From Harrington DO: The visual fields, ed 5, St Louis, 1981, Mosby.)
The blood supply to the optic chiasm is rich and anastomotic, with arterioles from the circle of Willis forming capillary beds at two levels.40,41 The superior network is supplied by the anterior cerebral and anterior communicating arteries, whereas the inferior network is supplied by the internal carotid, posterior cerebral, and posterior communicating arteries.6,8,12,13 The anterior choroidal artery, a branch of the internal carotid, is a primary supplier of the optic tract, although small branches from the middle cerebral artery also contribute.6,8,12,42,43 The blood supply to the LGN is derived from the anterior choroidal artery and the lateral choroidal and posterior choroidal branches of the posterior cerebral artery.8,42,44
The optic radiations can be divided into three sections: (1) the anterior radiations, which pass laterally over the inferior horn of the ventricle, are supplied by the anterior choroidal artery and the middle cerebral artery; (2) the middle group of fibers passing lateral to the ventricle is supplied by the deep optic branch of the middle cerebral artery; and (3) branches of the posterior cerebral artery, including the calcarine branch, supply the posterior radiations as they spread out in the occipital lobe. Branches from the middle cerebral artery also contribute.6,8,12 The calcarine branch of the posterior cerebral artery is the major blood supply for the striate cortex, often supplemented by the posterior temporal or parietooccipital branch of the posterior cerebral artery or the occipital branch of the middle cerebral artery.6,8,12,45
Fiber Orientation and Visual Fields
Retina
The axons of the retinal ganglion cells form characteristic patterns in the nerve fiber layer. The group of fibers that course from the macular area to the optic disc is called the papillomacular bundle. The superior and inferior temporal fibers, separated at the 180th meridian by the horizontal retinal raphe, must arch superiorly and inferiorly around the macular area, forming characteristic arcuate patterns in their course to the optic disc; the temporal retinal vessels usually do not cross the horizontal raphe either. The nasal fibers can travel directly to the optic disc and are described as radiating (Figure 13-12). Nasal and temporal fibers are separated by a theoretic vertical line passing through the center of the fovea. The long nerve fibers, from the peripheral retina, are more vitread in location than are the short peripapillary fibers, with extensive intermingling in the prelaminar optic nerve.46
Optic Disc
All of the axons in the nerve fiber layer come together at the optic disc, creating a specific pattern. The nasal fibers radiate directly to the nasal side of the disc, whereas the papillomacular bundle courses directly to the temporal side of the disc.46–48 The fibers from the superior temporal retina arch around the papillomacular bundle to enter the superior pole of the disc; fibers from the inferior temporal retina curve below the papillomacular bundle to the inferior pole.48 The macular fibers take up approximately one third of the disc, although the macular area encompasses only one twentieth of the retinal area.1,8 The temporal fibers occupy approximately one third of the disc, as do the nasal fibers (Figure 13-13, A). The boundaries between each set of fibers are not always clear-cut in all parts of the pathway. The fibers from the peripheral retina are more superficial than those coming from the central retina.48
Optic Nerve
Near the lamina cribrosa, the fibers have the same orientation as they do in the disc, but within a short distance the macular fibers move to the center of the nerve.3,8 The rest of the fibers take up their logical positions: superior temporal fibers in the superior temporal optic nerve, inferior temporal fibers in the inferior temporal nerve, superior nasal fibers in the superior nasal nerve, and inferior nasal fibers in the inferior nasal optic nerve (Figure 13-13, B).
Optic Chiasm
In the optic chiasm the nasal fibers cross (decussate); the ratio of crossed to uncrossed fibers in the chiasm is approximately 53 to 47.49 The crossing pattern depends on processes that occur during embryologic development, with certain molecular guides directing the path taken by nerve fibers. The inferior nasal retinal fibers are inferior in the anterior chiasm; they cross to the other side, and many loop into the terminal part of the opposite optic nerve before turning to run back through the chiasm into the contralateral optic tract1 (Figure 13-14). These anterior loops (anterior knees of Wilbrand) bring fibers from the opposite eye into the posterior optic nerve.50 Some investigators believe that these “knees” are artifacts and suggest that the fibers shift into such a location after loss from fiber degeneration.51
The superior nasal fibers enter the superior chiasm, where they cross and then leave the chiasm in the contralateral optic tract; some of these fibers loop posteriorly into the optic tract on the same side before crossing. The fibers were historically called the posterior knees of Wilbrand1,8 (see Figure 13-14). The fibers from the temporal retina course directly back through the chiasm into the optic tract. The nasal macular fibers also cross and are spread throughout most of the chiasm.51
A small number of fibers have been identified that exit the posterior of the chiasm and enter the suprachiasmatic nucleus in the hypothalamus and have a role in synchronization of circadian rhythm.52–54
Optic Tract
As the fibers leave the chiasm in the optic tract, the crossed and uncrossed fibers intermingle. The superior fibers (the fibers from both the ipsilateral superior temporal retina and the contralateral superior nasal retina) move to the medial side of the tract. The fibers from the inferior retina (ipsilateral inferior temporal retinal fibers and contralateral inferior nasal retinal fibers) occupy the lateral area of the tract.1,3 Figure 13-15 shows the regrouping that occurs as the fibers pass through the chiasm and into the optic tract. The macular fibers, crossed and uncrossed, are located between these two groups1 (Figure 13-16).
Lateral Geniculate Nucleus
Fibers from the superior retinal quadrants terminate in the medial aspect of the LGN, whereas fibers from the inferior retinal quadrants terminate in the lateral aspect.8 A dorsal wedge, composing two thirds to three fourths of the LGN, represents the macula.3,55,56 Based on animal study mapping, each of the magnocellular and parvocellular layers receives input from just one eye: Layers 1, 4, and 6 receive fibers from the contralateral nasal retina, whereas layers 2, 3, and 5 receive ipsilateral temporal retinal fibers49 (Figure 13-17). Most of the structure, including the wedge representing the macula, contains all layers, although in the far medial and lateral aspects, some of the layers merge.6,55
The anatomic structure of the human LGN is similar to that of the monkey, so detailed maps of the monkey LGN have been applied to the human structure.57 Each layer of the LGN contains a retinotopic map or representation of the contralateral hemifield of vision. A retinotopic map is a “point-to-point localization” of the retina.14 These maps are stacked on one another, such that if a line (called a line of projection) were passed through all six layers, perpendicular to the surface, the intercepted cells all would be carrying information about the same point in the visual field. This alignment is so precise that there is a gap in each contralateral layer along the line of projection that corresponds to the location of the optic disc.15 Thus the fibers that carry information from the same site in the visual field of each eye terminate in adjacent layers of the LGN, right next to one another8 (see Figure 13-17). The fibers course through the posterior limb of the internal capsule as they leave the LGN to form the optic radiations.
Optic Radiations
The fibers leaving the lateral aspect of the LGN, representing inferior retina, follow an indirect route to the occipital lobe. They pass into the temporal lobe and loop around the tip of the temporal horn of the lateral ventricle, forming Meyer loops; these fibers form the inferior radiations8,18 (Figure 13-18). Fibers from the medial aspect of the LGN, representing superior retina, lie superiorly as they pass through the parietal lobe. The fibers from the macula are generally situated between superior and inferior fibers.
Striate Cortex
The superior radiations terminate in the area of the striate cortex above the calcarine fissure, called the cuneus gyrus; the inferior radiations terminate in the region below the calcarine fissure—the lingual gyrus. Thus the cuneus gyrus receives projections from the superior retina and the lingual gyrus from the inferior retina. Only one third of the striate cortex is on the surface of the occipital lobe; the majority is buried within the calcarine fissure, and only a small portion is on the posterolateral aspect of the occipital posterior pole.58
Retinotopic representation is present in the striate cortex. Those fibers that are adjacent to one another in the layers of the LGN project to the same area in the visual cortex (see Figure 13-17). That is, corresponding points from the two retinas (ipsilateral temporal and contralateral nasal) that represent the same target in the visual field will project to neighboring locations in the primary visual cortex. All the cells in a column correspond to a stimulus presented at the same point in the visual field, and cells in an adjacent column correspond to an adjacent point in the visual field.
Clinical Comment: Visual Field Testing
The VISUAL FIELD is tested monocularly, with the patient looking straight ahead at a fixation point and responding when a target is seen anywhere in the area surrounding that fixation point, usually described to the patient as “seen out of the corner of your eye.” The field can be divided into four quadrants by a vertical line and a horizontal line that intersect at the point of fixation. The point of fixation is seen by the fovea and is eccentric because the temporal field is slightly larger than the nasal field. Inversion and reversal of the field are caused by the optical system of the eye. The superior field is imaged on the inferior retina and the inferior field on the superior retina; the nasal field is imaged on the temporal retina and the temporal field on the nasal retina (Figure 13-19). This orientation is maintained in the cortex, where the superior field is projected onto the visual cortex inferior to the calcarine fissure, and where the inferior visual field is projected onto the cortex superior to the calcarine fissure.
The visual field seen by the right eye is nearly the same as that seen by the left eye. The nasal part of the field for one eye is the same as the temporal part of the field seen by the other eye, with the exception of the far temporal periphery, which is called the temporal crescent. The temporal crescent is imaged on the nasal retina of one eye but not on the temporal retina of the other because the depth of the orbit and the prominence of the nose blocks the periphery of the field from the temporal retina. Within each temporal field is an absolute scotoma, the physiologic blind spot, a result of the lack of photoreceptors in the optic disc (Figure 13-20).
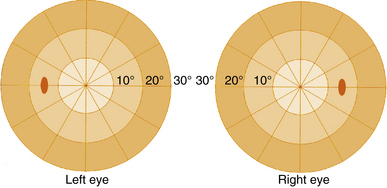
FIGURE 13-20 Central visual field plots showing scotoma of physiologic blind spot in the temporal field.
Because the fibers that emanate from nasal retina cross in the chiasm, the postchiasmal pathway carries information from the contralateral temporal field and the ipsilateral nasal field. These combined areas can be described as the contralateral hemifield (i.e., the right postchiasmal pathway carries information from the left side of the visual field for both eyes). Thus, the left side of the field is “seen” by the right striate cortex, paralleling the involvement of the right hemisphere in the motor and sensory activities of the left side of the body. Similarly, objects in the right side of the field are “seen” by the left striate cortex (see Figure 13-1).
Clinical Comment: Characteristic Visual Field Defects
Figure 13-21 depicts examples of various visual field defects.
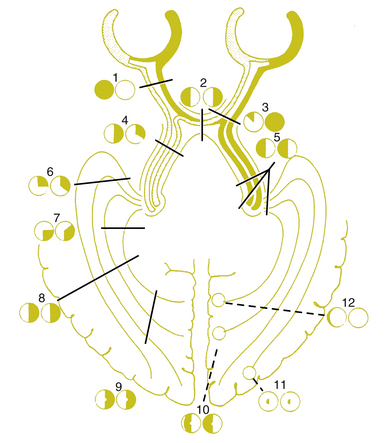
FIGURE 13-21 Visual field defects.
(From Hart WM Jr, editor: Adler’s physiology of the eye, ed 9, St Louis, 1992, Mosby.)
A lesion in the nerve fiber layer will cause a field defect corresponding to the location and configuration of the affected nerve fiber bundle. One of the disease processes that affects the nerve fiber layer is glaucoma. If temporal retinal fibers are affected, an arcuate defect can be produced that curves around the point of fixation from the blind spot to termination at the horizontal nasal meridian (Figure 13-22). This abrupt edge (at the horizontal meridian) is called a nasal step and results from the configuration of the fibers at the temporal retinal raphe. Less often, a lesion affects a nasal bundle of nerves, producing a wedge-shaped defect emanating from the physiologic blind spot into the temporal field.
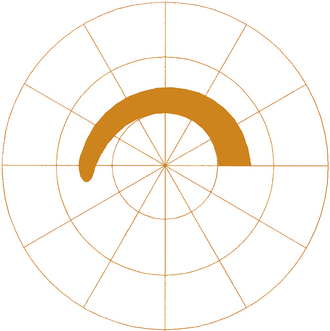
FIGURE 13-22 Automated visual field showing arcuate scotoma and nasal step in field for the left eye.
Injury to the optic nerve is accompanied by a visual field defect, a relative afferent pupillary defect, and atrophy of the affected nerve fibers, which eventually is manifested at the disc. The small-diameter, tightly packed fibers of the macula have the greatest metabolic need and often are affected first in both compressive and ischemic lesions.12
The optic chiasm brings all the visual fibers together; lesions of the chiasm usually will show bitemporal or binasal defects. The most common cause of a bitemporal field defect is a pituitary gland tumor, and a visual field defect is often the first clinical sign (Figure 13-23, A). A patient may not recognize the field loss because the nasal field of one eye overlaps the temporal field of the other eye. The crossed fibers seem to be damaged first in compressive lesions such as a tumor.14 This susceptibility to damage might be attributable to the purported weak blood supply of the median portion of the chiasm. Consequently, the crossed fibers also are more susceptible to ischemia in a vascular event.41 Involvement of both lateral sides of the chiasm, producing a binasal defect, might be caused by an aneurysm of the internal carotid artery that impinges on the chiasm and displaces it against the other internal carotid artery (Figure 13-23, B).
A homonymous field defect will be produced by a single lesion in the postchiasmal pathway, as the nasal fibers of the contralateral eye join the temporal fibers of the ipsilateral eye; visual acuity usually is not affected because one half the fovea is sufficient for 20/20 Snellen acuity.14 In this lesion the field loss is present on the side of the field contralateral to the lesion. Other signs or symptoms accompanying a homonymous defect can help the diagnostician determine more exactly the site of the lesion.
A lesion involving the optic tract eventually will produce optic nerve atrophy, which usually becomes evident as optic disc pallor. Because the optic tract is relatively small in cross section, a lesion often damages all of the fibers, causing a homonymous field defect that affects the entire half of the field; if a partial hemianopia results, the defects will be incongruent.59 The defects in a homonymous field are congruent if the two defects are similarly shaped and are incongruent if the defect shapes are dissimilar. Because crossed fibers outnumber uncrossed fibers, a lesion of the optic tract may be accompanied by a relative afferent pupillary defect of the contralateral eye.14
A lesion in the LGN would affect the contralateral field and eventually also cause optic atrophy; however, there would be no associated pupillary defect. Because of the point-to-point localization in the LGN, lesions here produce moderately to completely congruent field defects.42
Damage to the optic radiations or cortex does not normally cause atrophy of the optic nerve because it does not involve the fibers of the retinal ganglion cells. A lesion of the optic radiations causes a contralateral homonymous field defect and, because the fibers are so spread out, the defect often affects only one quadrant. If a lesion of the temporal lobe involves the Meyer loop, a superior quadrant field defect will result; parietal lobe lesions more commonly cause inferior field defects (Figure 13-23, C).14
The characteristic feature of a defect in the occipital lobe is congruency. Congruency depends on how closely fibers from corresponding points of each eye (carrying the same visual field information) are positioned to one another at the site of the lesion. As the fibers reach the occipital lobe and finally the striate cortex, the fibers emanating from corresponding points in the field come together to form a point-to-point representation of the field. Therefore, a lesion here will cause a congruent defect (Figure 13-24).
When visual association areas within the occipital, temporal, and parietal lobes are involved, higher cortical visual processes may be affected. Lesions of the parietal lobe can cause abnormal optokinetic nystagmus and affect visual attention; temporal lobe lesions can cause olfactory hallucinations, formed visual hallucinations, or déjà vu phenomenon; injury involving the occipitotemporal cortex can affect object and facial recognition.60 Blind sight occurs when there seems to be some sight in a hemifield but there is no conscious awareness of the sight. That is, a motor reflex response can be elicited with presentation of an unexpected stimulus in the affected field, but the patient has no awareness of the vision. It is likely that subcortical visual responses are mediated at the level of the superior colliculus and that the reflex does not initiate from the visual cortex.
Striate Cortex Maps
Early study correlating the visual field to striate cortex was done by Holmes and Lister,61 who studied injured soldiers from World War I and attempted to match visual field defects with injuries from shrapnel to the occipital lobe. The Holmes map was the most detailed source showing the representation of the visual field in human striate cortex. The macular portion extends from the posterior pole forward, with the periphery of the field represented in the anterior occipital lobe and the uniocular temporal crescent in the most anterior aspect of the striate cortex adjacent to the parietooccipital sulcus. However, detailed mapping of monkey striate cortex using electrophysiologic methods revealed discrepancies between monkey and human data. These findings suggested that either monkey cortex and human cortex were not as alike as believed or the Holmes map required some modification.
Technologies such as MRI have been used to study the human cortex, allowing more direct correlation of a lesion with a field defect. Some investigators suggest revision of the Holmes map58 similar to Figure 13-25. The primary change concerns the extent of the area depicting macular representation. A much greater area of the visual cortex is thought to be taken up by macular projection,58 with the central 30 degrees of the visual field represented in approximately 83% of the striate cortex58 (Figure 13-26). Other imaging studies more closely agree with the Holmes map and show that the central 15 degrees of vision occupies 37% of the surface area of the striate cortex.62 Some discrepancies may result from the nature of the lesion because an MRI may overestimate the actual area involved when edema is present.62
Macular Sparing
Macular sparing occurs when an area of central vision remains within a homonymous field defect. Because fixational eye movements of 1 to 2 degrees do occur during the visual field examination, the area spared within the defect should involve at least 3 degrees in order for macular sparing to be confirmed clinically.45 Because the macular area often was spared in homonymous defects caused by occipital lobe lesions, it once was supposed that the entire macula was represented in both sides of the striate cortex. We now know that this is not the case. However, even in the presence of an extensive lesion, some of the macular projection area might remain unaffected, either because the posterior pole of the occipital lobe has such an extensive blood supply or because the macular projection covers a very large area.14 Macular sparing can also be explained by the size and overlap of the receptive field of the retinal ganglion cells subserving the vertical meridian.63
Aging within the Visual Pathway
Neural cell death occurs throughout all structures of the visual pathway, although the extent varies significantly within the population.64 Age is accompanied by a decrease in the extent of the visual field, caused both by loss of cells and by a decrease in the transparency of the ocular media.12,65 The ability to perceive accurately the speed of moving objects declines with age, and animal studies have identified an age-related difference in temporal processing speed at the level of the visual cortex.66 This decline in accurately perceiving the speed of moving objects may contribute to the higher incidence of automobile accidents among the elderly population.
1. Hoyt W.F., Osman L. Visual fiber anatomy in the infrageniculate pathway of the primate. Arch Ophthalmol. 1962;68:124.
2. Polyak S. The vertebrate visual system. Chicago: University of Chicago Press; 1957.
3. Brouwer B., Zeeman W.P. The projection of the retina in the primary optic neuron in monkeys. Brain. 49, 1926.
4. Boyd J.D., Gu Q., Matsubara J.A. Overview of the central visual pathways. In: Kaufman P.L., Alm A., editors. Adler’s physiology of the eye. ed 10. St Louis: Elsevier; 2003:641.
5. Jonas J.B., Schmidt A.M., Muller-Bergh J.A., et al. Human optic nerve fiber count and optic disc size. Invest Ophthalmol Vis Sci. 2012;33(6):1992.
6. Sadun A.A., Glaser J.S. Anatomy of the visual sensory system. Tasman W., Jaeger E.A., editors. Duane’s foundations of clinical ophthalmology, vol 1. Philadelphia: Lippincott, 1994.
7. Mikelberg F.S., Drance S.M., Schulzer M., et al. The normal human optic nerve. Axon count and axon diameter distribution. Ophthalmology. 1989;96:1325.
8. Warwick R. Eugene Wolff’s anatomy of the eye and orbit, ed 7. Philadelphia: Saunders; 1976. p 325
9. Parravano J.G., Toledo A., Kucharczyk W. Dimensions of the optic nerves, chiasm, and tracts: MR quantitative comparison between patients with optic atrophy and normals. J Comput Assist Tomogr. 1993;17(5):688.
10. Levin L.A. Optic nerve. In: Kaufman P.L., Alm A., editors. Adler’s physiology of the eye. ed 10. St Louis: Elsevier; 2003:603.
11. Unsold R., Hoyt W.F. Band atrophy of the optic nerve. The histology of temporal hemianopsia. Arch Ophthalmol. 1980;98:1637.
12. Harrington D.O. The visual fields, ed 5. St Louis: Mosby; 1981.
13. Borchert M.S. Vascular anatomy of the visual system. Ophthalmol Clin North Am. 1996;9(3):327.
14. Horton J.C. The central visual pathways. In: Hart W.M.Jr., editor. Adler’s physiology of the eye. ed 9. St Louis: Mosby; 1992:728.
15. Casagrande V.A., Ichida J.M. The lateral geniculate nucleus. In: Kaufman P.L., Alm A., editors. Adler’s physiology of the eye. ed 10. St Louis: Elsevier; 2003:655.
16. Lachica E.A., Casagrande V.A. The morphology of collicular and retinal axons ending on small relay (W-like) cells of the primate lateral geniculate nucleus. Vis Neurosci. 1993;10(3):403.
17. Casagrande V.A., Ichida J.M. The primary visual cortex. In: Kaufman P.L., Alm A., editors. Adler’s physiology of the eye. ed 10. St Louis: Elsevier; 2003:669.
18. Krolak-Salmon P., Guenot M., Tiliket C., et al. Anatomy of optic nerve radiations as assessed by static perimetry and MRI after tailored temporal lobectomy. Br J Ophthalmol. 2000;84(8):884.
19. Gilbert C.D., Kelly J.P. The projections of cells in different layers of the cat’s visual cortex. J Comp Neurol. 1975;163:81.
20. Hubel D.H., Wiesel T.N. Laminar and columnar distribution of geniculocortical fibers in the macaque monkey. J Comp Neurol. 1972;146:421.
21. Horton J.C., Dagi L.R., McCrane E.P., et al. Arrangement of ocular dominance columns in human visual cortex. Arch Ophthalmol. 1990;108(7):1025.
22. Zeki S., Watson J.D., Lueck C.J., et al. A direct demonstration of functional specialization in human visual cortex. J Neurosci. 1991;11(3):641.
23. Livingstone M.S., Hubel D.H. Segregation of form, color, movement, and depth: anatomy, physiology, and perception. Science. 1988;240:740.
24. Hockfield S., Tootell R.B., Zaremba S. Molecular differences among neurons reveal an organization of human visual cortex. Proc Natl Acad Sci U S A. 1990;87(8):3027.
25. Hubel D.H., Livingstone M.S. Color and contrast sensitivity in the lateral geniculate body and primary visual cortex in the macaque monkey. J Neurosci. 1990;10(7):2223.
26. Silverman S.E., Trick G.L., Hart W.M.Jr. Motion perception is abnormal in primary open-angle glaucoma and ocular hypertension. Invest Ophthalmol Vis Sci. 1990;31(4):722.
27. Hubel D.H., Wiesel T.N. Receptive fields and functional architecture of monkey striate cortex. J Physiol. 1968;195:215.
28. Hubel D.H., Wiesel T.N., Stryker M.P. Anatomical demonstration of orientation columns in macaque monkey. J Comp Neurol. 1978;177:361.
29. Boyd J.D., Matsubara J.A. Extrastriate cortex. In: Kaufman P.L., Alm A., editors. Adler’s physiology of the eye. ed 10. St Louis: Elsevier; 2003:686.
30. Catani M., Jones D.K., Donato R., et al. Occipito-temporal connections in the human brain. Brain. 2003;126(pt 9):2093.
31. Wurtz R.H. Vision for the control of movement: the Friedenwald Lecture. Invest Ophthalmol Vis Sci. 1996;37:2310.
32. Roland E., Gulyás B., Seitz R.J., et al. Functional anatomy of storage, recall, and recognition of a visual pattern in man. Neuroreport. 1990;1:53.
33. Belliveau J.W., Kennedy D.N.Jr., McKinstry R.C., et al. Functional mapping of the human visual cortex by magnetic resonance imaging. Science. 1991;254(5032):716.
34. Belliveau J.W., Kwong K.K., Kennedy D.N., et al. Magnetic resonance imaging mapping of brain function: human visual cortex. Invest Radiol. 1992;27(Suppl 2):59.
35. Kwong K.K., Belliveau J.W., Chesler D.A., et al. Dynamic magnetic resonance imaging of human brain activity during primary sensory stimulation. Proc Natl Acad Sci U S A. 1992;89(12):5675.
36. Menon R.S., Ogawa S., Kim S.G., et al. Functional brain mapping using magnetic resonance imaging: signal changes accompanying visual stimulation. Invest Radiol. 1992;27(Suppl 2):47.
37. Le Bihan D., Turner R., Zeffiro T.A., et al. Activation of human primary visual cortex during visual recall: a magnetic resonance imaging study. Proc Natl Acad Sci U S A. 1993;90(24):11802.
38. Ogawa S., Menon R.S., Tank D.W., et al. Functional brain mapping by blood oxygenation level-dependent contrast magnetic resonance imaging: a comparison of signal characteristics with a biophysical model. Biophys J. 1993;64(3):803.
39. MacKenzie P.J., Cioffi G. Vascular anatomy of the optic nerve head. Can J Ophthalmol. 2008;43:308-312.
40. Francoisa J., Neetens A., Collette J.M. Vascularization of the optic pathway. Br J Ophthalmol. 1958;42:80.
41. Lao Y., Gao H., Zhong Y. Vascular architecture of the human optic chiasma and bitemporal hemianopia. Chin Med Sci J. 1994;9(1):38.
42. Ferreira A., Braga F.M. Microsurgical anatomy of the anterior choroidal artery. Arq Neuropsiquiatr. 1990;48(4):448. (abstract)
43. Margo C.E., Hamed K.M., McCarty J. Congenital optic tract syndrome. Arch Ophthalmol. 1991;109(8):1120.
44. Luco C., Hoppe A., Schweitzer M., et al. Visual field defects in vascular lesions of the lateral geniculate body. J Neurol Neurosurg Psychiatry. 1992;55(1):12.
45. McFadzean R., Brosnahan D., Hadley D., et al. Representation of the visual field in the occipital striate cortex. Br J Ophthalmol. 1994;78(3):185.
46. Ogden T.E. Nerve fiber layer of the macaque retina: retinotopic organization. Invest Ophthalmol Vis Sci. 1983;24:85.
47. Hoyt W.F., Tudor R.C. The course of parapapillary temporal retinal axons through the anterior optic nerve. A NAUTA degeneration study in the primate. Arch Ophthalmol. 1963;69:503.
48. Ballantyne A.J. The nerve fiber pattern of the human retina. Trans Ophthalmol Soc UK. 1946;66:179.
49. Kupfer C., Chumbley L., Downer J., et al. Quantitative histology of optic nerve, optic tract, and lateral geniculate nucleus of man. J Anat. 1967;101:393.
50. Wilbrand H.L. Schema des verlaufs der sehnervenfasern durch das chiasma. Z Augenheilk 59. 135, 1927.
51. Jeffery G. Architecture of the optic chiasm and the mechanisms that sculpt its development. Physiol Rev. 2001;81(4):1393.
52. Moore R.Y. Retinohypothalmic projection in mammals: a comparative study. Brain Res. 1973;49:403.
53. Berson M. Phototransduction in ganglion-cell photoreceptors. Eur J Physiol. 2007;454:849-855.
54. La Cour M. Ehinger B: The retina. In: In:Fischbarg J, editor. The biology of the eye. Elsevier: Amsterdam; 2006. p195-252
55. Kupfer C. The projection of the macula in the lateral geniculate nucleus of man. Am J Ophthalmol. 1962;54:597.
56. Hickey T.L., Guillery R.W. Variability of laminar patterns in the human lateral geniculate body. J Comp Neurol. 1979;183:221.
57. Malpeli J.G., Baker F.H. The representation of the visual fields in the lateral geniculate body of Macaca mulatta. J Comp Neurol. 1975;161:569.
58. Horton J.C., Hoyt W.F. The representation of the visual field in human striate cortex. Arch Ophthalmol. 1991;109:816.
59. Reese B.E., Cowey A. Fibre organization of the monkey’s optic tract. A revision of the classic Holmes map, II. Noncongruent representation of the two half-retinae. J Comp Neurol. 1990;295(3):401.
60. Balcer L.J. Anatomic review and topographic diagnosis. Ophthalmol Clin North Am. 2001;14:1.
61. Holmes G., Lister W.T. Disturbances of vision from cerebral lesions with special reference to the cortical representation of the macula. Brain. 1916;39:34.
62. Wong A.M., Sharpe J.A. Representation of the visual field in the human occipital cortex: a magnetic resonance imaging and perimetric correlation. Arch Ophthalmol. 1999;117(2):208.
63. Reinhard J., Trauzettel-Klonsinski S. Nasotemporal overlap of retinal ganglion cells in humans: a functional study. Invest Ophthalmol Vis Sci. 2003;44(4):1568.
64. Hirose T., Katsumi O. Functional changes: psychophysical and electrophysiologic measurements. In: Albert D.M., Jakobiec F.A., editors. Principles and practice of ophthalmology. Philadelphia: Saunders; 1994:728.
65. Trobe J.D., Glasser J.S. The visual field manual: a practical guide to testing and interpretation. Gainesville, Fla: Triad; 1983.
66. Mendelson J.R., Wells E.F. Age-related changes in the visual cortex. Vis Res. 2002;42:695.