Chapter 145 Subsidence and Dynamic Cervical Spine Stabilization
Fundamental Concepts
The loss of vertical height with aging has been observed for centuries. This process, resulting in humans losing height after the achievement of adult status, is multifactorial. It essentially involves the loss of disc height and vertebral body collapse.1 Both of these processes may involve deformation along the neutral axis (axial deformation), but often deformation around an axis of rotation (angular deformation) is also involved. Both angular and axial deformities result in loss of vertical height. Gravity and repetitive axial loading contribute to the deformation. Angular deformation most often occurs in the sagittal plane, resulting in kyphosis. This kyphosis results in an applied moment arm. As kyphosis progresses, the moment arm is lengthened, and deformity progresses further.1 This situation is portrayed by the phrase, “kyphosis begets kyphosis.” Kyphosis is usually undesirable. In the cervical region, it places the cervical musculature at a biomechanical disadvantage and may lead to mechanical neck pain. It may also accelerate adjacent segment degenerative changes.2,3 If kyphosis becomes severe, forward gaze and respiration may be adversely affected. Although subsidence occurs in all regions of the spine, subsidence after surgery is most evident in the cervical spine.
Graft Resorption and Remodeling
As a result of all of the aforementioned processes, bone grafts first partially resorb before being replaced by new living bone. The process results in subsidence of the bone graft after surgery. This subsidence is part of the normal biology of bone healing and not a pathologic process. It often is not appreciated in noninstrumented constructs. However, in studies that specifically measure construct height, subsidence is routinely observed.4–6 The amount of subsidence varies with the type of graft used and number of levels fused. Bishop et al.4 showed that in uninstrumented ventral cervical discectomies that used iliac crest autograft, the average settling was 1.4 mm for a single-level construct and 1.8 mm for two-level procedures. The amount of settling increased to 2.4 mm for single levels and 3.0 mm for two levels when iliac crest allograft was used.
Graft Collapse
A cervical interbody graft can collapse before its incorporation, also resulting in subsidence. Graft collapse occurs for numerous reasons. If the graft is inadequately sized to handle the loads placed on it, it may collapse. Collapse can occur if the graft is too narrow in width or depth with respect to the adjacent vertebrae. This size mismatch increases the load placed on a graft. A larger graft spreads the load over a larger area, reducing the load per unit of surface area, and the graft may be able to withstand axial loads more effectively. Finally, if there is a good match between the contours of the surface of the vertebrae and the graft (so-called gapless fusion), the weight-bearing forces are evenly distributed over the region of contact, diminishing the chance of graft collapse.
Pistoning (Subsidence)
Some surgeons have attempted to devise methods of interlocking the grafts and vertebrae when fixation is not used. Other surgeons advocate mortising the graft into the vertebrae, that is, countersinking the graft into the cancellous region of the vertebrae beyond the end plates. This approach minimizes the chance of graft migration or expulsion but at the expense of increasing the chance of subsidence. Other surgeons perforate the end plate in various patterns to encourage vascular ingrowth. However, extensive violation of the end plate, especially when used with dense cortical bone, is likely to increase pistoning. Lim et al.7 showed that it is important to preserve as much end plate as possible to prevent graft subsidence into the vertebral body. They found that making one central hole to increase vascular access to the graft rather than multiple smaller holes reduced stresses on the end plate.
Cervical Plating and Evolution of Dynamic Fixation
First-Generation Systems
The use of such plates helps preserve or restore lordosis, reduces graft extrusion, and improves graft union rates. This use also facilitates the performance of more extensive procedures when indicated by the disease process.8–13 Ventral cervical plating allows the surgeon to use allograft more liberally instead of autograft. Allograft success rates have been shown to equal success rates of autograft when ventral plating was used.14 Use of allograft helped avoid donor site complications, which can be a significant source of patient morbidity (i.e., pain at the graft harvest site).
The initial ventral cervical implants were not perfect, and implant-related complications were observed. The initial Caspar plating system used parallel slots for the screws, which were not locked or constrained to the plate. This was a truly axially dynamic implant. The implant required bicortical screw placement for optimal stability. Despite bicortical placement, screw backout was observed sometimes. The dynamic nature of the implant permitted axial subsidence. However, this subsidence was not recognized at the time as part of the normal biology of bone healing (as already discussed) and was thought to be undesirable. The plate was modified so that holes replaced one half of the slots (Fig. 145-1). Rather than preventing subsidence, this modification led to screw breakage. The screw breakage virtually always involved the screws placed in the holes, which were subjected to excessive bending moments (Fig. 145-2). Fixed in the cortex dorsally and the hole in the plate ventrally, the screws typically fractured in the middle at the point of both the maximum bending moment application and the maximum stress application (stress = bending moment/strength). The screws placed into the slots were able to toggle or slide along the slots and did not fracture.
Second-Generation Systems
In an attempt to prevent the aforementioned complications (presumed pathologic settling, screw backout, and screw fracture) and to avoid the need for bicortical screw purchase, a second generation of cervical fixation systems emerged. These devices featured screws fixed to the implant and permitted screw convergence on placement. The cervical spine locking plate (CSLP) from Synthes was the first such device. The ability to “toe-in” the screws secured the plate in part by triangulation and acted to reduce the chance of screw pull-out (Fig. 145-3). These devices were initially successful, but failures caused by plate or screw fracture, construct pull-out, and delayed union or nonunion were increasingly recognized. Attempts were made to remedy this situation by increasing the strength of the plate; examples are the Orion plate from Sofamor-Danek and the Codman plate (Fig. 145-4). These more rigid implants reduced hardware fracturing but may have increased the incidence of delayed union and pseudarthrosis caused by stress shielding (see later discussion).
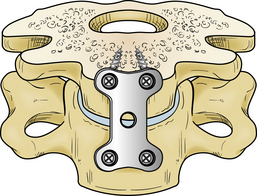
FIGURE 145-3 CSLP (Synthes). Triangulation of the locked unicortical screws was used to increase pull-out strength.
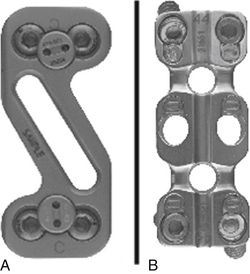
FIGURE 145-4 Orion plate (Sofamor-Danek) (A) and Codman plate (B) were made stronger to try to reduce hardware failures.
Despite their shortcomings, second-generation plating systems have achieved better results than noninstrumented fusions, and they have become quite popular. Multiple variations on this basic theme have been developed as other manufacturers entered the market. Despite the popularity and ease of use of these systems, failures continued to occur. At the time (early 1990s), the spine community did not fully appreciate the reason for these nonunions and failures. Because these were often late sequelae, many were not likely recognized or at least were underreported. Figure 145-5 illustrates such a case. This patient developed neck pain 3 years after an apparently successful fusion (see Fig. 145-5A). The growth of new osteophytes (shown by arrows on Fig. 145-5B) was the major clue that a nonunion was present. This nonunion was confirmed with flexion-extension films (see Figs. 145-5C and D), which showed 8 mm of motion at the tips of the spinous processes. No motion should have been present if a solid fusion was present.
Third-Generation Systems
History and Rationale
Benzel et al.15 focused attention on the problem of stress shielding. They used this knowledge to develop the first axially dynamic cervical implant. Although relatively new to the cervical spine (remember the slotted Caspar plates), the concept of dynamism has been used in other applications, in particular, orthopaedic surgery procedures involving the hip and long bones. Dynamic hip arthroplasties have been used for femoral neck fractures with significant success. This construct permits the femoral neck to deform along its axis so that the opposing fracture margins are exposed to optimal bone healing–enhancing forces. A meta-analysis of 2855 patients showed reductions in cutout (4% vs. 13%), nonunion (0.5% vs. 2%), breakage (0.7% vs. 14%), and reoperation (4% vs. 10%) when dynamic hip pinning was used.16 The Weiss-spring, as modified by Larson, is also a dynamic implant with a spine application. When this implant is combined with interbody fusion in the thoracic or lumbar spine, subsidence is encouraged, and bone healing–enhancing forces are increased; this increases fusion success.17
Understanding the implications of Wolff’s law, as applied to bone healing and specifically ventral cervical bone grafting, is crucial to understanding the rationale for and value of dynamic cervical spine stabilization. Wolff’s law essentially states that every change in the function of a bone is followed by definite changes in its internal architecture and secondary alterations in its external configuration. The implication is that bone is formed where stresses require its presence and is resorbed where stresses do not require it. The load “seen” by a bone to some extent determines its shape and strength. In long bone fracture fixation with rigid plates, if the plate is not removed after initial healing, late fracture or pseudarthrosis may occur. If the plate is removed after the initial healing process has occurred, the bone is able to “see” normal weight-bearing forces with resultant maximal healing occurring. Cases of successful fusion after rigid cervical implant fracture provide a further illustration. Fracture of the plate permitted the bone grafts to “see” bone healing forces and encouraged fusion. If the plate had not fractured, a pseudarthrosis may have developed. This phenomenon has been referred to as secondary dynamism by plate fracture (Fig. 145-6).
During the evolution of cervical fixation systems, it was recognized that a single fixed screw plate angle was not optimal for all patients. Variable screw angle designs were introduced. Some second-generation systems had the screw locked to the plate to prevent backout but did not lock the screw angulation when implanted, and this angle could change (i.e., toggle). Such designs theoretically could allow some settling as the graft absorbs or subsides. For this settling to occur, however, the screw must migrate or cut through the ventral cortex or toggle within the vertebrae (Fig. 145-7). That this activity can occur is clear; however, it is a slow process. This situation is in contrast to the early subsidence seen with true axially dynamic implants (see later disucssion). In some cases, the density of the vertebrae may prevent such screw migration, and screw fracture can occur (Fig. 145-8). In addition, such screw migration has the potential of weakening the screw-bone interface and may lead to implant failure by pull-out. In the series by Epstein,18 failure occurred with a variable angled screw plate in 16% of cases.
System
The third-generation ventral cervical fixation systems, which provided true axial dynamic stabilization, were pioneered by Benzel and Yuan with the introduction of the DOC VCSS system from DePuy AcroMed (Raynham, MA). This plating system was developed after it was perceived that there was a need for a system that would enhance fusion rates, decrease deformity progression, improve deformity correction, and decrease the chance of construct failure. The observation that rigid plates could fracture in patients who were subsequently observed to have a solid arthrodesis provided some additional impetus for the development of an axially dynamic cervical implant. If the plate had not fractured, a pseudarthrosis may have resulted. These observations led researchers such as Benzel and Yuan to believe that implants that permitted subsidence may be superior for the attainment of cervical fusion.
The DOC VCSS system consists of platforms that are rigidly affixed to the vertebral bodies via screws. Toggling the screws in the vertebral body is not permitted. The platforms slide along two rods, whereas a cross-fixator rigidly affixed to the rods limits the amount of sliding and the amount of subsidence permitted (Fig. 145-9). In this manner, axial deformation (subsidence) is controlled. The manufacturer sets this cross-fixator at 3 mm, but the surgeon may adjust it if deemed necessary. This system is not applicable to single-level constructs.
The ABC system (Aesculap Instrument Corp., Center Valley, PA) permits load sharing by allowing unrestricted subsidence. The plate has slots bilaterally at each level (Fig. 145-10). The screws are locked to the plate by a unique internal locking mechanism, which prevents backout but does not restrict either settling or screw rotation. This is the first implant with this unique design. It is available for single-level and multiple-level constructs. No attempt is made to limit the amount of subsidence, which occurs initially by the screws sliding in their respective slots. If more dynamism is required, screw angulation can occur. This situation is rarely seen. With true load sharing, early and more substantial graft incorporation and maturation is observed so that despite the absence of settling restriction, the extent of settling is not excessive, and often the full amount allowed by the slot does not occur. This situation has also been observed with the DOC VCSS system. Even with multiple-level discectomies, less than 3 mm of subsidence is the norm.
The Premier and C-Tek plates have similar slotted designs to allow settling, with a retaining band over the screw heads to prevent backout (Fig. 145-11). They differ functionally from the ABC plate in that they do not have a slot in the inferiormost (caudal) position but instead have a hole. It is unclear whether this restriction of caudal dynamism has a significant effect on outcomes, and no clinical series are yet available to answer such questions.
Clinical Experience
Clinical series reported with these dynamic systems have shown that they do achieve their goals and offer advantages to spine surgeons and their patients. With the ABC system, early and more substantial graft incorporation has resulted in a very low nonunion rate. A review of nearly 500 patients involving more than 800 motion segments showed that the ABC system effectively stabilizes the spine in various conditions, including surgery for degenerative disease, herniated discs, and trauma.19 This stabilization is achieved with both corpectomy and interbody approaches, with allograft and autograft iliac crest bone. Fusion was rigorously defined as absence of motion at the tips of the spinous processes between flexion and extension, in addition to the presence of bridging trabecular bone. When using this stringent definition, 67% of the levels (341 of 508) were fused at 3 months, 81% were fused at 6 months (310 of 384 levels), 93% were fused at 12 months (238 of 255 levels), and 100% (69 of 69 levels) were fused at 24 months. Essentially, early trabecular bridging and progressive graft maturation were observed. Measuring the deflection at the tips of the spinous processes magnifies the initial flexibility typically allowed by the weaker new bone. The amount of such movement progressively decreases as the bone graft matures. Most importantly, no regression with increasing motion is observed if the fusion is progressing.
Cobb angle measurements showed preservation of lordosis with minimal losses of 2 to 8 degrees in only 3% to 4% of patients. Kyphotic angulation corrections were maintained within 5 degrees. Dynamic fixation did not result in an increased angular deformation reported with uninstrumented fusion.4,5 The amount of settling observed is consistent with uninstrumented fusions, averaging 1.5 to 2.0 mm per level, with slightly more settling observed with allograft. Most of the settling occurred within the first month of surgery, and virtually all occurred within 3 months. The unrestricted settling allowed by dynamic plating did not cause excessive settling but instead facilitated earlier and more substantial fusion.
Epstein20 reported her experience with the ABC plate after single-level cervical corpectomy and fusion. Serial dynamic radiographs and two-dimensional CT scanning were used to analyze construct failure or nonunion, or both, up to 12 months after the procedure. Four patients developed postoperative plate or graft complications during the average of 34 months. One patient, with a plate and graft extrusion, required a second two-level corpectomy with posterior wiring and fusion. Two patients with nonunion and one with a delayed iliac crest strut fracture required secondary dorsal fusion. Epstein20 concluded “. . . effective arthrodesis and a low incidence of complications following one level ACF performed utilizing dynamic ABC plates were attributed to reduced stress shielding and greater graft compression afforded by the unique plate design. Applying dynamic ABC plates for one level ACF was biomechanically advantageous with low morbidity.”
Pitzen et al.21 reported their experience treating cervical spondylosis using autograft and either a rigid or axially dynamic (ABC) plate. Patients were followed for 2 years. Four patients in the rigid plate group had implant complications, whereas no complications were seen in the ABC group. Mean segmental mobility before discharge for the ABC group was 1.7 mm, 1.4 mm after 3 months, 0.8 mm after 6 months, and 0.4 mm after 2 years. For the rigid plate group, these values were 1.0 mm, 1.8 mm, 1.6 mm, and 0.5 mm. The difference at 6 months between both groups was significant. The loss of segmental lordosis for the ABC group with respect to intraoperative radiograph was 1.3 degrees at discharge and 4.3 degrees after 2 years. For the rigid plate group, these values were 0.9 degrees and 0.7 degrees. The difference at 2 years was significant. No difference was seen in clinical outcomes at 2 years (visual analogue scale, Oswestry Disability Index). The authors concluded that an axially dynamic implant should be the implant of choice because of the significantly lower incidence of construct complications with equivalent outcomes.
Steinmetz et al.22 reviewed their preliminary clinical experience with the DOC VCSS plate in the treatment of multiple-level cervical spondylosis. There were 34 patients with symptomatic multiple-level cervical spondylosis who underwent decompression and fusion with the DOC VCSS system. The operations included two-level, three-level, and four-level anterior cervical discectomy and fusions and one-level, two-level, and three-level corpectomies. Fusions included allograft (76%) and autograft (24%). Minimal follow-up was 6 months, with an average of 13 months. The DOC plate did permit subsidence, with an average of 1.7 mm of subsidence at 13 months after surgery. Most of this subsidence occurred within 3 months postoperatively, with 61% of patients showing 2 or more mm of subsidence in this early time period.
Lordosis was maintained after surgery in most patients, with an average of 14 degrees of lordosis observed in the entire patient population. This lordotic configuration was well maintained after surgery. An average change (postoperative compared with latest clinical follow-up) of 0.4 degrees of lordosis was shown at the latest clinical follow-up (13 months after surgery). These results show that the DOC plate permitted axial subsidence yet prevented angular deformation (kyphosis).
Bose23 reported experience using the DOC ventral cervical implant in the treatment of cervical spondylosis. Cervical discectomy or corpectomy, or both, were performed in 37 patients. At a mean follow-up time of 1.3 years, postoperative neck or arm pain was resolved in 52% of the patients, restriction on function was mild or absent in 88%, and fusion was successful in 80% of patients and 88% of the treated levels. There was one implant-related complication, one significant dysphagia complication, and a 10.8% donor graft site complication rate. Overall, the DOC implant resulted in excellent outcome, maintenance of sagittal angle, and controlled subsidence.
Saphier et al.24 studied the clinical and radiographic results of the Premier plate or a rigid plate used during a one-level or two-level anterior cervical discectomy and fusion with allograft. Follow-up ranged from 12 to 35 months. The authors showed superior clinical results in the load-sharing Premier group along with a lower symptomatic pseudarthrosis rate. Vertical translation was found to be greater with the use of the Premier plate.
Laboratory studies have shown that dynamic systems actually are more stable than their more rigid counterparts. In an instrumented laboratory model, Brodke et al.25 showed that DOC and ABC systems do load share with a simulated subsidence of 10% of the graft height in a corpectomy model. Compared with the CSLP and Atlantis systems, they provide equal initial stability in flexion and extension and lateral bending. Of great significance was the fact that they maintained a much higher level of stability after simulated subsidence. The CSLP and Atlantis systems lost 80% to 90% of their ability to resist flexion and extension, whereas ABC and DOC maintained most of their initial stiffness. Although perhaps counterintuitive, this result occurred because the construct was loaded axially, simulating the weight of the head and pull of the neck muscles.
With the dynamic systems, the graft-vertebra interface through load sharing participates in construct stabilization by remaining under axial load and imparting stability to the construct. With the more rigid systems, axial loading does not load the graft because of stress shielding. The axial forces are cantilevered through the screws to the plate and dorsal aspect of the spine (Fig. 145-12). The bone grafts are shielded from axial loads and are unable to participate in stabilizing the construct. Plate flexion or a degradation of the screw-bone interface can result. These data are supported by the laboratory report of DiAngelo et al.,2 in which they observed a significant buffering of the loading and unloading of interbody grafts during flexion and extension when axially dynamic fixators were used.
More recent clinical data also support these data. Epstein18 reviewed her series of multiple-level corpectomies performed for severe degenerative disease or ossification of the posterior longitudinal ligament with significant myelopathy. She used a ventral fibular strut graft and cervical plates augmented with dorsal wiring and grafting. In 56 consecutive cases, Epstein had a 10% ventral construct failure with Orion rigid plates, a 16% construct failure with Atlantis variable-angle plates, and no failures with the 18 ABC dynamic plates. Although not a pure ventral plating series, Epstein’s series18 shows that the dynamic systems are not weaker in these multiple-level constructs but rather appear superior.
Epstein26 reported on her experience using the ABC plate for the stabilization of multiple-level anterior cervical corpectomies. No construct failures occurred in these two-level to four-level corpectomies with the inclusion of halo immobilization and posterior wiring. It seems that the gradual and controlled subsidence is beneficial in promoting stability and fusion in these complex cases.
Khoo et al.27 reported a series of 61 patients with cervical trauma operated on at two large municipal trauma centers over a 5-year period. Four ventral cervical plates were used—CSLP, Atlantis, Codman, and ABC—representing a rigid plate, two variable-angle plates (one of which allowed more screw rotation [the Codman plate]), and a fully dynamic plate (the ABC plate) in conjunction with various constructs including interbody approaches in some and, more frequently, corpectomies. In some cases, supplemental dorsal fixation was also used. The follow-up was for a minimum of 12 months. All images were scanned and analyzed by a computerized program, ensuring consistent operator-independent measurements. In these grossly unstable spinal injuries, the ABC system performed substantially better than the rigid and semiconstrained variable-angle screw systems with less settling, better preservation of lordosis, and no construct failures. Screw pull-out was observed in 13% of CSLP plates, 20% of Atlantis plates, 31% of Codman plates, and 0% of ABC plates. The construct failed in 13% of CSLP cases and 7% of Atlantis cases. The graft dislodged in 17% of CSLP cases, 7% of Atlantis cases, and 15% of Codman cases, but no such failures occurred with the ABC cases. The loss of graft height and lordosis was also measured. At 18 months after surgery, the cases in which the Codman plate was used averaged 37% loss of graft height compared with 32% with CSLP plates, 19% with Atlantis plates, and 10% with ABC plates. At 12 months, 7.31 degrees of lordosis was lost with the Codman system, 6.84 with CSLP, 4.3 with Atlantis, and 3.2 with ABC.
Bishop R.C., Moore K.A., Weidner A., et al. Anterior cervical interbody fusion using autogeneic and allogeneic bone graft substrate: a prospective comparative analysis. J Neurosurg. 1996;85:206-210.
Brodke D.S., Gollogly S., Alexander Mohr R., et al. Dynamic cervical plates: biomechanical evaluation of load sharing and stiffness. Spine (Phila Pa 1976). 2001;26:1324-1329.
DiAngelo D.F., Foley K.T., Vossel K.A., et al. Anterior cervical plating reverses load transfer through multilevel strut grafts. Spine (Phila Pa 1976). 2000;25:783-795.
Pitzen T.R., Chrobok J., Stulik J., et al. Implant complications, fusion, loss of lordosis, and outcome after anterior cervical plating with dynamic or rigid plates: two-year results of a multi-centric, randomized, controlled studies. Spine (Phila Pa 1976). 2009;34:641-646.
1. Benzel E.C. Biomechanics of spine stabilization, ed 2. Rolling Meadows, IL: American Association of Neurologic Surgeons Publications; 2001.
2. DiAngelo D.F., Foley K.T., Vossel K.A., et al. Anterior cervical plating reverses load transfer through multilevel strut grafts. Spine (Phila Pa 1976). 2000;25:783-795.
3. Matsunaga S., Kabayama S., Yamamoto T., et al. Strain on intervertebral discs after anterior cervical decompression and fusion. Spine (Phila Pa 1976). 1999;24(7):670-675.
4. Bishop R.C., Moore K.A., Weidner A., et al. Anterior cervical interbody fusion using autogeneic and allogeneic bone graft substrate: a prospective comparative analysis. J Neurosurg. 1996;85:206-210.
5. Geer C.P., Papadopoulos S.M. Instrumentation after anterior cervical fusion: the argument for single-level anterior cervical discectomy and fusion with anterior plate fixation. Clin Neurosurg. 1999;45:25-29.
6. Hughes S.S., Pringle T., Phillips F., Emery S., et al. Settling of fibula strut grafts following multilevel anterior cervical corpectomy: a radiographic evaluation. Spine (Phila Pa 1976). 2006;31(17):1911-1915.
7. Lim T.H., Kwon H., Jeon C.H., et al. Effect of endplate conditions and bone mineral density on the compressive strength of the graft-endplate interface in anterior cervical spine fusion. Spine (Phila Pa 1976). 2001;26:951-956.
8. Connolly P.J., Esses S.I., Kostuik J.P. Anterior cervical fusion: outcome analysis of patients fused with and without anterior cervical plates. J Spinal Disord. 1996;9:202-206.
9. Kostuik J.P., Connolly P.J., Esses S.I. Anterior cervical plate fixation with the titanium hollow screw plate system. Spine (Phila Pa 1976). 1993;18:1273-1278.
10. Shapiro S. Banked fibula and the locking anterior cervical plate in anterior cervical fusions following cervical discectomy. J Neurosurg. 1996;84:161-165.
11. Swank M.L., Lowery G.L., Bhat A.L. Anterior cervical allograft arthrodesis and instrumentation: multiple interbody grafting or strut graft reconstruction. Eur J Spine. 1997;6:138-143.
12. Vaccaro A.R., Balderston R.A. Anterior plate instrumentation for disorders of the subaxial cervical spine. Clin Orthop Relat Res. 1997;335:112-121.
13. Wang J.C., McDonough P.W., Endow K.K. Increased fusion rates with cervical plating for two-level anterior cervical discectomy and fusion. Spine (Phila Pa 1976). 2000;25:41-45.
14. Tippets R.H., Apfelbaum R.I. Anterior cervical fusion with the Caspar instrumentation system. Neurosurgery. 1988;22:1008-1013.
15. Benzel E.C., Yuan H.A., Weidner A., et al. Controlled intervertebral settling in multiple level ventral cervical fusion procedures with a dynamic stabilization implant. Rancho Mirage, CA: Presented at American Academy of Neurologic Surgeons Spine Section Meeting; 1998.
16. Chinoy M.A., Parker M.J. Fixed nail plates versus sliding hip systems for the treatment of trochanteric femoral fractures: a meta analysis of 14 studies. Injury. 1999;30:157-163.
17. Benzel E.C., Larson S.J. Operative stabilization of the posttraumatic thoracic and lumbar spine: a comparative analysis of the Harrington distraction rod and the modified Weiss spring. Neurosurgery. 1986;19:378-385.
18. Epstein N. Anterior approaches to cervical spondylosis and ossification of the posterior longitudinal ligament: review of operative technique and assessment of 65 multilevel circumferential procedures. Surg Neurol. 2001;55:313-324.
19. Apfelbaum R.I., Dailey A.T., Soleau S., et al. Clinical experience with a new load-sharing anterior cervical plate. In: Watanabe K., editor. Developments in neuroscience: Proceedings of the 2nd International Mt. BANDAI Symposium for Neuroscience, Spine Section. Philadelphia: Elsevier; 2002:563-580.
20. Epstein N.E. Anterior cervical dynamic ABC plating with single level corpectomy and fusion in forty-two patients. Spinal Cord. 2003;41:153-158.
21. Pitzen T.R., Chrobok J., Stulik J., et al. Implant complications, fusion, loss of lordosis, and outcome after anterior cervical plating with dynamic or rigid plates: two-year results of a multi-centric, randomized, controlled studies. Spine (Phila Pa 1976). 2009;34:641-646.
22. Steinmetz M.P., Warbel A., Whitfield M., Bingaman W. Preliminary experience with the DOC dynamic cervical implant for the treatment of multi-level cervical spondylosis. J Neurosurg. 2002;97(Suppl 3):330-336.
23. Bose B. Anterior cervical arthrodesis using DOC dynamic stabilization implant for improvement in sagittal angulation and controlled settling. J Neurosurg. 98(Suppl 1), 2003. 8–13
24. Saphier P.S., Arginteanu M.S., Moore F.M., et al. Stress-shielding compared with load-sharing anterior cervical plate fixation: a clinical and radiographic prospective analysis of 50 patients. J Neurosurg Spine. 2007;6:391-397.
25. Brodke D.S., Gollogly S., Alexander Mohr R., et al. Dynamic cervical plates: biomechanical evaluation of load sharing and stiffness. Spine (Phila Pa 1976). 2001;26:1324-1329.
26. Epstein N. Anterior dynamic plates in complex cervical reconstructive surgeries. J Spinal Disord Tech. 2002;15:221-227.
27. Khoo L.T., Kim A., Laich D.T., et al. Anterior plating of cervical trauma: the effect of plate design on graft subsidence and the preservation of segmental lordosis. Chicago, IL: Presented at 2002 American Association of Neurological Surgeons Meeting; April 2002.