Chapter 20 Structure, Function, and Pathology of Bruch’s Membrane
For additional online content visit http://www.expertconsult.com
Introduction, history, embryology
Bruch’s membrane is a thin (2–4 µm), acellular, five-layered extracellular matrix located between the retina and choroid.1,2 It extends anteriorly to the ora serrata, interrupted only by the optic nerve. Tissue resembling Bruch’s membrane is visible anterior to the ora serrata extending forward to the pigmented epithelium of the ciliary body. Bruch’s membrane lies between the metabolically active retinal pigment epithelium (RPE) and a capillary bed (choriocapillaris) and thus serves two major functions as the substratum of the RPE and a vessel wall. It has major clinical significance because of its involvement in age-related macular degeneration (AMD) and other chorioretinal diseases.
Early history
Carl Ludwig Wilhelm Bruch first isolated the “lamina vitrea” that we now know as Bruch’s membrane, and described it in his 1844 doctoral thesis,3,4 where he also first described the tapetum found in many mammals. By light microscopy, Bruch’s membrane appeared transparent with little internal structure. Later studies by Smirnow5 divided this membrane into an outer elastic layer (first described by Sattler in 1877) and an inner cuticular layer, separated by a dense plexus of very fine elastic fibers.6,7
Development of Bruch’s membrane
The bipartite character of Bruch’s membrane arises from the embryology of its tissue. When the optic cup invaginates and folds, its inner layer forms the neural retina, and its outer layer forms the RPE. The RPE lies in contact with mesenchyme. At this apposition, Bruch’s membrane forms by 6–7 weeks’ gestation. Thus, its inner layer is composed of ectodermal tissue and its outer layer is composed of mesodermal tissue. At the border of two layers, the elastic layer forms last, becoming histologically visible by 11–12 weeks.8–10
The collagen that fills the extracellular space and the later-appearing elastin appear to be made by invading fibroblasts and the filopodia of endothelial cells lining the adjacent choriocapillaris. The two basal laminas are produced by their associated cell layers.11 In addition to collagen IV subunits specific to specialized basal lamina, RPE expresses genes for structural collagen III and angiostatic collagen XVIII in a developmentally regulated manner linked to photoreceptor maturation.12
By week 13, fenestrations are apparent in the endothelium facing Bruch’s membrane,10 indicating that, at this stage, transport across this tissue may be functional. Choroidal endothelial cells originate from paraocular mesenchyme. Development of the choroidal vasculature, and Bruch’s as part of it, depends on differentiated RPE and its production of inductive signals, including basic fibroblast growth factor and vascular endothelial growth factor (VEGF).13
Structure of Bruch’s membrane in the young adult eye
Hogan’s five-layer nomenclature for Bruch’s membrane14 is commonly used. Gass proposed a three-layer system that did not include the cellular basal laminas as part of Bruch’s proper.15 These layers are shown in Fig. 20.1 and their constituents are given in Table 20.1.
Table 20.1 Structural and molecular components of Bruch’s membrane
Layer (common abbreviation) | Component; age change | References |
---|---|---|
Basal laminar deposit (BlamD) | + Fibronectin, laminin, IV α4–5, VI, endostatin, EFEMP1 | 164, 167, 206–209 |
RPE basal lamina (RPE-BL) | IV α1–5, V, laminins 1, 5, 10, and 11, nidogen-1, heparan sulfate, chondroitin sulfate | 18, 19, 21, 66, 210, 211 |
Lipid wall/basal linear deposit (BlinD) | + Lipoproteins | 38, 39, 212 |
Inner collagenous layer (ICL) | I, III, V, fibronectin, chondroitin sulfate, dermatan sulfate, lipoproteins ↑, apoE, heme, clusterin, vitronectin | 34, 35, 38, 39, 50, 66, 146, 152, 210, 213–215 |
Elastic layer (EL) | Elastin ↑, calcium phosphate ↑ | 14, 66–68, 210, 216 |
Outer collagenous layer (OCL) | I, III, V, fibulin-5, fibronectin, chondroitin sulfate, dermatan sulfate, lipoproteins ↑, apoE, clusterin | 21, 39, 50, 152, 210, 215, 217 |
ChC-basal lamina | IV α1, 2, V, VI, laminin, heparan sulfate, chondroitin sulfate, endostatin | 18, 208, 210, 211, 218 |
Bruch’s, throughout or layer not specified | I ↑, collagen solubility ↓, perlecan, MMP-2 ↑, MMP-9 ↑, TIMP-2; TIMP-3 ↑, pentosidine ↑, CML ↑, GA-AGE ↑, RGR-d, apoB, oxidized apoB-100, 7-KCh, LHP, HHE ↑, DHP-lys ↑, C3d ↑, C5b-9 ↑, pentraxin-3 ↑, thrombospondin-1, zinc | 62, 66, 138, 139, 147, 218–230 |
Table shows definitely localized components. Most determinations were made in macula. Studies showing histochemical/immunohistochemical verification of biochemistry and ultrastructural validation of structures identified by light microscopy techniques were given greater weight. Localizations were assigned to specific layers if immunogold-electron microscopy or high-magnification confocal microscopy images were available. Roman numerals denote collagens. Components are ordered within each layer: structural components, lipoproteins, extracellular matrix and its regulation, modified lipids and proteins, complement/immunity, cellular response/activity, metals. Known changes with advancing age are bold with an arrow indicating direction of change. New additions with age are shown with a plus (+). Plain text means no change or not tested.
CML, carboxymethyl-lysine226; 7-KCh, 7 keto-cholesterol229; GA-AGE, glycolaldehyde-derived advanced glycation end products221; HHE, 4-hydroxyhexenal66,218; DHP-lys, dihydropyridine lysine.66
RPE basal lamina (RPE-BL)
This ∼0.15-µm-thick layer is a meshwork of fine fibers like other basal laminas in the body.16,17 The RPE-BL resembles that of the choriocapillaris endothelium but does not contain collagen VI. The RPE-BL contains collagen IV α3–5,18 like that of kidney glomerulus, another organ with specialized filtration and transport functions. The RPE synthesizes specific laminins that preferentially adhere Bruch’s membrane to the RPE through interaction with integrins.19
Inner collagenous layer (ICL)
The ICL is ∼1.4 µm thick and contains 70-nm-diameter fibers of collagens I, III, and V in a multilayered criss-cross, parallel to the plane of Bruch’s membrane.1 The collagen grid is associated with interacting molecules, particularly the negatively charged proteoglycans chondroitin sulfate and dermatan sulfate.20,21
Elastic layer (EL)
The EL consists of stacked layers of linear elastin fibers, crisscrossing to form a 0.8-µm-thick sheet with interfibrillary spaces of ∼1 µm. This sheet extends from the edge of the optic nerve to the ciliary body pars plana.1 In addition to elastin fibers, the EL contains collagen VI, fibronectin, and other proteins, and collagen fibers from the ICL and outer collagenous layer (OCL) can cross the EL. Some EL elastin fibers are said to cross the tissue space between the choriocapillaris and join bundles of choroidal elastic tissue.22 The EL confers biomechanical properties, vascular compliance, and antiangiogenic barrier functions. It is more discontinuous in the macula, perhaps explaining why choroidal neovascularization (CNV) is more prominent there.23 This concept is supported by the extensive laser-induced neovascularization in mice deficient in lysyl oxidase-like 1, an enzyme required for elastin polymerization.24
Outer collagenous layer
The OCL contains many of the same molecular components as the ICL, and the collagen fibrils running parallel to the choriocapillaris additionally form prominent bundles. This layer, unlike the ICL, has periodic outward extensions between individual choriocapillary lumens called intercapillary pillars, where thickness cannot be determined due to the lack of a boundary. Between pillars, OCL thickness can range from 1 to 5 µm.25
Choriocapillaris basal lamina (ChC-BL)
This 0.07-µm-thick layer is discontinuous with respect to Bruch’s membrane due to the interruptions of the intercapillary pillars of the choroid. It is continuous with respect to the complex network of spaces defined by the choriocapillary lumens because the basal lamina envelops the complete circumference of the endothelium. A remarkable structural feature of the adjacent choriocapillary endothelium is fenestrations that are permeable to macromolecules (Fig. 20.2).26 This basal lamina may inhibit endothelial cell migration into Bruch’s membrane, as do basal laminas associated with retinal capillaries.27
Bruch’s membrane in an aged eye
Aging is the largest risk factor for developing AMD,28 and Bruch’s membrane undergoes significant age-related changes. Identification of factors predisposing to disease progression is a priority. This task has been challenged by difficulty imposed by the thinness of the tissue, and the closely integrated functions of RPE, Bruch’s, and choriocapillaris. Current opinion holds that RPE and Bruch’s membrane age in concert, and normal Bruch’s membrane aging transforms insidiously into AMD pathology.1,16,17,29 This section covers aging, to inform the following section on function.
Lipid accumulation: Bruch’s membrane lipoproteins
Early electron microscopists described aged Bruch’s membrane as being filled with debris, including amorphous electron-dense material, membrane fragments, vesicles, and calcification.1,25 Debris deposition in ICL and OCL begins in the second decade in the macula and is delayed in equatorial regions, a regional lag also reported for individual components.30 Identifying this material has been a fruitful approach to understanding antecedents of disease.
Most prominent among the changes in Bruch’s membrane is a profound accumulation of lipids. Clinical observations on fluid-filled RPE detachments in older adults led to Bird and Marshall’s hypothesis that a lipophilic barrier in Bruch’s blocked a normal, outwardly directed fluid efflux from the RPE31 (as opposed to leakage from CNV). This hypothesis motivated a seminal histochemical study by Pauleikhoff et al.32 that demonstrated oil red O-binding material (esterified cholesterol (EC), triglyceride (TG), fatty acid) localized exclusively to Bruch’s membrane, unlike other stains. This lipid was absent <30 years, variably present at 31–60 years, and abundant at ≥61 years.33,34 A specific fluorescent marker, filipin, which binds the 3β-hydroxy group of sterols to reveal unesterified (free) cholesterol (UC) or EC depending on tissue pretreatment,35 indicated that EC is a prominent component of the oil red O-binding deposition.35 Macular EC rose linearly from near zero at age 22 years to reach high and variable levels in aged donors. EC was detectable in periphery at ∼1/7 macular levels and increased significantly with age. Hot-stage polarizing microscopy34 similarly demonstrated prominent age-related increases in EC in Bruch’s membrane, manifest as liquid crystals (“Maltese crosses”) when examined through a polarizing filter. Few birefringent crystals signifying the neutral lipid TG were found.
Histochemical, ultrastructural, biochemical, gene expression, and cell biological evidence now indicate that the EC-rich material accumulating with age in Bruch’s membrane is a lipoprotein-containing apolipoprotein B, assembled by the RPE.36 This process, ongoing throughout life yet first revealed by aging, has implications for the formation of AMD-specific lesions, intraocular transport, RPE physiology, nutrition of outer retina, and maintenance of photoreceptor health. In 1926, Verhoeff and Sisson speculated that lipid deposition might precede Bruch’s membrane basophilia and fragmentation, common in older eyes due to “lime salts [calcification] in the elastic layer.”37
Ultrastructural studies described in Bruch’s membrane of older eyes36 numerous small (<100 nm), round, electron-lucent vesicular profiles, implying aqueous interiors. Lipid-preserving preparation techniques together with extraction studies show that these so-called vesicles are actually solid, lipid-containing particles, now considered lipoproteins (Fig. 20.3B). These methods include postfixation in osmium paraphenylenediamine (OTAP)35 and, most strikingly, quick-freeze/deep-etch (QFDE), a freeze fracture method with an etching step to remove frozen water.38–40 Particles vary in size from 60 to 100 nm but could be as large as 300 nm, occasionally appearing to coalesce (Fig. 20.3).
Lipoprotein particles are first seen among fibrils of the elastic layer in early adulthood, extending inward ultimately to fill most of the open space of the ICL by the seventh decade of life.40 Most fatefully, a new layer, the lipid wall,38 then forms with solid particles stacked 3–4 deep occupying nearly 100% of a space between RPE basal lamina and OCL of many older eyes. The lipid wall displaces ICL collagen fibrils that anchor the RPE basal lamina (Fig. 20.3). It is considered a precursor to basal linear deposits, a specific lesion of AMD (see below).
Lipoprotein composition can provide clues to sources of its components.41 When isolated (Fig. 20.4A), Bruch’s membrane lipoproteins are found to be EC-enriched (EC/total cholesterol = 0.56; EC/TG = 4–11; Fig. 20.4B). For comparison, hepatic very-low-density lipoprotein (VLDL), of similar diameter, is TG-rich. An early report of TG-enriched Bruch’s membrane neutral lipid42 was not replicated. Abundant EC points to the only mechanism by which neutral lipids are released directly from cells, an apoB-containing lipoprotein, like hepatic VLDL or intestinal chylomicrons. Significantly, RPE expresses the apoB gene and protein, along with microsomal triglyceride transfer protein (MTP), required for apoB lipidation and secretion. Lack of functional MTP is the basis of abetalipoproteinemia, a rare inherited disorder that includes a pigmentary retinopathy.43,44 The combination of apoB and MTP within native RPE marks these cells as constitutive lipoprotein secretors.45 Secretion of full-length apoB has been demonstrated in rat-derived and human-derived RPE cell lines.46,47 Consistent with an RPE origin, particles first appear in the elastic layer of Bruch’s membrane and fill in towards the RPE.39
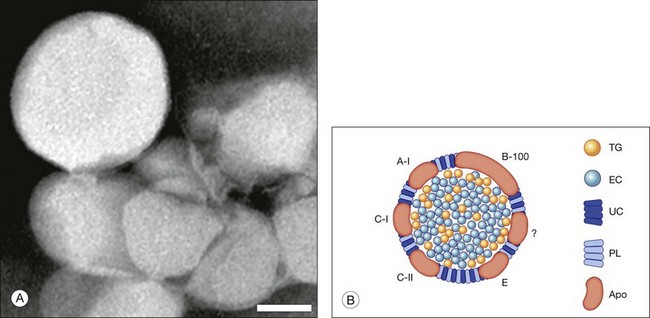
Fig. 20.4 Bruch’s membrane lipoprotein composition. (A) Lipoprotein particles isolated from Bruch’s membrane are large and spherical; negative stain.153 (Source: Li C-M, Chung BH, Presley JB, et al. Lipoprotein-like particles and cholesteryl esters in human Bruch’s membrane: initial characterization. Invest Ophthalmol Vis Sci 2005;46:2576–86). Bar = 50 nm. (B) Bruch’s membrane lipoprotein composition inferred from direct assay,50,153 (Sources: Wang L, Li C-M, Rudolf M, et al. Lipoprotein particles of intra-ocular origin in human Bruch membrane: an unusual lipid profile. Invest Ophthalmol Vis Sci 2009;50:870–7) (Li C-M, Chung BH, Presley JB, et al. Lipoprotein-like particles and cholesteryl esters in human Bruch’s membrane: initial characterization. Invest Ophthalmol Vis Sci 2005;46:2576–86), druse composition, and retinal pigment epithelium gene expression.139,154 (Sources: Malek G, Li C-M, Guidry C, et al. Apolipoprotein B in cholesterol-containing drusen and basal deposits in eyes with age-related maculopathy. Am J Pathoi 2003;162:413–25) and (TG, Li C-M, Clark ME, Chimento MF, et al. Apolipoprotein localization in isolated drusen and retinal apolipoprotein gene expression. Invest Ophthalmol Vis Sci 2006;47:3119–28) TG, triglyceride; EC, esterified cholesterol; UC, unesterified cholesterol; PL, phospholipid; Apo, apolipoproteins. The question mark signifies that not all apolipoproteins are known.
Indirect evidence that Bruch’s membrane lipoproteins are of intraocular origin also emerges from the epidemiologic literature. If the EC deposition in Bruch’s membrane and AMD-associated lesions were a manifestation of systemic perifibrous lipid and atherosclerosis, then a strong positive correlation between disease status and plasma lipoprotein levels, like that documented for coronary artery disease,48 might be expected but has not emerged.49
Identifying the upstream sources of Bruch’s membrane lipoprotein constituents is essential for understanding the biological purpose of this pathway and the prospects for eventual clinical exploitation. Studies using isolated lipoproteins from Bruch’s membrane50 and Bruch’s membrane choroid EC51 report a high mole percentage of linoleate (>40%) and low docosahexaenoate (<1%) for all lipid classes.52 This composition strongly points away from photoreceptor outer segments (35% docosahexaenoate in membrane phospholipids) as an upstream source, as long postulated,53,54 and towards plasma lipoproteins (45–55% linoleate in all lipid classes). These data have been interpreted to signify that plasma lipoproteins are major contributors upstream to an apoB lipoprotein of RPE origin. In contrast, the sources of UC in Bruch’s lipoproteins are not yet known and could be outer segments, plasma lipoproteins, endogenous synthesis, or a combination.
Lipoproteins may thus be assembled from several sources, including outer segments, remnant components from the photoreceptor nutrient supply system, and endogenous synthesis. According to this model,52 plasma lipoproteins serve as vehicles for delivery of lipophilic nutrients (carotenoids,55 vitamin E, and cholesterol56) to photoreceptors by RPE, which has functional receptors for low-density lipoprotein (LDL) and high-density lipoprotein.57,58 Nutrients are stripped from these lipoproteins by the RPE for delivery to the photoreceptors, and the remnants are repackaged for secretion into Bruch’s membrane as part of apoB-containing lipoproteins, where they begin to accumulate during age and become toxically modified to instigate inflammation in AMD.
Other aging changes
Bruch’s membrane thickens throughout adulthood (20–100 years) two- to threefold under the macula and becoming more variable between individuals at older ages.25,59,60 Equatorial Bruch’s membrane changes little while Bruch’s membrane near the ora serrata increases twofold during this time.25 In the macula, the OCL thickens more prominently than the ICL.61 A large ultrastructural study of 121 human donor eyes demonstrated that the macular EL is 3–6 times thinner than peripheral EL23 at all ages.
Unbalanced regulation of extracellular matrix molecules and their modulator matrix are thought to result in Bruch’s membrane thickening. Increased histochemical reactivity for glycoconjugates, glycosaminoglycans (GAGs), collagen, and elastin is seen in the macula relative to equator and near the ora serrata.25 Collagen solubility declines with age.62 Matrix metalloproteinases MMP-2 and MMP-3 increase with age, as does a potent tissue inhibitor of metalloproteinases, TIMP-3. TIMP-3 immunoreactivity reaches adult levels at 30 years of age near vasculature in lung, kidney, and in Bruch’s membrane, signifying the end of developmental organogenesis.63 The reduction or absence of TIMP-3 is proangiogenic, as this protein not only regulates metalloproteinases during the normal turnover of Bruch’s membrane matrix components, but it also binds to VEGF.64,65
The EL thickens with age but decreases relative to overall thickening of Bruch’s membrane.23 Thus elastin referenced to other Bruch’s constituents, as detected by Raman spectroscopy, decreases with age.66 Similar arguments can be made for collagen III and IV. A prominent age change,67 noted early,37 is calcification and ensuing brittleness. This process involves fine deposition of electron-dense particulate matter,14 confirmed as calcium phosphate68 on individual elastin fibrils.
Long-lived proteins like collagens are modified in vivo by nonenzymatic Maillard and free radical reactions to yield advanced glycation end products (AGEs) and the formation of lipid-derived reactive carbonyl species like 4-hydroxyhexenal and linoleate hydroperoxide, collectively called age-related lipoperoxidation end products (ALEs). Accumulation of AGEs and ALEs, characteristic of diabetes and atherosclerosis, also occurs in aging Bruch’s membrane (Table 20.1). Finally, other components more prominent in aged eyes include complement components C3d, C5b-9, and pentraxin-3, a homolog of the acute-phase respondent C-reactive protein. Thus, at the molecular level, aging Bruch’s membrane contains evidence of many biological activities, including remodeling, oxidative damage, and inflammation, in addition to lipoprotein accumulation.
Function of Bruch’s membrane
As a vessel wall of the choroid, Bruch’s membrane’s primary function is structural, like other vessel walls. Its architecture is similar to vascular intima, with a subendothelial extracellular matrix and elastic layer corresponding to the internal elastic lamina. The abluminal surface of Bruch’s differs from other vessel walls in that it abuts a basal lamina, that of the RPE. The luminal surface faces a fenestrated vascular endothelium and basal lamina, making Bruch’s membrane structurally analogous to the renal glomerulus and providing a basis for commonality between retinal and kidney disease.69–71 The importance of fluid and macromolecular transport across the renal glomerulus is well known.72 Transport is a second important function of Bruch’s membrane.
Structural role of Bruch’s membrane
Bruch’s membrane encircles more than half the eye and stretches with the corneoscleral envelope as intraocular pressure (IOP) increases. It therefore withstands this stretch and returns to its original shape when IOP decreases. This tissue also stretches to accommodate changes in choroidal blood volume. Finally, the choroid (and Bruch’s membrane with it) may act as a spring that pulls the lens during accommodation.73,74 For these reasons, then, Bruch’s membrane requires elasticity. Marshall and Hussain’s group estimated the modulus of elasticity in Bruch’s membrane choroid preparations to be 7–19 MPa.75 These values are similar to those of sclera (although sclera is much thicker and thus can support more load), consistent with the notion that Bruch’s membrane contributes to load bearing. After early adulthood, the modulus of elasticity of human Bruch’s membrane–choroid complex increases (P < 0.001) at a rate of ∼1% per year. Bruch’s membrane stiffness in AMD eyes does not differ from age-matched normals.76
Transport role of Bruch’s membrane
Hydraulic conductivity of Bruch’s membrane
GAGs are concentrated in the interphotoreceptor matrix77,78 and corneal stroma.79 In both locations, these highly charged macromolecules maintain geometric fidelity essential for vision (periodic collagen spacing for corneal transparency, orderly photoreceptor spacing for visual sampling78,80,81). GAGs generate significant swelling pressure (up to 50 mmHg in cornea).82,83 Without a mechanism to maintain tissue deturgescence, GAGs would imbibe fluid, swell, destroy tissue geometry, and interfere with visual function. Corneal endothelium forestalls swelling by continuously pumping fluid out. This function is accomplished for retina by the RPE, and its failure can lead to retinal detachment. A driving force adequate to overcome the collective flow resistance of RPE, Bruch’s membrane, and choriocapillaris endothelium is provided by a gradient in fluid pressure and oncotic pressure (the osmotic pressure generated by plasma proteins). This balance is embodied by Starling’s law that characterizes the relationship between fluid flux (q = flow per unit area; positive when flow is out of the blood vessel) across a capillary vessel wall and the forces driving this flow:
We can estimate the magnitude of ΔP – σΔΠ using measured value of q and Lp. The fluid pumping rate by human RPE has been measured as q = 11 µL/h/cm2, similar to that in other animals (Table 20.2). The hydraulic conductivity of macular Bruch’s membrane/choroid of healthy young humans ranges from 20 to 100 × 10−10 m/s/Pa.84 Then, using q = 11 µL/h/cm2 and Lp = 50 × 10−10 m/s/Pa, we can calculate that the magnitude of (ΔP − σΔΠ) necessary to drive this flow through Bruch’s membrane is roughly 0.05 mmHg. (This does not include the flow resistance of choriocapillaris endothelium, which is not measured when Lp of a Bruch’s membrane/choroidal preparation is determined. For this highly fenestrated endothelium, Lp can be estimated as roughly 25 × 10−10 m/s/Pa,85 which does not affect our conclusions below.)
Table 20.2 Retinal pigment epithelium (RPE) fluid pumping rates
Species | Fluid transport rate across RPE (µL/h/cm2) | References |
---|---|---|
Frog | 4.8–7.6 | 231, 232 |
Rabbit | 12 ± 4 | 233, 234 |
Canine | 6.4 | 235 |
Primate* | 14 ± 3 | 236, 237 |
Human | 11 | 238 |
RPE pumping rates were measured by readsorption of subretinal fluid or by direct measurement in culture.
* Cantrill and Pederson236 measured a much higher transport rate than that reported here, but used fluorescein as a tracer which likely does not track fluid flow due to its high diffusion coefficient.
σ can be roughly estimated by assuming that the fluid in the suprachoroidal space is in equilibrium with blood in the choroid. Using measurements of fluid pressure and of the plasma protein concentration (to estimate oncotic pressure) inside and outside the choriocapillaris,86–88 equation (1) can be used to find σ ≈ 0.5.
Allowing that Πcc = 27 mmHg,86 Pcc = IOP + 8 mmHg,87 and assuming that ΠRPE = 0 mmHg (fluid pumped by the RPE is assumed protein-free) and PRPE = IOP (assuming no pressure is generated by the RPE above that necessary for crossing Bruch’s), we find that ΔP – σΔΠ is approximately –5.5 mmHg pulling fluid into the choroid. Thus, in normal young adults, oncotic pressure within the choroid is more than sufficient to adsorb all the fluid pumped by the RPE. We can also use equation (1) to calculate that the lowest value of Lp that still adsorbs fluid pumped by the RPE without generating an elevated pressure at the RPE basal surface is Lp > 0.4 × 10−10 m/s/Pa.
Experiments using laser ablation of Bruch’s membrane/choroid explants allowed Starita et al.89 to conclude that the ICL was responsible for most of the flow resistance in Bruch’s membrane. Attempts to localize further the flow resistance using morphometric methods are complicated by first, stereological issues90 and second, the loss of ultrastructural fidelity from connective tissue conventionally processed for electron microscopy.38 Failure to appreciate the former difficulty can lead to unphysiologically low estimates for tissue porosity and thereby hydraulic conductivity.1
Age-related changes in hydraulic conductivity and disease
Fisher was the first to measure Lp of human Bruch’s membrane,91 finding that Lp decreased significantly with age. However, his values for Lp of Bruch’s membrane and other tissues are much lower than those found by later investigators.85,92,93 Marshall and Hussain’s group carefully revisited these measurements using Bruch’s membrane/choroid with RPE removed, a preparation that was simpler to create. They showed using laser ablation that the flow resistance of these preparations was entirely due to Bruch’s membrane.89 They also found that flow rate increased linearly with driving pressure, indicating that Lp of Bruch’s membrane is relatively insensitive to pressure up to 25 mmHg.
They reported that Lp of macular Bruch’s membrane exhibited a dramatic, exponential decline throughout life (Fig. 20.5), dropping from 130 × 10−10 m/s/Pa in young children to 0.52 × 10−10 m/s/Pa in old age. Lp of macular Bruch’s membrane dropped more rapidly with age than did that of the periphery, consistent with an accelerated process occurring in the macula.1,84,94,95 Note that the lowest value measured for Lp of Bruch’s membrane in normal eyes is similar to the calculated minimum value of Lp that allows complete fluid resorption (0.4 × 10−10 m/s/Pa; see above). Marshall and Hussain’s group reached similar conclusions regarding this process.94
Determining Lp of Bruch’s membrane in isolated macular samples of AMD eyes is difficult due to scar formation and other changes.94 However, Marshall and Hussein’s group showed that, in the periphery, Lp of Bruch’s membrane is decreased in AMD eyes as compared to age-matched normal eyes (Fig. 20.5).94 Assuming that similar processes occur in macular Bruch’s membrane due to the profound lipid accumulation in this region, then in diseased eyes, the RPE must generate higher pressures at its basal surface to drive fluid into the choriocapillaris, with further pathological consequences.31 Above an unknown threshold level, higher pressure will cause the RPE-BL to separate from the ICL, leading to RPE detachment and fluid accumulation, as seen in 12–20% of AMD patients.94
What causes the dramatic age-related decrease in Lp of Bruch’s membrane? It is natural to suspect the age-related lipid accumulation. In fact, McCarty et al.96 showed that lipid particles trapped in an extracellular matrix can generate very significant flow resistance, more than would be expected based simply on their size and number. However, Marshall and Hussain’s group observed that most of the marked change in Lp occurred before age 40 (Fig. 20.6A) while the increase in Bruch’s membrane lipid content occurred largely after this age. They thus concluded that other age-related changes must be responsible for changes in Lp.1,84
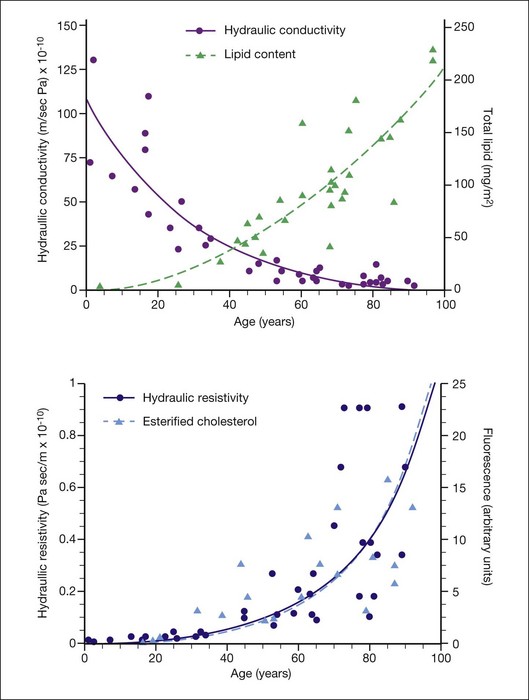
Fig. 20.6 (A) Hydraulic conductivity of human macular Bruch’s membrane/choroidal preparations as a function of age, as compared to lipid accumulation in human macular Bruch’s membrane; lines as exponential fits to the data. (B) Hydraulic resistivity of human macular Bruch’s membrane/choroidal preparations as a function of age,1 as compared to esterified cholesterol accumulation in human macular Bruch’s membrane35; lines are exponential fits to the data (the fits nearly overlie one another).
(Modified with permission from Marshall J, Hussain AA, Starita C, et al, editors. The retinal pigment epithelium: function and disease. New York: Oxford University Press; 1998. pp. 669–92.)
A different conclusion can be reached from examining age-effects on flow resistivity the inverse of Lp. Resistivity increases from a low of roughly R = 108 Pa/m/s for young individuals to R = 1010 Pa/m/s for aged persons. Thus, when hydraulic conductivity Lp drops from roughly 100 × 10−10 m/s/Pa to 25 × 10−10 m/s/Pa between birth and 40 years of age, 75% of its total possible decrease, resistivity R increases from 1 × 108 Pa/m/s to 4 × 108 Pa/m/s, only 4% of the ultimate increase. Simply put, hydraulic conductivity drops more rapidly with age at young ages because its value is high to start with. Fig. 20.6B plots resistivity and histochemically detected EC against age for Bruch’s membrane.97 The agreement between the trends and the fits to the data is striking. This is strong evidence that the increasing lipid content and progressively hydrophobic character of Bruch’s membrane are responsible for impairing fluid transfer with age, as postulated.31 The strong correlation between flow resistivity of Bruch’s membrane and lipid content was likewise found by Marshall and Hussain’s group.1,84,95 Laser ablation studies localizing flow resistance to the ICL89 further support this conclusion, because lipids accumulate prominently in the ICL with aging.39 Further, more laser pulses were required to abolish flow resistance in the oldest eyes, consistent with presence of a lipid wall, requiring prior removal.
Thus, it appears that decreased Lp and increased resistivity of Bruch’s membrane with aging are closely related to the age-related accumulation of lipids, primarily EC. Lipids accumulate more rapidly in the macular Bruch’s membrane than in the periphery.35,98 Thus, Lp of the macula decreases more rapidly with age than it does in the periphery.
Permeability of Bruch’s membrane to solute transport
Along with bulk fluid flow, there is significant transport of individual molecular species across Bruch’s membrane, including dissolved gases, nutrients, cytokines, and waste products driven by passive diffusion. Flow crossing Bruch’s membrane is too slow to influence this process. This can be seen through calculation of the Peclet number, the relative magnitude of convection of a species due to bulk flow to that of diffusion99:
where V is the velocity of the flow, L is the transport path length, and D0 the free diffusion coefficient of the species being transported. (The free diffusion coefficient in saline is used rather than its value in tissue, since the species carried by flow is constrained to the same extent by the tissue as is its diffusion). Using the RPE pumping rate (Table 20.2) for V, Bruch’s membrane thickness (average of 3 µm59) for L, and a range of diffusion coefficients of species crossing Bruch’s membrane (2 × 10−7 cm2/s for LDL to 2 × 10−5 cm2/s for oxygen99,100), we find that the Peclet number ranges in value from 5 × 10−5 to 5 × 10−3. Thus, convection is negligible in transporting species across Bruch’s membrane under physiological conditions.
The transport of amino acids,101 serum proteins,102 drugs,103 and LDL104 across Bruch’s membrane has been examined. There are technical challenges to these experiments. First, as indicated in equation (3), diffusional flux depends on the length of the tissue. Since the diffusion coefficient of the transported species is likely different in Bruch’s membrane than in the choroid in a combined preparation, but the path lengths of both tissue components are usually not determined, it is difficult to use the measured values to determine absolute values of permeability. Instead the more easily measured flux rate (j: see equation 3) is usually presented. Second, since larger macromolecules used in diffusion studies are hindered in their passage into the tissue, an oncotic pressure could develop, generating a fluid flow in the opposite direction of transport and thus complicating the results. Nonetheless, useful comparative results can be generated.
The transport rate across human Bruch’s membrane declines linearly with age for all molecules measured. Amino acids exhibited permeabilities of 0.6 × 10−4 cm/s (phenylalanine) to 1.2 × 10−4 cm/s (glycine) for young Bruch’s membrane and exhibited a modest decline (twofold or less) with aging.101 Serum proteins decrease more markedly, dropping from 3.5 × 10−6 cm/s in the first decade to 0.2 × 10−6 cm/s in the ninth decade, a >10-fold decrease.102 In particular, proteins larger than 100 kDa have significantly decreased flux through Bruch’s membrane of older individuals. Macular Bruch’s membrane showed a steeper decrease with age than did the periphery.105 Permeability was reduced in eyes with AMD relative to age-matched normal eyes.105
Decreased permeability of Bruch’s membrane to transport is likely due to a decrease in diffusion coefficients, especially for the larger species affected by interaction with extracellular matrix and lipoproteins. As indicated in equation (3), increased path length due to age-related thickening of Bruch’s membrane59 could also have a significant effect.
An original proposal of a molecular weight exclusion limit to Bruch’s membrane macromolecule transport of 66–200 kDa101,102 has been questioned by more recent work suggesting that, if such a limits exists, it is much higher.105 Because of the importance of lipoproteins in transporting lipophilic nutrients to the RPE for ultimate use by the photoreceptors, and also because lipoproteins accumulate with age in Bruch’s membrane, Cankova et al.104 specifically examined the reflection coefficient of bovine Bruch’s membrane to plasma LDL. They measured a reflection coefficient of 0.58 (compared to a reflection coefficient of arterial endothelium to LDL of 0.998 and arterial intima to LDL of 0.827106). Thus, while LDL did not pass freely through Bruch’s membrane, it could nonetheless pass. Hussain et al.105 also concluded that particles as large as LDL could cross Bruch’s membrane. Accordingly, RPE cells have been shown to internalize plasma LDL from the choroid.56,107,108
These considerations are relevant not only to understanding mass transfer between the choriocapillaris and the RPE, but also for transscleral drug delivery strategies, including antiangiogenic agents for treating AMD and steroids for treating diabetic retinopathy.109,110 Lipophilic solutes are significantly hindered in their transport by Bruch’s membrane/choroid,103 while hydrophilic moieties are blocked by the RPE.111
Summary and implications
Bruch’s membrane’s physiological roles are structural and facilitating transport. Transport across Bruch’s membrane is increasingly hindered with age, due at least partly to the marked age-related accumulation of EC-rich lipoproteins in this tissue, impeding pumping of fluid from RPE.94 A ≥90% decrease in transport of some species from the choroid102,105 may include lipophilic essentials delivered by lipoproteins. This decline in transport capability is thought to have functional consequences for photoreceptors.112 A well-characterized change occurring through the lifespan of individuals with healthy maculas is slowed dark adaptation,113 attributed to impaired translocation of retinoids across the RPE–Bruch’s interface. This slowing, worse in AMD patients,114,115 can be partly ameliorated by short-term administration of high-dose vitamin A,116 presumably overcoming the translocation deficit via mass action.
Pathology of Bruch’s membrane
AMD lesions
In aging and AMD, characteristic extracellular lesions accumulate in tissue compartments anterior to the ICL. Known as drusen and basal deposits,29,117 these lipid-containing aggregations ultimately impact RPE and photoreceptor health by impairing transport, causing inflammation, and predisposing to CNV (Fig. 20.7). Basal linear deposit (BlinD) forms consequent to lipoprotein accumulation in Bruch’s membrane and formation of the lipid wall, likely involving oxidation of individual lipid classes and local inflammation. Drusen could form by similar mechanisms, plus lipoprotein aggregation and other undefined processes that cause the distinctive dome shape of these lesions. Basal laminar deposit (BlamD) forms in parallel with lipid deposition in Bruch’s and may indicate RPE stressed by it. We begin by discussing drusen, due to their importance in AMD.
Drusen
In a fundus view, drusen are yellow-white deposits 30–300 µm in diameter posterior to the RPE. By optical coherence tomography, they appear as variably hyporeflective spaces in the same location.118 Histologically, drusen are focal, domed lesions between the RPE basal lamina and the ICL, i.e., in the same sub-RPE tissue compartment as the lipid wall and BlinD. Found in most older adults,67,119 drusen are more numerous in peripheral retina than in macula.120–122 Drusen are typically classified as “hard” and “soft” by the appearance of their borders. Soft drusen confer high risk of advanced disease123–126 and, importantly, are found only in the macula.122 Other rare druse types exist and are less well characterized.127
In separate 1854 publications, Donders (a Dutch ophthalmologist) and Wedl (an Austrian pathologist) described “colloid bodies” (Colloidkugeln) or “hyaline deposits” on the inner surface of the choroid in older or diseased human eyes3,128,129 (translated by Busk). Both authors interpreted the droplets that filled these deposits as “fat globules.” The term “drusen” originated with Müller in 1856, from the German word for the geological term geode (not to be confused with Drüse, meaning gland).130 The name drusen was adopted by English writers early in the 20th century,131 yet “colloid body” was used by Holloway and Verhoeff into the 1920s.132 The basis of the fatty content emerged slowly. Lauber133,134 noted that deposits between the lamina vitrea and the RPE were sudanophilic in 1924. Wolter and Falls135 stated that “hyaline bodies [drusen] … stain reddish with … oil red O” in 1962.
Extant theories for druse formation, extending back to their discovery,130 fit into two general categories: transformation of the overlying RPE and deposition of materials on to Bruch’s membrane. The latter is now accepted.129,135 The RPE has been implicated as a source of many druse components, via budding of membrane-bound packets of cytoplasm or secretion. The contribution of plasma-derived components, in contrast, has not been well characterized. The existence of druse subregions additionally suggests remodeling in the extracellular compartment, such as cellular invasion and enzymatic activity23,136–138 and uplifting of the lipid wall.35,139
Most prominent among druse constituents are lipids, an observation made by their earliest discoverers. All drusen contain EC and UC, in addition to phosphatidylcholine, other phospholipids, and ceramides.34,35,137,139–142 Extractable lipids account for ≥40% of hard druse volume143 and likely more for macular soft drusen.139 This includes large EC-rich lakes in soft drusen (Fig. 20.8), reminiscent of atherosclerotic plaques.144 Only half of macular drusen take up hydrophilic fluorescein in angiography,145 possibly reflecting differing proportions of polar and neutral lipids in individual lesions.141 Discrete nonlipid components in some drusen include amyloid assemblies and granules of lipofuscin or melanin, indicating cellular origin (Table 20.3, online). Other constituents present in all drusen include vitronectin, TIMP-3, complement factor H, complement components C3 and C8, crystallins, and zinc.23,136,138,146–150
Apolipoprotein immunoreactivity appears in drusen with high frequency (100%, apoE; >80% apoB; 60%, A–I).139,151–154 Plasma-abundant apoC-III is present in fewer drusen than plasma-sparse apoC-I, indicating a specific retention mechanism for plasma-derived apolipoproteins or an intraocular source. Importantly, hard drusen contain many solid, Folch-extractable electron-dense particles of the same diameter as the lipoproteins that accumulate with age in Bruch’s membrane. These observations together with the appearance of membranous debris in soft drusen (below) make an RPE-secreted apoB-containing lipoprotein particle an efficient mechanism to place multiple lipids and apolipoproteins within lesion compartments.
The principal lipid-containing component of soft drusen and BlinD was called “membranous debris” by the Sarks.123,155,156 These lesions are richer in histochemically detectable UC than surrounding cellular membranes.141,142 By transmission electron microscopy following osmium tetroxide postfixation, membranous debris appears as variably sized, contiguous coils of uncoated membranes consisting of uni- or multilamellar electron-dense lines, denser than cellular membranes, surrounding an electron-lucent center (Fig. 20.1). Since conventional ultrastructural preparation methods can remove lipids, the building blocks of membranous debris are more plausibly the UC-rich exteriors of lipoproteins (native and fused) whose neutral lipid interiors are not well preserved in postmortem tissue.39,153,157 Rather than vesicles, then, membranous debris is likely aggregated or fused particles that could collectively account for the abundant EC in sub-RPE deposits. EC abundance and ultrastructural evidence for solid, nonvesicular particles suggest that the major lipid-containing component of AMD-specific lesions can be called “lipoprotein-derived debris” rather than membranous debris.
Basal linear deposit
BlinD is a thin (0.4–2 µm) layer located in the same sub-RPE compartment as soft drusen. BlinD is not visible clinically except as associated with other pathology. By OTAP and QFDE, BlinD is rich in solid lipoprotein particles and lipid pools (Fig. 20.9A, C). BlinD and soft drusen are considered alternate forms (layer and lump) of the same entity158 and may interchange over time. Soft drusen are oily, difficult to isolate individually, and are biomechanically more fragile than hard drusen,122 properties applicable to BlinD by inference. Both lesions could thus be permissive to invading capillaries of type I CNV.159,160 ApoE and apoB are present in BlinD and its precursor, the lipid wall.139,151,152 Transitional morphologies between lipid wall and BlinD have been reported.161
Basal laminar deposit
BlamD forms small pockets between the RPE and the RPE-BL in many older normal eyes or a continuous layer as thick as 15 µm in AMD eyes142,156,162 (Fig. 20.7). Some authors consider a continuous layer of BlamD a histological definition of AMD.163 Ultrastructurally, BlamD resembles basement membrane material (Fig. 20.9B), containing laminin, fibronectin, type IV and type VI collagen.164–167 The latter is a distinctive banded material with 120 nm periodicity, called wide- or long-spacing collagen, which also appears in other ocular locations like epiretinal membranes. Thick BlamD, associated with advanced AMD risk,156 contains histochemically detectable lipid, including UC and EC141,142 and is a classically described site for membranous debris (Fig. 20.1C). By lipid-preserving methods, solid particles are seen in BlamD (Fig. 20.9A, B). Especially enriched in basal mounds156 (Fig. 20.7), lipoprotein-derived debris in BlamD may be considered as retained in transit from the RPE to BlinD and/or drusen.139,141,142 Morphologically heterogeneous BlamD also contains vitronectin, MMP-7, TIMP-3, C3, and C5b-9,162 EC, and UC.142 Evoked in numerous mouse models of aging, stress, and genetic manipulation, BlamD is a reliable marker of RPE stress.168
Subretinal drusenoid debris
Hypotheses of druse formation must eventually also account for subretinal drusenoid debris (SDD). Located adjacent to RPE in the subretinal space, SDD was first described in AMD eyes by the Sarks.155 Ultrastructurally similar to soft drusen, these deposits are enriched in UC, apoE, vitronectin, and complement factor H, and, like drusen, they lack markers for photoreceptors, Müller cells, and RPE apical processes.142,169 Clinically this material is called reticular drusen in a fundus view170 and subretinal drusenoid debris in a cross-sectional view.171 Conferring lower risk for advanced AMD than conventional drusen,172 SDD appear in up to 60% of geographic atrophy eyes,172,173 appearing as focal deposits near the fovea and part of large sheets elsewhere in the macula.174 This coherent morphology suggests a specific formative process, possibly involving microglia resident in that compartment.175,176
Summary
Levels of significance ascribed to molecules sequestered in drusen (Table 20.3, online), and by inference, BlinD, include toxicity to the overlying RPE, stigmata of formative processes (extrusion of cellular materials, secretion, extracellular enzymatic processing, cellular activity), and markers of a diffusely distributed disease process affecting RPE and Bruch’s membrane. Additional significance can be ascribed to these lesions as physical objects that increase path length between choriocapillaries and retina and provide a biomechanically unstable cleavage plane between RPE BL and ICL.
Response-to-retention hypothesis of AMD
The parallels between the pathology of arterial intima of large arteries and that of Bruch’s membrane are striking. Both diseases feature cholesterol-rich lesions in subendothelial compartments within the systemic circulation, involving many of the same molecules and biological processes at multiple steps, as long anticipated.177,178 According to the response-to-retention theory of atherosclerosis, plasma lipoproteins cross the vascular endothelium of large arteries, and bind to extracellular matrix. By itself, this process is not pathological. However, lipoprotein components become modified via oxidative and nonoxidative processes, and launch numerous downstream deleterious events, including inflammation, macrophage recruitment, and neovascularization, leading to disease.179,180 Parallel with apoB lipoprotein-instigated disease in arterial intima, an intraocular response to retention involving the RPE and Bruch’s membrane in aging and AMD would begin with age-related accumulation of lipoproteins of local origin. Oxidation, perhaps driven by reactive oxygen species from adjacent RPE mitochondria, would then initiate a pathological process resembling that in the vascular system with inflammation-driven downstream events including complement activation and structurally unstable lesions.36
Neovascular AMD
CNV, the major sight-threatening complication of AMD, involves angiogenesis along vertical and horizontal vectors: vertically across Bruch’s membrane, and laterally external to the RPE (type 1 CNV181), laterally within the subretinal space (type 2 CNV), or further anteriorly into the retina (type 3 CNV).181–183 Of 40+ conditions involving CNV, AMD is the most prevalent, followed by ocular histoplasmosis,181 and including angioid streaks (see below). CNV is a multifactorial nonspecific wound-healing response to various specific stimuli, involving VEGF stimulation of choriocapillaris endothelium, compromise to Bruch’s membrane, and participation of macrophages.181 Impaired transport across Bruch’s membrane in AMD increasingly isolates the RPE from its metabolic source in the choriocapillaries and enhances the challenge in waste product disposal. VEGF released by RPE as a stress signal initiates an angiogenic response by the endothelium. However, Bruch’s membrane compromise is essential for CNV to proceed, as evidenced by intrachoroidal neovascularization without CNV in a mouse overexpressing VEGF in the setting of an intact Bruch’s membrane.184
Bruch’s membrane in a state of compromise can be breached easily by new vessels in AMD. It is notable that the EL is thinner and more interrupted in eyes with neovascular AMD.23 The length of gaps in the EL is greater in eyes with early AMD and any CNV.23 In paired donor eyes with and without CNV secondary to AMD, progressed eyes are distinguished by calcification and breaks in Bruch’s membrane.185 In contrast, calcification in a small number of geographic atrophy eyes is unremarkable.186
BlinD furthers this process by presenting a horizontal cleavage plane for vessel formation to exploit. The lipid-rich composition, relative lack of structural elements like collagen fibrils, lesion biomechanical instability,122 and proinflammatory, proangiogenic compounds like 7-ketocholesterol and linoleate hydroperoxide160,181,187 likely promote vessel growth in this plane.160
Angioid streaks (ABCC6, MTP genes)
Angioid streaks are ruptures in Bruch’s membrane associated with multiple disorders, caused by excess calcification of the elastic layer188 and often accompanied by CNV. They are prominent ocular manifestation of pseudoxanthoma elasticum (PXE), a systemic connective tissue disorder. PXE patients harbor mutations of a hepatically expressed lipid transporter ABCC6.189 Clinical presentation includes, in addition to streaks and CNV, peau d’orange (flat, yellow, drusen-like lesions), optic nerve head drusen, outer retinal tubulations, subretinal fluid, and pigmentary changes.190 PXE clinical manifestations are believed to be related to ectopic mineralization of nonhepatic tissues, suggesting a defect in the transport of antimineralization agents.191
Angioid streaks are associated with abetalipoproteinemia,192–195 an extremely rare disorder with low plasma apoB-containing lipoproteins, acanthocytosis of erythrocytes, neuropathy, and pigmentary retinopathy. It is historically attributed to lack of lipophilic vitamins delivered by plasma LDL.44 The RPE is now known to express the abetalipoproteinemia gene (MTP),46 which cotranslationally lipidates apoB (see above). How MTP deficiency leads to angioid streaks is unknown. The finding, however, highlights that lack of apoB lipoproteins has negative consequences for Bruch’s membrane health, just as an excess of retained apoB lipoproteins has negative consequences via lesion formation and impaired transport (see above). Good chorioretinal function thus requires an optimal balance between these extremes.
Thick basal laminar deposits (TIMP-3, CTRP5, EFEMP1 genes)
Three autosomal dominant inherited disorders with adult onset – Sorsby fundus dystrophy, late-onset retinal degeneration (LORD) and malattia leventinese-Doyne honeycomb retinal dystrophy (ML-DH) – share phenotypic similarities with AMD and provide mechanistic support for many aspects of Bruch’s membrane physiology and pathophysiology, discussed above. All three conditions result from mutations in genes encoding extracellular matrix proteins or their regulators (Sorsby, TIMP3196; LORD – CTRP5197; and ML-DH, EFEMP1198). All three can progress to CNV to varying degrees (Sorsby > LORD > ML-DH). All three have visual dysfunction, especially rods, attributed to a nutritional night blindness that is responsive to short-term administration of high-dose vitamin A in Sorsby and LORD.199–201 Sorsby and LORD are notable for thick BlamD and areas of RPE atrophy202 and may involve macula and periphery, while ML-DH is notable for radially distributed drusen and peripapillary deposits. In Sorsby eyes mutant TIMP-3 localizes to BlamD. In ML-DH, EFEMP1 localizes to BlamD and not to the pathognomonic drusen themselves, suggesting an important role of BlamD in druse formation.
BlamD in Sorsby and LORD, like that in AMD, is notably rich in oil red O-binding lipid.203–205 The significance of these findings was unclear until a model of a Bruch’s membrane lipoprotein was articulated. In LORD eyes,205 deposits contain EC, UC, and apoB, and lipid-preserving ultrastructural methods revealed solid electron-dense particles tracking in intersecting networks across the BlamD. In hindsight, these may represent native lipoproteins in transit from RPE to the choriocapillaris rather than depositions/aggregations of plasma LDL, as originally speculated. Lipid particle disposition within these thick deposits has been replicated in a mouse model expressing the R345W EFEMP1 mutation.168
1 . The retinal pigment epithelium: function and disease. Marshall J. Hussain AA. Starita C. et al. New York: Oxford University Press; 1998:669–692.
2 Harris A, Moss A, Erhlich R. The choroid. Tasman W, Jaeger EA. Duane’s ophthalmology, 18th ed, Philadelphia, PA: Lippincott Williams & Wilkins, 2011.
3 Wedl C. Grundzüge der pathologischen Histologie. Vienna: Carl Gerold & Sohn; 1854.
4 Bruch CLW. Untersuchungen zur Kenntniss des Kornigen Pigments der Wirbelthiere. Zurich: University of Zurich; 1844.
5 Smirnow AE. Zum Baue der Chorioidea propia des erwachsenen Menschen (Stratum elasticum supracapillare). Graefes Arch. 1899;57:451.
6 Salzmann M. The anatomy and histology of the human eye in the normal state. Its development and senescence. Chicago: University of Chicago; 1912.
7 Wolfensberger TJ. The historical discovery of the retinal pigment epithelium. In: Marmor MF, Wolfensberger TJ. The retinal pigment epithelium: function and disease. New York: Oxford University Press; 1998:13–22.
8 Duke-Elder S, Cook C. Part 1, Embryology. St Louis: Mosby; 1967.
9 Mann I. The development of the human eye. New York: Grune and Stratton; 1964.
10 Scales D, Fryczkowski A, Opremcak E. The choroid. In: Jakoviec F, ed. Principle and practices of ophthalmology. Philadelphia, PA: WB Saunders; 1994:252–261.
11 Takei Y, Ozanics V. Origin and development of Bruch’s membrane in monkey fetuses: an electron microscopic study. Invest Ophthalmol. 1975;14:903–916.
12 Rizzolo LJ, Chen X, Weitzman M, et al. Analysis of the RPE transcriptome reveals dynamic changes during the development of the outer blood–retinal barrier. Mol Vis. 2007;13:1259–1273.
13 Saint-Geniez M, D’Amore PA. Development and pathology of the hyaloid, choroidal and retinal vasculature. Int J Dev Biol. 2004;48:1045–1058.
14 Hogan MJ, Alvarado JA, Weddell JE. Histology of the human eye. An atlas and textbook. Philadelphia, PA: WB Saunders; 1971.
15 Gass JDM. Stereoscopic atlas of macular diseases: diagnosis and treatment, 4th ed. St Louis: Mosby; 1997.
16 Guymer R, Bird A. Age changes in Bruch’s membrane and related structures. In: Ryan SJ, ed. Retina. St Louis: Mosby; 2006:1030–1039.
17 Booij JC, Baas DC, Beisekeeva J, et al. The dynamic nature of Bruch’s membrane. Prog Retin Eye Res. 2009;9:1–18.
18 Chen L, Miyamura N, Ninomiya Y, et al. Distribution of the collagen IV isoforms in human Bruch’s membrane. Br J Ophthalmol. 2003;87:212–215.
19 Aisenbrey S, Zhang M, Bacher D, et al. Retinal pigment epithelial cells synthesize laminins, including laminin 5, and adhere to them through alpha3- and alpha6-containing integrins. Invest Ophthalmol Vis Sci. 2006;47:5537–5544.
20 Hewitt TA, Nakasawa K, Newsome DA. Analysis of newly synthesized Bruch’s membrane proteoglycans. Invest Ophthalmol Vis Sci. 1989;30:478–486.
21 Call TW, Hollyfield JG. Sulfated proteoglycans in Bruch’s membrane of the human eye: localization and characterization using cupromeronic blue. Exp Eye Res. 1990;51:451–462.
22 Korte GE, D’Aversa G. The elastic tissue of Bruch’s membrane. Arch Ophthalmol. 1989;107:1654–1658.
23 Chong NH, Keonin J, Luthert PJ, et al. Decreased thickness and integrity of the macular elastic layer of Bruch’s membrane correspond to the distribution of lesions associated with age-related macular degeneration. Am J Pathol. 2005;166:241–251.
24 Yu HG, Liu X, Kiss S, et al. Increased choroidal neovascularization following laser induction in mice lacking lysyl oxidase-like 1. Invest Ophthalmol Vis Sci. 2008;49:2599–2605.
25 Newsome DA, Huh W, Green WR. Bruch’s membrane age-related changes vary by region. Curr Eye Res. 1987;6:1211–1221.
26 Lutty GA, Hasegawa T, Baba T, et al. Development of the human choriocapillaris. Eye. 2010;24:408–415.
27 Roberts JM, Forrester JV. Factors affecting the migration and growth of endothelial cells from microvessels of bovine retina. Exp Eye Res. 1990;50:165–172.
28 Smith W, Assink J, Klein R, et al. Risk factors for age-related macular degeneration. Pooled findings from three continents. Ophthalmology. 2001;108:697–704.
29 Sarks SH. Ageing and degeneration in the macular region: a clinico-pathological study. Br J Ophthalmol. 1976;60:324–341.
30 Johnson M, Huang J-D, Presley JB, et al. Comparison of morphology of human macular and peripheral Bruch’s membrane in older eyes. Curr Eye Res. 2007;32:791–799.
31 Bird AC, Marshall J. Retinal pigment epithelial detachments in the elderly. Trans Ophthalmol Soc UK. 1986;105:674–682.
32 Pauleikhoff D, Harper CA, Marshall J, et al. Aging changes in Bruch’s membrane: a histochemical and morphological study. Ophthalmology. 1990;97:171–178.
33 Pauleikhoff D, Wojteki S, Müller D, et al. [Adhesive properties of basal membranes of Bruch’s membrane. Immunohistochemical studies of age-dependent changes in adhesive molecules and lipid deposits.]. Ophthalmologe. 2000;97:243–250.
34 Haimovici R, Gantz DL, Rumelt S, et al. The lipid composition of drusen, Bruch’s membrane, and sclera by hot stage polarizing microscopy. Invest Ophthalmol Vis Sci. 2001;42:1592–1599.
35 Curcio CA, Millican CL, Bailey T, et al. Accumulation of cholesterol with age in human Bruch’s membrane. Invest Ophthalmol Vis Sci. 2001;42:265–274.
36 Curcio CA, Johnson M, Huang J-D, et al. Aging, age-related macular degeneration, and the response-to-retention of apolipoprotein B-containing lipoproteins. Prog Ret Eye Res. 2009;28:393–422.
37 Verhoeff FH, Sisson RJ. Basophilic staining of Bruch’s membrane. Arch Ophthalmol. 1926;55:125–127.
38 Ruberti JW, Curcio CA, Millican CL, et al. Quick-freeze/deep-etch visualization of age-related lipid accumulation in Bruch’s membrane. Invest Ophthalmol Vis Sci. 2003;44:1753–1759.
39 Huang J-D, Presley JB, Chimento MF, et al. Age-related changes in human macular Bruch’s membrane as seen by quick-freeze/deep-etch. Exp Eye Res. 2007;85:202–218.
40 Huang J-D, Curcio CA, Johnson M. Morphometric analysis of lipoprotein-like particle accumulation in aging human macular Bruch’s membrane. Invest Ophthalmol Vis Sci. 2008;49:2721–2727.
41 Smith E. The influence of age and atherosclerosis on the chemistry of aortic intima. I. The lipids. J Atheroscler Res. 1965;5:224–240.
42 Holz FG, Sheraidah G, Pauleikhoff D, et al. Analysis of lipid deposits extracted from human macular and peripheral Bruch’s membrane. Arch Ophthalmol. 1994;112:402–406.
43 Shoulders CC, Shelness GS. Current biology of MTP: implications for selective inhibition. Curr Top Med Chem. 2005;5:283–300.
44 Chowers I, Banin E, Merin S, et al. Long-term assessment of combined vitamin A and E treatment for the prevention of retinal degeneration in abetalipoproteinaemia and hypobetalipoproteinaemia patients. Eye. 2001;15:525–530.
45 Shelness GS, Ledford AS. Evolution and mechanism of apolipoprotein B-containing lipoprotein assembly. Curr Opin Lipidol. 2005;16:325–332.
46 Li C-M, Presley JB, Zhang X, et al. Retina expresses microsomal triglyceride transfer protein: implications for age-related maculopathy. J Lipid Res. 2005;46:628–640.
47 Wu T, Fujihara M, Tian J, et al. Apolipoprotein B100 secretion by cultured ARPE-19 cells is modulated by alteration of cholesterol levels. J Neurochem. 2010;114:1734–1744.
48 Contois JH, McConnell JP, Sethi AA, et al. Apolipoprotein B and cardiovascular disease risk: position statement from the AACC Lipoproteins and Vascular Diseases Division Working Group on Best Practices. Clin Chem. 2009;55:407–419.
49 Dashti N, McGwin G, Jr., Owsley C, et al. Plasma apolipoproteins and risk for age-related maculopathy. Br J Ophthalmol. 2006;90:1028–1033. (Erratum Br J Ophthalmol. 2007;91:403.)
50 Wang L, Li C-M, Rudolf M, et al. Lipoprotein particles of intra-ocular origin in human Bruch membrane: an unusual lipid profile. Invest Ophthalmol Vis Sci. 2009;50:870–877.
51 Bretillon L, Thuret G, Gregoire S, et al. Lipid and fatty acid profile of the retina, retinal pigment epithelium/choroid, and the lacrimal gland, and associations with adipose tissue fatty acids in human subjects. Exp Eye Res. 2008;87:521–528.
52 Curcio CA, Johnson M, Rudolf M, et al. The oil spill in aging Bruch’s membrane. Br J Ophthalmol. 2011;95:1638–1645.
53 Hogan MJ. Role of the retinal pigment epithelium in macular disease. Trans Am Acad Ophthalmol Otolaryngol. 1972;76:64–80.
54 Grindle CFJ, Marshall J. Ageing changes in Bruch’s membrane and their functional implications. Trans Ophthalmol Soc U K. 1978;98:172–175.
55 Loane E, Nolan JM, O’Donovan O, et al. Transport and retinal capture of lutein and zeaxanthin with reference to age-related macular degeneration. Surv Ophthalmol. 2008;53:68–81.
56 Tserentsoodol N, Sztein J, Campos M, et al. Uptake of cholesterol by the retina occurs primarily via a low density lipoprotein receptor-mediated process. Mol Vis. 2006;12:1306–1318.
57 Tserentsoodol N, Gordiyenko NV, Pascual I, et al. Intraretinal lipid transport is dependent on high density lipoprotein-like particles and class B scavenger receptors. Mol Vis. 2006;12:1319–1333.
58 Provost A, Vede L, Bigot K, et al. Morphological and electroretinographic phenotype of SRBI knock-out mice after a long term atherogenic diet. Invest Ophthalmol Vis Sci. 2009;50:3931–3942.
59 Ramrattan RS, van der Schaft TL, Mooy CM, et al. Morphometric analysis of Bruch’s membrane, the choriocapillaris, and the choroid in aging. Invest Ophthalmol Vis Sci. 1994;35:2857–2864.
60 Okubo A, Rosa RH, Jr., Bunce CV, et al. The relationships of age changes in retinal pigment epithelium and Bruch’s membrane. Invest Ophthalmol Vis Sci. 1999;40:443–449.
61 Killingsworth MC. Age-related components of Bruch’s membrane. Graefes Arch Clin Exp Ophthalmol. 1987;225:406–412.
62 Karwatowski WSS, Jeffried TE, Duance VC, et al. Preparation of Bruch’s membrane and analysis of the age-related changes in the structural collagens. Br J Ophthalmol. 1995;79:944–952.
63 Macgregor AM, Eberhart CG, Fraig M, et al. Tissue inhibitor of matrix metalloproteinase-3 levels in the extracellular matrix of lung, kidney, and eye increase with age. J Histochem Cytochem. 2009;57:207–213.
64 Qi JH, Ebrahem Q, Moore N, et al. A novel function for tissue inhibitor of metalloproteinases-3 (TIMP3): inhibition of angiogenesis by blockage of VEGF binding to VEGF receptor-2. Nat Med. 2003;9:407–415.
65 Ebrahem Q, Qi JH, Sugimoto M, et al. Increased neovascularization in mice lacking tissue inhibitor of metalloproteinases-3. Invest Ophthalmol Vis Sci. 2011;52:6117–6123.
66 Beattie JR, Pawlak AM, Boulton ME, et al. Multiplex analysis of age-related protein and lipid modifications in human Bruch’s membrane. FASEB J. 2010;24:4816–4824.
67 van der Schaft TL, Mooy CM, de Bruijn WC, et al. Histologic features of the early stages of age-related macular degeneration. Ophthalmol. 1992;99:278–286.
68 Davis WL, Jones RG, Hagler HK. An electron microscopic histochemical and analytical X-ray microprobe study of calcification in Bruch’s membrane from human eyes. J Histochem Cytochem. 1981;29:601–608.
69 Colville D, Savige J. Alport syndrome. Review of the ocular manifestations. Ophthalm Genet. 1997;18:161–173.
70 Kim RY, Faktorovich EG, Kuo CY, et al. Retinal function abnormalities in membranoproliferative glomerulonephritis type II. Am J Ophthalmol. 1997;123:619–628.
71 Weiner DE, Tighiouart H, Reynolds R, et al. Kidney function, albuminuria and age-related macular degeneration in NHANES III. Nephrol Dialysis Transplant. 2011;26:3159–3165.
72 Maddox DA, Brenner BM. Glomerular filtration of fluid and macromolecules: the renal response to injury. Annu Rev Med. 1977;28:91–102.
73 Beers APA, Van Der Heijde GL. In vivo determination of the biomechanical properties of the component elements of the accommodation mechanism. Vision Res. 1994;34:2897–2905.
74 Charman WN. The eye in focus: accommodation and presbyopia. Clin Exp Optom. 2008;91:207–225.
75 Chan WH, Hussain AA, Marshall J. Young’s modulus of Bruch’s membrane: implications for AMD. Invest Ophthalmol Vis Sci. 2007;48:2187.
76 Ugarte M, Hussain AA, Marshall J. An experimental study of the elastic properties of the human Bruch’s membrane–choroid complex: relevance to ageing. Br J Ophthalmol. 2006;90:621–626.
77 Tawara A, Varner HH, Hollyfield JG. Proteoglycans in the mouse interphotoreceptor matrix. I. Histochemical studies using cuprolinic blue. Exp Eye Res. 1988;46:689–704.
78 Hollyfield JG. Hyaluronan and the functional organization of the interphotoreceptor matrix. Invest Ophthalmol Vis Sci. 1999;40:2767–2769.
79 Klintworth GK. The cornea – structure and macromolecules in health and disease. A review. Am J Pathol. 1977;89:718–808.
80 Maurice DM. The structure and transparency of the cornea. J Physiol. 1957;136:263–286.
81 Hassell JR, Birk DE. The molecular basis of corneal transparency. Exp Eye Res. 2010;91:326–335.
82 Dohlman CH, Hedbys BO, Mishima S. The swelling pressure of the corneal stroma. Invest Ophthalmol. 1962;1:158–162.
83 Wiig H. Cornea fluid dynamics. I: measurement of hydrostatic and colloid osmotic pressure in rabbits. Exp Eye Res. 1989;49:1015–1030.
84 Moore DJ, Hussain AA, Marshall J. Age-related variation in the hydraulic conductivity of Bruch’s membrane. Invest Ophthalmol Vis Sci. 1995;36:1290–1297.
85 Levick JR, Smaje LH. An analysis of the permeability of a fenestra. Microvasc Res. 1987;33:233–256.
86 Navar PD, Navar LG. Relationship between colloid osmotic pressure and plasma protein concentration in the dog. Am J Physiol. 1977;233:H295–H298.
87 Maepea O. Pressures in the anterior ciliary arteries, choroidal veins and choriocapillaris. Exp Eye Res. 1992;54:731–736.
88 Emi K, Pederson JE, Toris CB. Hydrostatic pressure of the suprachoroidal space. Invest Ophthalmol Vis Sci. 1989;30:233.
89 Starita C, Hussain AA, Patmore A, et al. Localization of the site of major resistance to fluid transport in Bruch’s membrane. Invest Ophthalmol Vis Sci. 1997;38:762–767.
90 Overby D, Johnson M. Studies of depth-of-field effects in microscopy supported by numerical simulations. J Microscopy. 2005;220:176–189.
91 Fisher RF. The influence of age on some ocular basement membranes. Eye. 1987;1:184–189.
92 Daniels BS, Hauser EB, Deen WN, et al. Glomerular basement membrane: in vitro studies of water and protein permeability. Am J Physiol. 1992;262:F919–F926.
93 Renkin EM. Multiple pathways of capillary permeability. Circ Res. 1977;41:735–743.
94 Hussain AA, Starita C, Marshall J. Transport characteristics of ageing human Bruch’s membrane: implications for age-related macular degeneration (AMD). In: Ioseliani OR, ed. Focus on macular degeneration research. Hauppauge, New York: Nova Biomedical Books, 2004.
95 Starita C, Hussain AA, Pagliarini S, et al. Hydrodynamics of ageing Bruch’s membrane: implications for macular disease. Exp Eye Res. 1996;62:565–572.
96 McCarty WJ, Chimento MF, Curcio CA, et al. Effects of particulates and lipids on the hydraulic conductivity of Matrigel. J Appl Physiol. 2008;105:621–628.
97 Ethier CR, Johnson M, Ruberti J. Ocular biomechanics and biotransport. Annu Rev Biomed Eng. 2004;6:249–273.
98 Holz FG, Piguet B, Minassian DC, et al. Decreasing stromal iris pigmentation as a risk factor for age-related macular degeneration. Am J Ophthalmol. 1994;117:19–23.
99 Truskey GA, Yuan F, Katz DF. Transport phenomena in biological systems. Upper Saddle River, NJ: Pearson Prentice Hall; 2004.
100 Fisher WR, Granade ME, Mauldin JL. Hydrodynamic studies of human low density lipoproteins. Evaluation of the diffusion coefficient and the preferential hydration. Biochemistry. 1971;10:1622–1629.
101 Hussain AA, Rowe L, Marshall J. Age-related alterations in the diffusional transport of amino acids across the human Bruch’s–choroid complex. J Opt Soc Am A. 2002;19:166–172.
102 Moore DJ, Clover GM. The effect of age on the macromolecular permeability of human Bruch’s membrane. Invest Ophthalmol Vis Sci. 2001;42:2970–2975.
103 Cheruvu NP, Kompella UB. Bovine and porcine transscleral solute transport: influence of lipophilicity and the choroid-Bruch’s layer. Invest Ophthalmol Vis Sci. 2006;47:4513–4522.
104 Cankova Z, Huang J-D, Kruth H, et al. 2011. Passage of low-density lipoproteins through Bruch’s membrane and choroid. Exp Eye Res. 2011;93:947–955.
105 Hussain AA, Starita C, Hodgetts A, et al. Macromolecular diffusion characteristics of ageing human Bruch’s membrane: implications for age-related macular degeneration (AMD). Exp Eye Res. 2010;90:703–710.
106 Yang N, Vafai K. Modeling of low-density lipoprotein (LDL) transport in the artery – effects of hypertension. Int J Heat Mass Transfer. 2006;49:850–867.
107 Elner VM. Retinal pigment epithelial acid lipase activity and lipoprotein receptors: effects of dietary omega-3 fatty acids. Trans Am Ophthalmol Soc. 2002;100:301–338.
108 Gordiyenko N, Campos M, Lee JW, et al. RPE cells internalize low-density lipoprotein (LDL) and oxidized LDL (oxLDL) in large quantities in vitro and in vivo. Invest Ophthalmol Vis Sci. 2004;45:2822–2829.
109 Martidis A, Duker JS, Greenberg PB, et al. Intravitreal triamcinolone for refractory diabetic macular edema. Ophthalmology. 2002;109:920–927.
110 Anderson OA, Bainbridge JW, Shima DT. Delivery of anti-angiogenic molecular therapies for retinal disease. Drug Discov Today. 2010;15:272–282.
111 Pitkanen L, Ranta VP, Moilanen H, et al. Permeability of retinal pigment epithelium: effects of permeant molecular weight and lipophilicity. Invest Ophthalmol Vis Sci. 2005;46:641–646.
112 Jackson GR, Curcio CA, Sloan KR, et al. Photoreceptor degeneration in aging and age-related maculopathy. In: Penfold PL, Provis JM. Macular degeneration. Berlin: Springer-Verlag; 2005:45–62.
113 Jackson GR, Owsley C, McGwin G. Aging and dark adaptation. Vision Res. 1999;39:3975–3982.
114 Owsley C, McGwin G, Jr., Jackson GR, et al. Cone- and rod-mediated dark adaptation impairment in age-related maculopathy. Ophthalmology. 2007;114:1728–1735.
115 Steinmetz RL, Haimovici R, Jubb C, et al. Symptomatic abnormalities of dark adaptation in patients with age-related Bruch’s membrane change. Br J Ophthalmol. 1993;77:549–554.
116 Owsley C, McGwin G, Jr., Jackson GR, et al. Effect of short-term, high-dose retinol on dark adaptation in aging and early age-related maculopathy. Invest Ophthalmol Vis Sci. 2006;47:1310–1318.
117 Green WR, Enger C. Age-related macular degeneration histopathologic studies: the 1992 Lorenz E. Zimmerman Lecture. Ophthalmology. 1993;100:1519–1535.
118 Khanifar AA, Koreishi AF, Izatt JA, et al. Drusen ultrastructure imaging with spectral domain optical coherence tomography in age-related macular degeneration. Ophthalmology. 2008;115:1883–1890.
119 Klein R, Klein BEK, Linton KLP. Prevalence of age-related maculopathy. Ophthalmol. 1992;99:933–943.
120 Friedman E, van Buskirk EM, Fineberg E, et al. Pathogenesis of senile disciform degeneration of the macula. XXI Concilium Ophthalmologicum. Mexico: Elsevier; 1971. p. 454–8
121 Lengyel I, Tufail A, Hosaini HA, et al. Association of drusen deposition with choroidal intercapillary pillars in the aging human eye. Invest Ophthalmol Vis Sci. 2004;45:2886–2892.
122 Rudolf M, Clark ME, Chimento M, et al. Prevalence and morphology of druse types in the macula and periphery of eyes with age-related maculopathy. Invest Ophthalmol Vis Sci. 2008;49:1200–1209.
123 Sarks SH. Council lecture: Drusen and their relationship to senile macular degeneration. Aust J Ophthalmol. 1980;8:117–130.
124 Klein R, Davis MD, Magli YL, et al. The Wisconsin Age-Related Maculopathy Grading System. Ophthalmol. 1991;98:1128–1134.
125 Davis MD, Gangnon RE, Lee LY, et al. The Age-Related Eye Disease Study severity scale for age-related macular degeneration: AREDS report no. 17. Arch Ophthalmol. 2005;123:1484–1498.
126 Klein R, Klein BE, Knudtson MD, et al. Fifteen-year cumulative incidence of age-related macular degeneration: the Beaver Dam Eye Study. Ophthalmology. 2007;114:253–262.
127 Querques G, Guigui B, Leveziel N, et al. Insights into pathology of cuticular drusen from integrated confocal scanning laser ophthalmoscopy imaging and corresponding spectral domain optical coherence tomography. Graefes Arch Clin Exp Ophthalmol. 2011;249:1617–1625.
128 Donders F. Beitrage zur pathologischen Anatomie des Auges. Arch Ophthalmol. 1854;1:106–118.
129 Hageman GS, Mullins RF. Molecular composition of drusen as related to substructural phenotype. Mol Vis. 1999;5:28.
130 Müller H. Anatomische Beiträge zur Ophthalmologie. Graefes Arch Clin Exp Ophthalmol. 1856;2:1–69.
131 Oatman EL. Diagnostics of the fundus oculi. Troy, NY: Southworth; 1913.
132 Holloway TB, Verhoeff FH. Disc-like degeneration of the macula with microscopic report concerning a tumor-like mass in the macular region. Trans Am Ophthalmol Soc. 1928;26:206–228.
133 Lauber H. The origin of hyaline formations within the eye. Ber ü d deutsch ophth Gesellsch. 1924;44:216.
134 Rones B. Formation of drusen of the lamina vitrea. Arch Ophthalmol. 1937;18:288–402.
135 Wolter JR, Falls HF. Bilateral confluent drusen. Arch Ophthalmol. 1962;68:219–226.
136 Luibl V, Isas JM, Kayed R, et al. Drusen deposits associated with aging and age-related macular degeneration contain nonfibrillar amyloid oligomers. J Clin Invest. 2006;116:378–385.
137 Li C-M, Clark M, Rudolf M, et al. Distribution and composition of esterified and unesterified cholesterol in extra-macular drusen. Exp Eye Res. 2007;85:192–201.
138 Lengyel I, Flinn JM, Peto T, et al. High concentration of zinc in sub-retinal pigment epithelial deposits. Exp Eye Res. 2007;84:772–780.
139 Malek G, Li C-M, Guidry C, et al. Apolipoprotein B in cholesterol-containing drusen and basal deposits in eyes with age-related maculopathy. Am J Pathol. 2003;162:413–425.
140 Farkas TG, Sylvester V, Archer D, et al. The histochemistry of drusen. Am J Ophthalmol. 1971;71:1206–1215.
141 Pauleikhoff D, Zuels S, Sheraidah GS, et al. Correlation between biochemical composition and fluorescein binding of deposits in Bruch’s membrane. Ophthalmol. 1992;99:1548–1553.
142 Curcio CA, Presley JB, Medeiros NE, et al. Esterified and unesterified cholesterol in drusen and basal deposits of eyes with age-related maculopathy. Exp Eye Res. 2005;81:731–741.
143 Wang L, Clark ME, Crossman DK, et al. Abundant lipid and protein components of drusen. PLoS ONE. 2010;5:e10329.
144 Guyton JR, Klemp KF. The lipid-rich core region of human atherosclerotic fibrous plaques. Prevalence of small lipid droplets and vesicles by electron microscopy. Am J Pathol. 1989;134:705–717.
145 Friedman DA, Parker JS, Kimble JA, et al. Fluorescein localization in drusen associated with age-related macular degeneration. Retina. 2012;32:19–24.
146 Hageman GS, Mullins RG, Russell SR, et al. Vitronectin is a constituent of ocular drusen and the vitronectin gene is expressed in human retinal pigmented epithelial cells. FASEB J. 1999;13:477–484.
147 Fariss RN, Apte SS, Olsen BR, et al. Tissue inhibitor of metalloproteinases-3 is a component of Bruch’s membrane of the eye. Am J Pathol. 1997;150:323–328.
148 Hageman GS, Anderson DH, Johnson LV, et al. A common haplotype in the complement regulatory gene factor H (HF1/CFH) predisposes individuals to age-related macular degeneration. Proc Natl Acad Sci U S A. 2005;102:7227–7232.
149 Johnson LV, Leitner WP, Rivest AJ, et al. The Alzheimer’s Aβ-peptide is deposited at sites of complement activation in pathologic deposits associated with aging and age-related macular degeneration. Proc Natl Acad Sci U S A. 2002;99:11830–11835.
150 Nakata K, Crabb JW, Hollyfield JG. Crystallin distribution in Bruch’s membrane–choroid complex from AMD and age-matched donor eyes. Exp Eye Res. 2005;80:821–826.
151 Klaver CC, Kliffen M, van Duijn CM, et al. Genetic association of apolipoprotein E with age-related macular degeneration. Am J Hum Genet. 1998;63:200–206.
152 Anderson DH, Ozaki S, Nealon M, et al. Local cellular sources of apolipoprotein E in the human retina and retinal pigmented epithelium: implications for the process of drusen formation. Am J Ophthalmol. 2001;131:767–781.
153 Li C-M, Chung BH, Presley JB, et al. Lipoprotein-like particles and cholesteryl esters in human Bruch’s membrane: initial characterization. Invest Ophthalmol Vis Sci. 2005;46:2576–2586.
154 Li C-M, Clark ME, Chimento MF, et al. Apolipoprotein localization in isolated drusen and retinal apolipoprotein gene expression. Invest Ophthalmol Vis Sci. 2006;47:3119–3128.
155 Sarks JP, Sarks SH, Killingsworth MC. Evolution of geographic atrophy of the retinal pigment epithelium. Eye. 1988;2:552–577.
156 Sarks S, Cherepanoff S, Killingsworth M, et al. Relationship of basal laminar deposit and membranous debris to the clinical presentation of early age-related macular degeneration. Invest Ophthalmol Vis Sci. 2007;48:968–977.
157 Curcio CA, Presley JB, Millican CL, et al. Basal deposits and drusen in eyes with age-related maculopathy: evidence for solid lipid particles. Exp Eye Res. 2005;80:761–775.
158 Curcio CA, Millican CL. Basal linear deposit and large drusen are specific for early age-related maculopathy. Arch Ophthalmol. 1999;117:329–339.
159 Sarks SH, van Driel D, Maxwell L, et al. Softening of drusen and subretinal neovascularization. Trans Ophthalmol Soc U K. 1980;100:414–422.
160 Sarks JP, Sarks SH, Killingsworth MC. Morphology of early choroidal neovascularization in age-related macular degeneration: correlation with activity. Eye. 1997;11:515–522.
161 Messinger JD, Johnson M, Medeiros NE, et al. Transition from lipid wall to basal linear deposit in age-related maculopathy (ARM). Invest Ophthalmol Vis Sci. 2009;50:4933.
162 Lommatzsch A, Hermans P, Müller KD, et al. Are low inflammatory reactions involved in exudative age-related macular degeneration? Morphological and immunhistochemical analysis of AMD associated with basal deposits. Graefes Arch Clin Exp Ophthalmol. 2008;246:803–810.
163 Yamada Y, Ishibashi K, Ishibashi K, et al. The expression of advanced glycation endproduct receptors in RPE cells associated with basal deposits in human maculas. Exp Eye Res. 2006;82:840–848.
164 Löffler KU, Lee WR. Basal linear deposit in the human macula. Graefes Arch Clin Exp Ophthalmol. 1986;224:493–501.
165 Marshall GE, Konstas AGP, Reid GG, et al. Type IV collagen and laminin in Bruch’s membrane and basal linear deposit in the human macula. Br J Ophthalmol. 1992;76:607–614.
166 Knupp C, Munro PM, Luther PK, et al. Structure of abnormal molecular assemblies (collagen VI) associated with human full thickness macular holes. J Struct Biol. 2000;129:38–47.
167 Reale E, Groos S, Eckardt U, et al. New components of “basal laminar deposits” in age-related macular degeneration. Cells, Tissues, Organs. 2008;190:170–181.
168 Marmorstein LY, McLaughlin PJ, Peachey NS, et al. Formation and progression of sub-retinal pigment epithelium deposits in Efemp1 mutation knock-in mice: a model for the early pathogenic course of macular degeneration. Hum Mol Genet. 2007;16:2423–2432.
169 Rudolf M, Malek G, Messinger JD, et al. Sub-retinal drusenoid deposits in human retina: organization and composition. Exp Eye Res. 2008;87:402–408.
170 Smith RT, Sohrab MA, Busuioc M, et al. Reticular macular disease. Am J Ophthalmol. 2009;148:733–743. e2
171 Zweifel SA, Spaide RF, Curcio CA, et al. Reticular pseudodrusen are subretinal drusenoid deposits. Ophthalmology. 2010;117:303–312.
172 Zweifel SA, Imamura Y, Spaide TC, et al. Prevalence and significance of subretinal drusenoid deposits (reticular pseudodrusen) in age-related macular degeneration. Ophthalmology. 2010;117:1775–1781.
173 Schmitz-Valckenberg S, Alten F, Steinberg JS, et al. Reticular drusen associated with geographic atrophy in age-related macular degeneration. Invest Ophthalmol Vis Sci. 2011;52:5009–5015.
174 Spaide RF, Curcio CA. Drusen characterization with multimodal imaging. Retina. 2010;30:1441–1454.
175 Xu H, Chen M, Manivannan A, et al. Age-dependent accumulation of lipofuscin in perivascular and subretinal microglia in experimental mice. Aging Cell. 2008;7:58–68.
176 Ma W, Zhao L, Fontainhas AM, et al. Microglia in the mouse retina alter the structure and function of retinal pigmented epithelial cells: a potential cellular interaction relevant to AMD. PLoS ONE. 2009;4:e7945.
177 Verhoeff FH, Grossman HP. The pathogenesis of disciform degeneration of the macula. Trans Am Ophthalmol Soc. 1937;35:262–294.
178 Friedman E. The role of the atherosclerotic process in the pathogenesis of age-related macular degeneration. Am J Ophthalmol. 2000;130:658–663.
179 Williams KJ, Tabas I. The response-to-retention hypothesis of early atherogenesis. Arterioscler Thromb Vasc Biol. 1995;15:551–561.
180 Tabas I, Williams KJ, Boren J. Subendothelial lipoprotein retention as the initiating process in atherosclerosis: update and therapeutic implications. Circulation. 2007;116:1832–1844.
181 Grossniklaus HE, Green WR. Choroidal neovascularization. Am J Ophthalmol. 2004;137:496–503.
182 Yannuzzi LA, Freund KB, Takahashi BS. Review of retinal angiomatous proliferation or type 3 neovascularization. Retina. 2008;28:375–384.
183 Klein ML, Wilson DJ. Clinicopathologic correlation of choroidal and retinal neovascular lesions in age-related macular degeneration. Am J Ophthalmol. 2011;151:161–169.
184 Schwesinger C, Yee C, Rohan RM, et al. Intrachoroidal neovascularization in transgenic mice overexpressing vascular endothelial growth factor in the retinal pigment epithelium. Am J Pathol. 2001;158:1161–1172.
185 Spraul CW, Grossniklaus HE. Characteristics of drusen and Bruch’s membrane in postmortem eyes with age-related macular degeneration. Arch Ophthalmol. 1997;115:267–273.
186 Vogt SD, Curcio CA, Wang L, et al. Retinal pigment epithelial expression of complement regulator CD46 is altered early in the course of geographic atrophy. Exp Eye Res. 2011;93:413–423.
187 Spaide RF, Armstrong D, Browne R. Continuing medical education review: choroidal neovascularization in age-related macular degeneration – what is the cause? Retina. 2003;23:595–614.
188 Jampol LM, Acheson R, Eagle RC, et al. Calcification of Bruch’s membrane in angioid streaks with homozygous sickle cell disease. Arch Ophthalmol. 1987;105:93–98.
189 Bergen AA, Plomp AS, Schuurman EJ, et al. Mutations in ABCC6 cause pseudoxanthoma elasticum. Nat Genet. 2000;25:228–231.
190 Zweifel SA, Imamura Y, Freund KB, et al. Multimodal fundus imaging of pseudoxanthoma elasticum. Retina. 2011;31:482–491.
191 Uitto J, Bercovitch L, Terry SF, et al. Pseudoxanthoma elasticum: progress in diagnostics and research towards treatment. Summary of the 2010 PXE International Research Meeting. Am J Med Genet Part A. 2011;155:1517–1526.
192 Runge P, Müller DP, McAllister J, et al. Oral vitamin E supplements can prevent the retinopathy of abetalipoproteinaemia. Br J Ophthalmol. 1986;70:166–173.
193 Duker JS, Belmont J, Bosley TM. Angioid streaks associated with abetalipoproteinemia. Case report. Arch Ophthalmol. 1987;105:1173–1174.
194 Dieckert J, White M, Christmann L, et al. Angioid streaks associated with abetalipoproteinemia. Ann Ophthalmol. 1989;21:172–175.
195 Gorin MB, Paul TO, Rader DJ. Angioid streaks associated with abetalipoproteinemia. Ophthalmic Genet. 1994;15:151–159.
196 Weber BHF, Vogt G, Pruett RC, et al. Mutations in the tissue inhibitor of metalloproteinases-3 (TIMP3) in patients with Sorsby’s fundus dystrophy. Nat Genet. 1994;8:352–365.
197 Hayward C, Shu X, Cideciyan AV, et al. Mutation in a short-chain collagen gene, CTRP5, results in extracellular deposit formation in late-onset retinal degeneration: a genetic model for age-related macular degeneration. Hum Mol Genet. 2003;12:2657–2667.
198 Stone E, Lotery A, Munier F, et al. A single EFEMP1 mutation associated with both malattia leventinese and Doyne honeycomb retinal dystrophy. Nat Genet. 1999;22:199–202.
199 Jacobson SG, Cideciyan AV, Regunath G, et al. Night blindness in Sorsby’s fundus dystrophy reversed by vitamin A. Nat Genet. 1995;11:27–32.
200 Jacobson SG, Cideciyan AV, Wright E, et al. Phenotypic marker for early disease detection in dominant late-onset retinal degeneration. Invest Ophthalmol Vis Sci. 2001;42:1882–1890.
201 Michaelides M, Jenkins SA, Brantley MA, Jr., et al. Maculopathy due to the R345W substitution in fibulin-3: distinct clinical features, disease variability, and extent of retinal dysfunction. Invest Ophthalmol Vis Sci. 2006;47:3085–3097.
202 Isashiki Y, Tabata Y, Kamimura K, et al. Sorsby’s fundus dystrophy in two Japanese families with unusual clinical features. Jpn J Ophthalmol. 1999;43:472–480.
203 Capon MRC, Marshall J, Krafft JI, et al. Sorsby’s fundus dystrophy. A light and electron microscopic study. Ophthalmology. 1989;96:1769–1777.
204 Kuntz CA, Jacobson SG, Cideciyan AV, et al. Sub-retinal pigment epithelial deposits in a dominant late-onset retinal degeneration. Invest Ophthalmol Vis Sci. 1996;37:1772–1782.
205 Milam AH, Curcio CA, Cideciyan AV, et al. Dominant late-onset retinal degeneration with regional variation of sub-RPE deposits, retinal function, and photoreceptor degeneration. Ophthalmology. 2000;107:2256–2266.
206 Curcio CA, Mayne R, Ninomiya H. Localization of type IV collagen chains in AMD eyes. 1999. Unpublished observations.
207 Knupp C, Chong NH, Munro PM, et al. Analysis of the collagen VI assemblies associated with Sorsby’s fundus dystrophy. J Struct Biol. 2002;137:31–40.
208 Bhutto IA, Kim SY, McLeod DS, et al. Localization of collagen XVIII and the endostatin portion of collagen XVIII in aged human control eyes and eyes with age-related macular degeneration. Invest Ophthalmol Vis Sci. 2004;45:1544–1552.
209 Marmorstein LY, Munier FL, Arsenijevic Y, et al. Aberrant accumulation of EFEMP1 underlies drusen formation in malattia leventinese and age-related macular degeneration. Proc Natl Acad Sci U S A. 2002;99:13067–13072.
210 Das A, Frank RN, Zhang NL, et al. Ultrastructural localization of extracellular matrix components in human retinal vessels and Bruch’s membrane. Arch Ophthalmol. 1990;108:421–429.
211 Lin WL, Essner E, McCarthy KJ, et al. Ultrastructural immunocytochemical localization of chondroitin sulfate proteoglycan in Bruch’s membrane of the rat. Invest Ophthalmol Vis Sci. 1992;33:2072–2075.
212 Curcio C, Millican C, Kruth H. Cholesterol accumulates with age in human Bruch’s membrane. Invest Ophthalmol Vis Sci. 2000;41:S115.
213 Liu A, Chang J, Lin Y, et al. Long-chain and very long-chain polyunsaturated fatty acids in ocular aging and age-related macular degeneration. J Lipid Res. 2011;51:3217–3229.
214 Tezel TH, Geng L, Lato EB, et al. Synthesis and secretion of hemoglobin by retinal pigment epithelium. Invest Ophthalmol Vis Sci. 2009;50:1911–1919.
215 Sakaguchi H, Miyagi M, Shadrach KG, et al. Clusterin is present in drusen in age-related macular degeneration. Exp Eye Res. 2002;74:547–549.
216 Chong NH, Keonin J, Luthert PJ, et al. Decreased thickness and integrity of the macular elastic layer of Bruch’s membrane correspond to the distribution of lesions associated with age-related macular degeneration. Am J Pathol. 2005;166:241–251.
217 Mullins RF, Olvera MA, Clark AF, et al. Fibulin-5 distribution in human eyes: relevance to age-related macular degeneration. Exp Eye Res. 2007;84:378–380.
218 Yamada Y, Tian J, Yang Y, et al. Oxidized low density lipoproteins induce a pathologic response by retinal pigmented epithelial cells. J Neurochem. 2008;105:1187–1197.
219 Kamei M, Hollyfield JG. TIMP-3 in Bruch’s membrane: changes during aging and in age-related macular degeneration. Invest Ophthalmol Vis Sci. 1999;40:2367–2375.
220 Xu H, Chen M, Forrester JV. Para-inflammation in the aging retina. Prog Retin Eye Res. 2009;28:348–368.
221 Glenn JV, Mahaffy H, Wu K, et al. Advanced glycation end product (AGE) accumulation on Bruch’s membrane: links to age-related RPE dysfunction. Invest Ophthalmol Vis Sci. 2009;50:441–451.
222 Guo L, Hussain AA, Limb GA, et al. Age-dependent variation in metalloproteinase activity of isolated human Bruch’s membrane and choroid. Invest Ophthalmol Vis Sci. 1999;40:2676–2682.
223 Bhutto IA, Uno K, Merges C, et al. Reduction of endogenous angiogenesis inhibitors in Bruch’s membrane of the submacular region in eyes with age-related macular degeneration. Arch Ophthalmol. 2008;126:670–678.
224 Lin MY, Kochounian H, Moore RE, et al. Deposition of exon-skipping splice isoform of human retinal G protein-coupled receptor from retinal pigment epithelium into Bruch’s membrane. Mol Vis. 2007;13:1203–1214.
225 Handa JT, Verzijl N, Matsunaga H, et al. Increase in the advanced glycation end product pentosidine in Bruch’s membrane with age. Invest Ophthalmol Vis Sci. 1999;40:775–779.
226 Glenn JV, Beattie JR, Barrett L, et al. Confocal Raman microscopy can quantify advanced glycation end product (AGE) modifications in Bruch’s membrane leading to accurate, nondestructive prediction of ocular aging. FASEB J. 2007;21:3542–3552.
227 Johnson PT, Betts KE, Radeke MJ, et al. Individuals homozygous for the age-related macular degeneration risk-conferring variant of complement factor H have elevated levels of CRP in the choroid. Proc Natl Acad Sci U S A. 2006;103:17456–17461.
228 Spaide R, Ho-Spaide W, Browne R, et al. Characterization of peroxidized lipids in Bruch’s membrane. Retina. 1999;19:141–147.
229 Moreira EF, Larrayoz IM, Lee JW, et al. 7-Ketocholesterol is present in lipid deposits in the primate retina: potential implication in the induction of VEGF and CNV formation. Invest Ophthalmol Vis Sci. 2009;50:523–532.
230 Vranka JA, Johnson E, Zhu X, et al. Discrete expression and distribution pattern of TIMP-3 in the human retina and choroid. Curr Eye Res. 1997;16:102–110.
231 Hughes BA, Miller SS, Machen TE. Effects of cyclic AMP on fluid absorption and ion transport across frog retinal pigment epithelium. Measurements in the open-circuit state. J Gen Physiol. 1984;83:875–899.
232 Frambach DA, Weiter JJ, Adler AJ. A photogrammetric method to measure fluid movement across isolated frog retinal pigment epithelium. Biophys J. 1985;47:547–552.
233 Negi A, Marmor MF. Quantitative estimation of metabolic transport of subretinal fluid. Invest Ophthalmol Vis Sci. 1986;27:1564–1568.
234 Marmor MF, Negi A. Pharmacologic modification of subretinal fluid absorption in the rabbit eye. Arch Ophthalmol. 1986;104:1674–1677.
235 Tsuboi S. Measurement of the volume flow and hydraulic conductivity across the isolated dog retinal pigment epithelium. Invest Ophthalmol Vis Sci. 1987;28:1776–1782.
236 Cantrill HL, Pederson JE. Experimental retinal detachment: VI. The permeability of the blood–retinal barrier. Arch Ophthalmol. 1984;102:747–751.
237 Pederson JE, Cantrill HL. Experimental retinal detachment: V. Fluid movement through the retinal hole. Arch Ophthalmol. 1984;102:136–139.
238 Chihara E, Nao-i N. Resorption of subretinal fluid by transepithelial flow of the retinal pigment epithelium. Graefes Arch Clin Exp Ophthalmol. 1985;223:202–204.
239 Anderson DH, Talaga KC, Rivest AJ, et al. Characterization of beta amyloid assemblies in drusen: the deposits associated with aging and age-related macular degeneration. Exp Eye Res. 2004;78:243–256.
240 Malek G, Johnson LV, Mace BE, et al. Apolipoprotein E allele-dependent pathogenesis: A model for age-related retinal degeneration. Proc Natl Acad Sci U S A. 2005;102:11900–11905.
241 Hageman GS, Luthert PJ, Chong NHC, et al. An integrated hypothesis that considers drusen as biomarkers of immune-mediated processes at the RPE–Bruch’s membrane interface in aging and age-related macular degeneration. Progr Ret Eye Res. 2001;20:705–732.
242 Dentchev T, Milam AH, Lee VM, et al. Amyloid-beta is found in drusen from some age-related macular degeneration retinas, but not in drusen from normal retinas. Mol Vis. 2003;9:184–190.
243 Anderson DH, Radeke MJ, Gallo NB, et al. The pivotal role of the complement system in aging and age-related macular degeneration: hypothesis re-visited. Prog Retin Eye Res. 2010;29:95–112.
244 Kochounian H, Johnson LV, Fong HK. Accumulation of extracellular RGR-d in Bruch’s membrane and close association with drusen at intercapillary regions. Exp Eye Res. 2009;88:1129–1136.
245 Loeffler KU, Mangini NJ. Immunolocalization of ubiquitin and related enzymes in human retina and retinal pigment epithelium. Graefes Arch Clin Exp Ophthalmol. 1997;235:248–254.
246 Wang AL, Lukas TJ, Yuan M, et al. Autophagy and exosomes in the aged retinal pigment epithelium: possible relevance to drusen formation and age-related macular degeneration. PLoS ONE. 2009;4:e4160.
247 Gouras P, Braun K, Ivert L, et al. Bestrophin detected in the basal membrane of the retinal epithelium and drusen of monkeys with drusenoid maculopathy. Graefes Arch Clin Exp Ophthalmol. 2009;247:1051–1056.