Chapter 78 Spinal Cord Injury
Epidemiology
Acute spinal cord injury occurs worldwide, with an annual incidence of 5–40 cases per million. The greatest frequency of spinal cord injuries is between ages 15 and 25 years, with a male to female ratio of 4:1. Common causes are motor vehicle accidents (50 percent), falls and work-related injuries (30 percent), violent crime (11 percent), and sports-related injuries (9 percent) [Rihn et al., 2009; Rowland et al., 2008]. In the United States and Canada, the annual incidence of traumatic spinal cord injury is 30–46 cases per 1 million population [Decker and Hergenroeder, 2004]. In the United States, 8000–12,000 new spinal cord injuries occur annually. Fewer than 10 percent of these patients die from their acute injuries [Bramlett and Dietrich, 2007; Walker, 1991], with 200,000–250,000 persons now living in the United States with sequelae of spinal cord trauma [Bramlett and Dietrich, 2007; Green and Eismont, 1984; Meyer et al., 1991; Oliver, 1992; Walker, 1991]. Pediatric patients constitute 0.3–13 percent of that number [Apple et al., 1995; Dias, 2004; Hadley et al., 1988; Hill et al., 1984; Proctor, 2002], with 3–5 percent of the spinal cord injuries that occur each year in the United States being found in persons younger than 15 years of age, and 20 percent in persons younger than 20 years of age [Vogel and Anderson, 2003]. The risk of spinal cord injury is equal for males and females from birth to age 3 years; the male to female ratio is 6:4 from age 4–8 years, 7:3 from age 9–15 years, and 8.5:1.5 from age 16–20 years [Massagli, 2000].
Of all pediatric trauma admissions to hospital, 1.5 percent involve the cervical spine. Motor vehicle-related accidents account for 48–61 percent of all pediatric cervical spine injuries. Falls account for 18–30 percent of cervical spine injuries below 8 years of age, and 11 percent over age 8 years [Gore et al., 2009]. Each year, 11,000 neck injuries, sustained while playing American football, present to emergency departments in the United States [Rihn et al., 2009]. Review of the National Pediatric Trauma Registry from 1988 to 1998 identified 75,172 injured children, of which 1.5 percent had a cervical spine injury. Eighty-three percent of the children had an injury of the bony spine, involving the upper cervical spine in 52 percent, the lower cervical spine in 28 percent, and both in 7 percent (13 percent unspecified). In 35 percent of the children, there was a spinal cord injury, that was incomplete in 76 percent and complete in 24 percent [Patel et al., 2001]. The National Trauma Data Bank (NTBD) was reviewed from 2001 to 2005 for children less than 3 years of age with a blunt cervical spine injury. Of 95,664 children below 3 years of age, 1.6 percent had a cervical spinal injury (bony spine and/or spinal cord). Although the most commonly fractured spine was C2, as has been found by others [Ruge et al., 1988], nearly half of all cervical spine fractures (47 percent) and more than half of the cervical spinal cord injuries (53 percent) were in the lower cervical spine (C5–7) [Polk-Williams et al., 2008]. The frequency of high cervical spinal injuries in very young children has been explained by the higher fulcrum of motion in younger children, and by weakness of C2 as a result of incomplete ossification of its synchondrosis [Proctor, 2002].
Motor vehicle accidents, including lap belt injuries to the lumbar spine, are the principal cause of spinal cord injuries, followed in decreasing order of frequency by falls, recreational or sports activities (especially diving accidents and football injuries), and penetrating injuries of the spinal column (e.g., gunshot, knife) [Gibson, 1992; Maroon et al., 1980; Massagli, 2000; Meyer et al., 1991; Mueller and Blyth, 1987; Schneider, 1964; Vogel and Anderson, 2003]. Since the 1980s, violence as a cause of spinal cord injury has increased from 10 to 30 percent in patients aged 16–20 years [Vogel et al., 1997]. Traumatic birth injury accounts for only 10–15 percent of all cases [Hadley, 1992]. Determinants of the severity of injury occurring after spinal cord trauma include the following:
The mortality from spinal cord injury in children is more than twice that seen in adults [Hamilton and Myles, 1992].
Anatomy
Bony Spine and Ligaments
The structure of the bony spine provides protection for and prevents excessive movement of the underlying spinal cord. Major ligaments that functionally divide the spine into anterior and posterior compartments maintain stability of the bony spinal column during movement [Chilton and Dagi, 1985]. Anteriorly, these ligaments are the anterior and posterior longitudinal ligaments; posteriorly, the ligaments include the ligamentum flavum and the interspinous, intertransverse, supraspinous, and facet capsular ligaments. Additionally, the transverse or cruciate ligament provides important stability between the atlas and axis. Stability of the spine as a whole is ensured if all of the anterior ligaments or if all of the posterior ligaments plus one anterior ligament are intact [Chilton and Dagi, 1985].
The most mobile portion of the spinal column is the cervical region, making that area the most common site of spinal cord injury [Green and Eismont, 1984; Hill et al., 1984]. Younger children are particularly susceptible to injuries of the high cervical cord [Davis et al., 1993; Piper and Menezes, 1996; Vogel et al., 1997]. Factors predisposing the upper cervical spine to injury in the young child include a proportionally larger and heavier head, relative underdevelopment of the neck muscles and ligaments, incomplete ossification of the vertebral bodies with relative anterior wedging [Massagli, 2000], incomplete development of the uncinate processes, smaller vertebral bodies of C1–C2 compared with C3–C5, and the more horizontal orientation of the articular surfaces of the cervical facet joints [Glasser and Fessler, 1996; Hadley et al., 1988; White and Panjabi, 1990]. Approximately 55 percent of spinal cord injuries involve the cervical spine, 30 percent involve the thoracic spine, and 15 percent involve the lumbar spine [Marion, 1998]. With birth trauma, spinal cord injuries are most common in the high cervical region (usually during an instrumental cephalic delivery) and in the lower cervical and upper thoracic regions (usually during a breech delivery). With increased use of cesarean sections to avoid potentially problematic vaginal deliveries, the frequency of spinal cord injury incurred during delivery has lessened appreciably [Morgan and Newell, 2001]. Between infancy and 8 years of age, 75 percent of spinal injuries occur in the cervical spine, particularly in its upper portion. Between age 8 and 14 years, 60 percent of injuries occur in the cervical spine, and 20 percent occur in the thoracic region, with the remainder at lower spinal levels [Menezes et al., 1989]. Conditions predisposing to cervical spinal cord injury in children include Down syndrome (15 percent have atlantoaxial instability), Klippel–Feil syndrome, Morquio’s syndrome, Larsen’s syndrome, achondroplasia, and previous cervical spine surgery [Caviness, 2004; Massagli, 2000].
The most common causes of cervical spine trauma in persons 18 years old and younger are motor vehicle accidents (45 percent), diving (23 percent), falls (11 percent), gymnastics (7 percent), football (5 percent), and other miscellaneous causes (8 percent) [Hill et al., 1984]. The second most mobile portion of the spine is the thoracolumbar region. It also is the second most commonly injured area, with T10 the site most frequently fractured [Ruge et al., 1988]. Regardless of the mechanism of injury, the thoracic spine (T2–T10) is the most common site of fracture in pediatric trauma patients [Reddy et al., 2003]. The thoracic and lumbosacral regions are the portions of the cord least often injured after trauma [Green and Eismont, 1984].
Spinal Cord
A disparity exists between vertebral and segmental cord levels, which changes with age. Early in fetal life, the spinal cord extends throughout the bony vertebral column, but during later development, the vertebral column becomes longer than the spinal cord. The caudal end of the cord comes to lie at successively higher vertebral levels; at birth, it is at the level of the second lumbar vertebra; in adulthood, it is at the first lumbar level. Disparity also exists between the levels of exit of nerve roots from the spinal canal and corresponding spinal segmental levels from which they originate, with the nerve roots emerging below their sites of origin (see Chapter 2). Below the first lumbar segment, there is no spinal cord, only the lumbosacral roots composing the cauda equina (Figure 78-1).
Blood Supply
The anterior spinal artery is an unpaired vessel formed by junction of a branch from the two vertebral arteries. It descends the entire length of the spinal cord ventrally in the anterior median sulcus. Between C3 and L3, the anterior spinal artery receives additional blood supply from anterior radicular arteries derived from lateral spinal arteries. Branches from the anterior spinal artery supply the entire anterior columns and most of the lateral columns of the spinal cord. The two posterior spinal arteries communicate with posterior radicular branches of the lateral spinal arteries to form a rich plexus of collaterals that supply the entire posterior columns and the remainder of the lateral columns of the spinal cord [Herren and Alexander, 1939; Suh and Alexander, 1939].
Pathogenesis: Mechanisms of Spinal Cord Injury
When the spinal cord sustains traumatic injury, the forces applied to the spine usually have been sufficient to cause displacement of vertebral ligaments and bones [Kakulas, 1984]. There are five major ways in which trauma to the spine can result in injury to the spinal cord: forward flexion, lateral flexion, rotation, axial compression, and hyperextension [Chilton and Dagi, 1985]. The forces generated by these movements can result in vertebral distraction, dislocation, fracture, and disc herniation. Often, as is the case in motor vehicle accidents and birth trauma, several forces act at the same time to injure the spine [Glasser and Fessler, 1996]. Osseous disruption (fracture or displacement) is the most common cause of spinal cord injury. Ligamentous injury, without osseous disruption, causing spinal cord injury is common in children, resulting in spinal cord injury without radiographic abnormality (SCIWORA). Disc herniation occurs in approximately 50 percent of spinal cord-injured patients [Flanders et al., 1990].
Forward flexion can lead to wedge or teardrop fractures, which usually begin at or near the intervertebral disc (Figure 78-2). These fractures are often encountered in motor vehicle accidents involving passengers not wearing seat belts, but also can be seen in children wearing ill-fitting adult types of lap-sash belts (cervical seat-belt syndrome) [Hoy and Cole, 1993]. Excessive lateral flexion of the cervical spine is always accompanied by rotation and can cause a unilateral locked facet (Figure 78-3). This locked facet occurs when the inferior facet contralateral to the side of flexion rotates too far anteriorly and locks into the superior facet of the vertebral body below [Panjabi and White, 1980]. Simple flexion alone usually causes little damage, but with the addition of rotation, ligaments can rupture, rendering the spine unstable and prone to distraction and dislocation. Rotation and flexion, accompanied by posterior ligamentous tear, also can cause vertebral body shear fractures.
Axial compression typically occurs in diving accidents, falls, and sports injuries. Axial compression can result in a burst fracture in which the vertebral end plate is injured, allowing the intervertebral disc to rupture into the vertebral body, which then bursts. In the Jefferson fracture, a direct blow to the top of the head results in axial compression that is transmitted through the occipital condyles to the atlas, the arch of which bursts, allowing fragments to be displaced outward (Figure 78-4) [Shapiro et al., 1973]. Severe hyperextension is often encountered in rear-end motor vehicle accidents (whiplash injury) and in the head-shaking injury of child abuse. This hyperextension can result in transverse fractures of the neural arches of the cervical spine, usually accompanied by tearing of the anterior longitudinal ligament [Caffey, 1974; Janes and Hooshmand, 1965; Ommaya, 1969]. The so-called hangman’s fracture occurs from a hyperextension injury through the synchondrosis between the odontoid and the arch of C2 [Reynolds, 2000]. Because the vertebral arteries lie in the transverse processes of these vertebrae, they can be torn or thrombosed after such injuries, with resultant ischemia to the anterior two-thirds of the spinal cord. Such injuries have been reported after neck hyperextension in football games and in infants with C1–C2 instability [Gilles et al., 1979; Schneider et al., 1970]. Displacement of the vertebrae during spinal trauma can compress the arteries of the spinal cord, resulting in ischemic injury, often without radiographic abnormalities.
Neuropathology
The nature and severity of traumatic injury to the spinal cord are related to the type and location of vertebral damage, the presence of any pre-existing disorder affecting the size of the spinal canal or mobility of the spinal cord (e.g., spinal stenosis, spondylitis, prolapsed disc), and the occurrence of concomitant vascular injury [Braakman and Penning, 1976; Chilton and Dagi, 1985]. Pathologic examination after spinal cord trauma has disclosed injuries that are either intraspinal intramedullary (affecting the spinal cord primarily) or, less often, intraspinal extramedullary (affecting the spinal cord secondarily). Intraspinal intramedullary pathologies include concussion, ischemia, contusion, compression, laceration, and intramedullary hemorrhage [Jellinger, 1976]. Intraspinal extramedullary injuries include epidural, subdural, and subarachnoid hemorrhages; epidural abscess; arachnoid cyst; epidermoid tumor; herniation of the nucleus pulposus; and cauda equina injuries.
Intraspinal Intramedullary Lesions
Pathogenesis and Pathologies
In spinal concussion, there is a functional rather than pathologic disturbance, usually with return of function within hours [Tator, 1996]. Extravasation of potassium from neurons into the extracellular space, after direct injury of nerve cell membranes or secondary nerve cell damage from vascular disruption, is the most likely mechanism of spinal cord concussion. Ischemia of the spinal cord can occur from anterior spinal artery or other vascular compression during spinal cord trauma, but also can occur when the trauma does not involve the spinal cord directly. Hypotension, systemic shock, and vascular injury to the aorta or a vertebral artery are the most common causes of this type of injury. With compression of the anterior spinal artery, the anterior two-thirds of the spinal cord is affected, causing weakness and loss of pain and temperature perception, with preservation of proprioception and light touch. Contusion results from a blunt injury of the spinal cord without continued compression or disruption of nerve tissue, resulting in transient paresthesias and dysesthesias in the upper limbs, especially the hands (burning hands syndrome), accompanied by transient long tract signs. Recovery is incomplete, however, distinguishing contusion from concussion. Central cord necrosis (hemorrhagic or ischemic) is a common sequela that often can be seen on magnetic resonance imaging (MRI) [Bailes et al., 1991; Jellinger, 1976]. Compression of the spinal cord can be caused by vertebral dislocation or by pressure from bony fragments, a herniated disc, or extramedullary bleeding [Jellinger, 1976]. Laceration occurs when there is interruption of spinal cord tissue by bony fragments, knife or bullet wounds, fracture-dislocation, or severe stretching. In older children, the most frequent cause of laceration is a penetrating agent, whereas in newborns, hyperextension of the neck during breech delivery is the most likely cause [Allen et al., 1969; Bresnan and Abroms, 1974; Byers, 1975; Crothers, 1923]. Intramedullary hemorrhage, which can extend over several spinal cord segments (hematomyelia), is believed to result from direct injury to blood vessels or from altered vascular permeability, as from acidosis.
The pathology of intraspinal intramedullary injuries has been studied in detail in experimental animals and in human postmortem examination material [DeLaTorre, 1981; Dumont et al., 2001a; Jellinger, 1976; Kakulas, 1984; Norenberg et al., 2004; Park et al., 2004; Profyris et al., 2004]. To mimic most events that lead to various forms of human spinal cord injury, several experimental models have been developed, the most common being transection, compression, or contusion [Rosenzweig and McDonald, 2004]. Because there are striking pathophysiologic similarities between human spinal cord injury and experimental models of spinal cord injury, particularly in the rat, findings in experimental spinal cord injury are commonly extrapolated to human injury. Such extrapolation requires caution, however, because regulation of secondary events after spinal cord injury varies greatly among different animal species and strains [Profyris et al., 2004]. The pathologic changes observed in the spinal cord after trauma can be subdivided into early, late, and very late.
The pathophysiology of spinal cord injury is biphasic. First is the primary injury, which is mechanical (e.g., spinal fracture and/or dislocation), directly disrupting axons, blood vessels, and cell membranes. This is followed by the delayed onset of secondary injury, involving vascular dysfunction, edema, ischemia, excitotoxicity, electrolyte shifts, free radical production, inflammation, and delayed apoptotic cell death. While neurologic deficits are seen immediately following the initial injury, the secondary injury phase results in a protracted period of tissue destruction [Rowland et al., 2008].
Phases of secondary injury
Immediate Phase (0–2 Hours)
During the immediate phase of spinal cord injury, which can last for up to 2 hours, traumatic severing of axons, the immediate death of neurons and glia, and accompanying spinal shock result in immediate loss of function at and below the level of complete injury. Pathological changes consist of swelling of the spinal cord, often with hemorrhage in the central gray matter and surrounding white matter, and necrosis (Figure 78-5) and spinal cord ischemia extending for many segments rostral and caudal to the injury site. Activation of microglial cells begins almost immediately, with the upregulation of the proinflammatory cytokines tumor necrosis factor (TNF)α and interleukin (IL)-β, detectable within minutes of injury. Also within minutes, levels of extracellular glutamate can reach excitotoxic levels.
Acute Phase (2–48 Hours)
The acute phase of spinal cord injury lasts from 2 to 48 hours. It is characterized by continuing hemorrhage, increasing edema and inflammation, and the onset of additional secondary injury processes, including free radical production, ionic dysregulation, glutamate-mediated excitotoxicity, and immune-associated neurotoxicity, which cause vascular disruption, hemorrhage, and resulting ischemia, with further axonal injury and cell death. The ischemia results in cytotoxic cell swelling, affecting neurons and glia, and axonal swelling. Loss of ion homeostasis immediately following spinal cord injury and excitotoxicity both contribute significantly to the propagation of cell injury after spinal cord injury. Ion dysregulation is also a central feature of necrotic and apoptotic cell death. Excitotoxicity results from excessive activation of glutamate receptors and is believed to play a role in the death of neurons and glia. Production of free radicals gives rise to lipid peroxidation, contributing to axonal disruption and death of neurons and glia. The modest protective effect of methylprednisolone treatment following acute spinal cord injury is believed to be due, at least in part, to the inhibition of lipid peroxidation [Kwon et al., 2004]. An additional acute-phase change is a marked increase in permeability of the blood–brain barrier. Death of neurons at all stages of injury occurs mainly from necrosis; by contrast, oligodendroglia readily undergo apoptosis. Loss of oligodendroglia results in axonal demyelination; persistent demyelination and other forms of axonal injury can then cause death of associated cell bodies. Animal studies have provided strong evidence that spared demyelinated axons are present following contusive spinal cord injury, and represent an important therapeutic target for spinal cord injury treatments that either remyelinate (cell transplants) or improve axonal conduction in demyelinated fibers (4-aminopyridine) [Hayes et al., 2004; Nashmi and Fehlings, 2001]. On the other hand, postmortem human studies and a limited number of animal studies [Lasiene et al., 2008] have not convincingly shown significant numbers of spared demyelinated axons to be present after human spinal cord injury [Kakulas, 2004; Norenberg et al., 2004].
Chronic Phase (6 Months or More)
The chronic phase of spinal cord injury begins 6 months after injury and continues through the patients lifetime. Scar formation continues, and the central portion of the spinal cord is replaced by a cystic cavity extending for several segments above and below the point of impact, resulting in post-traumatic syringomyelia; this extension can continue over many years, significantly increasing the patient’s neurological deficit, including ascending paralysis, brainstem symptoms, and neuropathic pain [Barnett and Jousse, 1976; Profyris et al., 2004; Rowland et al., 2008; Tator, 1996]. Wallerian degeneration of ascending and descending tracts occurs within 6–12 months [Kakulas, 1999], and nerve roots at the level of the lesion become atrophic. The cord becomes thin in the area of injury, with fibrosis and thickening of the overlying meninges [Kakulas, 1984]. At this chronic phase, therapeutic strategies are directed to encouraging regeneration/sprouting of disrupted axons, promoting plasticity with rehabilitative strategies, and improving function of demyelinated axons. Despite much success with stem cell-based approaches when applied subacutely, use of stem cells during this chronic phase of injury has not been shown to be successful [Rowland et al., 2008].
Clinical Assessment
General Physical Examination
It is crucial to assess the entire child, even in the presence of an obvious spinal injury. Particular attention should be directed to the vital signs. Respiratory difficulty may be caused by a traumatic pneumothorax or by diaphragmatic paralysis consequent to injury of the midcervical spinal cord. Hypotension with tachycardia may result from intra-abdominal bleeding from a ruptured spleen. Temperature instability may accompany spinal shock. The entire spine, particularly the cervical portion, should be examined with great care. One should look for overlying bruising and any spinal deformity. While in-line immobilization of the spine, with particular attention to the neck, is maintained, the entire spine should be palpated for any point tenderness, deformities, crepitus, or muscle spasm [Kadish, 2001].
Neurologic Examination
The diagnosis of spinal cord injury is often overlooked in children with multiple injuries, unless constant vigilance to detect such injury is maintained. Spinal cord trauma should be suspected in cases of breech or otherwise difficult delivery; multiple trauma; child abuse; and certain sports injuries, such as football, gymnastics, and ice hockey [Braakman and Penning, 1976; Torg et al., 1979]. Patients with spinal cord injury can present with any combination of the following symptoms and signs: neck or back pain, weakness, sensory loss, decrease in deep tendon reflexes, loss of bladder or bowel control, autonomic dysfunction, and meningismus. Such symptoms may be classified further as acute, subacute, chronic, intermittent, delayed or late, or progressive. After the initial neurological deficit in acute spinal cord injury, secondary deterioration is sometimes seen. Such worsening can be:
Signs of spinal cord injury usually include impairment of motor and sensory function. The degree of motor involvement can vary from subtle weakness to complete paraplegia or quadriplegia. Loss of sensation is often ascertainable at an obvious dermatomal level. In 1992, the American Spinal Injury Association (ASIA) and the International Medical Society of Paraplegia (IMSOP) published a Standard Neurological Classification of Spinal Cord Injury (Figure 78-6). Such a classification offers a systematic documentation of motor, sensory, and sphincter function for the accurate classification and scoring of acute spinal cord injuries. Ten key muscle groups are tested for motor function, using the Medical Research Council 0–5 muscle grading system, and sensation is tested over 28 dermatomes on both sides of the body. The neurological levels and completeness or incompleteness (partial preservation) of spinal cord involvement are documented [American Spinal Injury Association, 1992].
In 1992, the ASIA with the IMSOP developed the ASIA/IMSOP Spinal Cord Impairment Scale (Box 78-1) [Tator, 1996]. The ASIA/IMSOP scale incorporates the Medical Research Council muscle grading and pinprick/light touch sensory testing into a system differentiating complete and incomplete spinal cord deficit. Complete injury is defined as loss of all motor and sensory function in all segments below the neurological level, including the sacral dermatomes (S4–S5). To make this determination, peroneal and deep anal sensation and digital sphincter tone and contraction must be totally lacking. If there is preservation of any sensory or motor function below the neurological level, the injury is classified as incomplete (see Figure 78-6). Using this classification system, the examiner identifies the sensory and motor levels, defined as the most caudal spinal cord segment with normal sensory and motor function, on both sides. The zone of partial preservation is defined as the dermatomes caudal to the neurological level with partial preservation of function in an otherwise complete injury. A zone of partial preservation implies injury of multiple spinal cord segments, whereas its absence implies injury at only one level.
Box 78-1 American Spinal Injury Association/International Medical Society of Paraplegia Spinal Cord Impairment Scale*
Occasionally, brachial or lumbosacral plexus injuries causing weakness and sensory loss in a radicular distribution may coexist with a spinal injury, complicating the clinical picture. After injury to the spinal cord, the deep tendon reflexes are depressed or absent, with this lack of response persisting until the phase of spinal shock has resolved (1–12 weeks) [Green and Eismont, 1984; Guttmann, 1976; Meinecke, 1976]. Thereafter, hyperreflexia and extensor posturing are evident below the level of the lesion. Accompanying autonomic dysfunction can be manifested by Horner’s syndrome, hypertension, bowel and bladder atonia, and disturbances in temperature regulation.
Laboratory Studies
Radiographic Evaluation
Radiographic evaluation of the child suspected of having spinal cord injury should begin in the emergency department with plain films of the entire spine. The purpose of the evaluation is to look for evidence of unstable fractures or vertebral dislocations that may require emergency surgical intervention. Radiography of the entire spine is important because approximately 15 percent of patients have injury at multiple sites [Hadley, 1992; Hadley et al., 1988]. Radiological evaluation of the spine is often neither possible nor desirable in an emergency situation, however, and may need to be deferred until the spine is stabilized. In such circumstance, only the spinal area clinically involved needs immediate imaging. In cases in which the patient cannot be assessed adequately by plain radiography, MRI may be required early in the evaluation. Stringer and Andersen suggested a diagnostic algorithm for spinal injury combining the findings on neurological examination and results of radiographic studies [Andersen and Stringer, 1996; Stringer and Andersen, 1996].
Children with spinal cord injury routinely have sandbag and tape fixation of the head and neck, making optimal radiographic examination more difficult. Nonetheless, it is crucial to obtain anteroposterior, lateral, and oblique views of the spine. All seven cervical vertebrae and the C7–T1 interspace must be visualized. Swimmer’s views are often necessary to visualize all of the lower cervical vertebrae, and an open-mouth view may be needed to visualize the odontoid process [Goldberg et al., 1990], although the open-mouth view can be safely omitted if the other views are normal [Dias, 2004]. Flexion/extension cervical x-rays and fluoroscopy are sometimes indicated to exclude ligamentous instability when there is still suspicion of cervical spine instability after static x-rays have been obtained [Hadley, 2002b]. Care must be taken not to misinterpret normal variations in cervical spine films of a child. These variations include pseudosubluxation of C2 on C3 (seen in 20 percent of normal children age 1–7 years) and the cartilaginous plate at the base of the odontoid (normally seen in children <3 years old and often mistaken for a recent fracture) [Cattell and Filtzer, 1965].
Occipitoaxial dislocations can be identified on plain radiographs in some patients using the Powers ratio (distance of the basion [midpoint of the anterior border of the foramen magnum] to the posterior arch of C1, divided by the distance of the opisthion [midpoint of the lower border of the foramen magnum] to the anterior arch of C1). This ratio is usually 0.77. Ratios greater than 1 are abnormal. Most often, MRI is needed to identify occipitoaxial dislocations. Instability of the atlantoaxial region is suspected when the preodontoid space exceeds 5 mm in a child or 3 mm in an adult. Fractures of the atlas often can be stabilized by using a rigid (Philadelphia) collar [Alker et al., 1975]. Odontoid fractures and hangman’s fractures are rare in childhood and, if present, usually can be treated nonoperatively (halo brace) [Anderson and D’Alonzo, 1974].
Measurements made to assess alignment of the spinal column follow the “rule of 2”: that is, the allowable displacement between vertebrae in any direction should not exceed 2 mm in adults, with a greater allowance in children. The evaluation of bony integrity can be difficult at times, especially of the odontoid process and C1. If adequate quality cannot be obtained by plain radiographs, computed tomography (CT) is indicated [Andersen and Stringer, 1996; Stringer and Andersen, 1996].
Further limitations of plain radiographs of the spine include 40 percent false-positive and 20 percent false-negative interpretations. Also, significant spinal cord injury frequently occurs in children without radiographic abnormality (SCIWORA) (e.g., vertebral fracture or dislocation) [Burke, 1974; Pang and Wilberger, 1982], with this discrepancy observed in 15–70 percent of all pediatric spinal cord injuries [Hadley et al., 1988; Menezes et al., 1989; Pang and Wilberger, 1982]. Based on radiographic findings, spinal column injuries can be classified as follows:
SCIWORA, as first described, referred to cases of spinal cord injury with objective signs of myelopathy in the absence of abnormalities on static and dynamic flexion/extension films, CT imaging, and plain or CT myelography [Gore et al., 2009]. With modern MRI, many such cases show radiological evidence of injury to the spinal cord or spinal cord ligaments [Caviness, 2004; Davis et al., 1993; Matsumura et al., 1990; Proctor, 2002], with MRI abnormalities seen in about two-thirds of cases [Massagli, 2000]. Pang found MRI in 50 SCIWORA patients to have high prognostic value. The findings were complete transection or major hemorrhage (both with profoundly poor outcomes); minor hemorrhage (40 percent improved to mild disability); edema only (75 percent improved to mild disability); and normal (all recovered completely) [Pang, 2004].
Because the vertebral column in early life is quite elastic, allowing for significant flexion, hyperextension, and vertebral distraction without accompanying fracture or dislocations, SCIWORA is seen most often in infants and young children, in whom the frequency is three times greater than in children older than 10 years of age [Massagli, 2000]. Also predisposing to SCIWORA in young children is the greater elasticity of the spinal column than of the spinal cord; the bony spine can tolerate 5 cm of distraction, whereas the spinal cord can tolerate only 5 mm before disruption [Caviness, 2004]. Spinal cord ischemia from vertebral artery injury may also be contributory [Gore et al., 2009]. SCIWORA most often affects the cervical spine, typically producing signs of a central cord syndrome [Babcock, 1975]. In children less than 8 years, SCIWORA is more likely to be more rostral and more severe; from 8 to 16 years, it tends to be more caudal and less severe [Gore et al., 2009]. In some cases, transient neurologic symptoms may be the only indication that the cervical spinal cord has been injured; in other cases, permanent deficits may result, reflecting more severe spinal cord injuries that can be partial or complete [Caviness, 2004; Massagli, 2000]. In one study, 52 percent of children with SCIWORA had delayed onset of paralysis 4 days after their injury [Pang and Wilberger, 1982]. Children with spinal trauma must be examined frequently for signs of clinical deterioration in the days after their injury, even when plain radiographs of the spine are normal. Cervical immobilization of patients with SCIWORA is controversial. If dynamic films demonstrate stability of the cervical spine, the role of cervical immobilization is unclear. With only extraneural findings on MRI, neurological recovery, and no neck pain, 2 weeks of hard collar immobilization is recommended, followed by dynamic films; but with neural findings on MRI, the recommendation is for 12 weeks of hard collar immobilization before dynamic films are obtained [Gore et al., 2009]. Avoidance of high-risk activities for 6 months is recommended [Gunnarsson and Fehlings, 2003].
CT is the best means of detecting subtle spinal fractures and clarifying equivocal areas seen on plain films. CT also should be used when plain films are normal, but clinical suspicion of instability or fracture still exists [Andersen and Stringer, 1996; Stringer and Andersen, 1996], or when fracture/ displacement is seen on plain radiography [Caviness, 2004]. More recently, MRI of the spine has replaced CT and myelography as the preferred means of imaging the injured spinal cord (Figure 78-7) [Goldberg et al., 1988; Greenberg, 1991; Hyman and Gorey, 1988]. Many MRI sequences can be used, depending on the type of injury and the spinal cord area of concern, including spin-echo, fast-spin-echo, gradient-recall-echo, and inversion-recovery sequences. Excellent review articles on the use of these different sequences in specific situations are available [Andersen and Stringer, 1996; Stringer and Andersen, 1996]. MRI can distinguish intramedullary lesions from extramedullary ones, and early pathologic changes from later ones [Davis et al., 1993; Kalfas et al., 1988; Levitt and Flanders, 1991; Sett and Crockard, 1991]. In general, findings on MRI correlate well with the degree of deficit found on neurological examination [Bondurant et al., 1990; Davis et al., 1993; Flanders et al., 1990]. Additionally, the type and severity of spinal cord injury seen on MRI correlate well with the degree of recovery. In one study, MRI findings were classified into the following three categories:


Neurological recovery was insignificant in patients with intraspinal hemorrhage, while patients with cord edema or contusion recovered significant neurological function [Kulkarni et al., 1987] (Figure 78-8).
In general, spinal cord injuries in which there is no identifiable MRI abnormality carry a better prognosis for recovery than injuries in which MRI abnormalities are found [Davis et al., 1993]. The usefulness of MRI in visualizing spinal cord injury in neonates, in whom neurologic abnormalities can be difficult to find and in whom significance can be difficult to interpret, has been documented [Lanska et al., 1990]. MRI also is useful in showing abnormalities that may not be seen with other imaging modalities, including ligamentous instability and disruptions, other soft-tissue injuries, disc herniations, intraspinal hemorrhage, and nerve root injuries [Kadish, 2001; Proctor, 2002]. In some cases, it may be necessary to use a combination of MRI and CT to identify coexisting injuries of the spinal cord and bony spine [Levitt and Flanders, 1991; Wittenberg et al., 1990]. MRI can be useful in predicting outcome in intramedullary lesions because it can differentiate hematoma (which often has a poor prognosis) from spinal cord edema, which usually has a better outcome [Schaefer et al., 1992]. In 49 patients who underwent MRI within 72 hours of sustaining a cervical spinal cord injury, the imaging features that correlated with a poor functional recovery included hemorrhage, long segments of edema, and high cervical lesions [Flanders et al., 1999].
Functional MRI has been used to assess the degree of spinal cord damage in 27 patients with complete and incomplete cervical and thoracic spinal cord injuries. With thermal stimuli applied to the inner calf (L4 sensory dermatome), activity in ipsilateral dorsal gray matter was diminished with complete and incomplete injuries; in the ventral regions, however, activity was increased with complete injuries, but with incomplete lesions, it was similar to or diminished compared with healthy subjects (i.e., it was notably less than in subjects with complete spinal cord injuries) [Stroman et al., 2004].
Serial MRI studies also can be helpful in following the evolution of traumatic spinal cord lesions [Sato et al., 1994; Sett and Crockard, 1991]. The need for metrizamide CT, used previously in evaluating post-traumatic spinal cord cysts and syringomyelia [Mace, 1985; Quencer et al., 1983; Rossier et al., 1983], has diminished substantially with the accuracy of MRI in showing these lesions. Also, because of the availability of MRI, the need for myelography to identify surgically remediable spinal cord injuries (e.g., extramedullary hematomas and major fracture-dislocations) has diminished greatly. When the patient’s clinical condition permits, most centers currently advocate early MRI for acute spinal trauma.
Electrophysiologic Evaluation
Motor-evoked potentials (MEPs) provide a means to assess descending spinal tract function. MEPs are induced by transcranial magnetic stimulation of the motor cortex and are recorded on muscles of interest using surface electrodes to determine the level and extent of the spinal cord injury. It has been shown that MEP amplitudes improve with spinal cord injury recovery but latencies do not [Xie and Boakye, 2008].
The usefulness of somatosensory-evoked potentials (SSEPs) and electromyography in evaluating the level of spinal cord injury and predicting clinical outcome is controversial. SSEPs are often useful in identifying the level of spinal cord injury [Louis et al., 1985] and in identifying areas of spinal cord damage that have been clinically unapparent [Toleikis and Sloan, 1987]. This test can be especially helpful in infants and young children in whom the degree of deficit often cannot be determined confidently by clinical examination [Kamimura et al., 1988]. It is unknown, however, whether SSEPs are superior to MRI in identifying the extent of a spinal cord injury and predicting clinical outcome. Some investigators have noted a high degree of correlation between abnormal SSEPs and potential for neurological recovery [Curt et al., 1997; Li et al., 1990; Perot, 1973; Rowed et al., 1976; Spiess et al., 2008; Ziganow, 1986], but others have not [Katz et al., 1991; York et al., 1983]. One study of experimental spinal cord injury revealed no correlation between the absence of SSEP waveforms and the degree of spinal cord injury [Singer et al., 1977]. In that study, animals demonstrated good return of function at 8–9 days, even though SSEP waveforms were not recordable 3–19 days after injury. Other studies have suggested, however, that patients with absent SSEPs after spinal cord injury are unlikely to have any meaningful recovery of neurologic function [Shurman et al., 1996].
The sympathetic skin response (SSR) is another noninvasive electrophysiological test, in which EMG electrodes placed on the skin surface record responses to electric or magnetic stimulation above the spinal lesion. These recordings can be used to assess damage to the spinal and peripheral efferent sympathetic pathways subserving corresponding skin areas. While SSR has been used less than MEPs and SSEPs to assess completeness of spinal cord injuries, the addition of sympathetic nervous system testing may provide a more complete picture of the spinal cord injury [Curt and Dietz, 1999; Xie and Boakye, 2008].
The presence of F-waves and H-reflexes depends on the integrity of the motor neuron pool. H-reflexes disappear in the first 24 hours of spinal shock, but return thereafter; F-waves disappear below the level of injury during spinal shock and reappear with its resolution [Horowitz and Patel, 2003]. F-wave and H-reflex latencies and electromyogram can show lower motor neuron unit abnormalities 3–4 myotome levels beyond the site of a spinal cord lesion [Shefner and Tun, 1991]. Based on such electrophysiologic findings, the extent of deficit, whether functional or anatomic, is often found to be more extensive than indicated by clinical examination. As with SSEPs, it is uncertain whether abnormalities on electromyogram can increase significantly the predictability of clinical outcome in spinal cord injury.
Clinical Syndromes
Intraspinal Intramedullary Injuries
Intraspinal intramedullary injuries can cause complete or incomplete loss of spinal cord function [Chilton and Dagi, 1985; Tator, 1983; Tator et al., 1993]. With complete loss, there is absence of all motor, sensory, and reflex function below the level of injury. With incomplete loss, some motor, sensory, and reflex function persists (see Box 78-1).
Complete Spinal Cord Injuries
Complete loss of spinal cord function, as defined by the ASIA/IMSOP Spinal Cord Impairment scale, can be either physiologic or pathologic. In physiologic loss, there is no morphologic alteration of the spinal cord, which becomes dysfunctional after impact. This dysfunction may occur with transient compression of the cord by a dislocated vertebra. With more sustained compression of the spinal cord, anatomic disruption of spinal cord elements may result, with accompanying pathologic loss of function. The frequency of complete spinal cord injury in pediatric patients varies widely, depending on the series reviewed, ranging from 20 to 95 percent of all pediatric spinal cord injuries [Anderson and Shutt, 1980; Burke, 1974].
The initial phase of complete loss of spinal cord function is characterized by spinal shock [Green and Eismont, 1984; Kiss and Tator, 1993]. The truncal and extremity muscles below the level of the lesion are flaccid, deep tendon and superficial reflexes are depressed or lost, plantar responses are absent, anesthesia is present to all modalities, and autonomic dysfunction (hypotension and bradycardia) is present. The mechanism of spinal shock is unknown, but its physiologic effects must be differentiated from the more permanent pathologic effects of spinal cord injury. Motor and sensory deficits resulting from spinal shock alone resolve within 1 hour. Persistence of motor and sensory deficits beyond 1 hour implies that pathologic, more permanent injury has occurred. After 6–13 weeks in adults and within 1 week in children, tendon reflexes return, and muscle tone improves. The reflexes later become pathologically active, and spasticity ensues if the injury is more permanent, and there usually is no significant return of motor or sensory function. A four-phase model of the sequential clinical changes seen in spinal shock and the neuronal mechanisms that may underlie those changes has been described:

After complete injury of the cervical or upper thoracic spinal cord, an acute syndrome of bradycardia, hypotension, and hypothermia can occur, probably because of disturbed sympathetic outflow at the cervical and upper thoracic levels; most patients with quadriplegia after spinal cord injury have chronic hypothermia with poor temperature control related to loss of sympathetic peripheral vascular control [Green and Eismont, 1984]. Recovery from complete spinal cord injury is rare. The prognosis is poor. Tator estimated that less than 2 percent of patients with complete injury recover some distal cord function [Tator, 1996]. More encouraging was a comprehensive review of several large series of patients with complete spinal cord injury, which found that about 2 percent of the patients became ambulatory [Hansebout, 1982].
Incomplete Spinal Cord Injuries
Cervical nerve root/brachial plexus neuropraxia
American football, a high-energy contact sport, places players at particularly high risk for cervical neuropraxic injuries. The relatively common “stinger” is a reversible peripheral injury resulting from neuropraxia of cervical nerve root(s) or brachial plexus, causing a temporary physiologic block in nerve conduction. It is characterized by unilateral burning pain radiating from the neck down the arm to the hand, usually lasting seconds to hours. There may be associated weakness of the deltoid and/or supraspinatus/infraspinatus muscles that resolves within 24 hours to 6 weeks. The most common mechanism of injury appears to be hyperextension, often with lateral flexion of the neck and an axial load, resulting in cervical nerve root compression caused by intervertebral foraminal narrowing. Additional mechanisms of injury are traction or stretching of the brachial plexus and direct trauma to the brachial plexus. Radiographs are usually normal. Cervical stenosis appears to be a predisposing cause [Rihn et al., 2009].
Cervical cord neuropraxia
Less common and more serious is cervical cord neuropraxia, due to hyperextension, hyperflexion, or axial loading of the cervical spine. Clinically, it is characterized by burning pain, paresthesias, and loss of sensation and/or weakness (ranging from no weakness to complete paralysis) in more than one limb, with rapid and complete resolution of symptoms within 10 minutes to 48 hours. Mechanically, the posterior-inferior margin of the vertebral body above and the anterior-superior portion of the lamina of the vertebra below combine to produce spinal cord compression (Penning’s pincer mechanism), with local anoxia and an increase in intracellular calcium temporarily disrupting spinal cord function. Radiographs often show congenital abnormalities, such as cervical stenosis and Klippel–Feil syndrome. Cervical cord neuropraxia occurs in 0.2 per 100,000 participants at the high-school level and 2 per 100,000 at the collegiate level [Rihn et al., 2009].
Cervicomedullary syndrome
With upper cervical injury, there is often injury to the medulla as well, causing respiratory arrest, hypotension, quadriparesis, and facial and upper cervical (C1–C4) anesthesia. The facial anesthesia is of onion skin or Dejerine type. It is important that facial sensation be tested carefully in all patients with spinal cord injury. As in the central spinal cord syndrome (see later), there often can be more weakness in the arms than in the legs. This pattern of weakness is due to the crossing of fibers subserving the arms in the pyramidal decussation so that they come to be located more centrally than the leg fibers, which cross at a lower (C1–C2) level [Tator, 1996]. There often is selective arm weakness with little, if any, weakness of the legs [Bell, 1970]. Several authors have challenged this concept, pointing to evidence that the fibers of the corticospinal tract may not be layered but are intermingled, and that pathologically there is little evidence of central necrosis or hydromyelia in such patients [Quencer et al., 1992]. The mechanisms of injury causing cervicomedullary syndrome include atlantoaxial dislocation, atlanto-occipital dislocation, odontoid fracture, burst fracture of C1, and a ruptured C1–C2 disc [Baskin, 1996; Donahue et al., 1994; Tator, 1996].
Central spinal syndrome
Central spinal syndrome can complicate hyperextension injury, particularly when there is a pre-existing spinal abnormality, such as spinal canal stenosis, disc herniation, bony spurs, or compression by an abnormal ligamentum flavum [Quencer et al., 1992; Rand and Crowdall, 1962;]. Conversely, this syndrome often can occur, particularly in young children, without an accompanying radiographic abnormality [Osenbach and Menezes, 1989, 1992; Ruge et al., 1988]. Here the central portion of the cord (usually cervical) is damaged, probably from ischemia, because the central cord is perfused by end arteries from the anterior spinal artery, making it vulnerable to states of low perfusion [Schneider et al., 1973]. Neurologically, motor and sensory deficits are partial and are greater in the arms than in the legs, with the greatest impairment in the distal upper limbs [Green and Eismont, 1984]. Bowel and bladder function can be lost early, but usually return. Patients are generally ambulatory, with a spastic gait; painful dysesthesias in the arms may persist for years. There is still debate as to whether early surgery is warranted in this condition because many patients recover spontaneously [Schneider et al., 1973; Sonntag and Francis, 1995].
Anterior spinal cord syndrome
Anterior spinal cord syndrome, which can follow hyperflexion injury of the spine, is characterized by analgesia and paresis below the level of the lesion, with preservation of proprioception, light touch, and vibration [Schneider, 1955]. This condition is usually caused by direct compression of the anterior and lateral white-matter tracts by a herniated disc or by a fractured vertebra with posterior dislocation [Schneider, 1951]. The posterior columns are spared. There is no evidence for compression of the anterior spinal artery in this disorder [Tator, 1996]. Early surgery is probably warranted in all cases.
Posterior spinal cord syndrome
Posterior spinal cord syndrome is an extremely rare disorder (some doubt its existence), characterized by major damage to the posterior spinal cord (resulting in loss of movement and proprioception), with some residual function of the anterior cord (with retained perception of pain and temperature) [Tator, 1996].
Brown–séquard syndrome
Brown–Séquard syndrome is caused by injury of the lateral half of the spinal cord (usually cervical) and is characterized by ipsilateral motor paralysis, ipsilateral loss of touch and proprioception, and contralateral loss of pain and temperature sensation below the level of the lesion [DeMyer, 1985]. Although this syndrome is often caused by penetrating trauma to the spine, cases also have been caused by hyperextension injury, compression fractures, and disc herniation. Blunt trauma with central cord injury sometimes can result in asymmetric pareses and sensory impairment that can mimic the true syndrome [Chilton and Dagi, 1985]. Recovery is variable.
Conus medullaris syndrome
Conus medullaris syndrome produces paralysis of the lower extremities and the anal sphincter. Such a lesion, by definition, would be a form of complete spinal cord injury [Tator, 1983]. There is sacral sparing in many cases, however, making the injuries frequently of the incomplete type. The lumbar cord is positioned opposite the T12 vertebra, and the sacral cord is opposite L1. Dislocation, displaced fractures, and disc herniation of T12 and L1 can produce this injury. Prognosis for recovery is poor [Tator, 1983].
Intraspinal Extramedullary Injuries
Spinal Epidural Hematoma
Spinal epidural hematoma can occur with substantial trauma to a normal spine, particularly in a newborn after breech delivery, or with mild trauma to the spine in a patient with a bleeding diathesis, with a spinal epidural hemangioma, or after multiple lumbar punctures [Bruyn and Bosma, 1976; Hehman and Norrell, 1968; Robertson et al., 1979]. In contrast to most intracranial epidural hematomas, spinal epidural hemorrhage is of venous (not arterial) origin [Bruyn and Bosma, 1976]. The main venous structure within the spinal epidural space lies ventrolateral to the cord and comprises a “rope ladder” plexus of thin-walled veins lacking true valves. This internal plexus connects with an external plexus of veins around the spinal column, with segmental spinal veins draining into the inferior vena cava, and with intracranial dural sinuses [Bruyn and Bosma, 1976]. Signs of spinal epidural hematoma can be acute, chronic, or intermittent. A newborn with acute spinal epidural hematoma typically manifests respiratory depression, hypotonia, areflexia, and other signs of spinal shock [Francisco, 1970; Towbin, 1964]. The occurrence of clinically significant traumatic spinal epidural bleeding is uncommon after infancy. When it occurs in older children (as with minor trauma complicating a blood dyscrasia), there is usually severe back pain that is worsened by pressure over the spine, neck flexion, or the Valsalva maneuver. Over the ensuing hours to weeks, signs of spinal cord compression usually develop [Bruyn and Bosma, 1976].
Spinal Subdural Hematoma
Spinal subdural hematoma occurs much less commonly; the source of bleeding in this hematoma is unclear. Similar to spinal epidural hematoma, it occurs mainly in the neonatal period, although it too is occasionally observed in children and adolescents with bleeding diatheses who sustain an otherwise insignificant injury to the spine, such as that occurring at the time of lumbar puncture [Edelson, 1976; Edelson et al., 1974; Towbin, 1964; Walter and Tedeschi, 1970]. Clinical features include back and radicular pain [Edelson, 1976].
Spinal Subarachnoid Hemorrhage
Traumatic subarachnoid hemorrhage caused by injury to the spine also is uncommon. This hemorrhage can occur after penetrating injury, but most often occurs after birth trauma (frequently with accompanying epidural, subdural, or intraspinal hemorrhage) [Plotkin et al., 1966; Towbin, 1964]. The amount of blood that accumulates is usually not great, and significant compression of the cord is rare. Signs of spinal subarachnoid hemorrhage are difficult to distinguish from signs of other spinal hemorrhages (e.g., epidural) that often coexist. Spinal subarachnoid hemorrhage should be suspected when back pain, meningismus, or fever occurs. The diagnosis is indicated by the presence of blood in the spinal fluid or by cerebrospinal fluid xanthochromia. Occasionally, the blood obstructs the subarachnoid space, resulting in a “dry tap.” Spinal subarachnoid hemorrhage must be differentiated from subarachnoid bleeding of intracranial origin and from blood caused by a traumatic lumbar puncture.
Spinal Epidural Abscess
Infections of the skin overlying the spine or of the bony spine itself (osteomyelitis) can occur after spinal trauma and may result in a spinal epidural abscess [Baker et al., 1975; Browder and Meyers, 1941; Hancock, 1976; Heusner, 1948]. Staphylococcus aureus is the organism most often responsible for such infections [Joshi et al., 2003; Pereira and Lynch, 2005]. The signs and symptoms of spinal epidural abscess are difficult to differentiate from the signs and symptoms of spinal epidural hematoma. Usually, other signs of inflammation and local infection of the overlying skin or bone can be identified.
Spinal Arachnoid Cyst
Spinal arachnoid cyst can develop after spinal trauma or may appear idiopathically and can cause significant spinal cord compression [Elsberg et al., 1934; Herskowitz et al., 1978; Nugent et al., 1959; Swanson and Fincher, 1947]. These cysts produce progressive signs, with lower limb weakness in neonates, and recurrent gait and sensory difficulties in older children. Scoliosis may accompany these cysts. The diagnosis is suggested on plain films by widening of the interpeduncular distances [Elsberg et al., 1934; Herskowitz et al., 1978; Nugent et al., 1959; Swanson and Fincher, 1947].
Spinal Epidermoid Tumor
Epidermoid tumors are infrequent complications of lumbar puncture [Batnitzky et al., 1977; Gibson and Norris, 1958; Manno et al., 1962; Shaywitz, 1972]. These tumors may develop 1–20 or more years after a lumbar puncture, and have been attributed to skin and subcutaneous tissues implanted intraspinally at the time of a spinal tap. The symptoms are slowly progressive and usually manifest with back and leg pain followed by gait difficulties [Batnitzky et al., 1977; Gibson and Norris, 1958; Manno et al., 1962; Shaywitz, 1972].
Herniation of the Nucleus Pulposus
Herniation of the nucleus pulposus (protrusion of central intervertebral disc tissue) can result from severe flexion/compression of the spine and can cause compression of the underlying spinal cord [Burke, 1976; Herkowitz and Samberg, 1978; Swischuk, 1969]. The symptoms and signs are similar to those of any intraspinal extradural mass: pain and dysesthesias in radicular distribution, loss of tendon reflexes, muscle weakness, and atrophy [Burke, 1976; Herkowitz and Samberg, 1978; Swischuk, 1969].
Cauda Equina Injuries
A special category of extramedullary injury comprises injury involving the cauda equina, which is technically not a spinal cord injury. The level of spinal column and ligament displacement in these injuries varies with age, depending at which vertebral level the spinal cord terminates (L2 in infancy and L1 in adulthood). When any of the extramedullary pathologies just discussed are localized below the spinal cord, they can cause injury to the cauda equina. In incomplete injuries of the cauda equina, there is always preservation of sensation, often with only a partial motor deficit [Tator, 1996]. Bowel and bladder involvement is common. The prognosis in cauda equina injury is better than the prognosis in spinal cord injury because the lower motor neuron apparently has a greater capacity to recover than the upper motor neuron [Tator, 1996].
Catastrophic Spinal Cord Injuries
Although quite rare, catastrophic neurological injury refers to a complication of a cervical spinal cord injury, probably most often caused by a neck injury suffered while playing tackle football, which results in permanent neurological injury or death. The incidence of this injury is 0.5 per 100,000 participants at a high-school level and 1.5 per 100,000 at the collegiate level. The mechanism of injury is most often forced hyperflexion, as occurs with “spear tackling,” in which the player initiates contact with the crown of his helmet, with his neck in a slightly flexed position, resulting in an axial force applied to the helmet being transmitted to the cervical spine, with fracture and/or subluxation or dislocation of the subaxial spine. Of the permanent neurological injuries that occur in football, 70 percent occur in defensive players, in the overwhelming majority while tackling or blocking [Rihn et al., 2009].
Supraspinal Changes
There is a rich literature on experimental spinal cord injuries in rodents and nonhuman primates showing evidence for degeneration of neurons in the cerebral cortex following the injury. Some cells undergo apoptotic cell death, while others may only undergo severe atrophy but not actually die. In the latter cases, the local addition of neurotrophic factors to a particular brain region can reverse some of these morphological changes [Bramlett and Dietrich, 2007]. Additionally, circuit reorganization and plasticity, resulting in deactivation and reactivation of certain brain areas, has been demonstrated after clinical and experimental spinal cord injury [Bramlett and Dietrich, 2007; Jain et al., 1997].
Management
Short-Term Management
Spine Immobilization and Supportive Care
The treatment of acute spinal cord injury must include stabilization of the spine to prevent further injury, in addition to maintenance of vital signs. First aid at the scene of the accident should include placement of the child on a rigid stretcher or firm backboard, and fixation of the head by sandbags or towel rolls with tape over the forehead to prevent movement of the head on the spine. A cervical collar alone does not accomplish this goal adequately [Green and Eismont, 1984]. Restriction of movement manually with the arm on the chest and hand on the mandible, exerting slight extension and traction on the head, can help stabilize the cervical spine until the head is taped. Care must be taken not to exert too much spinal distraction with this maneuver [Benzel and Doezema, 1996]. The disproportionately large head of a young child places the child into flexion when he or she is put on a neutral board. Because the high cervical region is the most likely level of injury, neck flexion is particularly dangerous. Flexion can be avoided with a special board with a recess for the occiput, allowing the head to rest in line with the body, or by placing something under the child’s shoulders to elevate the neck in line with the head [Proctor, 2002]. It has been estimated that careful attention to stabilization of the spine before moving the patient has reduced occurrence of complete spinal cord injury by approximately 10 percent [Gunby, 1981]. More recently, it has been suggested that this type of immobilization is painful and potentially harmful (restricted breathing, tissue ischemia, decubiti, intracranial hypertension), offering little, if any, benefit. Although a spine board eases transfer of a patient with a potentially unstable spinal injury to and from an ambulance stretcher, when the patient arrives at the hospital, a hard cervical collar and a firm mattress adequately immobilize the patient before application of traction or definitive stabilization [Hauswald and Braude, 2002].
A patent airway must be established, which may necessitate endotracheal intubation; this is frequently required in complete cervical cord injury (with impairment of diaphragmatic and intercostal muscle function). Intubation should be accomplished by chin lift without or with minimal neck extension. Tracheostomy and cricothyroidotomy should be avoided. Cardiac rate and rhythm should be monitored, and intravenous, central venous, and arterial lines should be placed. Cervical spinal cord trauma is often associated with significant hemodynamic deficits, including hypotension. In addition, injury to other organs (e.g., a ruptured liver or spleen) can cause significant blood loss and hypotension, which must be treated promptly by intravenous crystalloid or blood. Intravenous atropine, glycopyrrolate, phenylephrine, or dopamine may be needed to maintain normal blood pressure after volume depletion is corrected. Aggressive treatment of hypotension after spinal cord injury may improve outcome significantly [Levi et al., 1993; Vale et al., 1997]. The stomach should be emptied by a nasogastric tube. With urinary retention, the bladder should be catheterized. Radiologic studies usually should be performed (see section on radiologic evaluation earlier). In the case of cervical spine trauma, if the child is alert and interactive and has no neurologic deficit, no midline cervical tenderness, no painful distracting injury, and no evidence of intoxication, cervical spine films are probably not needed [Hadley, 2002b]. Spinal alignment is an important next step that usually can be accomplished with skeletal traction [Ducker et al., 1983] (see section on long-term management later).
Surgical Management of Acute Spinal Cord Injury
It is surprising that a question as fundamental as whether early decompression for acute spinal cord injury is or is not beneficial for neurological recovery remains incompletely answered. A significant body of animal research has demonstrated neurological benefit from early decompression of the injured spinal cord, but some clinicians prefer to delay decompression in patients with multiple trauma because of medical instability often seen in the acute post-injury phase. Earlier studies comparing surgical and nonsurgical management in acute spinal trauma come mainly from adult series. In patients with complete spinal cord lesions treated conservatively, 27–34 percent showed some improvement [Frankel et al., 1969; Guttmann, 1963]. Of 27 patients with complete lesions who were managed surgically, 25 improved, but this improvement was seen only in nerve-root function at the site of the injury [Stauffer, 1984]. In patients with incomplete spinal cord lesions, 64–90 percent have improved with conservative treatment [Guttmann, 1963; Meinecke, 1964]. In 22 cases of cervical spine dislocation with neurological changes, recovery (complete in 213) occurred in 82% of cases treated with early spinal fusion [Forsyth et al., 1959]. Thus, in incomplete and complete spinal cord lesions, there would appear to be no clear differences in neurological outcome between surgical and nonsurgical management. The Surgical Treatment of Acute Spinal Cord Injury Study (STASCIS), initiated in 2003 at the University of Toronto and Thomas Jefferson University, was designed to be randomized, but resistance to randomizing to an intentionally delayed decompression led to restructuring as a prospective observational study. The study has an accrual target of 450 patients with traumatic cervical spinal cord injuries ranging in age from 16 to 70 years. A 2-year follow-up period post injury is planned. Preliminary analysis suggests a benefit to decompression in less than 24 hours of injury [Hawryluk et al., 2008].
A Medline search of experimental and clinical studies describing on the effect of decompression on neurological outcome following spinal cord injury was reported in 2005 by Fehlings and Perrin. Animal studies consistently show that neurological recovery is enhanced by early decompression. One randomized controlled trial showed no benefit to decompression in less than 72 hours, but several prospective studies suggested that decompression in less than 12 hours could be performed safely and may improve neurological outcome. A recent meta-analysis showed that decompression in less than 24 hours resulted in statistically better outcomes than both delayed decompression and conservative management. In summary, there currently are no standards regarding the role and timing of decompression in acute spinal cord injury [Fehlings and Perrin, 2005].
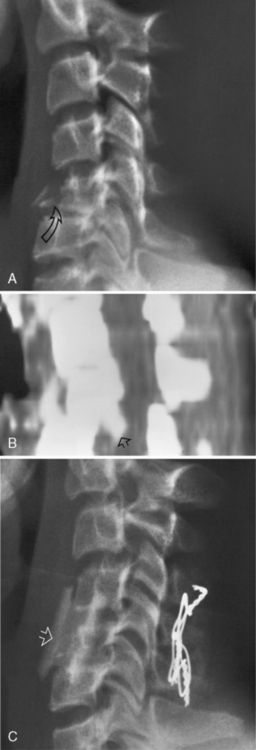
A, Plain lateral film of the cervical spine in a 12-year-old female. There is a wedge-shaped fracture of C5 (arrow) with posterior dislocation of C5 on C6 and loss of the disc space between those two vertebrae. B, Computed tomography sagittal reconstruction from 2-mm axial scans through the C4–C6 level (unenhanced study) in the same patient as in Figure 78-7. Posterior dislocation of a fracture fragment (arrow) from the inferoposterior margin of C5 has resulted in narrowing of the anteroposterior diameter of the spinal canal. C, Plain lateral film of the cervical spine in the same patient as in Figure 78-7. The spine was stabilized by anterior bony fusion (arrow) and posterior wiring to prevent further narrowing of the spinal canal.
Completed Randomized Controlled Clinical Trials for Medical Treatment of Acute Spinal Cord Injury
Ten randomized controlled treatment trials examining methylprednisolone, naloxone, tirilazad, GM1 ganglioside, thyrotropin releasing hormone, nimodipine, and gacyclidine have been completed [Hawryluk et al., 2008].
Methylprednisolone, naloxone, and tirilazad
Steroids have often been used in the treatment of acute spinal cord injury in an attempt to reduce cord swelling and limit central cord necrosis [Babcock, 1975; Ducker and Hamit, 1969]. In experimental models of spinal cord injury, methylprednisolone, given within hours of the injury, has been found to affect the cascade of inflammatory responses after spinal cord injury, mainly by scavenging damaging free radicals but also by influencing excessive calcium influx into cells and inhibiting the release of eicosanoids and cytokines. When methylprednisolone has been given later in such models, it has been found to block regenerative mechanisms. Other potential mechanisms of action of steroids include stabilizing membranes, maintaining the blood–spinal cord barrier, reducing vasogenic edema, enhancing spinal cord blood flow, altering electrolyte concentrations at the site of injury, blocking endorphin release, inhibiting lipid peroxidation, and limiting inflammation after injury [Ball and Nockels, 2001; Hadley, 2002a]. Review of 62 animal studies on effects of methylprednisolone administration on functional outcome in acute spinal cord injury showed a beneficial effect in 34 percent, no effect in 58 percent, and mixed results in 8 percent [Akhtar et al., 2009].
Until 1990, steroid treatment had shown no apparent benefit in humans, possibly related to the size and the timing of the steroid dose. In a randomized controlled study, a methylprednisolone bolus of 30 mg/kg, given intravenously after an acute spinal cord injury and followed by methylprednisolone infusion at 5.4 mg/kg/hr for the next 23 hours, was compared with naloxone and placebo, both also given by bolus followed by a 23-hour infusion (National Acute Spinal Cord Injury Study [NASCIS] 2 protocol) [Bracken et al., 1990]. Naloxone, an opiate-receptor antagonist, has been used with apparent success after spinal cord trauma in animals, demonstrating short-term motor function improvement [Baskin et al., 1993], and suggesting that endorphins might influence outcome in spinal cord injury [Faden, 1996]. Administration of methylprednisolone within 8 hours of injury was associated with a significant improvement in motor function and sensation at a 6-month follow-up examination, compared with patients receiving methylprednisolone more than 8 hours after injury and with patients receiving naloxone or placebo. This difference was found with complete and incomplete spinal cord lesions. Re-analysis of the data indicated, however, some benefit from the use of naloxone with injuries that were incomplete [Bracken and Holford, 1993]. At 1-year follow-up, patients who had received methylprednisolone within 8 hours of injury still exhibited significantly improved motor scores, but there were no significant differences in sensory scores among the three groups [Bracken et al., 1992]. The improvements noted were small, however, and the functional significance of these gains has been questioned [Wilberger, 1996]. Also, wound infections and gastrointestinal bleeding occurred more often in the patients given methylprednisolone than in the other two groups. A similar beneficial effect of methylprednisolone was not found when it was given in an identical fashion to patients after penetrating spinal cord injury [Levy et al., 1996]. In an earlier study by Bracken and colleagues (NASCIS 1), high-dose methylprednisolone (1000-mg bolus [14.3 mg/kg for a 70-kg patient], followed by 1000 mg/day for 10 days) was compared with standard-dose methylprednisolone (100-mg bolus [1.4 mg/kg for a 70-kg patient], followed by 100 mg/day for 10 days). No significant difference was found between the two groups in recovery of motor or sensory function 1 year after injury. There were no placebo control subjects in the study [Bracken et al., 1984].
A 1997 study by Bracken and colleagues compared the efficacy of methylprednisolone given for 24 hours with that of methylprednisolone given for 48 hours and with tirilazad, a 21-amino steroid lipid peroxidation inhibitor, given for 48 hours; there was no placebo control group (NASCIS 3). When treatment was begun 3–8 hours after injury, the patients who received methylprednisolone for 48 hours demonstrated significantly better motor function at 6 weeks and 6 months after injury than the other two groups. Also, at 6 months, the 48-hour methylprednisolone patients had significantly better functional recovery (self-care, sphincter control) than the other two groups. The 48-hour methylprednisolone patients also had more severe sepsis and severe pneumonia. When treatment was begun within 3 hours of injury, the rates of motor recovery at 6 weeks and 6 months were identical in the two groups given methylprednisolone and the one given tirilazad, confirming reports of tirilazad’s benefit in experimental spinal cord injury [Bracken et al., 1997; Francel et al., 1993]. One year after injury, when treatment had been started within 3 hours of the injury, neurologic and functional recovery was again equal among the three treatment groups. When treatment had not been started until 3–8 hours after injury, patients who had received methylprednisolone for 48 hours had greater motor recovery at 1 year, whereas motor recovery was diminished in patients who had received only 24 hours of methylprednisolone [Bracken et al., 1998]. Based on this study, it was concluded that patients who receive methylprednisolone within 3 hours of injury should be maintained on the drug for 24 hours, but when methylprednisolone is begun 3–8 hours after injury, it should be continued for 48 hours unless there are complicating medical factors.
In a study during a 2-year period (2003–2005) in Japan, all patients with a cervical cord injury were treated with high-dose methylprednisolone (NASCIS 2 protocol) (38 patients), while during the next 2 years (2005–2007), all patients were treated without steroids (41 patients). Early spinal decompression and stabilization were performed as soon as possible in both groups. There was no improvement in the ASIA motor score in either group, regardless of the degree of motor loss (complete, incomplete). In the steroid group, 19 of 38 patients (50 percent) developed pneumonia vs. 11 of 41 (27 percent) in the nonsteroid group (clinically significant); there were, however, no differences between the steroid and nonsteroid groups in frequency of urinary tract infections and wound infections [Ito et al., 2009].
It is not surprising that many investigators have disagreed with the recommendation that steroids be given for the immediate management of acute spinal cord injuries, citing largely insignificant differences in motor recovery scores and in functional outcome measures among study patients, while also detailing methodologic, scientific, and statistical flaws in the NASCIS 2 and 3 studies. The role of steroids as neuroprotective agents in acute spinal cord injury remains controversial [Ball and Nockels, 2001; Hadley, 2002a; Nesathurai, 1998]. Although the NASCIS 2 and 3 studies show some improvement in neurological function from high-dose methylprednisolone, the benefit seems to be modest at best, and against, this one needs to weigh the risk of potentially serious complications of high-dose steroid therapy [Galandiuk et al., 1993; Hadley, 2002a].
GM1 Ganglioside
Based on experimental studies that have shown GM1 ganglioside to induce the regeneration of damaged neurons, a randomized, placebo-controlled trial of GM1 was performed on 34 patients after spinal cord injury [Geisler et al., 1991]. Subjects were given 100 mg of either GM1 or placebo intravenously within 72 hours of injury and continued daily for a mean duration of 26 days. No untoward neurologic events related to GM1 administration were seen, and better recovery occurred in the GM1-treated patients than in the controls after 1 year of follow-up. The number of patients treated was small (16 GM1, 18 placebo), and there was an uneven distribution between the two groups, with a smaller number of patients with the most severe injuries (complete spinal cord injury) randomized to the GM1 group (38 percent of GM1-treated patients versus 56 percent of the placebo group) [Landi and Ciccone, 1992]. Functional outcomes of patients were considered to be equivalent in the two groups.
A multicenter GM1 ganglioside study in patients with acute nonpenetrating spinal cord injury of at least moderate severity was initiated in 1992. The study was prospective, double-blind, randomized, and stratified. By the study’s end in 1997, 797 patients had been enrolled. (A primary efficacy analysis was done in 760 of the patients.) All patients first received intravenous methylprednisolone within 8 hours of injury and following the NASCIS 2 protocol. The patients were randomized into three study groups: placebo, low-dose GM1 (300-mg loading dose followed by 100 mg/day for 56 days), and high-dose GM1 (600-mg loading dose followed by 200 mg/day for 56 days). A planned interim analysis resulted in discontinuation of the high-dose GM1 treatment strategy because of an early trend for higher mortality. There was no significant difference in mortality between treatment groups. Although the data suggested improved neurologic recovery in patients with acute spinal cord injury given GM1 ganglioside for 56 days after the administration of methylprednisolone within 8 hours of an acute spinal cord injury, the primary analysis failed to prove this difference to be statistically significant. Nonetheless, because numerous secondary analyses indicated that GM1 was beneficial in the treatment of acute spinal cord injury, the use of low-dose GM1 after initial intravenous methylprednisolone remains a therapeutic option. The authors could not confirm the NASCIS 2 and NASCIS 3 findings indicating that the timing of methylprednisolone therapy had an impact on spinal cord recovery [Geisler et al., 2001; Hadley, 2002a].
Thyrotropin releasing hormone
Thyrotropin releasing hormone (TRH) has been shown to antagonize secondary injury mediators, such as excitotoxic amino acids, peptidoleukotrienes, endogenous opioids, and platelet-activating factor [Dumont et al., 2001b]. In the only randomized, double-blind, placebo-controlled trial of TRH in the treatment of acute spinal cord injuries, patients with incomplete injuries treated with TRH showed significantly better functional outcomes than the placebo group; there were no differences in outcomes with complete spinal cord injuries [Pitts et al., 1995]. This result must be interpreted with caution, however, because only 20 patients were ultimately analyzed.
Nimodipine
Intracellular calcium levels are tightly controlled, as high concentrations can activate intracellular calpains and other destructive enzymes, leading to apoptosis. Release of excitotoxic glutamate is also calcium-dependent. Nimodipine is an l-type calcium channel blocker that may antagonize these processes [Hawryluk et al. 2008; Lyden and Wahlgren, 2000]. One hundred and six patients with acute spinal cord injury were treated with one of the following methylprednisolone and nimodipine, and placebo. At 1-year follow-up, no neurologic benefit from treatment was found, but it is quite likely that the study had inadequate power to show a therapeutic effect [Petitjean et al., 1998].
Gacyclidine
This molecule is a noncompetitive antagonist of N-methyl-d-aspartate (NMDA) receptors, derived from phencyclidine. A double-blind, placebo-controlled trial of 280 patients with acute spinal cord injury were given three concentrations of gacyclidine. No improvement was seen, except in patients with partial cervical lesions given the highest gacyclidine dosage, but because of low numbers in each subgroup, statistical significance was not reached [Bauchet et al., 2009].
Additional Clinical Trials
Pharmacological trials
Minocycline
Minocycline is a synthetic tetracycline derivative that has been shown to attenuate secondary injury and enhances functional recovery in various animal models of spinal cord injury. Its mechanism of action in spinal cord injury appears to be mediated in part by the inhibition of microglial activation, in addition to reducing cell death [Hawryluk et al., 2008; Lee et al., 2003; Teng et al., 2004]. The results of two on-going clinical trials, one in Canada and one in Saudi Arabia, are awaited with interest [Hawryluk et al., 2008].
Riluzole
Riluzole is a benzothiazole anticonvulsant that has been shown to slow disease progression in ALS (amyotrophic lateral sclerosis). Its neuroprotective effects appear to result from blockade of voltage-sensitive sodium channels, whose persistent activation following injury is associated with cellular toxicity and neural degeneration [Schwartz and Fehlings, 2001]. Additionally, riluzole is a glutamate release inhibitor [Mu et al., 2000]. In experimental spinal cord contusion in rats, riluzole, in combination with methylprednisolone, has been shown to improve behavioral recovery and promote tissue sparing [Mu et al., 2000]. Further, in adult rats in whom the L4 root was avulsed and reimplanted into the spinal cord, the survival and reinnervating capacity of injured motor neurons was dramatically enhanced by riluzole, even when treatment was delayed for as long as 10 days after the injury [Nógrádi et al., 2007]. Hepatotoxicity is sometimes a limiting side effect of riluzole therapy [Hawryluk et al., 2008].
Erythropoietin
Erythropoietin (EPO) is a glycoprotein that mediates cytoprotection in a variety of tissues, including spinal cord. It has been felt to exert its beneficial effects by preventing apoptosis, reducing inflammation, and restoring vascular integrity, and also, possibly, by fostering neuronal regeneration. Studies in animal models of spinal cord injury have indicated effectiveness of treatment with recombinant human EPO (rhEPO). Human studies have yet to be done, in part because EPO, in addition to its tissue-protective effects, has hematopoietic properties increasing the risk of thrombosis [Matis and Birbilis, 2009]. In animal studies, rhEPO has been shown to counteract secondary injury and to enhance neurological recovery markedly following experimental spinal cord trauma; additional protective mechanisms suggested for rhEPO include lessening of neuronal ischemia and inhibition of nitric oxide production [Gorio et al., 2002].
Nonpharmacological trials
Hypothermia
The evidence supporting the beneficial effect of hypothermia in animal models of spinal cord injury is mixed, with the interpretation of animal studies made difficult by differing animal models, mechanisms of cord injury, severities of injury, and variations in the timing, duration, and extent of hypothermia [Kwon et al., 2008]. Human studies of hypothermia for spinal cord injury are similarly difficult to interpret because of small numbers of patients of different ages, injury severity, timing and duration of hypothermia, and additional treatments received (such as steroids and decompressive surgery). For example, in studies of regional hypothermia in human spinal cord injuries, the perfusate in different studies has been chilled saline, ice cold saline, 4 or 5°C chilled saline, a 6°C cooling saddle, epidural or intradural perfusion, given from 3 to 26 hours after the injury, for periods ranging from 30 minutes to 6 days. As a result, high-quality clinical studies that support the use of systemic hypothermia for spinal cord injury do not exist [Kwon et al., 2008].
While early investigations, such as those noted above, evaluated the effects of profound local hypothermia following spinal cord injury, recent studies have concentrated on the effects of modest hypothermia [Dietrich, 2009]. A recent retrospective review of 14 patients with acute complete cervical cord injuries treated with modest hypothermia (33°C), using an intravascular cooling catheter, showed that, while most of the patients developed respiratory complications (atelectasis [n = 12], pneumonia [n = 8], acute respiratory distress syndrome [n = 2]), those numbers were no different from those found in 14 matched controls, and none of the patients developed life-threatening complications, such as deep vein thrombosis, pulmonary embolism, or myocardial infarction [Levi et al., 2009]. Until multicenter, randomized, double-blind studies can be conducted to assess comparable acute spinal cord injuries, treated with different forms of hypothermia, the true efficacy of this therapy will not be known [Dietrich, 2009].
Oscillating Field Stimulation
Neurites grow toward the negative pole (cathode) in an electrical field [Hinkle et al., 1981]. An implantable oscillating field stimulator, capable of creating an electrical field along the rostrocaudal axis of the spinal cord, was built and implanted in 10 patients with complete spinal cord injuries from C5 to T10. The stimulator was implanted within 18 days and removed 15 weeks post injury. All 10 patients in this phase I trial had also received intravenous methylprednisolone, per the NASCIS 3 protocol, and 8 of the 10 additionally had surgical decompression/stabilization of the spine. Because neurite outgrowth is stimulated only toward the negative pole, the device reverses the polarity of the applied electrical field every 15 minutes to promote axonal growth in both directions. Patients were followed for up to 1 year and all showed significant neurological improvement from baseline in motor and sensory function. (One patient was lost to follow-up after 6 months.) Complications were low. Since all patients had also received methylprednisolone therapy and most had also undergone surgical decompression, the extent of neurological recovery directly associated with the oscillating field stimulation (OFS) alone cannot be assessed. Thus, while OFS technology is fascinating, it is only with a multicenter, randomized double-blind, controlled clinical trial that it will be possible to judge its full therapeutic potential [Shapiro et al., 2005].
Targeting Myelin-Associated and Glial Scar-Associated Inhibitors of Regeneration
Molecular Mechanisms
Unlike neonatal axons, mammalian adult axons do not regenerate after injury. Myelin, a major factor in inhibiting regeneration in adult neurons, does not inhibit regeneration in younger neurons, in which a specific myelin component, myelin-associated glycoprotein (MAG), promotes axonal growth; but the same types of neurons, when older, are inhibited by MAG. Endogenous levels of the nucleotide, cyclic adenosine monophosphate (cAMP), are much higher in young neurons than in older ones. By inhibiting a downstream effector of cAMP, protein kinase A, in young neurons, MAG promotion of neurite outgrowth can be prevented, while elevating cAMP in older neurons will counter myelin-MAG inhibition of neurite outgrowth [Cai et al., 2001].
The elevation of intracellular cAMP levels using cAMP analogs or the phosphodiesterase inhibitor, rolipram, has been shown to reduce the effects of myelin-associated inhibitors and to promote axonal regeneration [Hannila and Filbin, 2008].
Myelin-Associated Inhibitors
Multiple components of central nervous system myelin that inhibit axonal growth include Nogo, MAG, OMgp, semaphorin 4D, ephrin B3, repulsive guidance molecule, and Netrin-1. A therapeutic approach that has emerged from research into myelin inhibitors is the use of anti-Nogo antibodies as a directed therapy to neutralize this myelin inhibitor. This approach provoked much interest after reports that a Nogo-A specific neutralizing antibody was found to promote sprouting and improve functional recovery of manual dexterity in primates after cervical spinal cord injury. This treatment strategy has been undergoing early human evaluation in Europe and Canada. One outstanding question regarding the use of anti-Nogo antibodies and other myelin inhibitors is the functionality of the axonal sprouting that might be induced. Along with promoting regenerative growth, such treatments can also stimulate aberrant sprouting of uninjured axons or inappropriate growth of regenerating axons. Whether this overall increase in plasticity is beneficial has yet to be determined [Rowland et al., 2008].
Glial Scar-Associated Inhibitors
The glial scar is the result of reactive astrocytosis. The astrocytes that comprise the scar secrete a number of growth inhibitory extracellular matrix components known as chondroitin sulphate proteoglycan (CSPG) [McKeon et al., 1991]. The strategy for overcoming this glial barrier is by direct degradation of the CSPGs with a bacterial enzyme known as ChABC. This approach has been evaluated in numerous in vivo animal models of spinal cord injury, and many investigators have reported increased sprouting and improved behavioral recovery after treatment with ChABC [Barritt et al., 2006; Rowland et al., 2008].
Cell Transplantation Therapies
Because limited substrate for neural repair is a significant obstacle following spinal cord injury, cell replacement strategies are believed to be among the most promising new therapies for treating spinal cord injuries. Many cell types have been tried, including neural precursor cells, olfactory ensheathing cells, bone marrow-derived stromal cells, and others. Although the goals of these strategies have differed, functional benefit has been consistently seen, although the magnitude of this benefit has been uniformly modest. Cell transplantation into injured spinal cords is being conducted in numerous centers outside of North America; here, many believe that these approaches require greater optimization and understanding before human trials are undertaken [Hawryluk et al., 2008]. Some transplantation regimens have been complicated by genesis or exacerbation of neuropathic pain [Macias et al., 2006] that is currently poorly understood. Other serious complications have been described, such as tumor-like overgrowth [Hawryluk et al., 2008].
Activated Autologous Macrophages
The strategy of transplanting activated autologous macrophages (AAM) into injured spinal cord is based on the thought that differences in the ability of axons within the central nervous system and those within the peripheral nervous system to regenerate are related to differences in the macrophage response within the two environments [Hawryluk et al., 2008]. In contrast to central nervous system macrophages, those in the peripheral nervous system are abundant, they clear myelin debris rapidly, and they secrete nerve growth factor [Perry et al., 1987].
In an initial phase 1 clinical trial of AAM transplantation for complete spinal cord injuries in Israel, 8 patients were transplanted within 14 days of injury with incubated autologous macrophages, injected into the spinal cord immediately below the level of the lesion. Three of the 8 patients showed significant motor and sensory improvement (moving from ASIA grade A status to grade C) [Knoller et al., 2005]. In 6 dogs that underwent a thoracic spinal cord hemisection, 4 were implanted at both ends of the lesion with AAMs, while 2 received sham injections. Partial electrophysiological and functional motor recovery was seen in all animals, but there was no morphological or histological evidence of axonal regeneration in any of the animals. The authors concluded that AAM implantation requires further investigation before it can be relied upon to ameliorate spinal cord injury [Assina et al., 2008].
Schwann Cells
Schwann cells, the myelinating cells of the peripheral nervous system, may represent an environment permissive of regeneration. Another potential benefit of these cells is the ability to harvest them from an autologous source, such as the sural nerve. A limitation of this technique, however, appears to be that regenerating central nervous system axons readily grow into the permissive environment that these cells provide, but they are not prone to growing into a hostile central nervous system environment [Hawryluk et al., 2008]. In a human trial, 4 patients with stable, chronic, midthoracic spinal cord injuries were treated with autologous Schwann cell transplants. One year later, none of the patients had suffered any adverse effects from the transplantation; 1 of the 4 showed motor and sensory improvement but she had also received intensive and continuous rehabilitation [Saberi et al., 2008].
Olfactory Ensheathing Cells
Olfactory ensheathing cells (OECs), specialized glia of the olfactory system, escort regenerating axons of olfactory receptor neurons from the peripheral nervous system of the nasal epithelium to the “hostile” central nervous system of the olfactory bulb [Hawryluk et al., 2008]. In a study of adult rats rendered paraplegic from complete spinal cord transection and transplanted with OECs, all recovered locomotor functions and sensorimotor reflexes in 3–7 months post surgery, accompanied by regeneration for long distances of relevant motor axons [Ramón-Cueto et al., 2000].
In a single-blind, phase I clinical trial, three adult men with chronic, complete thoracic spinal cord injuries were injected with autologous OECs into the areas of damaged spinal cord; a matched injury group served as controls. One year after transplantation, there were no complications and there was no deterioration in neurological status [Féron et al., 2005]. At 3 years follow-up, there still had been no complication in the patients who had received OEC transplants, including an absence of neuropathic pain. There were, however, no significant functional changes found in any of the patients [Mackay-Sim et al., 2008].
Bone Marrow Stromal Cells
Surprisingly, bone marrow stromal cells (BMSCs) are able to migrate slowly to the site of a central nervous system injury following intravascular or intrathecal administration. Importantly, they also can be transplanted in an autologous fashion, like Schwann cells and OECs [Hawryluk et al., 2008].
In Korea, a phase I/II open-label, nonrandomized study was conducted on 35 patients with complete spinal cord injuries. Following decompressive surgery, all received autologous BMSC transplants, and a granulocytic macrophage-colony stimulating factor (GM-CSF), injected into the areas surrounding the spinal cord injuries. The transplants were given acutely, within 14 days of injury (n = 17); subacutely, between 2 and 8 weeks after injury (n = 6); or chronically, more than 8 weeks after injury (n = 12). Mean follow-up was 10.4 months. The BMSC transplantation and GM-CSF administration were not associated with any serious side effects, and the ASIA grade increased in 30 percent of the acutely and subacutely treated patients (ASIA grade A to B or C); no significant improvement was seen in the chronic treatment group [Yoon et al., 2007].
In Prague, 20 patients with complete spinal cord injuries underwent intra-arterial or intravenous transplantation with autologous bone marrow 10–467 days post injury. There were two groups: acute (treated 10–30 days after injury; n = 7) and chronic (treated 2–17 months post injury; n = 13). Improvement in motor and/or sensory functions was seen in 5 of 6 patients with intra-arterial injections, 5 of 7 patients treated acutely, and 1 of 13 chronic patients. In 11 patients followed for more than 2 years, no complications were seen. The authors concluded that transplantation within a therapeutic window of 3–4 weeks following injury will play an important role in any type of stem-cell treatment of spinal cord injury [Syková et al., 2006]. Similar transplant studies have been conducted in China, Russia, and Brazil [Tator, 2006].
Human Embryonic Stem Cells
Transplantation of human embryonic stem cell (hESC)-derived oligodendrocyte progenitor cells (OPCs) into adult rat spinal cord injuries was shown to enhance remyelination and to improve locomotor ability substantially, if given 7 days after injury. By contrast, when the OPCs were transplanted 10 months after injury, there was no enhanced remyelination or locomotor recovery [Keirstead et al., 2005].
Other Investigational Therapies
A variety of substances have been studied in different animal models, most often the rat, to assess their efficacy in promoting repair following experimentally induced spinal cord injury. The results of most of these studies have been encouraging. The agents studied include: brain-derived neurotrophic factor and glial-derived neurotrophic factor [Sharma, 2006]; dynorphin A (1–17) antibodies [Sharma et al., 2006a]; antioxidant compound H-290/51 [Sharma et al., 2006b]; Lipitor [Pannu et al., 2007]; C1 esterase inhibitor [Tei et al., 2008]; activated spinal cord ependymal stem cells [Moreno-Manzano et al., 2009]; adenosine triphosphate (ATP)-sensitive receptor P2X7 antagonist [Peng et al., 2009]; magnesium [Wiseman et al., 2009]; tamoxifen [Tian et al., 2009]; and calpain inhibitor [Colak et al., 2009].
Gene Therapy for Spinal Cord Injury
The simplest and most elegant strategy is the direct gene therapy approach that uses a single injection for delivery of a gene therapy vehicle. Among the vectors used to transduce neural tissue in vivo are nonviral vectors and viral ones (herpes simplex [HSV]; retroviral [RV]; lentiviral [LV]; adenoviral [Ad]; adeno-associated viral [AAV]), each with its own merits and limitations. HSV and Ad vectors have proved to be excessively toxic, but after a decade of work, nontoxic AAV and LV vectors, suitable for neuroregenerative research, have been developed [Blits and Bunge, 2006].
Long-Term Management
Cervical Spine Immobilization
In spine and spinal cord injuries in children, the main imperatives are to decompress the neural elements and to stabilize the spine to prevent further injury [Proctor, 2002]. Cervical traction serves to immobilize the spine and reduce fractures. Reduction of fractures or subluxation must be undertaken with care in young patients because overdistraction can worsen the patient’s neurologic dysfunction. Manual positioning under fluoroscopy often is required in cases of cervical ligamentous injury. Crown halo rings can be applied in younger patients at a pressure of 2 inches/pound of torque per pin [Pang and Hanley, 1990]. In older patients, cervical traction can be used with relative safety. Many spine-injured children can be managed with external stabilization without a need for later surgery [Hadley, 1992; Proctor, 2002]. Various devices can be used, depending on the level and severity of injury, including the Philadelphia cervical collar, Yale braces, sternal occipital mandibular immobilizer (SOMI) braces, four-poster braces, halo rests, crown halo rings, and thoracolumbar orthoses. When such devices are used, frequent radiographic reevaluations are needed to ensure continuing proper alignment of spinal elements because adequate external (and internal) fixation can be difficult to achieve in a young child [Proctor, 2002]. Specific recommendations for the management of SCIWORA were discussed earlier [Gunnarsson and Fehlings, 2003].
Supportive Medical Care
For patients with midcervical or upper cervical spinal cord injuries, there is a high risk of respiratory failure. Because the lower motor neurons for the phrenic nerve are at cervical levels 3 through 5, patients with severe spinal cord injuries above this level require permanent mechanical ventilation. Although patients with mid cervical spinal cord injuries initially may have adequate spontaneous ventilation, their ventilatory ability may deteriorate within a few days consequent to ascending spinal cord edema [Marion, 1998]. Children with high cervical lesions who are ventilator-dependent may be candidates for phrenic nerve pacing [Massagli, 2000].
In addition to causing respiratory difficulties, spinal cord trauma can result in a variety of other medical problems requiring on-going care [Luce, 1985]. Cardiovascular function can be altered by the trauma and its acute management. In one study, 4 of 9 patients developed pulmonary edema after spinal cord injury, probably from overhydration during acute resuscitation [Meyer et al., 1971]. Patients with spinal cord lesions above T7 requiring long-term management have decreased cardiac output, hypotension, and problems with temperature regulation, limiting adaption to exercise. Nasogastric tube feeding after restoration of normal intestinal activity, antacids, hyperalimentation when bowel atonia persists, and digital stimulation, especially in young children, may be needed [Goetz et al., 1998]. In some children, gentle disimpaction is indicated. The use of enema continence catheters for bowel dysfunction after spinal cord trauma may significantly facilitate bowel care in such patients [Liptak and Revell, 1992]. Intermittent catheterization of the bladder is preferred to placement of an indwelling catheter to reduce the risk of urinary tract infection and renal failure [Luce, 1985; Nygaard and Kreder, 1996; Pannek et al., 1997].
Renal failure is a major cause of mortality in patients with chronic spinal cord injury [Hackler, 1984]. Anticholinergic and botulinum toxin therapy can reduce the effects of detrusor hyperreflexia (neurogenic bladder), present in approximately 60 percent of patients rendered quadriparetic from spinal cord injuries [Curt et al., 1997; Schurch et al., 1997]. Hyperhidrosis, fever, and disrupted temperature regulation are additional autonomic dysfunctions frequently encountered in patients with spinal cord injury. Anticholinergic treatment may help [Canaday and Stanford, 1995].
Autonomic dysreflexia (AD) is a well-known clinical emergency in subjects who have had a spinal cord injury, especially at T6 or above. It is characterized by acute elevation of arterial blood pressure and bradycardia, though tachycardia can also occur. Accompanying symptoms include severe headache, blurred vision, feeling of anxiety, profuse sweating, flushing and piloerection above the level of injury, and dry and pale skin below the injury. The higher the injury level, the more severe is the AD. Untreated episodes can have serious consequences, including intracranial hemorrhage, retinal detachment, seizures, and death. Physiologically, AD is caused by a massive sympathetic discharge, triggered by either a noxious or non-noxious stimulus, originating below the level of the spinal cord injury. The more complete the spinal injury, the more likely is AD to occur. The most frequent triggers are irritation of the urinary bladder or colon. Symptoms usually are short-lived, although there have been reports of AD sustained for days to weeks. It is usually evident a month or more post injury, although cases have been reported after 1 week. Management of an episode of AD includes placing the patient upright, loosening any tight clothing, eliminating any precipitating stimulus (bladder distention or bowel impaction in 85 percent of cases) and antihypertensive drugs in the presence of sustained hypertension [Krassioukov et al., 2009].
Other general measures include chest physiotherapy to prevent pneumonia, frequent repositioning of the patient and the use of spinal beds (Stryker frame RotaBed) to avoid decubiti, and the use of Jobst stockings to minimize the risk of deep vein thromboses and the attendant potential for pulmonary emboli. Occasionally, ventilation-perfusion scanning and pulmonary angiography are needed to establish the diagnosis of pulmonary emboli. It is estimated that one-third of the deaths within the first weeks of spinal cord injury are due to pulmonary embolism. The use of mini-heparin treatment (1 μg/kg/hr, continuous infusion) or intermittent low-dose heparin therapy may reduce the risk of pulmonary embolism [Borow and Goldson, 1983]. In patients for whom anticoagulant therapy is contraindicated, placement of an inferior vena cava filter may be necessary. The healing of decubitus ulcers is aided by treatment with vitamin C (15–20 mg/kg/day) [Taylor et al., 1974]. Muscle relaxants, such as diazepam, baclofen, cyclobenzaprine, and intravenous orphenadrine citrate [Casale et al., 1995], may be helpful in the long-term management of spasticity after spinal cord injury. Additional antispasmodic treatments include intrathecal baclofen and tizanidine [Abel and Smith, 1994; Lewis and Mueller, 1993; Nance et al., 1994]. Progressive spinal deformity is common in children after spinal cord injury, particularly after laminectomy [Lonstein, 1995]. Chronic pain is also common, and its management can be difficult, necessitating intervention by pain management specialists [Chiou-Tan et al., 1996; Drewes et al., 1994; Siddall et al., 1995; Yezierski, 1996]. In patients in whom there is delayed deterioration of neurological functioning, the presence of a syrinx should be sought; if one is found, the syrinx may need to be shunted [Proctor, 2002].
Physical Therapy
Physical therapy is an important component in the long-term care of all patients with spinal cord injury [Jacobs and Nash, 2004]. Physical therapy should begin early and continue indefinitely. Measures such as proper limb positioning and passive muscle stretching can be instituted when the patient is medically and surgically stable. Later, after spinal shock has resolved and early spasticity is evident, inhibitive casting can be used to minimize the development of limb contractures. Despite such measures, many patients later require phenol blocks, botulinum toxin injections, tendon lengthenings, and tendon transfers. In the 1970s and 1980s, functional electrical stimulation (FES) raised hopes in many plegic patients, with apparent efficacy in some children and adolescents rendered paraplegic after a spinal cord injury. Electrodes were placed in 9 such patients to provide constant stimulation to their hip and knee extensors and hip abductors and adductors; the 7 who were able to complete their training all gained increased functional abilities over traditional long leg braces, and a decreased need for physical assistance by a caregiver [Johnston et al., 2003]. FES has been used to augment function of the lower limbs, upper limbs, bladder, bowel, respiration, and erection/ejaculation [Creasey et al., 2004; Kirshblum, 2004]. Short-term stimulation can be provided by electrodes on the skin or by percutaneous fine wires, but implanted systems are preferable for long-term use [Creasey et al., 2004]. FES-assisted stepping can be triggered automatically at a fixed rate (autotrigger), by a manual switch (switch-trigger), or by an electromyogram-based gait event-detector (EMG-trigger) [Dutta et al., 2009].
Despite spectacular videos in selected patients, FES has not yet fulfilled expectations with respect to achievable gait function. The best paraplegic patients have covered a maximum uninterrupted gait distance of up to 500 meters at a velocity of approximately 20 meters per min [Hesse and Werner, 2009]. Further, there is an absence of randomized clinical trials to assess the true efficacy of FES in improvement of walking in spinal cord injury patients.
In the early 1990s, treadmill training with partial body-weight support opened new perspectives for patients with incomplete spinal cord injuries – the harness substituted for deficient equilibrium reflexes, the body weight reduction compensated for the paresis, and the moving treadmill enforced locomotion. Numerous open studies have suggested efficacy of body-weight-supported treadmill training in improving gait ability and function in spinal cord injury patients [Hesse and Werner, 2009]. A recent controlled trial in 146 patients with acute incomplete spinal cord injuries, however, failed to show any superiority of manually assisted treadmill training when compared to repetitive gait training on the floor assisted by early use of technical aids [Dobkin et al., 2006].
FES and gait training have often been used together. Twenty-seven subjects with chronic incomplete spinal cord injuries received 12 weeks of body-weight support training, with and without FES, on the treadmill and during over-ground walking. Walking speed was measured before and after training; those subjects with initial slower speed increased their speed in 85 percent of cases, whereas those with initial fast speed showed an increase in only 9 percent of cases [Ditunno and Scivoletto, 2009].
Both human and animal studies show that the spinal cord has the potential to reorganize and/or readjust to the loss of supraspinal input and to utilize the remaining peripheral input to control stepping and standing. Motor training can be used to provide sensory ensembles within the spinal circuitry that are task-specific, i.e., step training improves stepping, and stand training improves standing. The use of robotics for training specific motor tasks has become more prevalent recently. Using an “assist-as-needed” approach for step training after a severe spinal cord injury provides a high probability of successful rehabilitation, enhancing the ability of spinal cord-injured individuals to regain the maximum locomotor ability possible [Edgerton and Roy, 2009].
Psychological Therapy
Counseling should be provided, beginning early in the postinjury period and continuing throughout all stages of rehabilitation, to provide maximal, on-going support for the child and family [Geller and Greydanus, 1979; Talbot, 1971]. Health professionals from many disciplines can assist in providing such support.
Prognosis
The most widely used rating scale to assess the severity of spinal cord injury and to quantify spinal cord function during recovery is the ASIA/IMSOP scale, a modification of the older Frankel scale. Other rating scales that have been used include the University of Miami Neurospinal Index and the Barthel Index [Klose et al., 1980; Mahoney and Barthel, 1965]. The disadvantage of such scoring systems is the difficulty in translating numerical scores into descriptions of clinical capability or activities of daily living [Walker, 1991]. A variety of functional scales have been used to measure changes that correlate highly with activity level and lifestyle [Geisler et al., 1991; Piepmeier and Collins, 1992].
Despite modern intensive care management and advances in therapy, mortality from spinal cord injury remains high, averaging 10–15 percent, with higher mortalities in patients rendered quadriplegic [Bracken et al., 1981; Ducker et al., 1983; Krause et al., 1997; Michaelis, 1976]. Two-year survival rates are better in younger patients (16–30 years old) than in older ones (61–86 years old), 95 percent versus 59 percent [DeVivo et al., 1990]. Overall morbidity (gastrointestinal hemorrhage, pulmonary emboli, and renal stones) also is lower in younger age groups. Limited recovery of neurological function is possible after complete spinal cord injury, especially when there has been some improvement within the first 24 hours [Chilton and Dagi, 1985]. Nonetheless, approximately 70–95 percent of patients with complete lesions exhibit no improvement on long-term follow-up. Patients with complete injury persisting for longer than 1 week usually do not recover neurological function, although some improvement may occur if there is a zone of partial preservation [Ditunno, 1996]. Recovery from thoracolumbar lesions is better than with higher lesions [Patel et al., 2001]. Patients with partial spinal cord lesions can recover 25 percent of their power, and in patients with central cord lesions, recovery of power can be 80 percent [Ducker et al., 1983]. Additionally, patients with complete paralysis but with preserved sensation (ASIA/IMSOP grade B) have substantially greater improvement than patients with complete loss of motor and sensory function (ASIA/IMSOP grade A) [Landi and Ciccone, 1992].
Age at time of injury also influences recovery from spinal cord trauma. In one study, 90 percent of younger patients (<50 years old) with incomplete injury and preserved motor function (but with a muscle grade <3; ASIA/IMSOP grade C) became ambulatory, compared with 42 percent of older patients. All patients with incomplete ASIA/IMSOP grade D lesions (muscle grade ≥3) developed independent ambulation [Burns et al., 1997]. Similar poor outcome in older patients with complete and incomplete spinal cord injury was noted in another study, in which only 14 percent of complete injury and 50 percent of incomplete injury patients survived beyond 1 year [Alander et al., 1997]. Recovery from spinal injury is not only better in children than in adults, but also in children improvement can continue for many months after the injury [Wang et al., 2004].
MRI has been more helpful than electrophysiological testing in assisting prediction of outcome after spinal cord injury. MRI can distinguish intramedullary hematomas (poorer prognosis) from spinal cord edema (better prognosis). Patients in whom the edema is restricted to a single spinal segment or less have the best prognosis [Schaefer et al., 1992]. The clinical and prognostic value of emergency MRI was studied in 55 patients with acute cervical spinal cord injuries. Although the best single predictor of long-term improvement in neurological function was the initial neurological examination, four MRI characteristics provided additional prognostic information – the presence of intra-axial hematoma; the sagittal length of spinal cord hematoma; the extent of spinal cord edema; and spinal cord compression by extra-axial hematoma [Selden et al., 1999]. Conclusions about the usefulness of different laboratory investigations in aiding the prediction of neurological outcome must be viewed with some circumspection, however, because in most series relatively few patients of different ages have been studied.
Prevention
Spinal cord injuries are devastating at any age, but are especially tragic and expensive in children. Preventing these injuries is probably the greatest challenge in dealing with spinal cord injuries. Continued investment in educational programs, with on-going efforts to heighten public awareness of the risks and consequences of spinal cord injuries, is crucial. Highway driving speed limits should be strictly enforced. People who drink alcohol should not drive. Risk-taking behavior must be minimized, particularly in people taking sedating medications or illicit drugs. The need for protective helmets in sports such as skiing, snowboarding, and bicycling cannot be overstressed. Diving into unfamiliar waters must be avoided. Certain sports, particularly tackle football, carry with them a substantial risk of spinal injury, and this needs to be recognized. Appropriate seat-belt designs, proper use of child restraints, and positioning small children in the rear seats of automobiles with air bags save lives and neurologic function. Further, any efforts that can result in reduction of societal violence, particularly involving guns and knives, can be expected to result in a corresponding reduction in spinal cord injuries [Ackery et al., 2004; Rekate et al., 1999]. Finally, faced with a patient who has just suffered an acute spinal cord injury, one should promptly employ any potentially useful strategies [Bauchet et al., 2009]. A valuable resource for parents is the website of the ASIA at http://www.asia-spinalinjury.org/home/index.html.
References
The complete list of references for this chapter is available online at www.expertconsult.com.
Abel N.A., Smith R.A. Intrathecal baclofen for treatment of intractable spinal spasticity. Arch Phys Med Rehabil. 1994;75:54.
Ackery A., Tator C., Krassioukov A. A global perspective on spinal cord injury epidemiology. J Neurotrauma. 2004;21:1355.
Akhtar A.Z., Pippin J.J., Sandusky C.B. Animal studies in spinal cord injury: a systematic review of methylprednisolone. Altern Lab Anim. 2009;37:43.
Alander D.H., Parker J., Stauffer E.S. Intermediate-term outcome of cervical spinal cord-injured patients older than 50 years of age. Spine. 1997;22:1189.
Alker G.J., Oh Y.S., Leslie E.V., et al. Postmortem radiology of head and neck injuries in fatal traffic accidents. Radiology. 1975;14:611.
Allen J.P., Myers G.G., Condon V.R. Laceration of the spinal cord related to breech delivery. JAMA. 1969;208:1019.
American Spinal Injury Association, International Medical Society of Paraplegia (ASIA/IMSOP). International standards for neurological and functional classification of spinal cord injury. Revised. 1992.
Andersen B.J., Stringer W.A. Imaging after spinal injury. In: Narayan R.K., Wilberger J.E., Povlishock J.T., editors. Neurotrauma. New York: McGraw-Hill, 1996.
Anderson J.M., Shutt A.H. Spinal injury in children: A review of 156 cases seen from 1950 through 1978. Mayo Clin Proc. 1980;55:499.
Anderson L.D., D’Alonzo R.T. Fractures of the odontoid process of the axis. J Bone Joint Surg Am. 1974;56:1663.
Apple D.F., Anson C.A., Hunter J.D., et al. Spinal cord injury in youth. Clin Pediatr. 1995;34:90.
Assina R., Sankar T., Theodore N., et al. Activated autologous macrophage implantation in a large-animal model of spinal cord injury. Neurosurg Focus. 2008;25:1.
Babcock J.L. Spinal injuries in children. Pediatr Clin North Am. 1975;22:487.
Bailes J.E., Hadley M.N., Quigley M.R., et al. Management of athletic injuries of the cervical spine and spinal cord. Neurosurgery. 1991;29:491.
Baker A.S., Ojemann R.G., Swartz M.N., et al. Spinal epidural abscess. N Engl J Med. 1975;293:463.
Ball P.A., Nockels R.P. Summary statement: Nonoperative management and critical care of acute spinal cord injury. Spine. 2001;26:S38.
Barnett H.J.M., Jousse A.T.. Posttraumatic syringomyelia (cystic myelopathy). Vinken P.J., Bruyn C.H., Braakman R., editors. Injuries of the spine and spinal cord, II. Handbook of clinical neurology. Amsterdam: North Holland Publishing Company; 1976;26.
Barritt A.W., Davies M., Marchand F., et al. Chondroitinase ABC promotes sprouting of intact and injured spinal systems after spinal cord injury. J Neurosci. 2006;26:10856.
Baskin D.S. Spinal cord injury. In: Evans R.W., editor. Neurology and trauma. Philadelphia: WB Saunders, 1996.
Baskin D.S., Simpson R.K.Jr, Browning J.L., et al. The effect of long-term high-dose naloxone infusion in experimental blunt spinal cord injury. J Spinal Disord. 1993;6:38.
Batnitzky S., Keucher T.R., Mealey J.Jr, et al. Iatrogenic intraspinal epidermoid tumors. JAMA. 1977;237:148.
Bauchet L., Lonjon N., Perrin F.-E., et al. Strategies for spinal cord repair after injury: A review of the literature and information. Ann Phys Rehabil Med. 2009;52:330.
Bell H.S. Paralysis of both arms from injury of the upper portion of the pyramidal decussation: “cruciate paralysis”. J Neurosurg. 1970;33:376.
Benzel E.C., Doezema D. Prehospital management of the spinally injured patient. In: Narayan R.K., Wilberger J.E., Povlishock J.T., editors. Neurotrauma. New York: McGraw-Hill, 1996.
Blits B., Bunge M.B. Direct gene therapy for repair of the spinal cord. J Neurotrauma. 2006;23:508.
Bondurant F.J., Cotler H.B., Kulkarni M.V., et al. Acute spinal cord injury: A study using physical examination and magnetic resonance imaging. Spine. 1990;15:161.
Borow M., Goldson H.J. Prevention of postoperative deep venous thrombosis and pulmonary emboli with combined modalities. Am Surg. 1983;49:599.
Braakman R., Penning L.. Injuries of the cervical spine. Vinken P.J., Bruyn G.W., editors. Handbook of clinical neurology, 25. Amsterdam: North Holland, 1976.
Bracken M.B., Collins W.F., Freeman D.F., et al. Efficacy of methylprednisolone in acute spinal cord injury. JAMA. 1984;251:45.
Bracken M.B., Freeman D.H., Hellenbrand K. Incidence of acute traumatic hospitalized spinal cord injury in the United States, 1970–1977. Am J Epidemiol. 1981;113:615.
Bracken M.B., Holford T.R. Effects of timing of methylprednisolone or naloxone administration on recovery of segmental and long-tract neurological function in NASCIS 2. J Neurosurg. 1993;79:500.
Bracken M.B., Shepard M.J., Collins W.F., et al. A randomized, controlled trial of methylprednisolone or naloxone in the treatment of acute spinal-cord injury: Results of the second national acute spinal cord injury study. N Engl J Med. 1990;322:1405.
Bracken M.B., Shepard M.J., Collins W.F., et al. Methylprednisolone or naloxone treatment after acute spinal cord injury: 1-Year follow-up data – results of the Second National Acute Spinal Cord Injury Study. J Neurosurg. 1992;76:23.
Bracken M.B., Shepard M.J., Holford T.R., et al. Administration of methylprednisolone for 24 or 48 hours or tirilazad mesylate for 48 hours in the treatment of acute spinal cord injury: Results of the Third National Spinal Cord Injury Randomized Control Trial. National Acute Spinal Cord Injury Study. JAMA. 1997;277:1597.
Bracken M.B., Shepard M.J., Holford T.R., et al. Methylprednisolone or tirilazad mesylate administration after acute spinal cord injury: 1-Year follow up. J Neurosurg. 1998;89:699.
Bramlett H.M., Dietrich W.D.. Progressive damage after brain and spinal cord injury: pathomechanisms and treatment strategies. Weber, Maas. Progress In Brain Research. Elsevier; 2007;161:125.
Bresnan M.J., Abroms I.F. Neonatal spinal cord transection secondary to hyperextension of the neck in breech presentation. J Pediatr. 1974;84:734.
Browder J., Meyers R. Pyogenic infections of the spinal epidural space. Surgery. 1941;10:296.
Bruyn G.W., Bosma M.J.. Spinal extradural haematoma. Vinken P.J., Bruyn G.W., editors. Handbook of clinical neurology, 25. Amsterdam: North Holland, 1976.
Burke D.C.. Injuries of the spinal cord in children. Vinken P.J., Bruyn G.W., editors. Handbook of clinical neurology, 25. Amsterdam: North Holland, 1976.
Burke D.C. Traumatic spinal paralysis in children. Paraplegia. 1974;11:268.
Burns S.P., Golding D.G., Rolle W.A.Jr, et al. Recovery of ambulation in motor-incomplete tetraplegia. Arch Phys Med Rehabil. 1997;78:1169.
Byers R.K. Spinal-cord injuries during birth. Dev Med Child Neurol. 1975;17:103.
Caffey J. The whiplash shaken infant syndrome. Pediatrics. 1974;54:396.
Cai D., Qiu J., Cao Z., et al. Neuronal cyclic AMP controls the developmental loss in ability of axons to regenerate. J Neurosci. 2001;21:4731.
Canaday B.R., Stanford R.H. Propantheline bromide in the management of hyperhidrosis associated with spinal cord injury. Ann Pharmacother. 1995;29:489.
Casale R., Glynn C.J., Buonocore M. Reduction of spastic hypertonia in patients with spinal cord injury: A double-blind comparison of intravenous orphenadrine citrate and placebo. Arch Phys Med Rehabil. 1995;76:660.
Cattell H.S., Filtzer D.L. Pseudosubluxation and other normal variations in the cervical spine in children. J Bone Joint Surg Am. 1965;47:1295.
Caviness A.C.. Evaluation of cervical spine injuries in children and adolescents. 2004. UpToDate, Version 12.3
Chilton J., Dagi T.F. Acute cervical spinal cord injury. Am J Emerg Med. 1985;3:340.
Chiou-Tan F.Y., Tuel S.M., Johnson J.C., et al. Effect of mexiletin on spinal cord injury dysesthetic pain. Am J Phys Med Rehabil. 1996;75:84.
Colak A., Kaya M., Karaoğlan A., et al. Calpain inhibitor AK 295 inhibits calpain-induced apoptosis and improves neurologic function after traumatic spinal cord injury in rats. Neurocirugia (Astur). 2009;20:245.
Creasey G.H., Ho C.H., Triolo R.J., et al. Clinical applications of electrical stimulation after spinal cord injury. J Spinal Cord Med. 2004;27:365.
Crothers B. Injury of the spinal cord in breech extraction as an important cause of fetal death and of paraplegia in childhood. Am J Med Sci. 1923;165:94.
Curt A., Dietz V. Electrophysiological recordings in patients witjh spinal cord injury: Significance for predicting outcome. Spinal Cord. 1999;37:157.
Curt A., Nitsche B., Rodic B., et al. Assessment of autonomic dysreflexia in patients with spinal cord injury. J Neurol Neurosurg Psychiatry. 1997;62:473.
Davis P.C., Reisner A., Hudgins P.A., et al. Spinal injuries in children: Role of MR. Am J Neuroradiol. 1993;14:607.
Decker J.E., Hergenroeder A.C.. Overview of cervical spinal cord and cervical peripheral nerve injuries in the young athlete. 2004. UpToDate, Version 12.3
DeLaTorre J.C. Spinal cord injury: Review of basic and applied research. Spine. 1981;6:315.
DeMyer W.. Anatomy and clinical neurology of the spinal cord. Baker A.B., Joynt R.J., editors. Clinical neurology, 3. Philadelphia: Harper & Row, 1985.
DeVivo M.J., Kurtus P.L., Rutt R.D., et al. The influence of age at time of spinal cord injury on rehabilitation outcome. Arch Neurol. 1990;47:687.
Dias M.S. Traumatic brain and spinal cord injury. Pediatr Clin North Am. 2004;51:271.
Dietrich W.D. Therapeutic hypothermia for spinal cord injury. Crit Care Med. 2009;37:S238.
Ditunno J.F. Rehabilitation assessment and management in the acute spinal cord injury (SCI) phase. In: Narayan R.K., Wilberger J.E., Povlishock J.T., editors. Neurotrauma. New York: McGraw-Hill, 1996.
Ditunno J.F., Little J.W., Tessler A., et al. Spinal shock revisited: A four-phase model. Spinal Cord. 2004;42:383.
Ditunno J., Scivoletto G. Clinical relevance of gait research applied to clinical trials in spinal cord injury. Brain Res Bull. 2009;78:35.
Dobkin B., Apple D., Barbeau H., et al. Weight-supported treadmill vs over-ground training for walking after acute incomplete SCI. Neurology. 2006;66:484.
Donahue D.J., Muhlbauer M.S., Kaufman R.A., et al. Childhood survival of atlantooccipital dislocation: Underdiagnosis, recognition, treatment, and review of the literature. Pediatr Neurosurg. 1994;21:105.
Drewes A.M., Andreasen A., Poulsen L.H. Valproate for treatment of chronic central pain after spinal cord injury: A double-blind cross-over study. Paraplegia. 1994;32:565.
Ducker T.B., Hamit H.F. Experimental treatments of acute spinal cord injury. J Neurosurg. 1969;30:693.
Ducker T.B., Lucas J.T., Wallace C.A. Recovery from spinal cord injury. Clin Neurosurg. 1983;30:495.
Duh M., Shepard M.J., Wilberger J.E., et al. The effectiveness of surgery on the treatment of acute spinal cord injury and its relation to pharmacological treatment. Neurosurgery. 1994;35:240.
Dumont R.J., Okonkwo D.O., Verma S., et al. Acute spinal cord injury, Part I: Pathophysiologic mechanisms. Clin Neuropharmacol. 2001;24:254.
Dumont R.J., Verma S., Okonkwo D.O., et al. Acute spinal cord injury, Part II: Contemporary phamacotherapy. Clin Neuropharmacol. 2001;24:265.
Dutta A., Kobetic R., Triolo R.J. Gait initiation with electromyographically triggered electrical stimulation in people with partial paralysis. J Biomech Eng. 2009;131(8):081002.
Edelson R.N.. Spinal subdural hematoma. Vinken P.J., Bruyn G.W., editors. Handbook of clinical neurology, 26. Amsterdam: North Holland, 1976.
Edelson R.N., Chernik N.L., Posner J.D. Spinal subdural hematomas complicating lumbar puncture. Arch Neurol. 1974;31:134.
Edgerton V.R., Roy R.R. Robotic training and spinal cord plasticity. Brain Res Bull. 2009;78:4.
Elsberg C.A., Dyke C.G., Brewer E.D. The symptoms and diagnosis of extradural cysts. Bull Neurol Inst N Y. 1934;3:39.
Faden A.I. Pharmacological treatment approaches for brain and spinal cord trauma. In: Narayan R.K., Wilberger J.E., Povlishock J.T., editors. Neurotrauma. New York: McGraw-Hill, 1996.
Fehlings M.G., Perrin R.G. The role and timing of early decompression for cervical spinal cord injury: Update with a review of recent clinical evidence. Injury. 2005;36:S-B13.
Féron F., Perry C., Cochrane J., et al. Autologous olfactory ensheathing cell transplantation in human spinal cord injury. Brain. 2005;128:2951.
Flanders A.E., Schaefer D.M., Doan H.T., et al. Acute cervical spine trauma: Correlation of MR imaging findings with degree of neurologic deficit. Radiology. 1990;177:25.
Flanders A.E., Spettell C.M., Friedman D.P., et al. The relationship between the functional abilities of patients with cervical spinal cord injury and the severity of damage revealed by MRI imaging. Am J Neuroradiol. 1999;20:926.
Forsyth H.F., Alexander E., Underal R. The advantages of early spine fusion in the treatment of fracture-dislocation of the cervical spine. J Bone Joint Surg. 1959;41:17.
Francel P.C., Long B.A., Malik J.M., et al. Limiting ischemic spinal cord injury using a free radical scavenger 21-aminosteroid and/or cerebrospinal fluid drainage. J Neurosurg. 1993;79:742.
Francisco J.T. Smothering in infancy: Its relationship to the “crib death syndrome”. South Med J. 1970;63:1110.
Frankel H.L., Hancock D.O., Hyslop G., et al. The value of postural reduction in the initial management of closed injuries of the spine with paraplegia and tetraplegia. Paraplegia. 1969;7:179.
Galandiuk S., Raque G., Appel S., et al. The two-edged sword of large-dose steroids for spinal cord trauma. Ann Surg. 1993;218:419.
Geisler F.H., Coleman W.P., Grieco G., et al. The Sygen Study Group: The GM1 ganglioside multicenter acute spinal cord injury study. Spine. 2001;26(Suppl 24):S87.
Geisler F.H., Dorsey F.C., Coleman W.P. Recovery of motor function after spinal-cord injury – a randomized, placebo-controlled trial with GM-1 ganglioside. N Engl J Med. 1991;324:1829.
Geller B., Greydanus D.E. Psychological management of acute paraplegia in adolescence. Pediatrics. 1979;63:562.
Gibson C.J. An overview of spinal cord injury. Phys Med Rehabil Clin N Am. 1992;3:699.
Gibson T., Norris W. Skin fragments removed by injection needles. Lancet. 1958;2:983.
Gilles F.H., Bina M., Sotrel A. Infantile atlanto-occipital instability. Am J Dis Child. 1979;133:30.
Glasser R.S., Fessler R.G. Biomechanics of cervical spine trauma. In: Narayan R.K., Wilberger J.E., Povlishock J.T., editors. Neurotrauma. New York: McGraw-Hill, 1996.
Goetz L.L., Hurvitz E.A., Nelson V.S., et al. Bowel management in children and adolescents with spinal cord injury. J Spinal Cord Med. 1998;21:335.
Goldberg A.L., Daffner R.H., Schapiro R.L. Imaging of acute spinal trauma: An evolving multi-modality approach. Clin Imaging. 1990;14:11.
Goldberg A.L., Rothfus W.E., Deeb Z.L., et al. The impact of magnetic resonance on the diagnostic evaluation of acute cervicothoracic spinal trauma. Skeletal Radiol. 1988;17:89.
Gore P.A., Chang S., Theodore N. Cervical spine injuries in children: Attention to radiographic differences and stability compared to those in the adult patient. Semin Pediatr Neurol. 2009;16:42.
Gorio A., Gokmen N., Erbayraktar S., et al. Recombinant human erythropoietin counteracts secondary injury and markedly enhances neurological recovery from experimental spinal cord trauma. PNAS. 2002;99:9450.
Green B.A., Eismont F.J. Acute spinal cord injury: A systems approach. Cent Nerv Syst Trauma. 1984;1:173.
Greenberg J.O. Neuroimaging of the spinal cord. Neurol Clin North Am. 1991;9:679.
Gunby P. New focus on spinal cord injury. JAMA. 1981;245:1201.
Gunnarsson T., Fehlings M.G. Acute neurosurgical management of traumatic brain injury and spinal cord injury. Curr Opin Neurol. 2003;16:717.
Guttmann L. Early management of the paraplegic: Symposium on spinal injuries. J Roy Coll Surg. 1963;43:133.
Guttmann L.. Spinal shock. Vinken P.J., Bruyn G.W., editors. Handbook of clinical neurology, 26. Amsterdam: North Holland, 1976.
Hackler R.H. Urologic care of the spinal cord injured patient. AUA Update Series. 1984;3:1.
Hadley M.N. Pediatric spine injuries. In: Camins M.B., O’Leary P.F., editors. Disorders of the cervical spine. Baltimore: Williams & Wilkins, 1992.
Hadley M.N. Pharmacological therapy after acute cervical spinal cord injury. Neurosurgery. 2002;50:S63.
Hadley M.N. Management of pediatric cervical spine and spinal cord injuries. Neurosurgery. 2002;50:S85.
Hadley M.N., Zabramski J.M., Browner C.M., et al. Pediatric spinal trauma: Review of 122 cases of spinal cord and vertebral column injuries. J Neurosurg. 1988;68:18.
Hamilton M.G., Myles S.T. Pediatric spinal injury: A review of sixty-one deaths. J Neurosurg. 1992;77:705.
Hancock D.O.. Spinal extradural abscess. Vinken P.J., Bruyn G.W., editors. Handbook of clinical neurology, 26. Amsterdam: North Holland, 1976.
Hannila S.S., Filbin M.T. The role of cyclic AMP signaling in promoting axonal regeneration after spinal cord injury. Exp Neurol. 2008;209:321.
Hansebout R.R. A comprehensive review of methods of improving cord recovery after acute spinal cord injury. In: Tator C.H., editor. Early management of acute spinal cord injury. New York: Raven Press, 1982.
Harrop J.S., Sharan A.D., Vaccaro A.R., et al. The cause of neurologic deterioration after acute cervical spinal cord injury. Spine. 2001;26:340.
Hauswald M., Braude D. Spinal immobilization in trauma patients: is it really necessary? Curr Opin Crit Care. 2002;8:566.
Hawryluk G.W.J., Rowland J., Kwon B.K., et al. Protection and repair of the injured spinal cord: a review of completed, ongoing, and planned clinical trials for acute spinal cord injury. Neurosurg Focus. 2008;25:1.
Hayes K.C., Potter P.J., Hsieh J.T., et al. Pharmacokinetics and safety of multiple oral doses of sustained-release 4-aminopyridine (Fampridine-SR) in subjects with chronic, incomplete spinal cord injury. Arch Phys Med Rehabil. 2004;85:29.
Hehman K., Norrell H. Massive chronic spinal epidural hematoma in a child. Am J Dis Child. 1968;116:308.
Herkowitz H.N., Samberg L.C. Vertebral column injuries associated with tobogganing. J Trauma. 1978;18:806.
Herren R.Y., Alexander L. Sulcal and intrinsic blood vessels of human spinal cord. Arch Neurol Psychiatry. 1939;41:678.
Herskowitz J., Bielawski M.A., Venna N., et al. Anterior cervical arachnoid cyst simulating syringomyelia. Arch Neurol. 1978;35:57.
Hesse S., Werner C. Connecting research to the needs of patients and clinicians. Brain Res Bull. 2009;78:26.
Heusner A.P. Non-tuberculous spinal epidural infections. N Engl J Med. 1948;239:845.
Hill S.A., Miller C.A., Kosnik E.J., et al. Pediatric neck injuries: A clinical study. J Neurosurg. 1984;60:700.
Hinkle L., McCaig C.D., Robinson K.R. The direction of growth of differentiating neurones and myoblasts from frog embryos in an applied electric field. J Physiol. 1981;314:121.
Horowitz S.H., Patel N. Peripheral neurophysiology of acute distal spinal cord infarction. Pediatr Neurol. 2003;28:64.
Hoy G.A., Cole W.G. The paediatric cervical seat belt syndrome. Injury. 1993;24:297.
Hyman R.A., Gorey M.T. Imaging strategies for MR of the spine. Radiol Clin North Am. 1988;26:505.
Ito Y., Sugimoto Y., Tomioka M., et al. Does high dose methylprednisolone sodium succinate really improve neurological status in patients with acute cervical cord injury? Spine. 2009;34:2121.
Jacobs P.L., Nash M.S. Exercise recommendations for individuals with spinal cord injury. Sports Med. 2004;34:727.
Jain N., Catania K.C., Kaas J.H. Deactivation and reactivation of somatosensory cortex after dorsal spinal cord injury. Nature. 1997;386:495.
Janes J.M., Hooshmand H. Severe extension-flexion injuries of the cervical spine. Mayo Clin Proc. 1965;40:353.
Jellinger K.. Neuropathology of cord injury. Vinken P.J., Bruyn G.W., editors. Handbook of clinical neurology, 25. Amsterdam: North Holland, 1976.
Johnston T.E., Betz R.R., Smith B.T., et al. Implanted functional electrical stimulation: An alternative for standing and walking in pediatric spinal cord injury. Spinal Cord. 2003;41:144.
Joshi S.M., Hatfield R.H., Martin J., et al. Spinal epidural abscess: A diagnostic challenge. Br J Neurosurg. 2003;17:160.
Kadish H.A. Cervical spine evaluation in the pediatric trauma patient. Clin Pediatr Emerg Med. 2001;2:41.
Kakulas B.A. A review of the neuropathology of human spinal cord injury with emphasis on special features. J Spinal Cord Med. 1999;22:119.
Kakulas B.A. Neuropathology: the foundation for new treatments in spinal cord injury. Spinal Cord. 2004;42:549.
Kakulas B.A. Pathology of spinal injuries. Cent Nerv Syst Trauma. 1984;1:117.
Kalfas I., Wilberger J., Goldberg A., et al. Magnetic resonance imaging in acute spinal cord trauma. Neurosurgery. 1988;23:295.
Kamimura N., Shichida K., Tomita Y., et al. Spinal somatosensory evoked potentials in infants and children with spinal cord lesions. Brain Dev. 1988;10:355.
Katz R.T., Toleikis R.J., Knuth A.E. Somatosensory-evoked and dermatomal-evoked potentials are not clinically useful in the prognostication of acute spinal cord injury. Spine. 1991;16:730.
Keirstead H.S., Nistor G., Bernal G., et al. Human embryonic stem cell-derived oligodendrocyte progenitor cell transplants remyelinate and restore locomotion after spinal cord injury. J Neurosci. 2005;25:4694.
Kirshblum S. New rehabilitation interventions in spinal cord injury. J Spinal Cord Med. 2004;27:342.
Kiss Z.H.T., Tator C.H. Neurogenic shock. In: Geller E.R., editor. Shock and resuscitation. New York: McGraw-Hill, 1993.
Klose K.J., Green B.A., Smith R.S., et al. University of Miami Neuro-Spinal Index (UMNI): A quantitative method for determining spinal cord function. Paraplegia. 1980;18:331.
Knoller N., Auerbach G., Fulga V., et al. Clinical experience using incubated autologous macrophages as a treatment for complete spinal cord injury: phase I study results. J Neurosurg Spine. 2005;3:173.
Krassioukov A., Warburton D.E., Teasell R., et al. A systematic review of the management of autonomic dysreflexia after spinal cord injury. Arch Phys Med Rehabil. 2009;90:682.
Krause J.S., Sternberg M., Lottes S., et al. Mortality after spinal cord injury: An 11-year prospective study. Arch Phys Med Rehabil. 1997;78:815.
Kulkarni M.V., McArdle C.B., Kopanicky D., et al. Acute spinal cord injury: MR imaging at 1.5T. Radiology. 1987;164:837.
Kwon B.K., Mann C., Sohn H.M., et al. Hypothermia for spinal cord injury. Spine J. 2008;8:859.
Kwon B.K., Tetzlaff W., Grauer J.N., et al. Pathophysiology and pharmacologic treatment of acute spinal cord injury. Spine J. 2004;4:451.
Landi G., Ciccone A. GM-1 ganglioside for spinal-cord injury. [Letter to the editor]. N Engl J Med. 1992;326:493.
Lanska M.J., Roessmann U., Wiznitzer M. Magnetic resonance imaging in cervical cord birth injury. Pediatrics. 1990;85:760.
Lasiene J., Shupe L., Perlmutter S., et al. No evidence for chronic demyelination in spared axons after spinal cord injury in a mouse. J Neurosci. 2008;28:3887.
Lee S.M., Yune T.Y., Kim S.J., et al. Minocycline reduces cell death and improves functional recovery after traumatic spinal cord injury in the rat. J Neurotrauma. 2003;20:1017.
Levi A.D., Green B.A., Wang M.Y., et al. Clinical application of modest hypothermia after spinal cord injury. J Neurotrauma. 2009;26:407.
Levi L., Wolf A., Belzberg H. Hemodynamic parameters in patients with acute cervical cord trauma: Description, intervention, and prediction of outcome. Neurosurgery. 1993;33:1007.
Levitt M.A., Flanders A.E. Diagnostic capabilities of magnetic resonance imaging and computed tomography in acute cervical spinal column injury. Am J Emerg Med. 1991;9:131.
Levy M.L., Gans W., Wijesinghe H.S., et al. Use of methylprednisolone as an adjunct in the management of patients with penetrating spinal cord injury: Outcome analysis. Neurosurgery. 1996;39:1141.
Lewis K.S., Mueller W.M. Intrathecal baclofen for severe spasticity secondary to spinal cord injury. Ann Pharmacother. 1993;27:767.
Li C., Houlden D.A., Rowed D.W. Somatosensory evoked potentials and neurological grades as predictors of outcome in acute spinal cord injury. J Neurosurg. 1990;72:600.
Liptak G.S., Revell G.M. Management of bowel dysfunction in children with spinal cord disease or injury by means of the enema continence catheter. J Pediatr. 1992;120:190.
Lonstein J.E. Post-laminectomy spine deformity. In: Lonstein J.E., Bradford D.S., Winter R.B., et al, editors. Moe’s textbook of scoliosis and other spinal deformities. ed 3. Philadelphia: WB Saunders; 1995:506.
Louis A.A., Gupta P., Perkash I. Localization of sensory levels in traumatic quadriplegia by segmental somatosensory evoked potentials. Electroencephalogr Clin Neurophysiol. 1985;62:313.
Luce J.M. Medical management of spinal cord injury. Crit Care Med. 1985;13:6.
Lyden P., Wahlgren N.G. Mechanisms of action of neuroprotectants in stroke. J Stroke Cerebro Dis. 2000;9:9.
Mace S.E. Emergency evaluation of cervical spine injuries: CT versus plain radiographs. Ann Emerg Med. 1985;14:973.
Macias M.Y., Syring M.B., Pizzi M.A., et al. Pain with no gain: allodynia following neural stem cell transplantation in spinal cord injury. Exp Neurol. 2006;201:335.
Mackay-Sim A., Féron F., Cochrane J., et al. Autologous olfactory ensheathing cell transplantation in human paraplegia: a 3-year clinical trial. Brain. 2008:131. 2376
Mahoney F.I., Barthel D.W. Functional evaluation: The Barthel index. Md Med J. 1965;14:61.
Manno N.J., Uhlein A., Kernohan J.W. Intraspinal epidermoids. J Neurosurg. 1962;19:754.
Marion D.W. Head and spinal cord injury. Neurol Clin North Am. 1998;16:485.
Maroon J.C., Steele P.B., Berlin R. Football head and neck injuries – an update. Clin Neurosurg. 1980;27:414.
Massagli T.L. Medical and rehabilitation issues in the care of children with spinal cord injury. Phys Med Rehabil Clin N Am. 2000;11:169.
Matis G.K., Birbilis T.A. Erythropoietin in spinal cord injury. Eur Spine J. 2009;18:314.
Matsumura A., Meguro K., Tsurushima H., et al. Magnetic resonance imaging of spinal cord injury without radiographic abnormality. Surg Neurol. 1990;33:281.
McKeon R.J., Schreiber R.C., Rudge J.S., et al. Reduction of neurite outgrowth in a model of glial scarring following CNS injury is correlated with the expression of inhibitory molecules on reactive astrocytes. J Neurosci. 1991;11:3398.
Meinecke F.W. Early treatment of traumatic paraplegia. Paraplegia. 1964;1:262.
Meinecke F.W.. Initial clinical appraisal. Vinken P.J., Bruyn G.W., editors. Handbook of clinical neurology, 26. Amsterdam: North Holland, 1976.
Menezes A.H., Godersky J.C., Smoker W.R.K. Spinal cord injury. In McLaurin R.L., Venes J.L., Schut L., et al, editors: Pediatric neurosurgery: Surgery of the developing nervous system, ed 2, Philadelphia: WB Saunders, 1989.
Meyer G.A., Berman I.R., Doty D.B., et al. Hemodynamic responses to acute quadriplegia with or without chest trauma. J Neurosurg. 1971;34:168.
Meyer P.R., Cybulski G.R., Rusin J.J., et al. Spinal cord injury. Neurol Clin North Am. 1991;9:625.
Michaelis L.S.. Prognosis of spinal cord injury. Vinken P.J., Bruyn G.W., editors. Handbook of clinical neurology, 26. Amsterdam: North Holland, 1976.
Moreno-Manzano V., Rodríguez-Jiménez F.J., Garcia-Roselló M., et al. Activated spinal cord ependymal stem cells rescue neurological function. Stem Cells. 2009;27:733.
Morgan C., Newell S.J. Cervical spinal cord injury following cephalic presentation and delivery by Caesarean section. Dev Med Child Neurol. 2001;43:274.
Mueller F.O., Blyth C.S. Fatalities from head and cervical spine injuries occurring in tackle football: 40 years’ experience. Clin Sports Med. 1987;6:185.
Mu X., Azbill R.D., Springer J.E. Riluzone and methylprednisolone combined treatment improves functional recovery in traumatic spinal cord injury. J Neurotrauma. 2000;17:773.
Nance P.W., Bugaresti J., Shellenberger K., et al. Efficacy and safety of tizanidine in the treatment of spasticity in patients with spinal F cord injury. North American Tizanidine Study Group. Neurology. 1994;44(11 Suppl 9):S44.
Nashmi R., Fehlings M.G. Mechanisms of axonal dysfunction after spinal cord injury: with an emphasis on the role of voltage-gated potassium channels. Brain Res Rev. 2001;38:165.
Nesathurai S. Steroids and spinal cord injury: Revisiting the NASCIS 2 and NASCIS 3 trials. J Trauma Injury Infect Crit Care. 1998;45:1088.
Nógrádi A., Szabó A., Pintér S., et al. Delayed riluzole treatment is able to rescue injured rat spinal motoneurons. Neurosci. 2007;144:431.
Norenberg M.D., Smith J., Marcillo A. The pathology of human spinal cord injury: Defining the problems. J Neurotrauma. 2004;21:429.
Nugent G.R., Odom G.L., Woodhall B. Spinal extradural cysts. Neurology. 1959;9:397.
Nygaard I.E., Kreder K.J. Spine update: Urological management in patients with spinal cord injuries. Spine. 1996;21:128.
Oliver N.J. Annual estimates: Stroke and central nervous system trauma. Bethesda, MD: NINDS Office of Scientific and Health Reports; 1992.
Ommaya A.K. Whiplash injury: A review of clinical and experimental observations. Pak Med Rev. 1969;4:13.
Osenbach R.K., Menezes A.H. Pediatric spinal cord and vertebral column injury. Neurosurgery. 1992;30:385.
Osenbach R.K., Menezes A.H. Spinal cord injury without radiographic abnormality in children. Pediatr Neurosci. 1989;15:168.
Pang D. Spinal cord injury without radiologic abnormality in children, 2 decades later. Neurosurg. 2004;55:1325.
Pang D., Hanley E.N. Special problems of spinal stabilization in children. In: Cooper P.R., editor. Management of post-traumatic spinal instability. Park Ridge, IL: AANS, 1990.
Pang D., Wilberger J.E. Spinal cord injury without radiographic abnormalities in children. J Neurosurg. 1982;57:114.
Panjabi M., White A. Basic biomechanics of the spine. Neurosurgery. 1980;7:76.
Pannek J., Diederichs W., Botel U. Urodynamically controlled management of spinal cord injury in children. Neurourol Urodyn. 1997;16:285.
Pannu R., Christie D.K., Barbosa E., et al. Post-trauma Lipitor treatment prevents endothelial dysfunction, facilitates neuroprotection, and promotes locomotor recovery following spinal cord injury. J Neurochem. 2007;101:182.
Parisini P., Di Silverstre M., Greggi T. Treatment of spinal fractures in children and adolescents: Long-term results in 44 patients. Spine. 2002;27:1989.
Park E., Velumian A.A., Fehlings M.G. The role of excitotoxicity in secondary mechanisms of spinal cord injury: A review with an emphasis on the implications for white matter degeneration. J Neurotrauma. 2004;21:754.
Patel J.C., Tepas J.J., Mollitt D.L., et al. Pediatric cervical spine injuries: Defining the disease. J Pediatr Surg. 2001;36:373.
Peng W., Cotrina M.L., Han X., et al. Systemic administration of an antagonist of the ATP-sensitive receptor P2X7 improves recovery after spinal cord injury. Proc Natl Acad Sci USA. 2009;106:12489.
Pereira C.E., Lynch J.C. Spinal epidural abscess: An analysis of 24 cases. Surg Neurol. 2005;63:S26.
Perot P.L. The clinical use of somatosensory evoked potentials in spinal cord injury. Clin Neurosurg. 1973;20:367.
Perry V.H., Brown M.C., Gordon S. The macrophage response to central and peripheral nerve injury. A possible role for macrophages in regeneration. J Exp Med. 1987;165:1218.
Petitjean M.E., Pointillart V., Dixmerias F., et al. Medical treatment of spinal cord injury in the acute stage. Ann Fr Anesth Reanim. 1998;17:114.
Piepmeier J.M., Collins W.F.. Recovery of function following spinal cord injury. Vinken P.J., Bruyn G.W., Klawans H.L., editors. Handbook of clinical neurology, vol 61. Amsterdam: Elsevier Science, 1992. (RS 17)
Piper J.G., Menezes A.H. Pediatric spinal cord injury. In: Narayan R.K., Wilberger J.E., Povlishock J.T., editors. Neurotrauma. New York: McGraw-Hill, 1996.
Pitts L.H., Ross A., Chase G.A., et al. Treatment with thyrotropin-releasing hormone (TRH) in patients with traumatic spinal cord injuries. J Neurotrauma. 1995;12:235.
Plotkin R., Ronthal M., Froman C. Spontaneous spinal subarachnoid haemorrhage: report of 3 cases. J Neurosurg. 1966;25:443.
Polk-Williams A., Carr B.G., Blinman T.A., et al. Cervical spine injury in young children: a National Trauma Data Bank review. J Pediatr Surg. 2008;43:1718.
Proctor M.R. Spinal cord injury. Crit Care Med. 2002;30(Suppl):S489.
Profyris C., Cheema S.S., Zang D., et al. Degenerative and regenerative mechanisms governing spinal cord injury. Neurobiol Dis. 2004;15:415.
Quencer R.M., Bunge R.P., Egnor M., et al. Acute traumatic central cord syndrome: MRI-pathological correlations. Neuroradiology. 1992;34:85.
Quencer R.M., Green B.A., Eismont F.J. Post-traumatic spinal cord cysts: Clinical features and characterization with metrizamide computed tomography. Radiology. 1983;146:415.
Ramón-Cueto A., Cordero M.I., Santos-Benito F.F., et al. Functional recovery of paraplegic rats and motor axon regeneration in their spinal cords by olfactory ensheathing glia. Neuron. 2000;25:425.
Rand R.W., Crowdall P.H. Central spinal cord syndrome in hyperextension injuries of the cervical spine. J Bone Joint Surg. 1962;44:1415.
Reddy S.P., Junewick J.J., Backstrom J.W. Distribution of spinal fractures in children: Does age, mechanism of injury, or gender play a significant role? Pediatr Radiol. 2003;33:776.
Rekate H.L., Theodore N., Sonntag V.K.H., et al. Pediatric spine and spinal cord trauma: State of the art for the third millennium. Child Nerv Syst. 1999;15:743.
Reynolds R. Pediatric spinal injury. Curr Opin Pediatr. 2000;12:67.
Rihn J.A., Anderson D.T., Lamb K., et al. Cervical spine injuries in American football. Sports Med. 2009;39:697.
Robertson W.C.Jr, Lee Y.E., Edmonson M.B. Spontaneous spinal epidural hematoma in the young. Neurology. 1979;29:120.
Rosenzweig E.S., McDonald J.W. Rodent models for treatment of spinal cord injury: research trends and progress toward useful repair. Curr Opin Neurol. 2004;17:121.
Rosman N.P., Herskowitz J. Spinal cord trauma. In Swaiman K.F., Wright F.S., editors: Practice of pediatric neurology, ed 2, St. Louis: Mosby, 1982.
Rossier A.B., Foo D., Nabeedy M.H., et al. Radiography of posttraumatic syringomyelia. Am J Neuroradiol. 1983;4:637.
Rowed D.W., McLean J.A., Tator C.H. Somatosensory evoked potentials in acute spinal cord injury: Prognostic value. Surg Neurol. 1976;9:203.
Rowland J.W., Hawryluk G.W.J., Kwon B., et al. Current status of acute spinal cord injury pathophysiology and emerging therapies: promise on the horizon. Neurosurg Focus. 2008;25(5):E2.
Ruge J.R., Sinson G.P., McLone D.G., et al. Pediatric spinal injury: The very young. J Neurosurg. 1988;68:25.
Saberi H., Moshayedi P., Aghayan H.-R., et al. Treatment of chronic thoracic spinal cord injury patients with autologous Schwann cell transplantation: An interim report on safety considerations and possible outcomes. Neurosci Lett. 2008;443:46.
Sato T., Kokubun S., Rijal K.P., et al. Prognosis of cervical spinal cord injury in correlation with magnetic resonance imaging. Paraplegia. 1994;32:81.
Schaefer D.M., Flanders A.E., Osterholm J.L., et al. Prognostic significance of magnetic resonance imaging in the acute phase of cervical spine injury. J Neurosurg. 1992;76:218.
Schneider R.C. A syndrome in acute cervical injuries for which early operation is indicated. J Neurosurg. 1951;8:360.
Schneider R.C. The syndrome of acute anterior spinal cord injury. J Neurosurg. 1955;12:95.
Schneider R.C. Serious and fatal neurosurgical football injuries. Clin Neurosurg. 1964;12:226.
Schneider R.C., Crosby E.C., Russo R.H., et al. Traumatic spinal cord syndromes and their management. Clin Neurosurg. 1973;20:424.
Schneider R.C., Gosch H.H., Norrell H., et al. Vascular insufficiency and differential distortion of brain and cord caused by cervicomedullary football injuries. J Neurosurg. 1970;33:363.
Schurch B., Hodler J., Rodic B. Botulinum A toxin as a treatment of detrusor-sphincter dyssynergia in patients with spinal cord injury: MRI controlled transperineal injections. J Neurol Neurosurg Psychiatry. 1997;63:474.
Schwartz G., Fehlings M.G. Evaluation of the neuroprotective effects of sodium channel blockers after spinal cord injury: Improved behavioral and neuroanatomical recovery with riluzole. J Neurosurg. 2001;94:245.
Selden N.R., Quint D.J., Patel N. Emergency magnetic resonance imaging of cervical spinal cord injuries: Clinical correlation and prognosis. Neurosurgery. 1999;44:785.
Sett P., Crockard H.A. The value of magnetic resonance imaging (MRI) in the follow-up management of spinal injury. Paraplegia. 1991;29:396.
Shapiro R., Youngberg A., Rothman S. The differential diagnosis of traumatic lesions of the occipito-atlanto-axial segment. Radiol Clin North Am. 1973;11:505.
Shapiro S., Borgens R., Pascuzzi R., et al. Oscillating field stimulation for complete spinal cord injury in humans: a Phase 1 trial. J Neurosurg Spine. 2005;2:3.
Sharma H.S. Post-traumatic application of brain-derived neurotrophic factor and glia-derived neurotrophic factor on the rat spinal cord enhances neuroprotection and improves motor function. Acta Neurochir Suppl. 2006;96:329.
Sharma H.S., Nyberg F., Gordh T., et al. Topical application of dynorphin A (1-17) antibodies attenuates neuronal nitric oxide synthase up-regulation, edema formation, and cell injury following focal trauma to the rat spinal cord. Acta Neurochir Suppl. 2006;96:309.
Sharma H.S., Sjöquist P.O., Mohanty S., et al. Post-injury treatment with a new antioxidant compound H-290/51 attenuates spinal cord trauma-induced c-fos expression, motor dysfunction, edema formation, and cell injury in the rat. Acta Neurochir Suppl. 2006;96:322.
Shaywitz B.A. Epidermoid spinal cord tumors and previous lumbar punctures. J Pediatr. 1972;80:638.
Shefner J.M., Tun C. Clinical neurophysiology of focal spinal cord injury. Neurol Clin North Am. 1991;9:671.
Shurman G., Lobaugh P., Wilberger J.E. Application of evoked potential monitoring in spinal cord injury. In: Narayan R.K., Wilberger J.E., Povlishock J.T., editors. Neurotrauma. New York: McGraw-Hill, 1996.
Siddall P.J., Taylor D., Cousins M.J. Pain associated with spinal cord injury. Curr Opin Neurol. 1995;8:447.
Singer P.A., Prokop L.D., Anderson D.K. Somatosensory cortical evoked responses after feline experimental spinal cord injury. Paraplegia. 1977;15:160.
Sonntag V.K.H., Francis P.M. Patient selection and timing of surgery in contemporary management of spinal cord injury. Park Ridge, IL: AANS Publication Committee; 1995.
Spiess M., Schubert M., Kliesch U., et al. Evolution of tibial SSEP after traumatic spinal cord injury: baseline for clinical trials. Clin Neurophysiol. 2008;119:1051.
Stauffer E.S. Neurologic recovery following injuries to the cervical spinal cord and nerve roots. Spine. 1984;9:532.
Stringer W.A., Andersen B.J. Imaging after spine trauma. In: Evans R.W., editor. Neurology and trauma. Philadelphia: WB Saunders, 1996.
Stroman P.W., Kornelsen J., Bergman A., et al. Noninvasive assessment of the injured human spinal cord by means of functional magnetic resonance imaging. Spinal Cord. 2004;42:59.
Suh T.H., Alexander L. Vascular system of the human spinal cord. Arch Neurol Psychiatry. 1939;41:659.
Swanson H.S., Fincher E.F. Extradural arachnoidal cysts of traumatic origin. J Neurosurg. 1947;4:530.
Swischuk L.E. Spine and spinal cord trauma in the battered child syndrome. Radiology. 1969;92:733.
Syková E., Homola A., Mazanec R., et al. Autologous bone marrow transplantation in patients with subacute and chronic spinal cord injury. Cell Transplant. 2006;15:675.
Talbot H.S. Psycho-social aspects of sexuality in spinal cord injury patients. Paraplegia. 1971;9:37.
Tator C.H. Spine – spinal cord relationships in spinal cord trauma. Clin Neurosurg. 1983;30:479.
Tator C.H. Classification of spinal cord injury based on neurological presentation. In: Narayan R.K., Wilberger J.E., Povlishock J.T., editors. Neurotrauma. New York: McGraw-Hill, 1996.
Tator C.H. Review of treatment trials in human spinal cord injury: Issues, difficulties, and recommendations. Neurosurgery. 2006;59:957.
Tator C.H., Duncan E.G., Edmonds V.E., et al. Changes in epidemiology of acute spinal cord injury from 1947 to 1981. Surg Neurol. 1993;40:207.
Taylor T.V., Rimmer S., Day B., et al. Ascorbic acid supplementation in the treatment of pressure sores. Lancet. 1974;2:544.
Tei R., Kaido T., Nakase H., et al. Protective effect of C1 esterase inhibitor on acute traumatic spinal cord injury in the rat. Neurol Res. 2008;30:761.
Teng Y.D., Choi H., Onario R.C., et al. Minocyclinc inhibits contusion-triggered mitochondrial cytochrome c release and mitigates functional deficits after spinal cord injury. PNAS. 2004;101:3071.
Tian D.S., Liu J.L., Xie M.J., et al. Tamoxifen attenuates inflammatory-mediated damage and improves functional outcome after spinal cord injury in rats. J Neurochem. 2009;109:1658.
Toleikis J.R., Sloan T.B. Comparison of major nerve and dermatomal somatosensory evoked potentials in the evaluation of patients with spinal cord injury. In: Barber C., Blum T., editors. Evoked potentials III. The Third International Evoked Potentials Symposium. Boston: Butterworth, 1987.
Torg J.S., Truex R., Quedenfeld T.C., et al. The national football head and neck injury registry: Report and conclusions. 1979. JAMA. 1979;241:1477.
Towbin A. Spinal cord and brain stem injury at birth. Arch Pathol. 1964;77:620.
Vale F.L., Burns J., Jackson A.B., et al. Combined medical and surgical treatment after acute spinal cord injury: Results of a prospective pilot study to assess the merits of aggressive medical resuscitation and blood pressure management. J Neurosurg. 1997;87:239.
Vogel L., Mulcahy M.J., Betz R.R. The child with a spinal cord injury. Dev Med Child Neurol. 1997;39:202.
Vogel L.C., Anderson C.J. Spinal cord injuries in children and adolescents: a review. J Spinal Cord Med. 2003;26:193.
Walker M.D. Acute spinal-cord injury. N Engl J Med. 1991;324:1885.
Walter C.E., Tedeschi L.G. Spinal injury and neonatal death. Am J Obstet Gynecol. 1970;106:272.
Wang M.Y., Hoh D.J., Leary S.P., et al. High rates of neurological improvement following severe traumatic pediatric spinal cord injury. Spine. 2004;29:1493.
Weinshel S.S., Maiman D.J., Baek P., et al. Neurologic recovery in quadriplegia following operative treatment. J Spinal Disord. 1990;3:244.
White A.A., Panjabi M. Clinical biomechanics of the spine, ed 2. Philadelphia: JB Lippincott; 1990.
Wilberger J.E. Pharmacological resuscitation for spinal cord injury. In: Narayan R.K., Wilberger J.E., Povlishock J.T., editors. Neurotrauma. New York: McGraw-Hill, 1996.
Wiseman D.B., Dailey A.T., Lundin D., et al. Magnesium efficacy in a rat spinal cord injury model. J Neurosurg Spine. 2009;10:308.
Wittenberg R.H., Boetel U., Beyer H.K. Magnetic resonance imaging and computer tomography of acute spinal cord trauma. Clin Orthop. 1990;260:176.
Xie J., Boakye M. Electrophysiological outcomes after spinal cord injury. Neurosurg Focus. 2008;25(5):E11.
Yezierski R.P. Pain following spinal cord injury: The clinical problem and experimental studies. Pain. 1996;68:185.
Yoon S.H., Shim Y.S., Park Y.H., et al. Complete spinal cord injury treatment using autologous bone marrow cell transplantation and bone marrow stimulation with granulocyte macrophage-colony stimulating factor: Phase I/II clinical trial. Stem Cells. 2007;25:2066.
York D.H., Watts C., Raffensberger M., et al. Utilization of somatosensory evoked cortical potentials in spinal cord injury, prognostic limitations. Spine. 1983;8:832.
Ziganow S. Neurometric evaluation of the cortical somatosensory evoked potential in acute incomplete spinal cord injuries. Electroencephalogr Clin Neurophysiol. 1986;65:86.