Solutions, Body Fluids, and Electrolytes
After reading this chapter you will be able to:
Describe the characteristics of and key terms associated with solutions, colloids, and suspensions.
Describe the five factors that influence the solubility of a substance in a solution.
Describe how osmotic pressure functions and what its action is in relation to cell membranes.
Describe how to calculate the solute content of a solution using ratio, weight/volume, and percent methods.
State the ionic characteristics of acids, bases, and salts.
Describe how proteins can function as bases.
Describe how to calculate the pH of a solution when given the [H+] in nanomoles per liter.
Identify where fluid compartments are located in the body and what their volumes are.
Describe how water loss and replacement occur.
Define the roles played by osmotic and hydrostatic pressure in edema.
Identify clinical findings associated with excess or deficiency of the seven basic electrolytes.
Solutions, Colloids, and Suspensions
Definition of a Solution
Colloids (sometimes called dispersions or gels) consist of large molecules that attract and hold water (hydrophilic: “water loving”). These molecules are uniformly distributed throughout the dispersion, and they tend not to settle. The protoplasm inside cells is a common example of a colloid. Physiologically, colloids provide very little free water to the patient’s system, and care should be taken not to create a hypotonic environment.1
1. Nature of the solute. The ease with which substances go into a solution (dissociation) in a given solvent depends on the forces of the solute-solute molecules and varies widely.
2. Nature of the solvent. The ability of a solvent to dissolve a solute depends on the bonds of the solvent-solvent molecules and varies widely.
3. Temperature. Solubility of most solids increases with increased temperature. However, the solubility of gases varies inversely with temperature.
4. Pressure. The solubility of solids and liquids is not greatly affected by pressure. However, the solubility of gases in liquids varies directly with pressure.
5. Concentration. The concentration of a solute or available solvent affects how much of the substance goes into solution.
The effects of temperature and pressure on the solubility of gases are important. More gas dissolves in a liquid at lower temperatures. As the temperature of a liquid increases, gas dissolved in that liquid comes out of solution. Henry’s law describes the effect of pressure on solubility of a gas in a liquid. At a given temperature, the volume of a gas that dissolves in a liquid is proportional to the solubility coefficient of the gas and the partial pressure of gas to which the liquid is exposed. Oxygen (O2) and carbon dioxide (CO2) transport can change significantly with changes in body temperature or atmospheric pressure (see Chapter 6).
Concentration of Solutions
The term concentration refers to the amount of solute dissolved into the solvent. Concentration can be described either qualitatively or quantitatively. Calling something a dilute solution is an example of a qualitative description. Stating that a specific container holds 50 ml of 0.4 molar solution of sodium hydroxide (NaOH) is a quantitative description (Figure 12-1, A). Saturated solutions occur when the solvent has dissociated the maximal amount of solute into itself. Additional solute added to a saturated solution does not dissociate into solution but remains at the bottom of the container (see Figure 12-1, B). Solute particles precipitate into the solid state at the same rate at which other particles go into solution. This equilibrium characterizes a saturated solution.
A solution is characterized as being supersaturated when the solvent contains more solute than a saturated solution at the same temperature and pressure. If a saturated solution is heated, the solute equilibrium is upset, and more solute goes into solution. If undissolved solute is removed and the solution is cooled gently, there is an excess of dissolved solute (see Figure 12-1, C). The excess solute of supersaturated solutions may be precipitated out if the solution is disturbed or if a “seed crystal” is introduced.
Starling Forces
Starling was a nineteenth-century British physiologist who studied fluid transport across membranes. His hypothesis states that the fluid movement secondary to filtration across the wall of a capillary depends on both the hydrostatic and the oncotic pressure gradients across the capillary.2 The driving force for fluid filtration across the wall of the capillary is determined by four separate pressures: hydraulic (hydrostatic) and colloid osmotic pressure both within the vessel and in the tissue space.3 This process can be described mathematically using the following equation:
< ?xml:namespace prefix = "mml" />

Osmotic Pressure of Solutions
Osmotic pressure (oncotic pressure)4 is the force produced by solvent particles under certain conditions. A membrane that permits passage of solvent molecules but not solute is called a semipermeable membrane. If such a membrane divides a solution into two compartments, molecules of solvent can pass through it from one side to the other (Figure 12-2, A). The number of solvent molecules passing (or diffusing) in one direction must equal the number of solute molecules passing in the opposite direction. An equal ratio of solute to solvent particles (i.e., the concentration of the solution) is maintained on both sides of the membrane. A capillary wall is an example of a semipermeable membrane.5,6
If a solution is placed on one side of a semipermeable membrane and pure solvent is placed on the other, solvent molecules move through the membrane into the solution. The force driving solvent molecules through the membrane is called osmotic pressure. Osmotic pressure tries to redistribute solvent molecules so that the same concentration exists on both sides of the membrane. Osmotic pressure may be measured by connecting a manometer to the expanding column of the solution (see Figure 12-2, B and C).
Osmotic pressure can also be visualized as an attractive force of solute particles in a concentrated solution. If 100 ml of a 50% solution is placed on one side of a membrane and 100 ml of a 30% solution is placed on the other side, solvent molecules move from the dilute to the concentrated side (see Figure 12-2, D and E). The particles in the concentrated solution attract solvent molecules from the dilute solution until equilibrium occurs. Equilibrium exists when the concentrations (i.e., ratio of solute to solvent) in the two compartments are equal (40% in Figure 12-2).
Osmolality is defined as the ratio of solute to solvent. In physiology, the solvent is water.1,5,7 Osmotic pressure depends on the number of particles in solution but not on their charge or identity. A 2% solution has twice the osmotic pressure of a 1% solution under similar pressures. For a given amount of solute, osmotic pressure is inversely proportional to the volume of solvent. Most cell walls are semipermeable membranes. Through osmotic pressure, water is distributed throughout the body within certain physiologic ranges. Tonicity describes how much osmotic pressure is exerted by a solution. Average body cellular fluid has a tonicity equal to a 0.9% solution of sodium chloride (NaCl; sometimes referred to as physiologic saline). Solutions with similar tonicity are called isotonic. Solutions with more tonicity are hypertonic, and solutions with less tonicity are hypotonic. Most cells reside in a hypotonic environment in which the concentration of water (solute) is lower inside the cell than in the surroundings. Water flows into the cell causing it to expand until the cell membrane restricts further expansion. Pressure increases inside the cell to counteract osmotic pressure. This pressure is called turgor, and it is what prevents more water from entering the cell. The equilibrium that develops allows the cell to maintain a gradient across the cell membrane. Some cells have selective permeability, allowing passage not only of water but also of specific solutes. Through these mechanisms, nutrients and physiologic solutions are distributed throughout the body.
In electrochemical terms, there are three basic types of physiologic solutions. Depending on the solute, solutions are ionic (electrovalent), polar covalent, or nonpolar covalent (Table 12-1). In ionic and polar covalent solutions, some of the solute ionizes into separate particles known as ions. A solution in which this dissociation occurs is called an electrolyte solution (Figure 12-3). If an electrode is placed in such a solution, positive ions migrate to the negative pole of the electrode. These ions are called cations. Negative ions migrate to the positive pole of the electrode; they are called anions. In nonpolar covalent solutions, molecules of solute remain intact and do not carry electrical charges; these solutions are referred to as nonelectrolytes. These nonelectrolytes are not attracted to either the positive or the negative pole of an electrode (hence the designation nonpolar). All three types of solutions coexist in the body. These solutions also serve as the media in which colloids and simple suspensions are dispersed. Gases such as O2 and CO2 are nonpolar molecules (along with N2) and do not dissolve very well in water, which is a polar solvent.
TABLE 12-1
Types of Physiologic Solutions
Type | Characteristics | Physiologic Example |
Ionic (electrovalent) | Ionic compounds dissolved from crystalline form, usually in water (hydration); form strong electrolytes with conductivity dependent on concentration of ions | Saline solution (0.9% NaCl) |
Polar covalent | Molecular compounds dissolved in water or other solvents to produce ions (ionization); electrolytes may be weak or strong, depending on degree of ionization; solutions polarize and are good conductors | Hydrochloric acid (HCl) (strong electrolyte); acetic acid (CH3COOH) (weak electrolyte) |
Nonpolar covalent | Molecular compounds dissolved into electrically neutral solutions (do not polarize); solutions are not good conductors; nonelectrolytes | Glucose (C6H12O6) |
Solute Content by Weight


To convert a serum Na+ value of 322 mg/dl to mEq/L, the equation is used as follows:
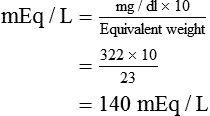
In clinical practice, electrolyte replacement is common when a laboratory test identifies a significant deficiency. The electrolyte content of intravenous solutions is usually stated in milligrams per deciliter or in mEq per liter. Lactated Ringer’s solution is one such infusion used for electrolyte replacement (Table 12-2).
TABLE 12-2
Concentration of Ingredients in Lactated Ringer’s Solution
Substance | mg/dl | Approximate mEq/L |
NaCl (sodium chloride) | 600 Na | 130 |
310 Cl | 109 | |
NaC3H5O3 (sodium lactate) | 30 C3H5O3 | 28 |
KCl (potassium chloride) | 30 K | 4 |
CaCl2 (calcium chloride) | 20 Ca | 27 |
Quantitative Classification of Solutions
The amount of solute in a solution may be quantified by the following six methods:
1. Ratio solution. The amount of solute to solvent is expressed as a proportion (e.g., 1 : 100). Ratio solutions are sometimes used in describing concentrations of drugs.
2. Weight-per-volume solution (W/V). The W/V solution is commonly used for solids dissolved in liquids. It is defined as weight of solute per volume of solution. This method is sometimes erroneously described as a percent solution. W/V solutions are commonly expressed in grams of solute per 100 ml of solution. For example, 5 g of glucose dissolved in 100 ml of solution is properly called a 5% solution, according to the W/V scheme. A liquid dissolved in a liquid is measured as volumes of solute to volumes of solution.
3. Percent solution. A percent solution is weight of solute per weight of solution. For example, 5 g of glucose dissolved in 95 g of water is a true percent solution. The glucose is 5% of the total solution weight of 100 g.
4. Molal solution. A molal solution contains 1 mole of solute per kilogram of solvent, or 1 mmol/g solvent. The concentration of a molal solution is independent of temperature.
5. Molar solution. A molar solution has 1 mole of solute per liter of solution, or 1 mmol/ml of solution. Solute is measured into a container, and solvent is added to produce the solution volume desired.
6. Normal solution. A normal solution has 1 gEq of solute per liter of solution, or 1 mEq/ml of solution. For all monovalent solutes, normal and molar solutions are the same. The equivalent weights of their solutes equal their gram formula weights. Equal volumes of solutions of the same normality contain chemically equivalent amounts of their solutes. If the solutes react chemically with one another, equal volumes of the solutions react completely. Neither substance remains in excess. In the analytic process of titration, normal solutions are often used as standards to determine the concentrations of other solutions.
Dilution Calculations

1. Diluting 10 ml of a 2% (0.02) solution to a concentration of 0.5% (0.005) requires finding the new volume (V2) by rearranging the dilution equation as follows:

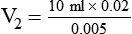
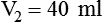
2. If 50 ml of water is added to 150 ml of a 3% (0.03) solution, the new concentration is calculated by rearranging the dilution equation to find C2 as follows:

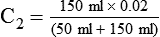

3. To dilute 50 ml of a 0.33 normal (N) solution to a 0.1N concentration, concentration is given as normality, but it can be used similar to a percentage. The new volume (V2) can be calculated by rearranging the dilution equation as follows:

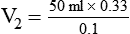

Electrolytic Activity and Acid-Base Balance
Acid-base balance depends on the concentration and activity of electrolytic solutes in the body. Clinical application of acid-base homeostasis is discussed in detail in Chapter 13.
Characteristics of Acids, Bases, and Salts
Acids
The term acid refers to either compounds that can donate [H+] (Brönsted-Lowry acid) or any compound that accepts an electron pair (Lewis acid). Although these two theories of acids differ in which is being transferred, both theories attempt to describe how reactive groups perform within an aqueous solution8,9:


Bases
Nonhydroxide Bases
Protein Bases
The imidazole group of the amino acid histidine is an example of an H+ acceptor on a protein molecule (Figure 12-4). The ability of proteins to accept hydrogen ions limits H+ activity in solution, which is called buffering. The buffering effect of hemoglobin is produced by imidazole groups in the protein. Each hemoglobin molecule contains 38 histidine residues. Each oxygen-carrying component (heme group) of hemoglobin is attached to a histidine residue. The ability of hemoglobin to accept (i.e., buffer) H+ ions depends on its oxygenation state. Deoxygenated (reduced) hemoglobin is a stronger base (i.e., a better H+ acceptor) than oxygenated hemoglobin. This difference partially accounts for the ability of reduced hemoglobin to buffer more acid than oxygenated hemoglobin can (see Chapter 13). Plasma proteins also act as buffers, although with less buffering power than hemoglobin, which contains more histidine.
Designation of Acidity and Alkalinity
pH Scale

Using this scheme, in a solution with a pH of 7.00, the [H+] is the same as would be seen in pure water, so by definition this is called “neutral.” As the pH decreases to less than 7.00, the solution is termed more acidic, and when the pH increases to greater than 7.00, the solution is considered to be basic. With a whole number change in pH (i.e., pH decreasing from 7.00 to 6.00), the [H+] is a factor of 10 less. With a pH increase from 7.00 to 8.00, the [H+] is 10 times greater (Figure 12-5).
The law of mass action states that acids and bases freely dissociate and r-associate in a solution at a constant rate relative to the structure of the acid and the temperature of the system.10 Using the Henderson-Hasselbalch equation, which describes the ratio of [H+] to base, we can calculate expected pH (see Chapter 13).

pK = Inverse log of dissociation constant of solution
log [H+] = Logarithm of [H+], which can be expressed as the ratio between conjugate acid and total acid concentration
Applying these concepts in an example pertinent to clinical medicine yields the following:

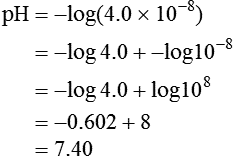
Body Fluids and Electrolytes
Body Water
Water is a major component of the body. It constitutes 45% to 80% of an individual’s body mass, depending on the mass, gender, and age of the individual. Leanness is associated with higher body water content. Obese individuals have a lower percentage of body water (≤30% less) than normal-weight individuals. Men have a slightly higher percentage of total body water than women. Total percentage of body water in infants and children is substantially greater than in adults. In a newborn, water accounts for 80% of the total body weight (Table 12-3).
TABLE 12-3
Body Water | Man (% body weight) | Woman (% body weight) | Infant (% body weight) |
Total body | 60 ± 15 | 50 ± 15 | 80 |
Water | |||
Intracellular | 45 | 40 | 50 |
Extracellular | 15-20 | 15-20 | 30 |
Interstitial | 11-15 | 11-15 | 24 |
Intravascular | 4.5 | 4.5 | 5.0 |
Transcellular | <1 | <1 | <1 |
Distribution
Total body water is divided into the following two major compartments: (1) intracellular (“within the cells”) and (2) extracellular (“outside the cells”). Intracellular water accounts for approximately two-thirds of the total body water, and extracellular water accounts for the remaining one-third. Extracellular water is found in three subcompartments: (1) intravascular water (plasma), (2) interstitial water, and (3) transcellular fluid. Intravascular water constitutes approximately 5% of the body weight. Interstitial water is water in the tissues between the cells. It constitutes approximately 15% of the body weight. The proportion of transcellular fluid is quite small in proportion to plasma and interstitial fluid. Interstitial fluid is a matrix—a collagen/gel substance that allows the interstitium to provide structural support during times of extracellular volume depletion.11 Examples of transcellular fluid include cerebrospinal fluid, digestive juices, and mucus. Transcellular fluid can become an important third space in some pathologic conditions, such as ascites (excess fluid in the peritoneal cavity) or pleural effusion (fluid collection in the pleural space).
Regulation
The kidneys maintain the volume and composition of body fluids via two related mechanisms. First, filtration and reabsorption of sodium adjust urinary sodium excretion to match changes in dietary intake. Second, water excretion is regulated by osmoreceptors which are located in the hypothalamus and modulate secretion of antidiuretic hormone (ADH, also known as vasopressin).7,12,13 These receptors are exceptionally sensitive; in vivo studies have shown that a single neuron can respond to either an osmotic or a nonosmotic baroreceptor simulus.13 These mechanisms allow the kidneys to maintain the volume and concentration of body fluid despite variations in salt and water intake. Analysis of the urine (urinalysis) often provides diagnostic clues in disorders of body fluid volume.
Water Losses
Water may be lost from the body through the skin, lungs, kidneys, and gastrointestinal (GI) tract. Water loss can be insensible, such as evaporation of water from the skin and lungs, or sensible, such as losses from urine and the GI tract (Table 12-4).14 Fluid losses from the body may also occur during vomiting, diarrhea, or suctioning from the stomach. Fever, in conjunction with sweating, also can cause significant losses.
TABLE 12-4
Regulation | Average Daily Volume (ml) | Maximum Daily Volume |
Water Losses | ||
Insensible | ||
Skin | 700 | 1500 ml |
Lung | 200 | |
Sensible | ||
Urine | 1000-1200 | >2000 ml/hr |
Intestinal | 200 | 8000 ml |
Sweat | 0 | >2000 ml/hr |
Water Gain | ||
Ingestion | ||
Fluids | 1500-2000 | 1500 ml/hr |
Solids | 500-600 | 1500 ml/hr |
Body metabolism | 250 | 1000 ml |
Other causes of abnormal fluid loss include certain renal and respiratory disorders. Patients with renal disease may have to excrete larger quantities of urine to get rid of extra nitrogenous wastes. Patients with increased ventilation also have increased water losses through increased evaporation from the respiratory tract. Patients with artificial airways are prone to evaporative water loss if inspired air is not adequately humidified. Infants have a greater proportion of body water than adults, particularly in the extracellular compartments (see Table 12-3). Water loss in infants may be twice of the water loss in adults. Infants also have a greater body surface area (in proportion to body volume) than adults, making their basal heat production twice as high. Higher metabolic rates in infants necessitate greater urinary excretion. Infants turn over approximately one-half of their extracellular fluid volume daily; adults turn over approximately one-seventh. Fluid loss or lack of intake can rapidly deplete an infant of water.
Transport Between Compartments
In a typical capillary, blood pressure is approximately 30 mm Hg at the arterial end and approximately 20 mm Hg at the venous end (Figure 12-6). Colloid osmotic pressure of the intravascular fluid remains constant at approximately 25 mm Hg. Hydrostatic pressure along the capillary continually decreases. At the arterial end, hydrostatic pressure normally exceeds osmotic pressure, and water flows out of the vascular space into the interstitial space. At the venous end, colloidal osmotic pressure exceeds hydrostatic forces. Water is pulled back into the vascular compartment.
The outflow of water and electrolytes from the capillary at the arterial end is not completely balanced by the return on the venous end. Slightly more water diffuses out than is reabsorbed. This slight outward excess is balanced by fluid return through the lymphatic circulation (see Chapter 8). Fluid return via lymphatic channels also depends on pressure differences. The pressure in the interstitial space is determined by the volume of interstitial fluid and its electrolyte content. Interstitial fluid moves from a region of higher pressure (interstitial space) to a region of lower pressure (lymphatic channels). This lymph fluid moves into larger lymphatic spaces, where the pressure is continuously decreasing.
These relationships may be expressed by the Starling equilibrium equation:

Qf = Bulk flow of fluid between intravascular and interstitial compartments
Pch = Capillary hydrostatic pressure
Pih = Interstitial fluid hydrostatic pressure
Pco = Capillary osmotic pressure
Pio = Interstitial osmotic pressure
K1 = Capillary permeability coefficient for fluids and electrolytes
1. High intravascular hydrostatic pressure is balanced by a proportionally greater interstitial pressure.
2. The “pumping” action of the skeletal muscles surrounding leg veins reduces venous pressures.
3. Lymph flow back to the thorax is enhanced via a similar mechanism; this facilitates clearance of excess interstitial fluid.
Edema can be caused by other factors. The Starling equilibrium equation given earlier shows that edema can be caused by a decrease in colloid osmotic pressure or an increase in capillary permeability. If albumin is depleted in the blood, the balance of forces is upset, favoring increased movement of fluid into the interstitium. Likewise, an increase in capillary permeability results in more fluid leaving the capillaries. Increased capillary permeability is a major factor in certain types of acute lung injuries (see Chapter 27).15
Electrolytes
Sodium (Na+)
Sodium is the major circulating cation within the body.16 Regulation of sodium concentration in plasma and urine is related to regulation of total body water. Of the total body stores of sodium, 50% are extracellular. The remaining sodium is found in bone (40%) and in cells (10%). The normal serum concentration of sodium is 136 to 145 mEq/L. In cells, the sodium concentration is much lower, averaging only 4.5 mEq/L.
The average adult ingests and excretes approximately 100 mEq of sodium every 24 hours. Children require approximately half this amount, and infants typically exchange 20 mEq of sodium per day. Most sodium is reabsorbed through the kidney. Approximately 80% of the sodium in the body is reclaimed passively in the proximal tubules. The remainder is actively reabsorbed in the distal tubules. Sodium reabsorption in the kidneys is governed mainly by the level of aldosterone, which is secreted by the adrenal cortex. Na+ reabsorption in the distal tubules of the kidney occurs in exchange for other cations. Sodium balance is involved in acid-base homeostasis (i.e., H+ exchange) and the regulation of potassium (K+). Abnormal losses of sodium can lead to hyponatremia (low sodium concentration in the plasma) and may occur for numerous reasons, as shown in Table 12-5.
TABLE 12-5
Electrolyte Disorders and Clinical Findings
Electrolyte | Imbalance | Causes | Symptoms |
Sodium (Na+) | Hyponatremia | GI loss, sweating, fever, diuretics, ascites, congestive heart failure, kidney failure | Weakness, lassitude, apathy, headache, orthostatic hypotension, tachycardia |
Hypernatremia | Net sodium gain, net water loss, increased aldosterone, steroid therapy | Tremulousness, irritability, ataxia, confusion, seizures, coma | |
Chloride (Cl−) | Hypochloremia | GI loss, diuretics | Metabolic alkalosis, muscle spasm, coma (severe cases) |
Hyperchloremia | Dehydration, metabolic acidosis, respiratory alkalosis | (Minimal) | |
Potassium (K+) | Hypokalemia | Diuretics, steroid therapy, renal tubular disease, vomiting, diarrhea, malnutrition, trauma | Muscle weakness, paralysis, ECG abnormalities, supraventricular arrhythmias, circulatory failure, cardiac arrest |
Hyperkalemia | Chronic renal disease, hemorrhage, tissue necrosis, nonsteroidal antiinflammatory drugs, ACE inhibitors, cyclosporine, K+-sparing diuretics | ECG changes, ventricular arrhythmias, cardiac arrest | |
Calcium (Ca++) | Hypocalcemia | Hyperparathyroidism, pancreatitis, renal failure, trauma | Hyperactive tendon reflexes, muscle twitching, spasm, abdominal cramps, ECG changes, convulsions (rarely) |
Hypercalcemia | Hyperthyroidism, hyperparathyroidism, metastatic bone cancer, sarcoidosis | Fatigue, depression, muscle weakness, anorexia, nausea, vomiting, constipation | |
Magnesium (Mg++) | Hypomagnesemia | Inadequate intake/impaired absorption of Mg++, pancreatitis, alcoholism | Muscle weakness, irritability, tetany, ECG changes, arrhythmias, delirium, convulsions |
Hypermagnesemia | Dehydration, renal insufficiency, tissue trauma, lupus erythematosus | ECG changes (along with hyperkalemia, cardiac arrest, respiratory muscle paralysis) | |
Phosphate (HPO42−) | Hypophosphatemia | Starvation, malabsorption, hyperparathyroidism, hyperthyroidism, uncontrolled diabetes mellitus | Diaphragmatic weakness |
Hyperphosphatemia | Endocrine disorders, acromegaly, chronic renal insufficiency, acute renal failure, tissue trauma | (Minimal) |
Hyponatremia, which is the most common electrolyte imbalance found in hospitalized patients, is defined as having serum sodium levels less than 135 mEq/L.7 Previously considered to be benign, mild hyponatremia has been shown in more recent studies to have a significant impact on a patient’s cognitive function and gait stability, and it is thought to be a contributing factor in falls.17 Hyponatremia can lead to cerebral edema owing to a change in osmotic pressure; the two most common causes for acute hyponatremia are postoperative iatrogenic and self-induced secondary to water intoxication.17 One type of normal-volume (euvolemic) hyponatremia is known as syndrome of inappropriate antidiuretic hormone secretion (SIADH).5,7,13,16–18
Treatment of hypovolemic hyponatremia can have dire consequences as well. If fluid is administered too quickly, damage to the central nervous system occurs. With significant fluid shifts in Na+ concentrations, rapid changes in cellular volume can lead to cell damage and cell death (apoptosis).7 Osmotic demyelination syndrome occurs when serum sodium concentration changes more than 10 mEq/L in chronic hyponatremia or 18 mEq/L over 48 hours.17
Chloride (Cl−)
Normal serum levels of chloride (Cl−) are 98 to 106 mEq/L. The concentration of extracellular chloride is inversely proportional to the concentration of the other major anion, bicarbonate (HCO3−). Cl− is regulated by the kidney in much the same manner as Na+ (80% reabsorbed in the proximal tubules and 20% reabsorbed in the distal tubules). Cl− is usually excreted with potassium in the form of KCl. An imbalance in one of these electrolytes usually affects both. Replacement therapy usually includes both K+ and Cl−. The stomach and the small bowel also affect the balance of Cl−, and sweat contains hypotonic quantities of Cl−. Abnormal Cl− levels may occur for various reasons (see Table 12-5).
Bicarbonate (HCO3−)
After chloride, bicarbonate (HCO3−) is the most important body fluid anion. It plays an important role in acid-base homeostasis and is the strong base in the bicarbonate–carbonic acid buffer pair (see Chapter 13). HCO3− is the primary means for transporting CO2 from the tissues to the lungs. The ratio of HCO3− to carbonic acid in healthy individuals is maintained near 20 : 1; this results in a pH of close to 7.40. HCO3− stores are evenly divided between intracellular and extracellular compartments. Normal serum HCO3− levels in arterial blood range from 22 to 26 mEq/L. HCO3− levels are slightly higher in venous blood as CO2 is being transported to the lungs.
In acid-base disorders, the kidneys regulate HCO3− levels to maintain a near-normal pH. In healthy individuals, more than 80% of blood HCO3− is reabsorbed in the proximal tubules of the kidney. The remainder is reclaimed in the distal tubules. In respiratory acidosis, the kidneys retain or produce HCO3− to buffer the additional acid caused by CO2 retention. In respiratory alkalosis, the opposite occurs. A reciprocal relationship exists between Cl− and HCO3− concentrations. HCO3− retention is associated with chloride excretion, and vice versa (see Chapter 13).
Potassium (K+)
Renal excretion of K+ is controlled by aldosterone levels.19 Aldosterone inhibits the enzyme responsible for K+ transport in the distal renal tubular cells of the kidney. Metabolic acidosis also inhibits the transport system. Na+ and H+ ions enter cells at the expense of increased K+ excretion. Alkalosis has the reverse effect. It stimulates cellular retention of K+. Kidney failure results in potassium retention and hyperkalemia.
Hypokalemia (reduced serum potassium) disturbs cellular function in numerous organ systems, including the GI, neuromuscular, renal, and cardiovascular systems (see Table 12-5), and is one of the most common electrolyte abnormalities within the hospital environment.19 Management of hypokalemia involves replacement of K+ losses and treatment of the underlying disorder. To manage the associated Cl− deficit, K+ is given with Cl−. Caution is required in the administration of intravenous K+ because cardiac muscle is very sensitive to extracellular concentrations of this electrolyte.
Hyperkalemia (elevated serum potassium) is most common in patients with renal insufficiency (see Table 12-5). The primary treatment of hyperkalemia is restriction of K+ intake. The processes that precipitated the hyperkalemia also must be controlled. Temporary measures for reducing serum K+ levels include administration of insulin, calcium gluconate, sodium salts, or large volumes of hypertonic glucose. Cation exchange resins may be given orally or rectally. If these measures fail, peritoneal or renal dialysis can aid in K+ removal.
Calcium (Ca++)
Abnormal levels of calcium can cause various serious symptoms (see Table 12-5). Treatment of hypocalcemia (low serum levels of calcium) consists of correcting the underlying cause and replacing Ca++ either orally or intravenously. Hypercalcemia (increased levels of calcium) can result from numerous disorders. The most common causes are hyperparathyroidism and malignancies (e.g., multiple myeloma, lung cancer). Acute hypercalcemia requires emergency treatment because death may occur quickly if serum Ca++ increases to more than 17 mg/L (8.5 mEq/L). In such cases, there is usually an associated deficit of extracellular fluid. Volume replacement reduces serum Ca++ by dilution. Steroids and loop diuretics are sometimes helpful in reducing serum calcium.
Magnesium (Mg++)
Magnesium (Mg++) is the second most abundant intracellular cation after potassium. Magnesium plays an important role in cellular functions, including energy transfer; metabolism of protein, carbohydrate, and fat; and maintenance of normal cell membrane function (see Table 12-5). Systemically, magnesium decreases blood pressure and alters peripheral vascular resistance. Abnormalities of magnesium levels can result in disturbances in nearly every organ system and can cause potentially fatal complications (e.g., ventricular arrhythmia, coronary artery vasospasm, sudden death). Hypomagnesemia is also associated with multiple neuromuscular symptoms, such as muscular weakness, tetany, coma, and seizures. There is some evidence that intercellular magnesium levels may be related to bronchial hyperresponsiveness.
Phosphorus (P)
An average adult has approximately 1 kg (1000 g) of phosphorus, of which 80% to 90% is in bone and teeth in the form of apatite. The remaining phosphate is mostly present in the viscera and skeletal muscle, with a very small amount (<0.1%) in the extracellular fluids.20 Of this total, 10% to 17% is combined with proteins, carbohydrates, and lipids in muscle tissue and blood, and the remainder is incorporated into complex organic compounds. Only about 1% of the total body phosphorus is available as free serum compounds, so the serum level (1.2 to 2.3 mEq/L) does not reflect total body content. Serum phosphate levels are influenced by several factors (see Table 12-5), including the serum calcium concentration and the pH of blood.
Organic phosphate (HPO42−) is the main anion within cells with 20% present in the mitochondria. Approximately 30% of cellular phosphate is stored in the endoplasmic reticulum and is used in the phosphorylation of various proteins.20 Inorganic phosphate plays a primary role in the metabolism of cellular energy, being the source from which adenosine triphosphate is synthesized. In acid-base homeostasis, phosphate is the main urinary buffer for titratable acid excretion (see Chapter 13).