Chapter 162 Second-Generation Total Disc Arthroplasty
The three basic functions of the spinal column are to allow movement, transmit loads, and protect the neural elements. The functional spinal unit (FSU) as defined by White and Panjabi1 consists of a three-joint complex including the disc (end plate, anulus fibrosus, and nucleus pulposus) and the dorsal facet joints. More recently, the FSU has been redefined to include the passive and active constraints that are provided by the attached ligaments and muscles. The FSU performs as a continuous, semiconstrained joint, where complex three-dimensional movements occur. These movements, which occur through 6 degrees of freedom, include axial compression and distraction and anterior, posterior, and lateral bending, translation, and rotation. These movements are constrained by the need to protect neurologic elements and yet maintain the head balanced over the pelvis to facilitate interaction with the surrounding environment.
The current surgical “gold standard” strategy for treating symptomatic degeneration of the FSU is to stabilize the degenerated segment by fusing the affected spinal level. The goal of fusion is to restore the native anatomic relationships including disc height, lordosis, and coronal alignment, while relieving the patient’s painful symptoms. Surgical strategies have evolved from dorsal, dorsolateral, ventral, instrumented, and uninstrumented fusions to contemporary techniques involving interbody cages and synthetic and biologic bone substitutes. Despite this evolution, the results of fusion are less than perfect, with fusion rates ranging from 60% to 90% and clinical outcome improvement rates ranging from 60% to 70%.2 Spinal fusion also is associated with the longer-term problem of increasing the strain at the adjacent levels, potentially contributing to premature degenerative changes.3 To date, none of the increasingly complex implants and instruments has resulted in an improvement in the outcomes of fusion; with this in mind, similar to the evolution to arthroplasty from fusion of a peripheral joint, a philosophy of spine arthroplasty instead of spinal fusion is now evolving.
Spinal Biomechanics in the Normal and Degenerated Spine
Disc degeneration is part of the natural aging process, and it does not always result in painful conditions. Lee4 compiled a critical literature review of spinal biomechanics for the lumbar spine, describing the significance of the individual spinal elements and their impact on maintaining a healthy, functioning spine. Analysis of the data Lee compiled shows that providing motion is not the primary function of the native disc, but that passive resistance to the forces during motion is the more important factor to be considered when designing a strategy for long-term restoration of the FSU. Likewise, Ito et al.5 verified the notion of passive resistance by describing the nonlinear relationship between compressive load and disc stiffness. They reported low stiffness at lower loads (800 N/mm at 1000 N) and proportionately higher stiffness at higher loads (2000 N/mm at 4000 N). This differential allows for less resistance to motion at the lower loads of daily activities and more resistance to motion at the higher loads. This phenomenon presumably works to maintain spinal alignment and to protect the neurologic structures throughout the full range of motion.
Kimura et al.6 illustrated and quantified the effect of loading on the lumbar spine in eight volunteers. These investigators described the functional response of the lumbar spine and the ability of the spine to adapt to normal loading. Their key finding was that the spine when loaded first responds by increasing the native lordotic or kyphotic curvature and subsequently responds by altering disc height. These changes in disc height and the differential stiffness described by Ito et al.5 are reflections of the viscoelastic and biomechanical nature of the nucleus, anulus, and end-plate complex.
During development of the “follower load” biomechanical model, Patwardhan et al.7 defined the mechanism by which the spinal system attempts to maintain and reduce the localized shear stress on each intervertebral disc at or near zero during body movements. The shear stress, if left unchecked, presumably can cause intradiscal disruption and injury, possibly contributing to degeneration and mechanical dysfunction. Clinically, any disruption of an index FSU either ventrally or dorsally by injury, aging, or surgery may exaggerate the degeneration observed at the adjacent levels, as a result of the spinal system attempting to compensate for a compromised index FSU.
Kirkaldy-Willis8,9 schematically depicted the pathophysiology of degeneration of the lumbar spine as a degenerative cascade (Fig. 162-1). He described the three phases of degeneration as dysfunction, instability, and ultimately restabilization. Early degenerative events, such as disc herniation, lead to decreased stiffness of the spine. The natural response of the body is to resist abnormal stresses and strains with physiologic responses, such as osteophyte formation. Osteophytes are a manifestation of the body’s attempt to reduce the shear stress toward zero and to restore the load-bearing capacity of the FSU. The fact that the restoration of stability and load bearing may lead to other clinical pathologies (e.g., nerve compression and loss of motion) emphasizes the critical importance of stability and load bearing even at the expense of neurologic compromise and loss of flexibility.
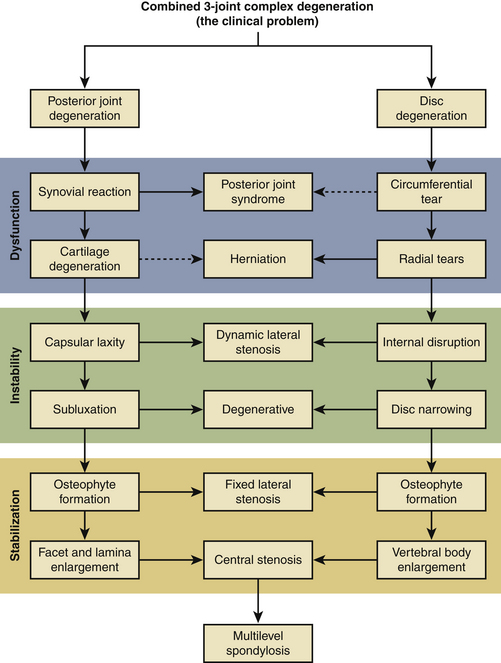
FIGURE 162-1 Kirkaldy-Willis degenerative cascade: pathophysiology of degenerative disease of the lumbar spine.
With the aforementioned in mind, any surgical intervention, especially disc arthroplasty, must take the biomechanical responses, the inherent disc function, and the degenerative cascade into account. Following the algorithm created by Kirkaldy-Willis,8,9 the body’s natural response to degenerative changes is to work toward stabilization, reducing motion through increased stiffness and osteophyte formation. It is imperative to match the proposed treatment to the stage of degeneration. If intervention on the degenerated disc uses a replacement prosthesis that introduces unnatural and unconstrained motion, it may reverse the process back toward the abnormally decreased stiffness phase.
First-Generation Artificial Discs
Over the last 3 decades, a considerable effort has been undertaken to develop alternatives to fusion. First-generation disc arthroplasty design evolved from experience with hip and knee replacement. These designs mimicked the ball-and-socket design used in artificial hips and knees. Although the first-generation devices may offer some benefit over fusion in that they reportedly preserve motion in the spinal segment, they do not function like the natural disc.10 These devices force a fixed axis of rotation on the spine and do not allow for true coupled motion.11 The natural disc provides for three-dimensional motion: flexion-extension, lateral bending, rotation, and compression. It is also viscoelastic, meaning that the stiffness varies with the loading rate and magnitude.5 The first-generation discs restore only two-dimensional motion, providing no mechanism for axial compression, and they have no viscoelastic properties. Although they may prove successful in replacing a painful, degenerated disc with some motion, they cannot replicate the native function of the natural disc. These shortcomings are more than just theoretical limitations and are likely to lead to problems and disappointing long-term results.
Definition of the Ideal Artificial Lumbar Intervertebral Disc
Spinal motion does not rely solely on the intervertebral disc. Any prosthetic replacement should offer more than one dimension of function and should not act in isolation from the native biomechanics of the spine. Lemaire suggested that the ideal substitute for the lumbar disc “… should meet the following criteria: geometry, motion, deformability, inherent stiffness, and acoustics.”11
The hallmark of a next-generation design is that it should replicate native function, embracing the four key biomechanical concepts: complex motion, viscoelasticity, load-bearing capacity, and passive resistance to loads. The load-bearing capability of the spine is also a function of anatomy and alignment. Kimura et al.6 illustrated and quantified the effect of loading on the lumbar spine, describing the functional response of the spine and its ability to adapt to normal loading. The key finding was that the spinal system responds to loading first by increasing the native lordotic or kyphotic curvature and subsequently by altering disc height. This finding highlights the fact that the spinal response to motion is a system-wide response and not just local to the segments.
Design of the Ideal Artificial Lumbar Intervertebral Disc
Biomechanical Characterization
An artificial disc should be thoroughly biomechanically characterized to show its strength and durability. The device should have strength that exceeds the strength of the natural disc and surrounding anatomy in compression, rotation, shear, flexion, and extension. Because axial compression is the primary load-bearing mode in an artificial disc, compressive strength should be of the utmost importance. Durability evaluations include fatigue testing in compression, rotation, flexion-extension, and lateral bending to determine the endurance limits, failure modes, and potential for wear debris generation. Additionally, coupled motion fatigue testing may be used to predict more physiologically realistic loading scenarios and resulting failure modes. Device creep and resilience are also important properties to characterize to predict long-term performance. Device stiffness and range of motion should also be characterized and compared with a healthy natural disc. Stiffness and range of motion may be characterized statically and dynamically in compression, rotation, and flexion-extension. The propensity for subsidence of the device into the vertebral bodies or expulsion of the device from the disc space should be evaluated. Testing in simulated vertebral bodies made of polyurethane foam eliminates the variability associated with bone specimens. For multiple-component bonded devices, extensive bond strength and durability testing should be conducted. Evaluations of the bond should be a part of the testing described previously or, alternatively, done as separate investigations, using nonphysiologic tests designed to focus the stress on the bond.
Early Polymeric Total Disc Replacement Experience
Clinical experience with polymeric discs began with Steffee, the inventor of the AcroFlex lumbar disc. Three generations of the AcroFlex lumbar disc (DePuy Spine, a Johnson & Johnson company, Raynham, MA) were used in several pilot studies or custom implantations. All three generations of the device incorporated titanium alloy end plates and an elastomeric core bonded to the metal plates. The first-generation disc incorporated a hexane-based polyolefin rubber and adhesive system and was implanted in six patients.12 Satisfactory results (one excellent, two good, and one fair) occurred in four of the six patients at 3 years of follow-up. The second-generation device, incorporating a silicone elastomer core, was approved by the U.S. Food and Drug Administration (FDA) in 1993 for implantation into 13 patients, but results have not been published.13
The third-generation AcroFlex lumbar artificial disc also used a hexane-based polyolefin rubber and adhesive. These discs had either flat end plates with a raised crescent or slightly domed end plates with six teeth for short-term fixation to bone.14 Both third-generation discs had a sintered bead coating for bony ingrowth. Two prospective, nonrandomized pilot studies were conducted with the third-generation AcroFlex disc.14 Although the overall clinical results up to 2 years appeared to be satisfactory, planned randomized studies were not carried out for the third-generation AcroFlex disc, because of the finding of mechanical failures in the elastomer cores.
Implants
All second-generation disc arthroplasty devices are elastomeric-based systems employing some form of compliant polymer. The characteristic of compression (compliance to loading) is the demonstrable difference between first-generation and second-generation total disc arthroplasty. The predominant lumbar next-generation devices include the following (Fig. 162-2):
• CADisc (Ranier, Cambridge, UK)
• eDisc (Integra Life Science, Plainsboro, NJ)
• Freedom (AxioMed Spine Corp, Cleveland, OH)
• M6 (Spinal Kinetics, Sunnyvale, CA)
CAdisc
The CAdisc (Compliant Artificial Spinal Disc) has no articulating surfaces, and it is an entirely elastomeric, polyurethane polycarbonate graduated modulus device with integrated end plates. The “anulus” is harder (higher modulus), and the “nucleus” is softer (low modulus), with a region of continuously varying modulus joining the anulus and nucleus. The device is manufactured using proprietary technology, a computer-controlled polymerization of polycarbonate polyurethanes, integrated with the molding process to achieve the graduated modulus design. The disc is covalently bonded throughout, with “no regions of high stress concentration.” A company-sponsored report in a baboon study showed that the implants did not migrate or subside after implantation for 6 months in baboons.15 The disc left impressions in the vertebral end plates, and micro-CT showed “evidence suggestive of positive bone remodeling.”
eDisc
The eDisc comprises a polycarbonate urethane core and titanium end plates, with a microelectronic module. The polymer, PH200, developed by Theken, is reported to have superior crack growth resistance, greater hydrolytic stability, and enhanced oxidation resistance.16 The microelectronic module provides treating physicians with feedback allowing them to manage patients’ postoperative courses.
Freedom Lumbar Disc
The Freedom disc is a one-piece viscoelastic artificial disc consisting of an elastomeric core bonded to titanium alloy retaining plates with end caps. The polymer core material is a silicone polycarbonate urethane thermoplastic elastomer. The polymer core is able to expand radially and axially. This axial feature, along with the mechanical characteristics of the polymer, is intended to allow the device to approximate the stiffness of a natural human disc. The manufacturer presented results of 50 patients with single-level, symptomatic lumbar degenerative disc disease at L4-5 or L5-S1, unresponsive to at least 6 months of nonoperative therapy.17 Mean preoperative Oswestry Disability Index scores decreased from 48% preoperatively to 24% at 2-year follow-up. Mean visual analogue scale scores decreased from preoperative 7.1 to 2.7 cm at 2 years for back pain, 3.8 to 0.3 cm for right leg pain, and 3.7 to 1.7 cm for left leg pain.
M6
Spinal Kinetics developed the M6 cervical total disc replacement and M6 lumbar total disc replacement. The intent of M6 is to “replicate the anatomic and biomechanical attributes of a natural intervertebral disc” via an artificial nucleus and anulus. The nucleus allows axial compression, whereas the woven fiber anulus controls range of motion in all 6 degrees of freedom.18
A single-arm prospective feasibility study was conducted to evaluate the preliminary safety and effectiveness of the M6 disc in the treatment of patients with symptomatic cervical radiculopathy.19 The study enrolled 32 cases, with 15 patients reaching 12 months of follow-up; 10 patients underwent artificial disc implantation at one level, and 5 patients had two levels treated. The mean Neck Disability Index (NDI) score improved from 48.1% before treatment to 20.4% at 12 months. The mean arm pain score improved from 6.8 before treatment to 3.2 at 12 months. The mean neck pain score improved from 7.2 before treatment to 3.5 at 12 months. The mean Physical Component Summary and Mental Component Summary scores of the 36-Item Short Form Health Survey improved from 34.7 and 43.6 before treatment to 44.6 and 50.4 at 12 months. All the improvements were statistically significant. Using dynamic flexion-extension radiographs, the mean range of motion at the treated level was 12.3 degrees before surgery and 9.7 degrees at 12 months. Mean global range of motion of the entire neck was approximately 47 degrees before treatment and returned to this value at 12 months. Disc height at the treated level was 3.4 mm before surgery, improved to approximately 6 mm, and remained constant through 12 months of follow-up. No cases of worsening neurologic status were reported, and there were no serious device-related adverse events; reoperations; or evidence of device migration, expulsion, or subsidence in this study group.
NeoDisc
The NeoDisc comprises an elastomeric nucleus contained within an embroidered polyester fabric, secured by four bone screws. The endurance limit of the NeoDisc implant under 500 N dynamic axial compression was determined to be at least 10 million cycles.20 Static axial compression to half of the core height did not cause damage or failure of the NeoDisc implant. At 20 million cycles, the average volumetric loss of material from the NeoDisc device was 13.8 mg (approximately 11.2 mm3); this corresponds to an average of 0.69 mg per 1 million cycles of testing (0.56 mm3 per 1 million cycles). The 20 million–cycle test can be considered to be a worst-case test.20 The investigators concluded that it is unlikely that any device in clinical use would experience this quantity of large, exaggerated motion. Animal trials provided macroscopic and histologic evidence of the tissue ingrowth.20 There were no unforeseen causes of wear debris, and the tissue response to the wear debris generated was analogous to the tissue response of other medical devices.
Conclusion
The next generation of disc replacements must more closely replicate the biomechanical properties of the normal human disc. Elastomeric materials, by virtue of their viscoelastic properties, can closely approximate the native properties of the human disc. These next-generation materials must conform to toxicology and wear standards. Because these devices are mainly used in younger individuals, bonding between the viscoelastic material and the retaining plates has to withstand millions of cycles. Because implant displacement can result in early failure, it is of paramount importance to make the most adaptable design. Device insertion should be easy and reproducible, and catastrophic failure prevention and contingency management plans should be in place.
Cunningham B. Basic scientific considerations in total disc arthroplasty. Spine J. 2004;4(Suppl 6):219S-230S.
Eck J., Humphreys S., Hodges S. Adjacent-segment degeneration after lumbar fusion: a review of clinical, biomechanical and radiologic studies. Am J Orthop. 1999;28:336-340.
Enker P., Steffee A., Mcmillin C., et al. Artificial disc replacement: preliminary report with a 3-year minimum follow-up. Spine (Phila Pa 1976). 1993;18:1061-1070.
Fritzell P., Hagg O., Wessberg P. Lumbar fusion versus nonsurgical treatment for chronic low back pain: a multicenter randomized controlled trial from the Swedish Lumbar Spine Study Group. Spine (Phila Pa 1976). 2001;26:2521-2532.
Yong-Hing K., Kirkaldy-Willis W. The pathophysiology of degenerative disease of the lumbar spine. Orthop Clin North Am. 1983;14:491-504.
1. White A., Panjabi M. Clinical biomechanics of the spine, ed 2, Philadelphia: Lippincott, 1990.
2. Fritzell P., Hagg O., Wessberg P. Lumbar fusion versus nonsurgical treatment for chronic low back pain: a multicenter randomized controlled trial from the Swedish Lumbar Spine Study Group. Spine (Phila Pa 1976). 2001;26:2521-2532.
3. Eck J., Humphreys S., Hodges S. Adjacent-segment degeneration after lumbar fusion: a review of clinical, biomechanical and radiologic studies. Am J Orthop. 1999;28:336-340.
4. Lee R. Kinematics of the lumbar spine (website). www.rs.polyu.edu.hk/RLee/Spine/LumbarKin/.
5. Ito M., Tadano S., Kaneda K. A biomechanical definition of spinal segmental instability taking personal and disc level differences into account. Spine (Phila Pa 1976). 1993;18:2295-2304.
6. Kimura S., Steinbach G., Watenpaugh D., et al. Lumbar spine disc height and curvature responses to an axial load generated by a compression device compatible with magnetic resonance imaging. Spine (Phila Pa 1976). 2001;26:2596-2600.
7. Patwardhan A., Havey R., Meade K., et al. A follower load increases the load-carrying capacity of the lumbar spine in compression. Spine (Phila Pa 1976). 1999;24:1003.
8. Kirkaldy-Willis W., Bernard T. The three phases and three joints. In In: Managing low back pain, ed 4, Philadelphia: Churchill Livingstone; 1999:249-262.
9. Yong-Hing K., Kirkaldy-Willis W. The pathophysiology of degenerative disease of the lumbar spine. Orthop Clin North Am. 1983;14:491-504.
10. Cunningham B. Basic scientific considerations in total disc arthroplasty. Spine J. 2004;4(Suppl 6):219S-230S.
11. O’Leary P., Nicolakis M., Lorenz M. Response of Charite total disc replacement under physiologic loads: prosthesis component motion patterns. Spine J. 2005;5:590-599.
12. Enker P., Steffee A., Mcmillin C., et al. Artificial disc replacement: preliminary report with a 3-year minimum follow-up. Spine (Phila Pa 1976). 1993;18:1061-1070.
13. Traynelis V., Haid R.. www.spineuniverse.com.
14. Fraser R.D., Ross E.R., Lowery G.L., et al. AcroFlex design and results. Spine J. 2004;4(Suppl 6):245S-251S.
15. Martin S., Steffen T., Aebi M., et al. A radiographic evaluation of the in vivo stability of CAdisc-L©, a polyurethane compliant artificial total lumbar disc replacement (TDR) device in the baboon. Miami, FL: Spine Arthroplasty Society Annual Meeting; 2008.
16. Navarro R., Theken R., Ananthan R., et al. Theken eDisc: a second-generation lumbar artificial disc. In: Yue J.J., Bertagnoli R., McAfee P.C., editors. Motion preservation studies of the spine: advanced techniques and controversies. Philadelphia: Elsevier, 2008.
17. Lieberman I., Ross ERS I., Benzel E., Linovitz R. The results of a pilot study with a second generation total lumbar disc replacement. London: Spine Arthroplasty Society Annual Meeting; 2009.
18. Reyes-Sánchez A.A., Patwardhan A.G. The M6 artificial cervical disc. In: Yue J.J., Bertagnoli R., McAfee P.C., An H., editors. Motion preservation studies of the spine: advanced techniques and controversies. Philadelphia: Elsevier., 2008.
19. Reyes-Sanchez A., Miramontes V., Olivrez L.R., et al. The M6 artificial cervical disc. In: Yue J.J., Bertagnoli R., McAfee P.C., editors. Motion preservation studies of the spine: advanced techniques and controversies. Philadelphia: Elsevier, 2008.
20. Jackowski A., McLeod A., Reah C., et al. The NeoDisc Elastomeric Cervical Total Disc Replacement. In: Yue J.J., Bertagnoli R., McAfee P.C., et al, editors. Motion preservation studies of the spine: advanced techniques and controversies. Philadelphia: Elsevier, 2008.