Chapter 4 Retina
The innermost coat of the eye is a neural layer, the retina, located between the choroid and the vitreous. It includes the macula, the area at the posterior pole used for sharpest acuity and color vision. The retina extends from the circular edge of the optic disc, where the nerve fibers exit the eye, to the ora serrata. It is continuous with the epithelial layers of the ciliary body, with which it shares embryologic origin. The retina is derived from neural ectoderm and consists of an outer pigmented layer, derived from the outer layer of the optic cup, and the neural retina, derived from the inner layer of the optic cup (see Chapter 7). The pigmented layer is tightly adherent to the choroid throughout, but the neural retina is attached to the pigmented epithelium and thus to the choroid only in a peripapillary ring around the disc and at the ora serrata. Although it contains millions of cell bodies and their processes, the neural retina has the appearance of a thin, transparent membrane.
The retina is the site of transformation of light energy into a neural signal. It contains the first three cells (photoreceptor, bipolar, and ganglion) in the visual pathway, the route by which visual information from the environment reaches the brain for interpretation. Photoreceptor cells transform photons of light into a neural signal through the process of phototransduction, then transfer this signal to bipolar cells, which in turn synapse with ganglion cells, which transmit the signal from the eye. Other retinal cells—horizontal cells, amacrine cells, and interplexiform neurons—modify and integrate the signal before it leaves the eye. This chapter discusses these cells and the detailed anatomy of the retina; the remainder of the visual pathway is described in Chapter 13.
Retinal Histologic Features
Under light microscopy, the retina has a laminar appearance in which 10 layers are evident (Figure 4-1). Closer examination reveals that these are not all “layers,” but rather a single layer of pigmented epithelium and three layers of neuronal cell bodies, between which lie their processes and synapses. This section describes the epithelial layer and the types and functions of the neural cells; the next section discusses the components of each of the 10 so-called retinal layers.
Retinal Pigment Epithelium
The retinal pigment epithelium (RPE), the outermost retinal layer, is a single cell thick and consists of pigmented hexagonal cells. These cells are columnar in the area of the posterior pole and are even longer, narrower, and more densely pigmented in the macular area.1 The cells become larger and more cuboidal as the layer nears the ora serrata, where the transition to the pigmented epithelium of the ciliary body is located. Because of the orientation of the embryologic cells, the basal aspect of the cell is adjacent to the choroid and the apical surface faces the neural retina. The basal aspect contains numerous infoldings and is adherent to its basement membrane, which forms a part of Bruch’s membrane of the choroid; therefore, its attachment to the choroid is strong. Despite its close association with the choroid, the RPE is considered a part of the retina because it is from the same embryologic germ cell layer—neural ectoderm.
The RPE cells contain numerous melanosomes, pigment granules, that extend from the apical area into the middle portion of the cell and somewhat obscure the nucleus, which is located in the basal region. Pigment density differs in various parts of the retina and in individual cells, which can give the fundus a mottled appearance when viewed with the ophthalmoscope. In the retina, melanin is densest in the RPE cells located in the macula and at the equator.2 Other pigmented bodies, lipofuscin granules, contain degradation products of phagocytosis, which increase in number with age.1,3–7 The cell cytoplasm also contains smooth and rough endoplasmic reticulum, Golgi apparatus, mitochondria, and numerous lysosomes.
The apical portion of an RPE cell consists of microvilli that extend into the layer of photoreceptors, enveloping the specialized outer segment tips (Figure 4-2). However, no intercellular junctions connect the RPE and photoreceptor cells. A potential space separates the epithelial cell and the photoreceptor.1 This subretinal space8 is a remnant of the gap formed between the two layers of the optic cup after invagination of the optic vesicle (see Chapter 7).
Terminal bars consisting of zonula occludens and zonula adherens join the RPE cells near their apices.1 Desmosomes are present throughout the layer, and gap junctions between the cells allow for electrical coupling, providing a low-resistance pathway for the passage of ions and metabolites.9
Photoreceptor Cells
Retinal Pigment Epithelium-Neuroretinal Interface
Several factors are involved in maintaining the close approximation between the photoreceptor cell layer and the RPE layer. Passive forces, such as intraocular pressure (IOP), osmotic pressure,10–12 fluid transport across the RPE,13,14 and presence of the vitreous,15 help preserve the position of the neural retina. Interdigitations between the RPE microvilli and the rod and cone outer segments provide a physical closeness between the two entities.1 The material that occupies the extracellular space between the two layers likely provides adhesive forces.16–18
An unstructured, amorphous material was once thought to occupy the extracellular space between the RPE cells and the photoreceptors, and to surround the outer and inner segments of the photoreceptors. More recent studies have described an organized honeycomb-like structure, the interphotoreceptor matrix (IPM), which is composed of proteins and glycosaminoglycans.19 The outer segments are surrounded completely by the IPM and extend through openings in its meshwork. The constituents of the IPM domains around rods differ from those around cones; those surrounding cones are described as a “matrix sheath.”19–21 The domains are believed to be bound together laterally, forming a highly coherent structural unit.19 The IPM constituents are bound tightly to both the epithelial cells and the photoreceptor cells, and may exceed the strength of the RPE cells. In laboratory experiments, a forceful separation between these two layers often ruptures the RPE cell, leaving remnants of pigment attached to the photoreceptors.3,22 The adhesive mechanism is attributed to molecular bonds within this extracellular material.16,18,22–24
The IPM provides a means for the exchange of metabolites25 and for interactions between the two layers.26 In addition, the IPM may be partly responsible for orienting the photoreceptor outer segments for optimal light capture.17 Despite these apparent forces, the interface between the RPE and the photoreceptor layer is the common location of separation in a retinal detachment.
Clinical Comment: Retinal Detachment
When a retinal detachment occurs, the separation usually lies between the RPE cells and the photoreceptors because no intercellular junctions join these cells. The RPE cells remain attached to the choroid and cannot be separated from it without difficulty. Bruch’s membrane contains fibronectin and laminin, large adhesive glycoproteins with many binding sites that help maintain the adherence of RPE cells to the membrane.27 Fluid can accumulate within the subretinal space separating the photoreceptors from the nutrients supplied by the choroid; if the layers are not repositioned quickly, the affected area of photoreceptor cells will necrose. An argon laser often is used to photocoagulate the border of a detachment, producing scar tissue. These act like a “spot weld” and prevent the detachment from enlarging and facilitates the repositioning of the photoreceptors.
Composition of Rods and Cones
Rods and cones are composed of several parts, starting nearest the RPE: (1) the outer segment, containing the visual pigment molecules for the conversion of light into a neural signal; (2) a connecting stalk, the cilium; (3) the inner segment, containing the metabolic apparatus; (4) the outer fiber; (5) the cell body; and (6) the inner fiber, which ends in a synaptic terminal (Figure 4-3).
Outer Segment
The outer segment is made up of a stack of membranous discs (600 to 1000 per rod)2 and is enclosed by the plasmalemma of the cell. Each disc is a flattened membrane sac with a narrow intradisc space; the discs are stacked on top of one another and are separated by an extradisc space. Visual pigment molecules are located within the disc membrane. A biochemical change is initiated within these molecules when they are activated by a photon of light. The tip of the outer segment is oriented toward the RPE, and the base is oriented toward the inner segment.
Cilium
A connecting stalk, or cilium, extends from the innermost disc, joining the outer segment with the inner segment and acting as a conduit between them (Figure 4-4). It is a modified cilium consisting of a series of nine pairs of tubules from which the central pair, usually present in motile cilia, is missing. The plasmalemma around the outer segment is continuous across the cilium with that of the inner segment.
Inner Segment
The inner segment contains cellular structures and can be divided into two parts. The ellipsoid is nearer the outer segment and contains the numerous mitochondria necessary for the many energy-dependent photoreceptor processes. The part closer to the cell body, which is sometimes called the myoid, contains other cellular organelles, such as the endoplasmic reticulum and Golgi apparatus; protein synthesis is concentrated in this area.28 The term “myoid,” however, is derived from a similar area in amphibians that contains a contractile structure that produces orientational movements of the outer segments of the cones.29 The human myoid does not have contractile properties,30 although the axis of the inner and outer segments is oriented toward the exit pupil of the eye, maximizing the ability of the photoreceptor to capture light. The radial orientation becomes more evident in cells located farther from the macula.8,31,32
Rod and Cone Morphology
Rods
The plasmalemma, enclosing the rod outer segment, is separate from the disc membrane except for a small region at the base where invaginations of the plasmalemma form discs, the intradisc space of which is continuous with the extracellular space (see Figure 4-3, A). The remainder of the discs form sacs that are closed at both ends and are free of attachment to the surrounding membrane and adjacent discs.8,33 The discs are fairly uniform in width, and the photosensitive pigment rhodopsin is located within the disc membrane.
Young28 applied a pulse of radiolabeled amino acids, which were incorporated into the disc components. He observed that the band of radioactivity moved from the inner segment, where protein is synthesized, into newly assembled discs in the outer segment. The labeled discs moved from the base to the tip, and the label was finally seen in phagosomes of the RPE cells. This study established that components of disc membranes are produced in the inner segment and move along the connecting stalk to be incorporated into discs at the outer segment base.28 The discs gradually are displaced outward by the formation of new discs and, as they reach the tip, are sloughed off, taken up by the RPE cells, and phagocytosed.34,35 This process, the rod outer segment renewal system, appears to involve active processes in both the RPE and the outer segment.28,36 The discs apparently are shed regularly, with most of the shedding occurring in the early morning.37–40
The rod inner and outer segments are approximately the same width. The inner segment is joined to the cell body by the relatively long and narrow outer fiber. The inner fiber extends from the cell body and terminates in a rounded, pear-shaped structure called a spherule (see Figure 4-3, A). The internal surface of the spherule is invaginated forming a synaptic complex that contains bipolar dendrites and horizontal cell processes.41,42 Rods release the neurotransmitter glutamate.
Cones
As in the rod, the outer segment of the cone is enclosed by a plasmalemma, but in this case the plasma membrane is continuous with the membranes forming most of the discs, and the discs are not separated easily from one another43 (Figure 4-5). In many cones the discs at the base are wider than those at the tip, giving the characteristic cone shape, although some cone outer segments have a shape similar to the rod.
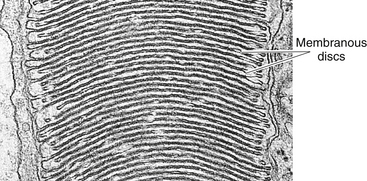
FIGURE 4-5 Cone outer segment. (Transmission electron microscope; ×56,000.)
(From Krause WJ, Cutts JH: Concise text of histology, Baltimore, 1981, Williams & Wilkins.)
The cone outer segment is shorter than that of the rod and may not reach the RPE layer. However, tubular processes protrude from the apical surface of the epithelial cell to surround the cone outer segment.44 One of three visual pigment molecules is contained within the disc membrane, and each pigment molecule is activated by the absorption of light in a specific range in the color spectrum. The peak absorptions occur at 420 nm (blue), 531 nm (green), and 588 nm (red).
Early studies of cone outer segment renewal theorized that the formation of discs at the cone base was not uniform and sequential because radiolabeled protein appeared to be distributed throughout the outer segment membrane, not merely in the disc membranes.35,45 More recently, electron-microscope studies suggest that formation of new discs does occur at the base, but because of the more extensive connections with the surrounding plasmalemma, the labeled molecules are able to diffuse throughout the cone outer segment membrane rather than being confined to discs, as occurs in the rods.43 Investigations continue into the mechanism by which the discs become narrower as they approach the tip.46 Cone discs too are shed periodically, often at the end of the day, and are phagocytosed by the RPE.40,43,47,48 The factors that regulate the cycle of disc shedding are still under investigation.
The shape of the inner segment also contributes to the cone shape. The ellipsoid area of the cone is wider and contains more mitochondria than the rod.1 The outer fiber is short and stout and may even be absent in the cone; thus, cone nuclei lie outer to rod nuclei. The inner fiber terminates in a broad, flattened structure called a pedicle, which has several invaginated areas within its flattened surface (see Figure 4-3, A). Cone pedicles have three types of synaptic contacts. Triads are found within the invaginations; contacts with bipolar cells occur on the flat surfaces; and gap junctions are located on the lateral expansions (telodendria) of the pedicle and permit electrical communication between adjacent rods or cones.8,49
As with rods, the neurotransmitter released by cones is glutamate.
Bipolar Cells
The bipolar cell is the second-order neuron in the visual pathway. The nucleus of the bipolar cell is large and contains minimal cell body cytoplasm. Its dendrite synapses with photoreceptor and horizontal cells, and its axon synapses with ganglion and amacrine cells; glutamate is its neurotransmitter. Bipolar cells relay information from photoreceptors to horizontal, amacrine, and ganglion cells and receive extensive synaptic feedback from amacrine cells.50 Their dendrites also have contact with interplexiform neuron processes.51 Eleven types of bipolar cells have been classified on the basis of morphology, physiology, and dendritic contacts with photoreceptors;51 all types except the rod bipolar cell are associated with cones.
Only one type of rod bipolar cell has been identified. It has a relatively large body and several spiky dendrites, usually arising from a single, thick process. Rod bipolar cells begin to appear 1 mm from the fovea and continue into the periphery. These are the only bipolar cells that contact rods.1,51 The expanse of the dendritic tree widens and the reach of the axonal terminals increases in the rod bipolar cells located in peripheral retina compared with those in central retina. The dendrites of a single rod bipolar cell contact 15 to 20 rods in central retina and up to 80 rods in the periphery, improving sensitivity.51,52 Often two dendrites lie within the spherule invagination flanked by two horizontal cell processes (Figure 4-6). The rod bipolar axon is large and unbranched, and rarely synapses directly with ganglion cells but instead synapses with amacrine processes, which then signal ganglion cells.53 This synaptic arrangement allows a ganglion cell to carry information from both the rod and the cone pathway.53
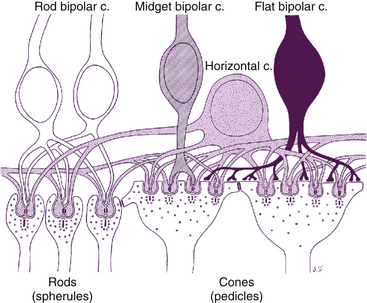
FIGURE 4-6 Rod spherule and cone pedicle and their synapses.
(From Hogan MJ, Alvarado JA, Weddell JE: Histology of the human eye, Philadelphia, 1971, Saunders.)
The midget bipolar cell has a relatively small body and can be either flat or invaginating. Dendritic terminals of the flat midget bipolar cell end in a flat expansion and make contact only with the flat area of the cone pedicle. In central retina, each flat midget bipolar cell contacts only one cone so its dendritic “bouquet” is small—the size of a single cone pedicle. In peripheral retina, each flat midget bipolar cell has two or three dendritic clusters and thus each cell contacts two or three neighboring cones.51,54 A single cone pedicle may have as many as 500 contacts on its flat surface.2 The axon of the flat midget bipolar cell has many endings and synapses with ganglion cells of all types.1
The invaginating midget bipolar cell is similar to the flat midget bipolar cell, but its dendritic processes are located within the pedicle invaginations, usually in groupings called a triad. A triad consists of a single central bipolar dendrite flanked by two horizontal cell processes within an invagination in the cone pedicle (see Figure 4-6). In central retina, the dendritic bouquet of an invaginating midget bipolar cell is the size of a single cone pedicle, implying that each cone is innervated by only one invaginating bipolar cell. Each pedicle can have 12 to 25 triads.2 In peripheral retina, each bipolar cell may have up to three such dendritic expansions, with the capacity to contact several pedicles.51 The axon of the invaginating midget bipolar cell synapses with the dendrite of a single midget ganglion cell and with amacrine processes.1
The two types of diffuse cone bipolar cells are designated type a and type b, called “flat bipolars” and “brush bipolars” by Polyak.55 In the central retina, the diffuse cone bipolar cell contacts approximately five neighboring cones, and in the periphery, each contacts 10 to 15 neighboring cones. The location of the axon terminal differentiates the two types.51
The blue cone bipolar cell synapses with up to three cone pedicles.56 It differs from diffuse cone bipolar cells in that it contacts widely spaced rather than neighboring cones.51
The giant cone bipolar cell derives its name from the extent of its dendritic tree. The major dendrite branches into three trees, and then clusters of processes branch from these, each group being the size of a cone pedicle. The two types, designated diffuse and bistratified, differ only in the location of their axon terminations.51,57
Figure 4-7 shows several of the bipolar cell types.
Ganglion Cells
The next cell in the visual pathway, the third-order neuron, is the ganglion cell. Ganglion cells can be bipolar (e.g., a single axon and a single dendrite) or multipolar (a single axon and more than one dendrite).58 Cell size varies greatly, with some large cell bodies measuring 28 to 36 μm.51 The various methods used to classify ganglion cells make classification rather confusing.
An older, broad classification groups ganglion cells into three types. W cells project to the midbrain, carrying information for the pupillary response and reflexive eye movements. Y cells project to the lateral geniculate nucleus (LGN), with some having collateral branches that travel to the midbrain, perhaps with pupillary information. X cells primarily respond in visual discrimination and project to the LGN.2
Ganglion cells have also been classified on the basis of cell body size, branching characteristics, termination of dendrites, and the expanse of the dendritic tree.51 Figure 4-8 shows several types of ganglion cells, both central and peripheral. These ganglion cell types are designated G3 to G23 (not all numbers are represented).
Another designation classifies ganglion cells based on the lateral geniculate nucleus layer in which they terminate. P cells terminate in the parvocellular layers. The P1 ganglion cell, also called the midget ganglion cell, is the most common P cell. This relatively small cell has a single dendrite and can be differentiated into two types, according to the stratification of the dendritic branching.51 Certain P1 midget cells are connected to only one midget bipolar cell, invaginating or flat, which in turn might be linked to a single cone receptor,59 providing a channel that processes high-contrast detail and color resolution. This situation is likely to occur in the fovea. A convergent pathway occurs in some P1 cells that receive input from two bipolar axons.
The P2 ganglion cell also terminates in the parvocellular layers but has a densely branched, compact dendritic tree that spreads horizontally. These cells can be differentiated into two types depending on the location of the dendrite termination.51
The M-type ganglion cell projects to the magnocellular layers of the LGN. The M cell has coarse dendrites (because of its shape can also be called a parasol ganglion cell) with spiny features, and the dendritic tree enlarges from central to peripheral retina.51
Each ganglion cell has a single axon, which emerges from the cell body and turns to run parallel to the inner surface of the retina; the axon releases glutamate at its synaptic cleft. The axons come together at the optic disc and leave the eye as the optic nerve. The termination for approximately 90% of these axons is the lateral geniculate nucleus; the other 10% project to subthalamic areas involved in processes such as the pupillary reflexes and the circadian rhythm.2,51
The photoreceptor cells, bipolar cells, and ganglion cells carry the neural signal in a three-step pathway through the retina. The neural signal is modified within the retina by other cells that create intraretinal cross-connections, provide feedback information, or integrate retinal function.1
Horizontal Cells
The horizontal cell transfers information in a horizontal direction parallel to the retinal surface. It has one long process, or axon, and several short dendrites with branching terminals; the processes spread out parallel to the retinal surface, and all terminate in the outer plexiform layer (Figure 4-9). Horizontal cells synapse with photoreceptors, bipolar cells, and other horizontal cells. Horizontal cells are joined to each other by an extensive network of gap junctions. One type of horizontal cell synapses only within a cone pedicle in the special triad junction. Horizontal cells can contact bipolar cells lying some distance from the photoreceptor that activated the horizontal cell. Horizontal cells can effect an inhibitory response, thus playing a role in the complex process of visual integration.57,60
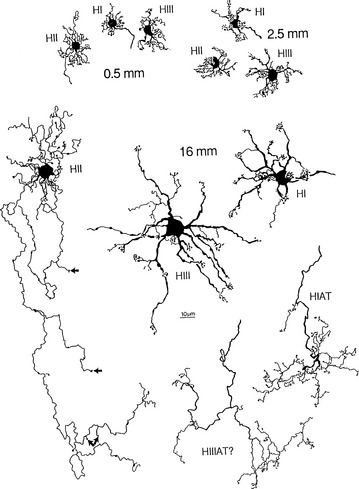
FIGURE 4-9 Whole-mount views of horizontal cells of human retina (scale bar = 10 mm).
(From Kolb H, Linberg KA, Fisher SK: Neurons of the human retina: a Golgi study, J Comp Neurol 318:147, 1992.)
Three types of horizontal cells have been differentiated: HI, HII, and HIII. HI cells have dendrites that synapse with 7 to 18 cones as lateral elements in triads and a large, thick axon ending in a fan-shaped expanse of terminals that end in rod spherules more than 1 mm away.61 All the HII processes (dendrites and axons) apparently contact cones and might be specific for blue cones.51 HIII cells have a large dendritic tree that synapses with many cones, (9 to 12 in the macular area and 20 to 25 in the periphery) not all of which are neighboring; evidence suggests that these horizontal cells avoid blue cones, thus being selective for red and green.51,62 The termination of the HIII axon has not yet been determined but probably contacts both rods and cones.60 Horizontal cells provide inhibitory feedback to photoreceptors or inhibitory feed forward to bipolar cells.61 Horizontal cells can modulate the cone response but are not thought to influence that of the rod.53,61
Amacrine Cells
The amacrine cell has a large cell body, a lobulated nucleus, and a single process with extensive branches that extend into the inner plexiform layer. The process, which has both dendritic and axonal characteristics and carries information horizontally, forms complex synapses with axons of bipolar cells, dendrites and the soma of ganglion cells; with processes of interplexiform neurons; and with other amacrine processes.1,63 Because of the extremely broad spread of its process, the amacrine cell plays an important role in modulating the information that reaches the ganglion cells.57
As many as 30 to 40 different amacrine cell types may be described as stratified or diffuse. They can also be classified into four groups—narrow field, small field, medium field, and large field—according to the extent of coverage by their intertwined branching processes. Each of these groups can be subdivided into different types according to the level of the retinal layer in which their nerve endings terminate.51,64
One of the most widely studied amacrine types is the AII cell (that is Roman numeral 2), a narrow-field type (Figure 4-10). The AII cells are the conduit by which the rod signal reaches ganglion cells.64 An AII cell may receive input from as many as 300 rods through 80 rod bipolar cells.31 The AII cell then synapses with a ganglion cell and may also relay information from the rod pathway to the cone pathway.
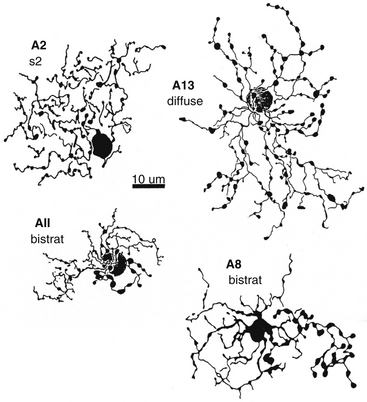
FIGURE 4-10 Whole-mount view of amacrine cells.
(From Kolb H: Amacrine cells of the mammalian retina: neurocircuitry and functional roles, Eye 11:904, 1997.)
Wide-field amacrine (A17) cells form reciprocal synapses with rod bipolar cells and appear to modify the signal transmitted from rod bipolar to AII cells.51 Most amacrine cells contain the inhibitory neurotransmitter gamma-aminobutyric acid (GABA) or glycine, and have both presynaptic and postsynaptic endings.2,64 Amacrine cells are joined to one another via gap junctions,64 and some cells have been found to combine information from rod and cone pathways before innervating a ganglion cell.59
Interplexiform Neurons
The interplexiform neuron has a large cell body and is found among the layer of amacrine cells. The processes extend into both synaptic layers and convey information between these layers (Figure 4-11), apparently providing feedback from inner to outer retinal layers.51,57 Some of their nerve endings are presynaptic and some are postsynaptic to amacrine processes or amacrine cell bodies in the inner plexiform layer. Interplexiform neurons are presynaptic to rod or cone bipolar cells in the outer plexiform layer.2,8,65
Neuroglial Cells
Müller Cells
Müller cells are large neuroglial cells that extend throughout much of the retina. There are 10 million Müller cells in the mammalian retina.66 They play a supportive role, providing structure. The apex of the Müller cell is in the photoreceptor layer, whereas the basal aspect is at the inner retinal surface. Cellular processes form a reticulum among the retinal cell bodies and fill in most of the space of the retina not occupied by neuronal elements (Figure 4-12). Müller cells ensheathe dendritic processes within the synaptic layers, giving structural support, and their processes envelop most ganglion axons.67
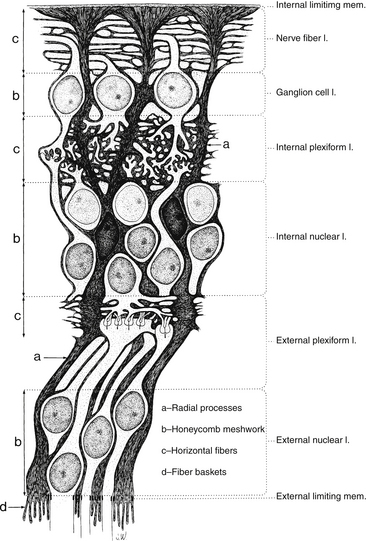
FIGURE 4-12 Structure of the Müller cell (dark gray). mem., membrane; l., layer.
(From Hogan MJ, Alvarado JA, Weddell JE: Histology of the human eye, Philadelphia, 1971, Saunders.)
Neuronal cell bodies and their processes appear to reside in tunnels within the Müller cell.52 Delicate apical villi, fiber baskets (of Schultze), terminate between the inner segments of the photoreceptors.1,29 On light microscopy, Müller cell processes can be seen passing through the layer containing the nerve fibers of the ganglion cells, perpendicular to the retinal surface. An expanded process, called the endfoot, along the basal aspect of the Müller cell contributes to the membrane separating the retina from the vitreous, and extensions of Müller cells wrap around blood vessels.1 The pervasiveness of the Müller cell results in very little extracellular space in the retina (see Figure 4-12). Besides providing structure, the Müller cell acts as a buffer by regulating the concentration of potassium ions (K+); they help maintain the extracellular pH by absorbing metabolic waste products68; they recycle GABA and glutamate, removing them from the extracellular space; and Müller cells metabolize, synthesize, and store glycogen.69–71
Microglial Cells and Astrocytes
Astrocytes are star-shaped fibrous cells found in the inner retina, usually in the nerve fiber and ganglion cell layers. These perivascular cells form an irregular supportive network that encircles nerve fibers and retinal capillaries.1 They may contribute to the internal limiting membrane as well as perform some of the same functions as the Müller cells.72
Ten Retinal Layers
The “10-layered” arrangement of the retina is actually a remarkable organization of alternate groupings of the retinal neurons just described and their processes. Traditionally, descriptive names were given to these so-called layers, and these designations are still in use today (Figure 4-13).
Retinal Pigment Epithelium
The RPE consists of a single layer of pigmented cells, as previously discussed. There are 4 to 6 million RPE cells, and each cell interacts with 30 to 40 photoreceptors.37,73,74 There is little cell division in the layer. The RPE is an active area with several functions that will be described in a later section.
External Limiting Membrane
The external limiting membrane (ELM, outer limiting membrane) is not a true membrane but is actually composed of zonula adherens junctions between photoreceptor cells and between photoreceptors and Müller cells at the level of the inner segments. On light microscopy, the so-called membrane appears as a series of dashes, resembling a fenestrated sheet through which processes of the rods and cones pass. This band of zonula adherens has the potential to act as a metabolic barrier restricting the passage of some large molecules.8,75
Outer Nuclear Layer
The outer nuclear layer (ONL) contains the rod and cone cell bodies; the cone cell body and nucleus are larger than those of the rod. Cone outer fibers are very short, and therefore the cone nuclei lie in a single layer close to the external limiting membrane; cell bodies of the rods are arranged in several rows inner to the cone cell bodies. The ONL is 8 to 9 cells thick on the nasal edge of the optic disc and 4 rows thick at the temporal edge and is thickest in the fovea, where it contains approximately 10 layers of cone nuclei.2
Outer Plexiform Layer
The outer plexiform layer (OPL; also outer synaptic layer) has a wide external band composed of inner fibers of rods and cones and a narrower inner band consisting of synapses between photoreceptor cells and cells from the inner nuclear layer. Rod spherules and cone pedicles synapse with bipolar cell dendrites and horizontal cell processes in the OPL. Many of these synapses consist of invaginations in the photoreceptor terminal; invaginations are deep in the spherule but more superficial in the pedicle.8 In these junctures the photoreceptor element contains a membranous plate, the synaptic ribbon8,59 (Figure 4-14). The invaginating synapse generally has three postsynaptic processes and is called a triad. The lateral elements are horizontal cell processes and are deep within the invagination, a bipolar dendrite is the center process (see Figure 4-6). Invaginating midget bipolar cells are involved in cone triads, and all cones have at least one invaginating midget bipolar and one flat midget bipolar contact.8
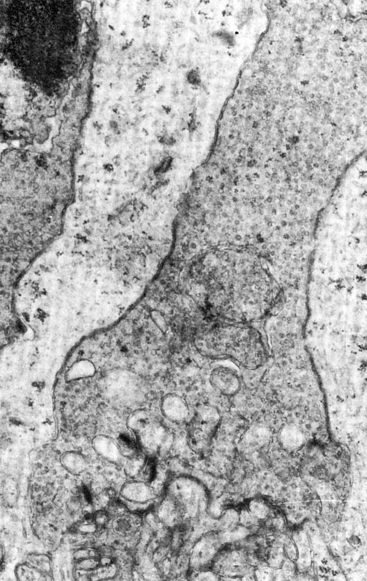
FIGURE 4-14 Electron micrograph of cone pedicle. Note dark linear bodies (synaptic ribbons) in pedicle. (×20,000.)
(From Leeson CR, Leeson ST: Histology, Philadelphia, 1976, Saunders.)
Synaptic contacts also occur outside invaginating synapses in the OPL. Horizontal cells make synaptic contact with bipolar dendrites and contact other horizontal cell processes via gap junctions.61,76 Bipolar dendrites synapse with photoreceptor cell endings; a single photoreceptor can have contact with more than one bipolar dendrite. The relatively long axon of the interplexiform neuron makes numerous synaptic connections with processes entering photoreceptor terminals.65,77
Desmosome-like attachments called synaptic densities are located within the arrangement of interwoven, branching, bipolar dendrites and horizontal cell processes in the OPL. These synaptic densities are seen as a series of dashed lines on light microscopy and resemble a discontinuous membrane, termed the middle limiting membrane. This “membrane” demarcates the extent of the retinal vasculature29 and may prevent retinal exudates and hemorrhages from spreading into the outer retinal layers.52
Inner Nuclear Layer
The inner nuclear layer (INL) consists of the cell bodies of horizontal cells, bipolar cells, amacrine cells, interplexiform neurons, Müller cells, and sometimes displaced ganglion cells. The nuclei of the horizontal cells are located next to the outer plexiform layer, where their processes synapse. The nuclei of the amacrine cells are located next to the inner plexiform layer, where their processes terminate. The bipolar cell has its dendrite in the outer plexiform layer and its axon in the inner plexiform layer (Figure 4-15). The interplexiform neuron also has processes in both synaptic layers. It is thought to receive input in the inner plexiform layer and project it to the outer plexiform layer.65 The retinal vasculature of the deep capillary network is located in the inner nuclear layer.
Inner Plexiform Layer
The inner plexiform layer (IPL; also inner synaptic layer) consists of synaptic connections between the axons of bipolar cells and dendrites of ganglion cells. The IPL contains the synapse between the second-order and third-order neuron in the visual pathway (see Figure 4-15). Generally, the axon of the invaginating midget bipolar cell ends in the inner half of the IPL, and the axon of the flat midget bipolar cell ends in the outer half of the IPL.51,54 Synapses also occur between (1) amacrine processes and bipolar axons, (2) amacrine processes and ganglion cell bodies and dendrites, (3) amacrine cells, and (4) amacrine cells and interplexiform neurons (Figure 4-16). The processing of motion detection and changes in brightness, as well as recognition of contrast and hue begin in this layer.78
Ribbon synapses in the IPL involve contact among a bipolar axon and a pair of postsynaptic processes, which may be amacrine or ganglion.8,79 A reciprocal synapse, thought to be inhibitory, involves the second contact of an amacrine process with a bipolar axon, providing negative feedback.52 Gap junctions between amacrine cells are also located in the IPL. Some displaced amacrine and ganglion cell bodies may also be seen.
Ganglion Cell Layer
The ganglion cell layer is generally a single cell thick except near the macula, where it might be 8 to 10 cells thick, and at the temporal side of the optic disc, where it is 2 cells thick. Although lying side by side, ganglion cells are separated from each other by glial processes of Müller cells.72 Displaced amacrine cells, which send their processes outward, may be found in the ganglion cell layer, as may some displaced Müller cell bodies and astroglial cells.8 Toward the ora serrata, the number of ganglion cells diminishes, and the nerve fiber layer thins.
Nerve Fiber Layer
Clinical Comment: Retinal Hemorrhages
HEMORRHAGES from retinal vasculature have a characteristic appearance. Because of the arrangement of the nerve fibers, the blood pools in a feathered pattern called a flame-shaped hemorrhage, which is indicative of the NFL location. Hemorrhages in the inner nuclear layer usually appear rounded and often are called dot or blot hemorrhages (Figure 4-17).
Internal Limiting Membrane
The internal limiting membrane (inner limiting membrane) forms the innermost boundary of the retina. The outer retinal surface of this membrane is uneven and is composed of extensive, expanded terminations of Müller cells (often called footplates) covered by a basement membrane. The inner or vitreal surface is smooth. The connection between this membrane and the vitreous is still under investigation and may actually occur at a biochemical level (see Chapter 6); only in the periphery are vitreal fibers incorporated into the internal limiting membrane.52
Anteriorly, the internal limiting membrane of the retina is continuous with the internal limiting membrane of the ciliary body. It is present over the macula but undergoes modification at the optic disc, where processes from astrocytes replace those of the Müller cells.58
Clinical Comment: Fundus View of the Internal Limiting Membrane
Reflections from the internal limiting membrane produce the retinal sheen seen with the ophthalmoscope. In younger persons, this membrane gives off many reflections and appears glistening; the sheen is less evident in older individuals (Figure 4-18).
Retinal Function
Physiology of the rpe
The RPE fosters the health of the neural retina and the choriocapillaris in several ways. First, the zonula occludens joining the RPE cells are part of the blood-retinal barrier and selectively control movement of nutrients and metabolites from the choriocapillaris into the retina and removal of waste products from the retina into the choriocapillaris.80 (In this regard, the RPE is analogous to the epithelium of the choroid plexus in the ventricles of the brain.)
A proposed model for RPE ion transport is shown in Figure 4-19. Ion movement occurs by Na+/K+ ATPase pumps, Na+/K+/2Cl− and Na+/2HCO3− cotransporters, Na+/H+ and Cl−/HCO3− exchangers, and gated and ungated ion channels.81 A proton-lactate-water cotransporter moves a significant amount of lactate (the product of anaerobic metabolism) across the RPE layer.81,82 Water passage occurs through aquaporins and Cl− and K+ are thought to be the primary ions driving the movement of water.83 Glucose transporters located in both the apical and basal membrane maintain a steady supply of glucose to the active photoreceptors.
Second, the RPE cells phagocytose fragments from the continual shedding of the photoreceptor outer segment discs; numerous lysosomes within each RPE cell enable it to ingest as many as 2000 discs daily.84 Undigested material accumulates as deposits of lipofuscin.81 Recently, a substance (A2E) has been identified in lipofuscin deposits that appears to inhibit RPE degradation of the outer segment remnants and contributes to RPE cell death.85 Third, the RPE metabolizes and stores vitamin A, one of the components of photopigment molecules86,87; it is the site for part of the biochemical process in the rod disc renewal system.84 Fourth, the cells contribute to the formation of the IPM between the RPE layer and the photoreceptors.75,88 Fifth, the RPE produces growth factors that drive certain cellular processes. It secretes vascular endothelial growth factor (VEGF), which helps maintain choriocapillaris function. However, the over-production of VEGF could result in neurovascularization, and so the RPE also produces an antiangiogenic factor, pigment epithelial derived factor (PEDF); the balance between these contributes to healthy function.80 Sixth, pigment granules within the RPE cells absorb light, thereby reducing excess light scatter.
Clinical Comment: Retinal Degenerations
RETINITIS PIGMENTOSA is an autosomal dominant retinal dystrophy, resulting in a progressive loss of RPE and photoreceptor function. Both rods and cones undergo apoptosis. Rods remain functional only in the far periphery and cones remain functional in the fovea, causing a ringlike scatomatous visual field defect. As the RPE degenerates, pigment migrates into the sensory retina, and accumulates around blood vessels in a characteristic bone-spicule pattern (Figure 4-20).
Stargardt’s macular dystrophy is a hereditary autosomal recessive disorder, resulting in vision loss occurring at an early age. A defect has been identified in a gene that directs the production of a protein that facilitates transport to and from photoreceptor cells. Early in the disease the RPE degenerates and as the disease progresses, lipofuscin-like deposits accumulate in the macular area (Figure 4-21).These deposits are yellow and fleck-shaped. Eventually the RPE atrophies and changes to the photoreceptors follow. Vision loss is progressive and by age 50, 50% of patients affected can have reduction of visual acuity to 20/200 or worse 89
Best’s disease, also called vitelliform macular dystrophy, is a rare autosomal dominant disorder. This disease also occurs because of a malfunctioning transport protein resulting in deposits between the RPE and neural retina.90 It usually presents in childhood as a striking yellow or orange egg yolklike elevated lesion in the macula (Figure 4-22).
Scotopic and Photopic Vision
In dim light the detection by rods predominates and in bright light, color detection takes precedence. Rods are extremely sensitive in poorly lit conditions (scotopic vision), when cones are least responsive. In scotopic vision, the light-sensitive retina allows detection of objects at low levels of illumination. Its ability to recognize fine detail is poor, however, and color vision is absent; objects are seen in shades of gray.60
Cone activity dominates in photopic vision, when the retina is responsive to a broader range of light wavelengths. Bright illumination is necessary for the sharp visual acuity and color discrimination of photopic vision. Cones are designated, depending on the wavelength that they absorb, as red (588 nm), green (531 nm), or blue (420 nm).91
Neural Signals
The neural signal generated by photoreceptors is modified and processed within the complex synaptic pathway through which it passes. There is a greater convergence of rods than of cones onto a ganglion cell. The ratio of rods to ganglion cells is high in most retinal regions, resulting in tremendous sensitivity for the detection of light and motion. It is estimated that 75,000 rods drive 5000 rod bipolar cells and 250 AII amacrine cells before converging onto a single ganglion cell.92 A relatively small number of cones drive the cone bipolar cell, and a small number of cone bipolar cells drive a single ganglion cell. In some situations, there is a 1:1 ratio between cones and ganglion cells, reflecting the significant amount of detail that the cone population can discriminate.1 A single midget bipolar dendrite may contact only one cone pedicle, and its axon then synapses on a single midget ganglion cell.92 The cone pathway involves a three-neuron chain, whereas the rod pathway involves a four-neuron chain because of the amacrine cell inclusion.2
Ganglion cell axons can be thought of as “carrying information in processing streams,” such that certain types of information are directed toward specific destinations.8 One major target is the lateral geniculate nucleus, wherein some axons terminate in the parvocellular layers, which process wavelength, shape, fine detail, and resolution of contrast. Other axons end in the magnocellular layers, which discern movements and flickering light but have poor wavelength sensitivity.93 Visual information terminating in the midbrain is important in the autonomic control of the ciliary and iris muscles. Other centers that receive visual information can influence motor pathways that control eye, head, and neck movements.
Number and Distribution of Neural Cells
In 1935, Østerberg94 estimated the cell count in the retina at 110 million to 125 million rods and 6.3 million to 6.8 million cones. More recent research indicates that there are 80 million to 110 million rods and 4 million to 5 million cones.95,96 The density of rods is greater than that of cones except in the macular region, where cones are concentrated; rods are absent from the foveola,15 the macular center (Figure 4-23). Rod density is greatest in an area concentric with the fovea, beginning approximately 3 mm (7 degrees) from it.52,60 The number of both types of photoreceptors diminishes toward the ora serrata.
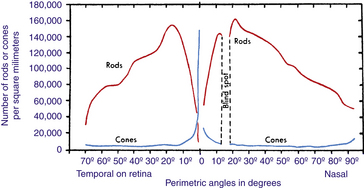
FIGURE 4-23 Distribution of rods and cones in human retina.
Instead of retinal distances, Østerberg’s values84 for corresponding perimetric angles are given. Although approximate only, especially at higher angles, such values are more useful in practice than are distances on the retina. Note that distribution of rods and cones on nasal side in and near the fovea, not given on this graph, would be approximately the same as distribution on temporal side of retina, which is seen to the left of vertical, passing through 0 degrees on the angle scale.
(From Pirenne M: Vision and the eye, London, 1948, Pilot Press.)
There are approximately 35.68 million bipolar cells97 and 1.12 million to 2.22 million ganglion cells.98 The signals from numerous photoreceptors converge at one ganglion cell, indicating integration and refinement of the initial response of the photoreceptor cells.
Physiology of the neural retina
Retinal Synapses
Information transmission between retinal neurons occurs by ion channel activity at gap junctions or by neurotransmitter release in chemical synapses. The gap junction is an electrical synapse, allowing current to pass directly between cells, ensuring a rapid rate of signal transmission; no chemical mediator is necessary. Gap junctions are found between photoreceptor and photoreceptor, between photoreceptor and horizontal cell, between horizontal cells, and between a bipolar axon and an amacrine process.8
Chemical synapses contain synaptic vesicles that release a neurotransmitter from the presynaptic terminal into the synaptic cleft. The transmitter binds to specific sites on the postsynaptic membrane, eliciting an excitatory or inhibitory change in that neuron. In the OPL synapses occur either on the flat part of the pedicle or in invaginations in spherules and pedicles. The synapses in the invaginations are often ribbon synapses; ribbon synapses allow for fast and sustained neurotransmitter release. An electron-dense bar, surrounded by a large number of synaptic vesicles, extends into the cytoplasm perpendicular to the pre-synaptic membrane. The ribbonlike structure seems to guide the vesicles to a release site on the pre-synaptic membrane, causing sustained release. Calcium ion channels facilitate vesicle fusion with the membrane and promote high-speed release. Ten times more vesicles per second are released at a ribbon synapse than at a conventional synapse.27 Triads are ribbon junctions, located in the OPL, that have three postsynaptic processes; dyads are ribbon synapses found in the IPL with two postsynaptic processes. Although visual interpretation occurs in the striate cortex, there is significant organization and processing of neural signals in excitatory and inhibitory circuits within the retina. The process is extremely complex and most current understanding is based on animal studies.
Neurotransmitters
Glutamate is the excitatory neurotransmitter released by photoreceptors, bipolars, and ganglion cells. Glycine and GABA are inhibitory neurotransmitters released from amacrines; it is unclear what neurotransmitter horizontal cells secrete. In addition to neurotransmitters, neuromodulators are chemicals that can alter neuron transmission. They are released by retinal cells into the extracellular space but not necessarily by synaptic vesicles at the synaptic cleft; they include dopamine, nitric oxide, and retinoic acid. An example of a neuromodulator effect: Dopamine can change the conductance of gap junctions between horizontal cells and modulate responses to changes in background illumination.99,100
Phototransduction
A visual pigment (photopigment) consists of two parts, a membrane protein, called an opsin, and a chromophore. The opsin forms a long helix that loops back and forth across the membrane bilayer seven times; the chromophore is the molecule that actually absorbs the photon, and is contained within the looped protein. 11-cis-retinal is the chromophore present in all photoreceptors; it is a derivative of vitamin A. The seven-looped opsin determines the wavelength absorbed by a photoreceptor. The photopigment in rods is arranged in the disc membranes and its protein is rhodopsin. In cones the photopigment is located throughout the continuous plasma membrane whose deep infoldings form the cone discs.45 The protein opsin in L-cone cells is red sensitive and in M-cones is green sensitive. The structure of these two photopigments differs by only a few amino acids, and the genes for them are located in a tandem array on the X-chromosome. Blue sensitive S-cones (comprising only 5% to 10% of the cone population) are structurally different.78
The photoreceptor is in the depolarized state when it is not stimulated by light. As neurons usually do in the depolarized state, the photoreceptor secretes its neurotransmitter. During depolarization, voltage-gated Ca++ channels are open and calcium ions facilitate the process by which the vesicles containing glutamate merge with the cell membrane, enabling the release of neurotransmitter into the synaptic cleft.78,101 Thus, in the dark the photoreceptor terminal is continually releasing glutamate. The depolarized state occurs because of an ion circuit within the photoreceptor. The photoreceptor outer segment is permeable to Na+; the cGMP-gated cationic channels in the outer segment membrane are kept open because of a high concentration of cytoplasmic cGMP. Na+ moves into the outer segment, through the open channels and the ions pass easily into the inner segment through the cilium, where Na+ is extruded by Na+/K+ ATPase pumps (Figure 4-24). This circuit (caused by Na+ moving into the outer segment and exiting the inner segment), is called the dark current. In this state the photoreceptor is depolarized with a membrane potential of approximately −40 mV.
Within a picosecond of light activating the visual pigment, a biochemical cascade occurs that results in a decrease in the concentration of cGMP closing the Na+ channels.78 The inside of the cell increases in negativity due to the continued loss of Na+ through the pumps in the inner segment membrane and the cell becomes hyperpolarized; the membrane potential approaches −75 mV. The change in potential is graded, the level of hyperpolarization depends on the amount of light absorbed and the number of visual pigment molecules activated. The magnitude of the hperpolarization determines the change in the amount of transmitter released, either slowing or stopping the flow.78 Once the level of cGMP is restored, the ion channels open and the cell once again becomes depolarized and releases glutamate. The amount of transmitter released by the photoreceptor decreases as the amount of light absorbed increases.
All-trans-retinal moves from the disc lumen into the cytoplasm where it is reduced to all-trans-retinol. The photoreceptor cannot re-isomerize the molecule, so it must be transported by specific carrier proteins within the interphotoreceptor matrix (IPM) to the RPE.81 These cells contain the enzymes that convert all-trans-retinol to 11-cis-retinol and finally oxidize it back to11-cis-retinal; 11-cis-retinal is then transported back through the IPM to be incorporated into the photopigment. In the cone recycling process, some animal models indicate that the Müller cell has a role in the visual cycle by taking up all-trans-retinol and re-isomerizing it to 11-cis-retinol, which is then transported back to the cone and oxidized to 11-cis-retinal and incorporated into the photopigment.102,103 The steps of the rod renewal system are well known, but those of the cone renewal system are still unclear.
Information Processing
Glutamate will cause a bipolar cell to either depolarize or hyperpolarize depending on the type of receptor present in the plasma membrane of the bipolar dendrite.104,105 Bipolars cells with ionotropic receptors in their membrane respond to glutamate with a depolarization and are OFF bipolars and bipolar cells that have metabotropic receptors in their membrane respond to glutamate with a hyperpolarization and are ON bipolars.104,105 The neurotransmitter at the axon terminal in bipolar cells is also glutamate (Glu) and bipolars release Glu when they are in the depolarized state.
When a photoreceptor is depolarized (thus it is in the dark, light is OFF) it is releasing Glu. When Glu binds to the ionotropic receptor on a bipolar dendrite, cation channels are opened in the cell membrane, causing the bipolar cell to depolarize and release Glu.106 This is an OFF bipolar because it is depolarized in the dark. When Glu binds to the metabotropic receptors on a bipolar cell dendrite, a decrease of cGMP occurs, closing cation channels in the cell membrane and causing the bipolar cell to hyperpolarize, resulting in a decrease of glutamate release.107 This is an ON bipolar because it is hyperpolarized in the dark.
When the photoreceptor is hyperpolarized (light is ON), Glu release is reduced or stopped. The lack of Glu at the ionotropic receptor causes the Glu-gated cationic channels in the bipolar membrane to close. The OFF bipolar cell hyperpolarizes, reducing its release of neurotransmitter.106 When Glu is reduced or no longer present, the lack of Glu at the metabotropic receptor signals a cGMP cascade, cGMP increases, cGMP-gated cation channels open, and the ON bipolar depolarizes, which increases its neurotransmitter release.107
Generally, the ON bipolar dendrite synapses within a photoreceptor invagination and the OFF bipolar dendrite synapses only with cones and on the flat part of the pedicle. Each cone in central retina contacts both an ON and an OFF midget bipolar.78 All rod bipolars are ON cells (Figure 4-25).
Bipolar axons end in the IPL. One synaptic configuration is a dyad, which consists of a synapse between a bipolar axon and two post synaptic elements, either two amacrine processes or one amacrine process and one ganglion dendrite. ON and OFF bipolar axons terminate in different tiers of the IPL. OFF bipolars synapse in the outer tier, sublamina a (nearest the INL), and ON bipolars synapse in the inner tier, sublamina b, closest to the ganglion cell layer.78 Rod bipolars do not synapse with ganglion cells directly but with amacrine cells; thus the rod signal must pass through a four neuron chain (see Figure 4-25).
The P ganglion cells terminate in the parvocellular layers of the LGN, are associated with cone bipolar cells, and carry color information. The P1 cells, also called mdget ganglion cells are concentrated in central retina, and constitute 80% of the ganglion cell population.78 M ganglion cells project to the magnocellular layers of the LGN. They have also been called parasol ganglion cells because of their large spreading dendritic trees. Because they have such expansive processes and cover a large area of retina, they can respond rapidly to moving or changing stimuli.
Horizontal cells communicate with other horizontal cells through gap junctions and receive excitatory input through chemical synapses from photoreceptors. Horizontal cells provide inhibitory feedback to photoreceptors and inhibitory feed forward to bipolars.78
In the dark, while the photoreceptor is continuously releasing the excitatory neurotransmitter glutamate, its horizontal cells are depolarized. With light stimulation the photoreceptor hyperpolarizes and transmitter release is reduced. Ligand-gated channels close in the horizontal cell membrane, causing it to hyperpolarize.108 The amplitude and duration of the response depends on the strength of the photoreceptor hyperpolarization, and thus on the intensity and duration of the light stimulus.92,108 Because horizontal cells are joined by gap junctions a great number of horizontal cells can be affected when just one is influenced by a photoreceptor.
The mechanism by which the inhibitory message is passed from the horizontal cell to the cone is not fully understood. It was once thought that the horizontal cell released the inhibitory neurotransmitter GABA. Subsequent studies have raised doubts that GABA is a major player in the feedback process from horizontal cells.99,109 It is speculated (based on animal models) that a change in the horizontal cell polarization causes a current change in the extracellular potential in the synaptic cleft within an invagination. This could affect the Ca++ channels in the synaptic membrane of the cone influencing the synaptic vesicle release of Glu without actually changing the cone membrane potential. The change in neurotransmitter release would affect the bipolar dendrites within the invagination and in some cases might reverse their reaction.99,108,109
Amacrine cells also carry information in a horizontal direction. There are 40 different types but the circuitry of only a few has been established. Amacrine cells are generally inhibitory and release either GABA or glycine. Amacrine processes make conventional synapses with bipolar axons and with ganglion cell dendrites or soma. The conventional chemical synapse with bipolar cell axons are feedback synapses; synapses on ganglion cells are feed-forward synapses.110 Amacrine cells also synapse with other amacrine cells.
The narrow-field rod amacrine cell, AII, releases glycine. It is the intermediary between the rod bipolar and the ganglion cell. An AII amacrine cell gathers information from about 300 rods.78 The AII provides connection between the ON and OFF pathways. The AII receives information from a rod bipolar axon (an ON cell) in sublamina b of the IPL, and relays information by a conventional synapse to an OFF cone bipolar in sublamina a, thereby influencing an OFF ganglion cell. The AII also carries rod information to an ON cone bipolar axon through gap junctions in sublamina b and influences an ON ganglion cell.61 AII amacrine cells, whose processes are joined by gap junctions, form a weak electrical syncytium.78
The A17 amacrine cells are wide-field diffusely branching cells. They appear to interconnect rod bipolar cells but do not appear to make synapses with other amacrines nor ganglion cells.110 A single A17 amacrine cell can receive input from as many as 1000 rod bipolars.110 They are thought to amplify signals in dim illumination.
The A18 amacrine cell is a wide-field amacrine with an extensive dendritic tree. It seems to have a role in the regulation of scotopic vision flow, and in modulating retinal adaptation to differing light conditions. It can interfere with the AII amacrine synapse with cone bipolar cells, and effectively reduce the size of the receptive field.92 The A18 releases dopamine, which can disrupt the gap junctions that forms the syncytium of AII amacrines. Dopamine released by the A18 amacrine may also have some function in the circadian cycle.111
Interplexiform neurons have processes in both the OPL and the IPL and convey signals between these layers, that is, from inner retina to outer. They might secrete dopamine as their transmitter but further details about their physiology is lacking.112
Receptive Fields
Retinal receptive fields are arranged in a center-surround pattern. When light activates cells in the center of the field, a given response occurs. When light falls on the surround (the annular region immediately around the center), an antagonistic response occurs. The response by the cells in the surround inhibits the response from the cells in the center. This pattern is seen at the level of the bipolar cells, the ganglion cells, and in LGN and the striate cortex. When cells in the surround are activated, the signal coming from the center cell is changed to the opposite response. The center-surround response occurs in part due to lateral inhibition by horizontal cells and because of amacrine cell activity on bipolar axon terminals.113
The center-surround configuration allows a neuron to not only respond to a direct message but to gather information from neighboring areas providing details about the bigger picture that then influences that neuron. This process aides in the detection of edges and in the recognition of contrast, and it maximizes retinal contrast sensitivity through a wide range of background illuminations.78
Light and Dark Adaptation
The visual system is highly specialized for the detection and analysis of patterns of light; by visual adaptation, it can modify its capacity to respond at extremely high and low levels of illumination. The level of background illumination can affect the both the ease and the speed with which a photoreceptor responds. When a significant change in light level occurs, adaptation can be prolonged; it can take 30 minutes for the retina to adapt fully when going from bright sunlight to complete dark (dark adaptation). At first only cones are functioning, but since they are now in the dark they are not stimulated and the rods take some time to reach maximum function. Light adaptation, going from complete dark to bright light, takes approximately 5 to 10 minutes; the cones reach their functional mode much more quickly than do rods. The state of adaptation (sensitivity) of a photoreceptor is regulated by Ca++, which can influence the concentration of cGMP, the messenger that controls gated ion channels in the photoreceptor membrane.78
Circadian Rhythm
The circadian rhythm is the light/dark or wake/sleep cycle, which usually extends over a period of 24 hours. It is under the direction of pineal melatonin secretion, which is influenced by the suprachiasmatic nucleus, the master biologic clock located in the hypothalamus.114 A special population of ganglion cells that contain melanopsin has been identified; they are photosensitive and can respond directly to light. It is estimated that there are 3000 of these ganglion cells dispersed across the retina.115,116 Their axons project to the suprachiasmatic nucleus and help in synchronizing the circadian rhythm to the wake/sleep cycle.78,116 Neuromodulators, such as dopamine, secreted by the interplexiform neuron and the amacrine A18 cells, may also have some role in regulating the circadian rhythm.113
Retinal Metabolism
The extensive network of continual intracellular communication requires extensive energy utilization by retinal tissue. The primary source of energy is provided by glucose metabolism. Glucose moves out of the blood and into retinal tissue via facilitated diffusion; glucose transporters are located on both the apical and basal membranes of the retinal pigmented epithelial cell and on the endothelium of retinal capillaries.27 The retina can switch from glycolysis to oxidative metabolism depending on need, but even under normal physiologic conditions the retina has a high rate of anaerobic glycolysis.78 The monophosphate pathway is particularly active in photoreceptors for rhodopsin regeneration and ribose production for nucleotide synthesis.27 Müller cells store glycogen, providing a ready source for glucose. Because energy requirements are high, oxygen consumption is high. Capillary blood flow in retinal tissue has been measured in primates and is approximately 60 ml/min/100 g of tissue, similar to the flow in the brain.117 Oxygen utilization by photoreceptors is 3 to 4 times higher than other CNS neurons.27 Because oxygen must diffuse from the choriocapillars to the inner segments where the mitochondria are located, blood flow is significantly higher in the choriocapillaris, i.e., approximately 2000 ml/min/100 g of tissue.116 In dark, the photoreceptors consume so much oxygen that the oxygen tension in the tissue is near zero and the photoreceptors are operating under near ischemic conditions.78
Regions of Retina
Central Retina
Macula Lutea
The macula lutea appears as a darkened region in the central retina and may seem to have a yellow hue because of the xanthophyll pigments, lutein, and zeaxanthin.52,118 These pigments are located throughout the retina, but the greatest concentration is in the macula. The pigments are primarily located in the photoreceptor inner fibers but are also found in the rod outer segments.78,118,119 The newborn has little if any of these pigments, but they gradually accumulate from dietary sources. These pigments apparently act as filters, absorbing short wavelength visible light to reduce chromatic aberration but may also have an antioxidant effect, suggesting a protective role against UVR damage.118 The macula lutea is approximately 5.5 mm in diameter; its center is approximately 3.5 mm lateral to the edge of the disc and approximately 1 mm inferior to the center of the disc. The pigment epithelial cells are taller and contain more pigment than cells elsewhere in the retina, contributing to the darkness of this area. However, the density of the pigment varies greatly from person to person.58 The choroidal capillary bed also is thicker in the macula lutea than elsewhere.
Useful color vision occupies an area approximately 9 mm in diameter, the center of which is the macula lutea.8 The entire macular region consists of the foveola, the fovea, and the parafoveal and perifoveal areas (both are annular regions) (Figure 4-26). These areas are described and delineated on the basis of histologic findings, with consideration given to the number and rows of cells in the nuclear layers. However, these areas are not easily differentiated on viewing the living retina.
Clinical Comment: Terminology
The terms used to describe the macular area differ between the histologist and the clinician. The histologist uses the word fovea to describe what a clinician would name macula, and the histologist calls the foveola that which a clinician would name the fovea. The term macula is purely a clinical one and usually refers to the area of darker coloration that is approximately the same size as the optic disc; clinically, the term fovea then refers to the very center of this area. The posterior pole is another term used in clinical descriptions of the fundus. There is no universal agreement regarding its definition, and its usage varies from clinician to clinician.29
Fovea (Fovea Centralis)
The shallow depression in the center of the macular region is the fovea, or central fovea of the retina (fovea centralis retinae). This depression is formed because the retinal neurons are displaced, leaving only photoreceptors in the center. The fovea has a horizontal diameter of approximately 1.5 mm. The curved wall of the depression is known as the clivus, which gradually slopes to the floor, the foveola. The fovea has the highest concentration of cones in the retina; estimates vary from 199,000 to 300,000 cones per square millimeter.96,120 The number falls off rapidly as one moves away from the fovea in all directions. In this area of the retina, specialized for discrimination of detail and color vision, the ratio between cone cells and ganglion cells approaches 1:1.8 In more peripheral areas of the retina, which are sensitive to light detection but have poor form discrimination, there is a high ratio of rods to ganglion cells.
Within the fovea is a capillary-free zone 0.4 to 0.5 mm in diameter (Figure 4-27).121 The lack of blood vessels in this region allows light to pass unobstructed into the photoreceptor outer segment.
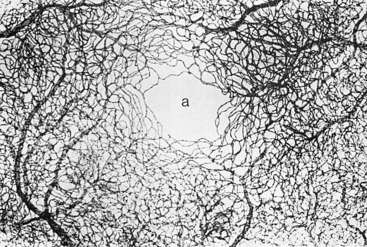
FIGURE 4-27 Capillary bed of macular region, with capillary-free zone (a) in its center. (×42.5.)
(From Hogan MJ, Alvarado JA, Weddell JE: Histology of the human eye, Philadelphia, 1971, Saunders.)
The only photoreceptors located in the center of the fovea are cones. These are tightly packed, and the outer segments are elongated, appearing rodlike in shape yet containing the visual pigments of the cone population. The external limiting membrane is displaced vitreally because of the lengthening of the outer segments. This rod-free region has a diameter of approximately 0.57 mm1 and represents approximately 1 degree of visual field.120 Most of the other retinal elements are displaced, allowing light to reach the photoreceptors directly without interference of other retinal cells (Figure 4-28).
The cells of the inner nuclear layer and ganglion cell layer are displaced laterally and accumulate on the walls of the fovea. The photoreceptor axons become longer as they deviate away from the center; these fibers are called Henle’s fibers. They must take an oblique course to reach the displaced bipolar and horizontal cells (Figure 4-29). This region of the OPL is known as Henle’s fiber layer.1 The retinal layers and the foveal indentation are clinically evident with a CRT view of the retina (Figure 4-30).
Foveola
The diameter of the foveola is approximately 0.35 mm. At the foveola, the retina is approximately 0.13 mm thick, compared with 0.18 mm at the equator and 0.11 mm at the ora serrata.1 The foveola contains the densest population of cones that have the smallest cross-sectional diameters of all the photoreceptors.8
Clinical Comment: Central Foveal Reflex
When the direct ophthalmoscope light shines directly into the fovea, it reflects a pinpoint of light called the central foveal reflex. This pinpoint reflection is caused by the parabolic shape formed by the clivus. Because the shape of the fovea is not always exactly parabolic, the reflection may vary in sharpness and regularity from person to person. The fovea is the site at which the object of interest is imaged. In younger persons the sheen from the internal limiting membrane sometimes is seen as a circular macular reflex (see Figure 4-18).
Clinical Comment: Metamorphopsia
The axis of the photoreceptor outer segment is oriented to accomplish capture of incident light rays. If a disruption occurs so that the outer segment is no longer oriented toward the exit pupil, vision may be altered. With macular edema, the orientation of the photoreceptors is changed, and metamorphopsia can often be elicited with an Amsler grid.122
Parafoveal and Perifoveal Areas
The annular zone surrounding the fovea can be divided into an inner parafoveal area and an outer perifoveal area (see Figure 4-26). The parafoveal area contains the largest accumulation of retinal bipolar and ganglion cells. The inner nuclear layer can be 12 cells thick and the ganglion cell layer 7 cells thick.52,58 At the maximum density of ganglion cells there can be 40,000 cells per square millimeter.78 The perifoveal area begins where the ganglion cell layer is four cells thick and ends where it is one cell thick. Within the perifoveal area, the fibers of Henle’s fiber layer revert to the usual orientation seen in the outer plexiform layer. The width of the parafoveal area is 0.5 mm and of the perifoveal area, 1.5 mm.1
Peripheral Retina
The ora serrata is the peripheral termination of the retina and lies approximately 5 mm anterior to the equator of the eye.123 Its name derives from the scalloped pattern of bays and dentate processes (see Chapter 3); the retina extends further anteriorly on the medial side of the eye. The ora serrata is approximately 2 mm wide and is the site of transition from the complex, multilayered neural retina to the single, nonpigmented layer of ciliary epithelium.123 A firm attachment between the retina and vitreous, the vitreous base, extends several millimeters posterior to the ora serrata.
Clinical Comment: Peripheral Retinal Degeneration
Cystic spaces and atrophied areas are often found in peripheral retina, and their incidence increases with age. One cause for these changes is the poor blood supply in the extreme retinal periphery.52,58 Some conditions affecting the peripheral retina are normal, age-related changes, and others might predispose the affected individual to more serious conditions, necessitating periodic, routine, dilated-fundus examinations.
Optic Disc
The optic disc, or optic nerve head, is the site where ganglion cell axons accumulate and exit the eye. It is slightly elongated vertically. The horizontal diameter of the disc is approximately 1.7 mm and the vertical diameter approximately 1.9 mm.124 The number of nerve fibers appears to be positively correlated with the size of the optic nerve head; larger discs have relatively more fibers than smaller discs. Smaller discs may demonstrate optic nerve head crowding.124,125 Fiber number decreases with age.98
Because the disc contains no photoreceptor cells, light incident on the disc does not elicit a response; thus it represents the physiologic blind spot. A depression in the surface of the disc, the physiologic cup, varies greatly in size and depth, according to embryologic development (Figure 4-31).
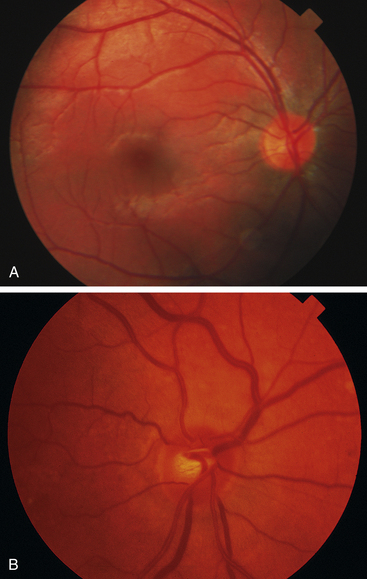
FIGURE 4-31 Variability in the normal cup-to-disc ratios.
(Courtesy Pacific University Family Vision Center, Forest Grove, Ore.)
Normally, the disc margins are flat and in the same plane as the retina, swelling only toward the vitreous in optic nerve head edema. Various types of crescents or rings are observed around the optic disc margin. In almost all individuals, the disc edges are emphasized by a white rim of scleral tissue, which separates the optic nerve from the choroid. Different configurations in the anatomic arrangement at the disc border produce the pigmented crescent often seen outer to the scleral crescent. The RPE may not extend to the edge of the disc, and the darkly pigmented choroid might be evident. Irregular areas of hypopigmentation and hyperpigmentation of the RPE are common near the disc.126,127
Clinical Comment: Papilledema
PAPILLEDEMA is edema of the optic disc secondary to an increase in intracranial pressure (ICP).128 As ICP increases, pressure within the meningeal sheaths around the optic nerve slows axoplasmic flow in the ganglion fibers, causing fluid to accumulate within the fibers so that they swell.129,130 This accumulation of fluid is seen at the disc as an elevation of the nerve head with blurring of the disc margins (Figure 4-32). This condition is or will become bilateral. The central retinal vein may also be compromised, with hemorrhages becoming evident in the nerve fiber layer in the vicinity of the disc.
Edema of the optic disc from any other cause is referred to as simply “edema of the optic disc.”
Retinal Blood Supply
The outer retinal layers receive nutrition from the choroidal capillary bed; metabolites diffuse through Bruch’s membrane and the RPE into neural retina. The central retinal artery provides nutrients to inner retinal layers. The artery enters the retina through the optic disc, usually slightly nasal of center, and branches into a superior and inferior retinal artery, each of which divides further into nasal and temporal branches. These vessels continue to bifurcate (see Figure 4-31). The nasal branches run a relatively straight course toward the ora serrata, but the temporal vessels arch around the macular area en route to the periphery. The vessels are located in the nerve fiber layer just below the transparent internal limiting membrane.
Two capillary networks are formed. The deep capillary network lies in the inner nuclear layer near the outer plexiform layer, and the superficial capillary network is in the nerve fiber layer or ganglion cell layer.58 The retina outer to the outer plexiform layer is avascular, and the outer plexiform layer is thought to receive its nutrients from both retinal and choroidal vessels. The middle limiting membrane usually is regarded as the border between the choroidal and retinal supplies.
A capillary-free zone directly surrounds the retinal vessels, and in the fovea, as mentioned, an area approximately 0.5 mm in diameter is free of all retinal vessels.1,29 Retinal vessels are said to be “end vessels” because they do not anastomose with any other system of blood vessels.58 Retinal vessels terminate in delicate capillary arcades approximately 1 mm from the ora serrata.29 The retinal capillaries are made up of a single layer of unfenestrated endothelium surrounded by a basement membrane and an interrupted layer of pericytes.29,58,131 Pericytes are cells with a contractile function that facilitate blood flow.132 The endothelial cells are one part of the blood-retinal barrier because they are joined by zonula occludens.80,133,134
A dense peripapillary network of capillaries, radially arranged around the optic nerve head, follows the arcuate course of the nerve fibers as they enter the disc.135
A cilioretinal artery is a vessel that enters the retina from the edge of the disc but has its origin in the choroidal vasculature. Such a vessel, that nourishes the macular area, is found in approximately 15% to 20% of the population (see Figure 11-4).136 A cilioretinal artery can maintain the viability of the macula if blockage of the central retinal artery occurs. Smaller, less significant cilioretinal vessels can be found in 25% of the population.136
Aging Changes in Retina
Normal aging is a slow, continuous process that may predispose to pathologic changes. It may be unclear, however, where normal aging changes stop and disease processes begin.
Because an estimated 33% to 50% of central nervous system neurons are lost during a lifetime, the number of retinal neurons will decrease, with ganglion cell loss especially noted in the fovea.137 The number of nerve fibers in the optic nerve decreases, and the fibers are replaced with connective tissue as they degenerate.138–140 Some studies report a decrease in foveal cones with age141; others do not.142 Rod density declines with age,142 but no decrease is evident in scotopic sensitivity.143 Some bipolar dendrites and horizontal cell processes lengthen and extend into the ONL.144 The number of astroglial cells is reduced.145
The number of retinal pigmented epithelial cells is reduced from 4000/mm2 to 2000/mm2; other changes in the RPE layer include pleomorphism, atrophy, depigmentation, and hyperplasia.2,81,146 Lipofuscin accumulates throughout life in the RPE and the cone inner segments and may be linked to a decrease in the lysosomal activity of enzymes in the metabolically active RPE.2,146–148
Peripapillary chorioretinal atrophy, usually evident as a pale, temporal crescent, is an age-related degeneration of RPE and Bruch’s membrane, and may be caused by attenuation of the peripapillary circulation.149 With age there is a decrease in neuroretinal rim tissue, and the vertical optic cup diameter and the area of the optic cup both increase; these factors need to be considered when assessing the optic nerve head for glaucoma.150
Müller cells become hypertrophic with age.138,139
Clinical Comment: Alzheimer’s Disease
Some speculate that early changes in the retina might be diagnostic in patients with Alzheimer’s disease. Some investigators report a reduction in NFL thickness related to retinal dysfunction,151–153 with the greatest reduction in the foveal region.153 Others have not found a reduction in NFL thickness.154 Evaluating the nerve fiber layer can be difficult but is becoming more accurate with improvements in technology. An extensive loss of neurons throughout the retina, particularly ganglion cells and glial cells, has been histologically documented in specimens from patients with Alzheimer’s disease.155 An increased cup-to-disc ratio and decreased rim tissue have also been observed in these patients.156
1. Hogan M.J., Alvarado J.A. Retina. In: Hogan M.J., Alvarado J.A., Weddell J.E., editors. Histology of the human eye. Philadelphia: Saunders; 1971:393.
2. Sharma R.K., Ehinger B.E.J. Development and structure of the retina. In: Kaufman P.L., Alm A., editors. Adler’s physiology of the eye. ed 10. St Louis: Mosby; 2003:319.
3. Young R.W. Pathophysiology of age-related macular degeneration. Surv Ophthalmol. 1987;31:291.
4. Ko M.K., Lee W.R., McKechnie N.M., et al. Post-traumatic hyperlipofuscinosis in the human retinal pigment epithelium. Br J Ophthalmol. 1991;75(1):54.
5. Feeney-Burns L., Hilderbrand E.S., Eldridge S. Aging human RPE: morphometric analysis of macular, equatorial, and peripheral cells. Invest Ophthalmol Vis Sci. 1984;25:195.
6. Weiter J.J., Delori F.C., Wing G.L., et al. Retinal pigment epithelial lipofuscin and melanin and choroidal melanin in human eyes. Invest Ophthalmol Vis Sci. 1986;27:145.
7. Guymer R., Luthert P., Bird A. Changes in Bruch’s membrane and related structures with age. Prog Retin Eye Res. 1999;18(1):59.
8. Cohen A.I. The retina. In: Hart M.J.Jr., editor. Adler’s physiology of the eye. ed 9. St Louis: Mosby; 1992:579.
9. Hudspeth A.J., Yee A.G. The intercellular junctional complexes of retinal pigment epithelia. Invest Ophthalmol. 1973;12:354.
10. Fatt I., Shantinath K. Flow conductivity of retina and its role in retinal adhesion. Exp Eye Res. 1971;12:218.
11. Kita M., Marmor M.F. Systemic mannitol increases the retinal adhesive force in vivo. Arch Ophthalmol. 1991;109:1449.
12. Yao X.Y., Moore K.T., Marmor M.F. Systemic mannitol increases retinal adhesiveness measured in vitro. Arch Ophthalmol. 1991;109:275.
13. Marmor F.M., Abdul-Rahim A.S., Cohen D.S. The effect of metabolic inhibitors on retinal adhesion and subretinal fluid resorption. Invest Ophthalmol Vis Sci. 1980;19:893.
14. Kita M., Marmor M.F. Effects on retinal adhesive force in vivo of metabolically active agents in the subretinal space. Invest Ophthalmol Vis Sci. 1992;33:1883.
15. Foulds W.S. The vitreous in retinal detachment. Trans Ophthalmol Soc U K. 1975;95:412.
16. DeGuillebon H., Zanberman H. Experimental retinal detachment: biophysical aspects of retinal peeling and stretching. Arch Ophthalmol. 1972;87:545.
17. Hollyfield J.G., Varner H.H., Rayborn M.E., et al. Retinal attachment to the pigment epithelium. Retina. 1989;9:59.
18. Hageman G.S., Marmor M.F., Yao X.Y., et al. The interphotoreceptor matrix mediates primate retinal adhesion. Arch Ophthalmol. 1995;113(5):655.
19. Hollyfield J.G., Varner H.H., Rayborn M.E. Regional variation within the interphotoreceptor matrix from fovea to the retinal periphery. Eye. 1990;4:333.
20. Hollyfield J.G., Rayborn M.E., Landers R.A., et al. Insoluble interphotoreceptor matrix domains surround rod photoreceptors in the human retina. Exp Eye Res. 1990;51:107.
21. Mieziewska K. The interphotoreceptor matrix, a space in sight. Microsc Res Tech. 1996;35(6):463.
22. Marmor M.F., Yao X.Y., Hageman G.S. Retinal adhesiveness in surgically enucleated human eyes. Retina. 1994;14(2):181.
23. Nicolaisson B.Jr. Connections between the sensory retina and the retinal pigment epithelium. Acta Ophthalmol (Copenh). 1985;63:68.
24. Lazarus H.S., Hageman G.S. Xyloside-induced disruption of interphotoreceptor matrix proteoglycans results in retinal detachment. Invest Ophthalmol Vis Sci. 1992;33:364.
25. Sigleman J., Ozanics V. Retina. In: Jakobiec F.A., editor. Ocular anatomy, embryology, and teratology. Philadelphia: Harper & Row; 1982:441.
26. Tombran-Tink J., Shivaram S.M., Chader G.J., et al. Expression, secretion, and age-related downregulation of pigment epithelium-derived factor, a serpin with neurotrophic activity. J Neurosci. 1995;15(7, pt 1):4992.
27. Picaud S. Retinal biochemistry. In: Kaufman P.L., Alm A., editors. Adler’s physiology of the eye. St Louis: Mosby; 2003:382-408.
28. Young R.W. The renewal of the photoreceptor cell outer segments. J Cell Biol. 1967;33:61.
29. Fine B.S., Yanoff M. The retina. In: Fine B.S., Yanoff M., editors. Ocular histology. ed 2. Hagerstown, Md: Harper & Row; 1979:59.
30. Fine B.S., Zimmerman L.E. Observations on the rod and cone layer of the retina. A light and electron microscopic study. Invest Ophthalmol. 1963;2:446.
31. Laties A., Liebman P., Campbell C. Photoreceptor orientation in the primate eye. Nature. 1968;218:172.
32. Laties A., Enoch J. An analysis of retinal receptor orientation. I. Angular relationship of neighboring photoreceptors. Invest Ophthalmol. 1971;10:69.
33. Arikawa K., Molday L.L., Molday R.S., et al. Localization of peripherin/RDS in the disk membranes of cone and rod photoreceptors: relationship to disk membrane morphogenesis and retinal degeneration. J Cell Biol. 1992;116(3):659.
34. Young R.W., Bok D. Participation of the retinal pigment epithelium in the rod outer segment renewal process. J Cell Biol. 1969;42:392.
35. Young R.W. The renewal of rod and cone outer segments in rhesus monkey. J Cell Biol. 1971;49:303.
36. Bok D. Retinal photoreceptor-pigment epithelium interactions. Invest Ophthalmol Vis Sci. 1985;26:1659.
37. Young R.W. Shedding of discs from rod outer segments in the rhesus monkey. J Ultrastruct Res. 1971;34:190.
38. LaVail M.M. Rod outer segment disk shedding in rat retina: relationship to cyclic lighting. Science. 1976;194:1071.
39. Basinger S., Hoffman R., Matthews M. Photoreceptor shedding is initiated by light in the frog retina. Science. 1978;194:1074.
40. Young R.W. The daily rhythm of shedding and degradation of rod and cone outer segment membranes in the chick retina. Invest Ophthalmol Vis Sci. 1976;17:105.
41. Migdale K., Herr S., Klug K., et al. Two ribbon synaptic units in rod photoreceptors of macaque, human, and cat. J Comp Neurol. 2003;455(1):100. (abstract)
42. Kolb H., Famiglietti E.V. Rod and cone pathways in retina of cat. Invest Ophthalmol Vis Sci. 1976;15:935.
43. Anderson D.H., Fisher S.K., Steinberg R.H. Mammalian cones: disc shedding, phagocytosis, and renewal. Invest Ophthalmol Vis Sci. 1978;17:117.
44. Walls G.L. Human rods and cones. Arch Ophthalmol. 1934;12:914.
45. Young R.W. A difference between rods and cones in the renewal of outer segment protein. Invest Ophthalmol. 1969;8:222.
46. Eckmiller M.S. Distal invaginations and the renewal of cone outer segments in anuran and monkey retinas. Cell Tissue Res. 1990;260:19.
47. Steinberg R.H. Phagocytosis by pigment epithelium of human retinal cones. Nature. 1974;25:305.
48. O’Day W.T., Young R.W. Rhythmic daily shedding of outer segment membranes by visual cells in the goldfish. J Cell Biol. 1978;76:593.
49. Raviola E., Gilula N.B. Intramembrane organization of specialized contacts in the outer plexiform layer of the retina: a freeze-fracture study in monkey and rabbits. J Cell Biol. 1975;65:192.
50. Ayoub G.S., Matthews G. Substance P modulates calcium current in retinal bipolar neurons. Vis Neurosci. 1992;8(6):539.
51. Kolb H., Linberg K.A., Fisher S.K. Neurons of the human retina: a Golgi study. J Comp Neurol. 1992;318(2):147.
52. Park S.S., Sigelman J., Gragoudas E.S. The anatomy and cell biology of the retina. Tasman W., Jaeger E.A., editors. Duane’s foundations of clinical ophthalmology, vol 1. Philadelphia: Lippincott, 1994.
53. Bloomfield S.A., Dacheux R.F. Rod vision: pathways and processing in the mammalian retina. Prog Retin Eye Res. 2001;20(3):351.
54. Boycott B.B., Hopkins J.M. Cone bipolar cells and cone synapses in the primate retina. Vis Neurosci. 1991;7(1-2):49.
55. Polyak S.L. The retina. Chicago: Chicago University Press; 1941.
56. Mariani A.P. Bipolar cells in the monkey retina selective for cones likely to be blue sensitive. Nature. 1984;308:184.
57. Witkorsky P. Functional anatomy of the retina. Tasman W., Jaeger E.A., editors. Duane’s foundations of clinical ophthalmology, vol 1. Philadelphia: Lippincott, 1994.
58. Warwick R. The eyeball. In: Warwick R., editor. Eugene Wolff’s anatomy of the eye and orbit. ed 7. Philadelphia: Saunders; 1976:99.
59. Kolb H., Dekorver L. Midget ganglion cells of the parafovea of the human retina: a study by electron microscopy and serial section reconstructions. J Comp Neurol. 1991;303(4):617.
60. Hart M. Visual adaptation. In: Hart W.M.Jr., editor. Adler’s physiology of the eye. ed 9. St Louis: Mosby; 1992:523.
61. Kolb H., Fernandez E., Nelson R.: The organization of the vertebrate retina, Webvision (website). 2011Webvision.med.utah.edu/.Accessed March 22, 2011
62. Kolb H., Ahuelt P., Fisher S.K., et al. Chromatic connectivity of the three horizontal cell types in the human retina. Invest Ophthalmol Vis Sci. 1989;30(suppl):348.
63. Dowling J.E., Boycott B.B. Neural connections of the retina: fine structure of the inner plexiform layer. Cold Spring Harb Symp Quant Biol. 1965;30:393.
64. Kolb H. Amacrine cells of the mammalian retina: neurocircuitry and functional roles. Eye. 1997;11:904.
65. Linberg K.A., Fisher S.K. Ultrastructure of the interplexiform cell of the human retina. Invest Ophthalmol Vis Sci. 1983;24(suppl):259.
66. Sarthy V., Ripps H. Structural organization of retinal glia. In: The retinal Müller cell: structure and function. New York: Kluwer Academic/Plenum Press; 2001.
67. Ogden T.E. Nerve fiber layer of the primate retina: thickness and glial contents. Vision Res. 1983;23:581.
68. Newman E., Reichenback A. The Müller cell: a functional element of the retina. Trends Neurosci. 1996;19(8):307.
69. Kuwabara T., Cogan D. Retinal glycogen. Arch Ophthalmol. 1961;66:680.
70. Newman E.A. Membrane physiology of retina glial (Müller) cells. J Neurosci. 1985;5:2225.
71. Reichenbach A., Stolzenburg J.U., Eberhardt W., et al. What do retinal Müller (glial) cells do for their neuronal “small siblings”? J Chem Neuroanat. 1993;6(4):201.
72. Ramírez J.M., Triviño A., Ramírez A.I., et al. Structural specializations of human retinal glial cells. Vision Res. 1996;36(14):2029.
73. LaCour M. The retinal pigment epithelium. In: Kaufman P.L., Alm A., editors. Adler’s physiology of the eye. ed 10. St Louis: Mosby; 2003:348.
74. Panda-Jonas S., Jonas J.B., Jakobczyk-Zmija M. Retinal pigment epithelial cell count, distribution, and correlations in normal human eyes. Am J Ophthalmol. 1996;121:181.
75. Zinn K.M., Benjamin-Henkind J. Retinal pigment epithelium. In: Jakobiec F.A., editor. Ocular anatomy, embryology, and teratology. Philadelphia: Harper & Row; 1982:533.
76. Usui S., Kamiyama Y., Ishii H., et al. Reconstruction of retinal horizontal cell responses by the ionic current model. Vision Res. 1996;36(12):1711. (abstract)
77. Kolb H. Organization of the outer plexiform layer of the primate retina: electron microscopy of Golgi-impregnated cells. Philos Trans R Soc Lond B Biol Sci. 1970;258:261.
78. la Cour M., Ehinger B. The Retina. In: The biology of the eye, Fischbarg J, ed. Amsterdam, the Netherlands. Elsevier; 2006:195-252.
79. Dowling J.E., Boycott B.B. Organization of the primate retina: electron microscopy. Proc R Soc Lond B Biol Sci. 1966;166:80.
80. Cunha-Va J.G. The blood-ocular barriers: past, present, and future. Doc Ophthalmol. 1997;93:149.
81. la Cour M., Tezel T. The retinal pigment epithelium. In Fischbarg J, ed. The biology of the eye. 2006. Elsevier, 253–271
82. la Cour M., Lin H., Kenyon E., et al. Lactate transport in freshly isolated human fetal retinal pigment epithelium. Invest Ophthalmol Vis Sci. 1994;35:434-442.
83. Strauss O. The retinal pigment epithelium in visual function. Physiol Rev. 2005;85:845-881.
84. Young R.W. Renewal systems in rods and cones. Ann Ophthalmol 1973:843-854.
85. Sparrow J.R., Nakanishi K., Parish C.A. The lipofuscin fluorophore A2E mediates blue light-induced damage to retinal pigmented epithelial cells, Invest Ophthalmol Vis Sci. 2000;41:1981-1989.
86. Bok D. The retinal pigment epithelium: a versatile partner in vision. J Cell Sci Suppl. 1993;17:189.
87. Grierson I., Hiscott P., Hogg P., et al. Development, repair and regeneration of the retinal pigment epithelium. Eye. 1994;8(pt 2):255.
88. Martini B., Pandey R., Ogden T.E., et al. Cultures of human retinal pigment epithelium. Modulation of extracellular matrix. Invest Ophthalmol Vis Sci. 1992;33(3):516.
89. Wiggs J.L. Molecular genetics of selected ocular disorders. In: Yanoff M., Duker J.S., editors. Ocular pathology (e-book). St Louis: Mosby, 2008.
90. Altawel M. Best disease, eMedicine (website). http://emedicine.medscape.com/article/1227128-overview. Accessed March 22, 2011
91. Marks W.B., Dobelle W.H., MacNichol E.F.Jr. Visual pigments of single primate cones. Science. 1964;43:1181.
92. Kolb H. The architecture of functional neural circuits in the vertebrate retina. Invest Ophthalmol Vis Sci. 1994;35(5):2385.
93. Horton J.C. The central visual pathways. In: Hart W.M.Jr., editor. Adler’s physiology of the eye. ed 9. St Louis: Mosby; 1992:728.
94. Østerberg G.A. Topography of the layer of rods and cones in the human retina. Acta Ophthalmol (Copenh). 1935;6:1.
95. Farber D.B., Flannery J.G., Lolley R.N., et al. Distribution patterns of photoreceptors, protein, and cyclic nucleotides in the human retina. Invest Ophthalmol Vis Sci. 1985;26:1558.
96. Curcio C.A., Sloan K.R., Kalina R.E., et al. Human photoreceptor topography. J Comp Neurol. 1990;292:497.
97. Oppel O. Untersuchungen Über Die Retinaganglien und Optikusfasern. Rohen J.W., editor. The structure of the eye, vol 2. Stuttgart, Germany: Verlag; 1965:97.
98. Jonas J.B., Gusek G.C., Naumann G.O. Optic disc, cup, and neuroretinal rim size, configuration and correlations in normal eyes. Invest Ophthalmol Vis Sci. 1988;29:1151.
99. Twig G., Levy H., Perlman I. Color opponency in horizontal cells of the vertebrate retina. Prog Retin Eye Res. 2003;22:31-68.
100. Kolb H. How the retina works. Am Sci. 2003;91:28-35.
101. Barnes S., Kelly M.E. Calcium channels at the photoreceptor synapse. Adv Exp Med Biol. 2002;514:465-476.
102. Arshavsky V.Y. Like night and day: rods and cones have different pigment regeneration pathways, Neuron. 2002;36:1-3.
103. Muniz A., Villazana-Espinoza E.T., Hatch A.L., et al. A novel cone visual cycle in the cone-dominated retina, Exp Eye Res. 2007;83:175-184.
104. Boycott B., Wässle H. Parallel processing in the mammalian retina, Invest Ophthalmol Vis Sci. 1999;40:1313-1327.
105. Wässle H., Boycott B.B. Functional architecture of the mammalian retina. Physiol Rev. 1991;71:447-480.
106. Slaughter M.M., Miller R.F. An excitatory amino acid antagonist blocks cone input to sign-conserving second-order retinal neurons. Science. 1983;219:1230-1232.
107. Dhingra A., Jiang M., Wang T.L., et al. Light response of retinal ON bipolar cells requires a specific splice variant of Galpha(o), J Neurosi. 2002;22:4878-4884.
108. Fahrenfort I., Klooster J., Sjoerdsma T., et al. The involvement of glutamate-gated channels in negative feedback from horizontal cells to cones, Prog Brain Res. 2005;147:219-229.
109. Kamermans M., Spekreijse H. The feedback pathway from horizontal cells to cones. A mini review with a look ahead. Vision Res. 1999;39:2449-2468.
110. Kolb H., Nelson R. Functional neurocircuitry of amacrine cells in the cat retina. Neurocircuitry of the retina. In: a Cajal memorial Eds. Gallego, A. Gouras, P. New York: Elsevier Press; 1985:215-232.
111. Jensen R.J., Daw N.W. Effects of dopamine and its agonists and antagonists on the receptive field properties of ganglion cells in the rabbit retina. Neuroscience. 1986;17:837-855.
112. Dowling J.E. Retinal neuromodulation: the role of dopamine, Vis Neurosci. 1991;7:87-97.
113. Wu S.M. Intracellular light responses and synaptic organization of the vertebrate retina. In: Kaufman P.L., Alm A., editors. Adler’s physiology of the eye. ed 10. St Louis: Mosby; 2003:422-438.
114. Reppert S.M., Weaver D.R., Rivkees S.A., et al. Putative melatonin receptors in human biological clock. Science. 1988;242:78-81.
115. Turner P.L., Manister M.A. Circadian photoreception: ageing and the eye’s important role in systemic health. Br J Ophthalmol. 2008;92:1439-1444.
116. Berson M. Phototransduction in ganglion-cell photoreceptors. Pflugers Arch. 2007;454:849-855.
117. Gioffi G.A., Grandtam E., Alm A. Ocular circulation In: Adler’s physiology of the eye. Kaufman P.L., Alm A., editors, ed 10. St Louis: Mosby; 2003:747-784.
118. Rapp L.M., Maple S.S., Choi J.H. Lutein and zeaxanthin concentrations in rod outer segment membranes from perifoveal and peripheral human retina. Invest Ophthalmol Vis Sci. 2000;41:1200.
119. Nussbaum J.J., Pruett R.C., Delori F.C. Historic perspectives. Macular yellow pigment. The first 200 years. Retina. 1981;1(14):296.
120. Ahnelt P.K. The photoreceptor mosaic. Eye. 1998;12:531.
121. Yamada E. Some structural features of the fovea centralis in the human retina. Arch Ophthalmol. 1969;82:151.
122. Kanski J.J. Clinical ophthalmology, ed 3. London: Butterworth- Heinemann; 1994. p 383
123. Pei T.F., Smelser G.K. Some fine structural features of the ora serrata region in primate eyes. Invest Ophthalmol Vis Sci. 1968;7:672.
124. Jonas J.B., Schmidt A.M., Müller-Bergh J.A., et al. Human optic nerve fiber count and optic disc size. Invest Ophthalmol Vis Sci. 2012;33(6):1992.
125. Quigley H.A., Brown A.E., Morrison J.D., et al. The size and shape of the optic disc in normal human eyes. Arch Ophthalmol. 1990;108(1):51.
126. Hogan M.J., Alvarado J.A., Weddell J.E., editors. Histology of the human eye. Philadelphia: Saunders; 1971:p 523.
127. Fantes F.E., Anderson D.R. Clinical histologic correlation of human peripapillary anatomy. Ophthalmology. 1989;96(1):20.
128. Alexander L.J. Diseases of the optic nerve. In: Bartlett J.D., Jaanus S.D., editors. Clinical ocular pharmacology. ed 2. Butterworth: Boston; 1989:690.
129. Hayreh S.S. Optic disc edema in raised intracranial pressure. Associated visual disturbances and their pathogenesis. Arch Ophthalmol. 1975;95:1566.
130. Wirtschafter J.D., Rizzo F.J., Smiley B.C. Optic nerve axoplasm and papilledema. Surv Ophthalmol. 1977;20:157.
131. Cogan D.G., Kuwabara T. The mural cell in perspective. Arch Ophthalmol. 1967;78:133.
132. Chakravarthy U., Gardiner T.A. Endothelium-derived agents in pericyte function/dysfunction. Prog Retin Eye Res. 1999;18(4):511.
133. Shakib M., Cunha-Vaz J.G. Studies on the permeability of the blood-retinal barrier. IV. Junctional complexes of the retinal vessels and their role in the permeability of the blood-retinal barrier. Exp Eye Res. 1966;5:229.
134. Bernstein M.H., Hollenberg M.J. Fine structure of the choriocapillaris and retinal capillaries. Invest Ophthalmol. 1965;4(6):1016.
135. Henkind P. Radial peripapillary capillaries of the retina, II. Anatomy-human and comparative. Br J Ophthalmol. 1967;51:115.
136. Hayreh S.S. The central artery of the retina: its role in the blood supply of the optic nerve. Br J Ophthalmol. 1963;47:651.
137. Gao H., Hollyfield J.G. Aging of the human retina. Differential loss of neurons and retinal pigment epithelial cells. Invest Ophthalmol Vis Sci. 1992;33:1-17.
138. Paasche G., Gärtner U., Germer A., et al. Mitochondria of retina Müller (glial) cells: the effects of aging and of application of free radical scavengers. Ophthalmic Res. 2000;32:229.
139. Bringmann A., Biedermann B., Schnurbusch U., et al. Age- and disease-related changes of calcium channel-mediated currents in human Müller glial cells. Invest Ophthalmol Vis Sci. 2000;14:2791.
140. Dohlman C.L., McCormick A.Q., Drance S.M. Aging of the optic nerve. Arch Ophthalmol. 1980;98:2053.
141. Yuodelis C, Hendrickson A: A qualitative and quantitative analysis of the human fovea during development, Vision Res 26:847, 1986.
142. Curcio C.A. Photoreceptor topography in ageing and age-related maculopathy. Eye. 2001;15(3):376.
143. Jackson G.R., Owsley C., Cordle E.P., et al. Aging and scotopic sensitivity. Vision Res. 1998;38:3655.
144. Eliasieh K., Liets L.C., Chalupa L.M. Cellular reorganization in the human retina during normal aging, Invest Ophthalmol Vis Sci. 2007;48:2824-2830.
145. Ramírez J.M., Ramírez A.I., Salazar J.J., et al. Changes of astrocytes in retinal ageing and age-related macular degeneration. Exp Eye Res. 2001;73:601.
146. Yoo S.H., Adamis A.P. Retinal manifestations of aging. Int Ophthalmol Clin. 1998;38(1):95.
147. Boulton M., Dayhaw-Barker P. The role of the retinal pigment epithelium: topographical variation and ageing changes. Eye. 2001;15:384.
148. Sparrow J.R., Boulton M. RPE lipofuscin and its role in retinal pathobiology, Exp Eye Res. 2005;80:595-606.
149. Curcio C.A., Saunders P.L., Younger P.W., et al. Peripapillary chorioretinal atrophy: Bruch’s membrane changes and photoreceptor loss. Ophthalmology. 2000;107:334.
150. Garway-Heath D.F., Wollstein G., Hitchings R.A. Aging changes of the optic nerve head in relation to open angle glaucoma. Br J Ophthalmol. 1997;81(10):840.
151. Parisi V., Restuccia R., Fattapposta F., et al. Morphological and functional retinal impairment in Alzheimer’s disease patients. Clin Neurophysiol. 2001;112(10):1860.
152. Hedges T.R.III, Perez Galves .S., Speigelman D., et al. Retinal nerve fiber layer abnormalities in Alzheimer’s disease. Acta Ophthalmol Scand. 1996;74(3):271.
153. Blanks J.C., Schmidt S.Y., Torigoe Y., et al. Retinal pathology in Alzheimer’s disease. II. Regional neuron loss and glial changes in GCL. Neurobiol Aging. 1996;17(3):385.
154. Justino L., Kergoat M., Bergman H., et al. Neuroretinal function is normal in early dementia of the Alzheimer type. Neurobiol Aging. 2001;22(4):691.
155. Blanks J.C., Torigoe Y., Hinton D.R., et al. Retinal pathology in Alzheimer’s disease. I. Ganglion cell loss in foveal/parafoveal retina. Neurobiol Aging. 1996;17(3):377.
156. Tsai C.S., Ritch R., Schwartz B., et al. Optic nerve head and nerve fiber layer in Alzheimer’s disease. Arch Ophthalmol. 1991;109:199.