CHAPTER 3 Respiratory Physiology in Infants and Children
Development of the respiratory system
Prenatal Development of the Lungs
The morphologic development of the human lung is seen as early as several weeks into the embryonic period and continues well into the first decade of postnatal life and beyond (Fig. 3-1). The fetal lungs begin to form within the first several weeks of the embryonic period, when the fetus is merely 3 mm in length. A groove appears in the ventral aspect of the foregut, creating a small pouch. The outgrowth of the endodermal cavity, with a mass of surrounding mesenchymal tissue, projects into the pleuroperitoneal cavity and forms lung buds. The future alveolar membranes and mucous glands are derived from the endoderm, whereas the cartilage, muscle, elastic tissue, and lymph vessels originate from the mesenchymal elements surrounding the lung buds (Emery, 1969).
During the pseudoglandular period, which extends until 17 weeks’ gestation, the budding of the bronchi and lung growth rapidly take place, forming a loose mass of connective tissue. The morphologic development of the human lung is illustrated in Figure 3-2. By 16 weeks’ gestation, preacinar branching of the airways (down to the terminal bronchioli) is complete (Reid, 1967). A disturbance of the free expansion of the developing lung during this stage, as occurs with diaphragmatic hernia, results in hypoplasia of the airways and lung tissue (Areechon and Reid, 1963). During the canalicular period, in midgestation, the future respiratory bronchioli develop as the relative amount of connective tissue diminishes. Capillaries grow adjacent to the respiratory bronchioli, and the whole lung becomes more vascular (Emery, 1969).
At about 24 weeks’ gestation, the lung enters the terminal sac period, which is characterized by the appearance of clusters of terminal air sacs, termed saccules, with flattened epithelium (Hislop and Reid, 1974). These saccules are large and irregular with thick septa and have few capillaries in comparison with the adult alveoli (Boyden, 1969). At about 26 to 28 weeks’ gestation, proliferation of the capillary network surrounding the terminal air spaces becomes sufficient for pulmonary gas exchange (Potter, 1961). These morphologic developments may occur earlier in some premature infants (born at 24 to 25 weeks’ gestation) who have survived through neonatal intensive care. Starting at 28 weeks’ gestation, air space wall thickness decreases rapidly. From this period onward toward term, there is further lengthening of saccules with possible growth of additional generations of air spaces. Some mammalian species, such as the rat, have no mature alveoli at birth (Burri, 1974). In contrast, alveolar development from saccules begins in some human fetuses as early as 32 weeks’ gestation, but alveoli are not uniformly present until 36 weeks’ gestation (Langston et al., 1984). Most alveolar formation in humans takes place postnatally during the first 12 to 18 months of postnatal life, and development of respiratory bronchioles by transformation of preexisting terminal airways does not take place until after birth (Langston et al., 1984).
The fetal lung produces a large quantity of liquid, which expands the airways while the larynx is closed. This expansion produces the growth factor, such as human bombesin, and helps to stimulate lung growth and development (Sunday et al., 1988). The lung fluid is periodically expelled into the uterine cavity and contributes about one third of the total amniotic fluid. Prenatal ligation or occlusion of the trachea was tried in the 1990s with some success for the treatment of the fetus with congenital diaphragmatic hernia (Harrison et al., 1993). This treatment causes the expansion of the fetal airways and results in an accelerated growth of the otherwise hypoplastic lung.
The type II pneumocytes, which produce pulmonary surfactant that forms the alveolar lining layer, reduces surface tension and stabilizes air spaces after air breathing, appear at about 24 to 26 weeks’ gestation but occasionally as early as 20 weeks (Spear et al., 1969; Lauweryns, 1970). Idiopathic (or infantile) respiratory distress syndrome (IRDS), also known as hyaline membrane disease (HMD), which occurs in premature infants, is caused by the immaturity of the lungs with their insufficient pulmonary surfactant production and their inactivation by plasma proteins exudating onto the alveolar surface (see Surface Activity and Pulmonary Surfactant).
Experimental evidence from animals indicates that certain pharmacologic agents such as cortisol and thyroxin administered to the mother or directly to the fetus accelerate the maturation of the lungs, resulting in the early appearance of type II pneumocytes and surfactant (deLemos et al., 1970; Motoyama et al., 1971; Wu et al., 1973; Smith and Bogues, 1982; Rooney, 1985). Liggins and Howie (1972) reported accelerated maturation of human fetal lungs after the administration of corticosteroids to mothers 24 to 48 hours before the delivery of premature babies. Despite initial concern that steroids might potentially be toxic to other organs of the fetus, particularly to the development of the central nervous system, prenatal glucocorticoid therapy has been used widely since the 1980s to induce lung maturation and surfactant synthesis in mothers at risk of premature delivery (Avery, 1984; Avery et al., 1986).
Neonatal respiratory adaptation
To introduce air into the fluid-filled lungs at birth, the newborn infant must overcome large surface force with the first few breaths. Usually a negative pressure of 30 cm H2O is necessary to introduce air into the fluid-filled lungs. In some normal full-term infants, even with sufficient surfactant, a force of as much as −70 cm H2O or more must be exerted to overcome the surface force (Fig. 3-3) (Karlberg et al., 1962). Usually fluid is rapidly expelled via the upper airways. The residual fluid leaves the lungs through the pulmonary capillaries and lymphatic channels over the first few days of life, and changes in compliance parallel this time course. All changes are delayed in the premature infant.
As the lungs expand with air, pulmonary vascular resistance decreases dramatically and pulmonary blood flow increases markedly, thus allowing gas exchange between alveolar air and pulmonary capillaries to occur. Changes in Po2, Pco2 (P stands for partial pressure), and pH are largely responsible for the dramatic decrease in pulmonary vascular resistance (Cook et al., 1963). The resultant large increases in pulmonary blood flow and the increase in left atrial pressure with a decrease in right atrial pressure reverse the pressure gradient across the atria and close (initially functionally and eventually anatomically) the foramen ovale, a right-to-left one-way valve. With these adjustments, the cardiopulmonary system approaches adult levels of ventilation/perfusion (balance within a few days (Nelson et al., 1962, 1963). The process of expansion of the lungs during the first few hours of life and the resultant circulatory adaptation for establishing pulmonary gas exchange are greatly influenced by the adequacy of pulmonary surfactant. It should be remembered that these changes are delayed in immature newborns.
Postnatal Development of the Lungs and Thorax
The development and growth of the lungs and surrounding thorax continue with amazing speed during the first year of life. Although the formation of the airway system all the way to the terminal bronchioles is complete by 16 weeks’ gestation, alveolar formation begins only at about 36 weeks’ gestation. At birth, the number of terminal air sacs (most of which are saccules) is between 20 and 50 million, only one tenth that of fully grown lungs of the child. Most postnatal development of alveoli from primitive saccules occurs during the first year and is essentially completed by 18 months of age (Langston et al., 1984). The morphologic and physiologic development of the lungs, however, continues throughout the first decade of life (Mansell et al., 1972).
In the neonate, static (elastic) recoil pressure of the lungs is very low (i.e., compliance, normalized for volume, is unusually high), which is not dissimilar to that of geriatric or emphysematous lungs, because the elastic fibers do not develop until the postnatal period (whereas elastic fibers in geriatric lungs are brittle and not functional) (Mansel et al., 1972; Fagan, 1976; Bryan and Wohl, 1986). In addition, the elastic recoil pressure of the infant’s thorax (chest wall) is extremely low because of its compliant cartilaginous rib cage with poorly developed thoracic muscle mass, which does not add rigidity. These unique characteristics make infants more prone to lung collapse, especially under general anesthesia when inspiratory muscles are markedly relaxed (see maintenance of FRC below). Throughout infancy and childhood, static recoil pressure of the lungs and thorax steadily increases (compliance, normalized for volume, decreases) toward normal values for young adults (Zapletal et al., 1971; Motoyama, 1977).
The actual size of the airway from the larynx to the bronchioles in infants and children, of course, is much smaller than in adolescents and adults, and flow resistance in absolute terms is extremely high. When normalized for lung volume or body size, however, infants’ airway size is relatively much larger; airway resistance is much lower than in adults (Polgar, 1967; Motoyama, 1977; Stocks and Godfrey, 1977). Infants and toddlers, however, are more prone to severe obstruction of the upper and lower airways because their absolute (not relative) airway diameters are much smaller than those in adults. As a consequence, relatively mild airway inflammation, edema, or secretions can lead to far greater degrees of airway obstruction than in adults (e.g., as with subglottic croup [laryngotracheobronchitis] or acute supraglottitis [epiglottitis]).
Prenatal Development of Breathing
Respiratory rhythmogenesis occurs long before parturition. Dawes and others (1970) were the first to demonstrate “breathing” activities with rhythmic diaphragmatic contractions in the fetal lamb. They found it to be episodic and highly variable in frequency. Boddy and Robinson (1971) recorded movement of the human fetal thorax with an ultrasound device and interpreted this as evidence of fetal breathing. Later studies have shown that during the last 10 weeks of pregnancy, fetal breathing is present approximately 30% of the time (Patrick et al., 1980). The breathing rate in the fetus at 30 to 31 weeks’ gestation is higher (58 breaths/min) than that in the near-term fetus (47 breaths/min). A significant increase in fetal breathing movements occurs 2 to 3 hours after a maternal meal and is correlated with the increase in the maternal blood sugar level (Patrick et al., 1980).
Spontaneous breathing movements in the fetus occur only during active, or rapid eye movement (REM), sleep and with low-voltage electrocortical activity, and they appear to be independent of the usual chemical and nonchemical stimuli of postnatal breathing (Dawes et al., 1972; Jansen and Chernick, 1983). Later studies, however, have clearly shown that the fetus can respond to chemical stimuli known to modify breathing patterns postnatally (Dawes et al., 1982; Jansen et al., 1982; Rigatto et al., 1988, 1992). In contrast, hypoxemia in the fetus abolishes, rather than stimulates, breathing movements. This may be related to the fact that hypoxemia diminishes the incidence of REM sleep (Boddy et al., 1974). It appears that normally low arterial oxygen tension, or Pao2 (19 to 23 mm Hg), in the fetus is a normal mechanism inhibiting breathing activities in utero (Rigatto, 1992). Severe hypoxia induces gasping, which is independent of the peripheral chemoreceptors and apparently independent of rhythmic fetal breathing (Jansen and Chernick, 1974).
The near-term fetus is relatively insensitive to Paco2 changes. Extreme hypercapnia (Paco2 greater than 60 mm Hg) in the fetal lamb, however, can induce rhythmic breathing movement that is preceded by a sudden activation of inspiratory muscle tone with expansion of the thorax and inward movement (inspiration) of amniotic fluid, as much as 30 to 40 mL/kg (an apparent increase in functional residual capacity [FRC]) (Motoyama, unpublished observation). When Pao2 was reduced, breathing activities ceased, and there was a reversal of the sequence of events noted above (i.e., relaxation of the thorax, decreased FRC as evidenced by outward flow of amniotic fluid) (Motoyama, 2001).
The Hering-Breuer (inflation) reflex is present in the fetus. Distention of the lungs by saline infusion slows the frequency of breathing (Dawes et al., 1982). Transection of the vagi, however, does not change the breathing pattern (Dawes, 1974).
Maternal ingestion of alcoholic beverages abolishes human fetal breathing for up to 1 hour. Fetal breathing movement is also abolished by maternal cigarette smoking. These effects may be related to fetal hypoxemia resulting from changes in placental circulation Jansen and Chernick, 1983). It is not clear why the fetus must “breathe” in utero, when gas exchange is handled by the placental circulation. Dawes (1974) suggested that fetal breathing might represent “prenatal practice” to ensure that the respiratory system is well developed and ready at the moment of birth. Another reason may be that the stretching of the airways and lung parenchyma is an important stimulus for lung development; bilateral phrenic nerve sectioning in the fetal lamb results in hypoplasia of the lungs (Alcorn et al., 1980).
Perinatal Adaptation of Breathing
During normal labor and vaginal delivery, the human fetus goes through a period of transient hypoxia, hypercapnia, and acidemia. The traditional view of the mechanism of the onset of breathing at birth until the 1980s was that the transient fetal asphyxia stimulates the chemoreceptors and produces gasping, which is followed by rhythmic breathing at birth that is aided by thermal, tactile, and other sensory stimuli. Subsequent studies have challenged this concept (Chernick et al., 1975; Baier et al., 1990; Rigatto, 1992). The current concept regarding the mechanism of continuous neonatal breathing is summarized in Box 3-1.
Box 3-1 Mechanism of Continuous Neonatal Breathing
Once the newborn has begun rhythmic breathing, ventilation is adjusted to achieve a lower Paco2 than is found in older children and adults (Table 3-1). The reason for this difference is not clear but most likely is related to a poor buffering capacity in the neonate and a ventilatory compensation for metabolic acidosis. The Pao2 of the infant approximates the adult level within a few weeks of birth (Nelson, 1976).
Control of breathing
Rhythmic contraction of the respiratory muscles is governed by the respiratory centers in the brainstem and tightly regulated by feedback systems so as to match the level of ventilation to metabolic needs (Fig. 3-4) (Cherniack and Pack, 1988). These feedback mechanisms include central and peripheral chemoreceptors, stretch receptors in the airways and lung parenchyma via the vagal afferent nerves, and segmental reflexes in the spinal cord provided by muscle spindles (Cherniack and Pack, 1988). The control of breathing comprises neural and chemical controls that are closely interrelated.
Neural Control of Breathing
Respiratory neurons in the medulla have inherent rhythmicity even when they are separated from the higher levels of the brainstem. In the cat, respiratory neurons are concentrated in two bilaterally symmetric areas in the medulla near the level of the obex. The dorsal respiratory group of neurons (DRG) is located in the dorsomedial medulla just ventrolateral to the nucleus tractus solitarius and contains predominantly inspiratory neurons. The ventral respiratory group of neurons (VRG), located in the ventrolateral medulla, consists of both inspiratory and expiratory neurons (Fig. 3-5) (von Euler, 1986; Tabatabai and Behnia, 1995; Berger, 2000).
Dorsal Respiratory Group of Neurons
The DRG is spatially associated with the tractus solitarius, which is the principal tract for the ninth and tenth cranial (glossopharyngeal and vagus) nerves. These nerves carry afferent fibers from the airways and lungs, heart, and peripheral arterial chemoreceptors. The DRG may constitute the initial intracranial site for processing some of these visceral sensory afferent inputs into a respiratory motor response (Berger, 2000).
On the basis of lung inflation, three types of neurons have been recognized in the DRG: type Iα (I stands for inspiratory), type Iβ, and pump (P) cells. Type Iα is inhibited by lung inflation (Cohen, 1981a). The axons of these neurons project to both the phrenic and the external (inspiratory) intercostal motoneurons of the spinal cord. Some type Iα neurons have medullary collaterals that terminate among the inspiratory and expiratory neurons of the ipsilateral VRG (Merrill, 1970).
The second type, Iβ, is excited by lung inflation and receives synaptic inputs from pulmonary stretch receptors. There is controversy as to whether Iβ axons project into the spinal cord respiratory neurons; the possible functional significance of such spinal projections is unknown. Both Iα and Iβ neurons receive excitatory inputs from the central pattern generator (or central inspiratory activity) for breathing, so that when lung inflation is terminated or the vagi in the neck are cut, the rhythmic firing activity of these neurons continues (Cohen, 198la, 1981b; Feldman and Speck, 1983).
The third type of neurons in the DRG receives no input from the central pattern generator. The impulse of these neurons, the P cells, closely follows lung inflation during either spontaneous or controlled ventilation (Berger, 1977). The P cells are assumed to be relay neurons for visceral afferent inputs (Berger, 2000).
The excitation of Iβ neurons by lung inflation is associated with the shortening of inspiratory duration. The Iβ neurons appear to promote inspiration-to-expiration phase-switching by inhibiting Iα neurons. This network seems to be responsible for the Hering-Breuer reflex inhibition of inspiration by lung inflation (Cohen, 198la, 1981b; von Euler, 1986, 1991).
The DRG thus functions as an important primary and possibly secondary relay site for visceral sensory inputs via glossopharyngeal and vagal afferent fibers. Because many of the inspiratory neurons in the DRG project to the contralateral spinal cord and make excitatory connections with phrenic motoneurons, the DRG serves as a source of inspiratory drive to phrenic and possibly to external intercostal motoneurons (Berger, 2000).
Ventral Respiratory Group of Neurons
The VRG extends from the rostral to the caudal end of the medulla and has three subdivisions (Fig. 3-5). The Bötzinger complex, located in the most rostral part of the medulla in the vicinity of the retrofacial nucleus, contains mostly expiratory neurons (Lipski and Merrill, 1980; Merrill et al., 1983). These neurons send inhibitory signals to DRG and VRG neurons and project into the phrenic motoneurons of the spinal cord, causing its inhibition (Bianchi and Barillot, 1982; Merrill et al., 1983). The physiologic significance of these connections may be to ensure inspiratory neuronal silence during expiration (reciprocal inhibition) and to contribute to the “inspiratory off-switch” mechanism.
The nucleus ambiguus (NA) and nucleus paraambigualis (NPA), lying side by side, occupy the middle portion of the VRG. Axons of the respiratory motoneurons originating from the NA project along with other vagal efferent fibers and innervate the laryngeal abductor (inspiratory) and adductor (expiratory) muscles via the recurrent laryngeal nerve (Barillot and Bianchi, 1971; Bastel and Lines, 1975). The NPA contains mainly inspiratory (Iγ) neurons, which respond to lung inflation in a manner similar to that of Iα neurons. The axons of these neurons project both to phrenic and external (inspiratory) intercostal motoneuron pools in the spinal cord. The nucleus retroambigualis (NRA) occupies the caudal part of the VRG and contains expiratory neurons whose axons project into the spinal motoneuron pools for the internal (expiratory) intercostal and abdominal muscles (Merrill, 1970; Miller et al., 1985).
The inspiratory neurons of the DRG send collateral fibers to the inspiratory neurons of the NPA in the VRG. These connections may provide the means for ipsilateral synchronization of the inspiratory activity between the neurons in the DRG and those in the VRG (Merrill, 1979, 1983). Furthermore, axon collaterals of the inspiratory neurons of the NPA on one side project to the inspiratory neurons of the contralateral NPA, and vice versa. These connections may be responsible for the bilateral synchronization of the medullary inspiratory motoneuron output, as evidenced by synchronous bilateral phrenic nerve activity (Merrill, 1979, 1983).
Pontine Respiratory Group of Neurons
In the dorsolateral portion of the rostral pons, both inspiratory and expiratory neurons have been found. Inspiratory neuronal activity is concentrated ventrolaterally in the region of the nucleus parabrachialis lateralis (NPBL). The expiratory activity is centered more medially in the vicinity of the nucleus parabrachialis medialis (NPBM) (Fig. 3-5) (Cohen, 1979; Mitchell and Berger, 1981). The respiratory neurons of these nuclei are referred to as the pontine respiratory group (PRG), which was, and sometimes still is, called the pneumotaxic center, although the term is generally considered obsolete (Feldman, 1986). There are reciprocal projections between the PRG neurons and the DRG and VRG neurons in the medulla. Electrical stimulation of the PRG produces rapid breathing with premature switching of respiratory phases, whereas transaction of the brainstem at a level caudal to the PRG prolongs inspiratory time (Cohen, 1971; Feldman and Gautier, 1976). Bilateral cervical vagotomies produce a similar pattern of slow breathing with prolonged inspiratory time; a combination of PRG lesions and bilateral vagotomy in the cat results in apneusis (apnea with sustained inspiration) or apneustic breathing (slow rhythmic respiration with marked increase end inspiratory hold) (Feldman and Gaultier, 1976; Feldman, 1986). The PRG probably plays a secondary role in modifying the inspiratory off-switch mechanism (Gautier and Bertrand, 1975; von Euler and Trippenbach, 1975).
Respiratory Rhythm Generation
Rhythmic breathing in mammals can occur in the absence of feedback from peripheral receptors. Because transection of the brain rostral to the pons or high spinal transection has little effect on the respiratory pattern, respiratory rhythmogenesis apparently takes place in the brainstem. The PRG, DRG, and VRG have all been considered as possible sites of the central pattern generator, although its exact location is still unknown (Cohen, 1981b; von Euler, 1983, 1986). A study with an in vitro brainstem preparation of neonatal rats has indicated that respiratory rhythm is generated in the small area in the ventrolateral medulla just rostral to the Bötzinger complex (pre-Bötzinger complex), which contains pacemaker neurons (Smith et al., 1991).
The pre-Bötzinger complex contains a group of neurons that is responsible for respiratory rhythmogenesis (Smith et al., 1991; Pierrefiche et al., 1998; Rekling and Feldman, 1998). Although the specific cellular mechanism responsible for rhythmogenesis is not known, two possible mechanisms have been proposed (Funk and Feldman, 1995; Ramirez and Richter, 1996). One hypothesis is that the pacemaker neurons possess intrinsic properties associated with various voltage- and time-dependent ion channels that are responsible for rhythm generation. Rhythmic activity in these neurons may depend on the presence of an input system that may be necessary to maintain the neuron’s membrane potential in a range in which the voltage-dependent properties of the cell’s ion channels result in rhythmic behavior. The network hypothesis is the alternative model in which the interaction between the neurons produces respiratory rhythmicity, such as reciprocal inhibition between inhibitory and excitatory neurons and recurrent excitation within any population of neurons (Berger, 2000). The output of this central pattern generator is influenced by various inputs from chemoreceptors (central and peripheral), mechanoreceptors (e.g., pulmonary receptors and muscle and joint receptors), thermoreceptors (central and peripheral), nociceptors, and higher central structures (such as the PRG). The function of these inputs is to modify the breathing pattern to meet and adjust to ever-changing metabolic and behavioral needs (Smith et al., 1991).
Airway and Pulmonary Receptors
Upper Airway Receptors
Stimulation of receptors in the nose can produce sneezing, apnea, changes in bronchomotor tone, and the diving reflex, which involves both the respiratory and the cardiovascular systems. Stimulation of the epipharynx causes the sniffing reflex, a short, strong inspiration to bring material (mucus, foreign body) in the epipharynx into the pharynx to be swallowed or expelled. The major role of receptors in the pharynx is associated with swallowing. It involves the inhibition of breathing, closure of the larynx, and coordinated contractions of pharyngeal muscles (Widdicombe, 1985; Nishino, 1993; Sant’Ambrogio et al., 1995).
The larynx has a rich innervation of receptors. The activation of these receptors can cause apnea, coughing, and changes in the ventilatory pattern (Widdicombe, 1981, 1985). These reflexes, which influence both the patency of the upper airway and the breathing pattern, are related to transmural pressure and air flow. Based on single-fiber action-potential recordings from the superior laryngeal nerve in the spontaneously breathing dog preparation in which the upper airway is isolated from the lower airways, three types of receptors have been identified: pressure receptors (most common, about 65%), “drive” (or irritant) receptors (stimulated by upper airway muscle activities), and flow or cold receptors (Sant’Ambrogio et al., 1983; Fisher et al., 1985). The laryngeal flow receptors show inspiratory modulation with room air breathing but become silent when inspired air temperature is raised to body temperature and 100% humidity or saturation (Sant’Ambrogio et al., 1985). The activity of pressure receptors increases markedly with upper airway obstruction (Sant’Ambrogio et al., 1983).
Tracheobronchial and Pulmonary Receptors
Three major types of tracheobronchial and pulmonary receptors have been recognized: slowly adapting (pulmonary stretch) receptors and rapidly adapting (irritant or deflation) receptors, both of which lead to myelinated vagal afferent fibers and unmyelinated C-fiber endings (J-receptors). Excellent reviews on pulmonary receptors have been published (Pack, 1981; Widdicombe, 1981; Sant’Ambrogio, 1982; Coleridge and Coleridge, 1984).
Slowly adapting (pulmonary stretch) receptors
Slowly adapting (pulmonary stretch) receptors (SARs) are mechanoreceptors that lie within the submucosal smooth muscles in the membranous posterior wall of the trachea and central airways (Bartlett et al., 1976). A small proportion of the receptors are located in the extrathoracic upper trachea (Berger, 2000). SARs are activated by the distention of the airways during lung inflation and inhibit inspiratory activity (Hering-Breuer inflation reflex), whereas they show little response to steady levels of lung inflation. The Hering-Breuer reflex also produces dilation of the upper airways from the larynx to the bronchi. Although SARs are predominantly mechanoreceptors, hypocapnia stimulates their discharge, and hypercapnia inhibits it (Pack, 1981). In addition, SARs are thought to be responsible for the accelerated heart rate and systemic vasoconstriction observed with moderate lung inflation (Widdicombe, 1974). These effects are abolished by bilateral vagotomy.
Studies by Clark and von Euler (1972) have demonstrated the importance of the inflation reflex in adjusting the pattern of ventilation in the cat and the human. In cats anesthetized with pentobarbital, inspiratory time decreases as tidal volume increases with hypercapnia, indicating the presence of the inflation reflex in the normal tidal volume range. Clark and von Euler demonstrated an inverse hyperbolic relationship between the tidal volume and inspiratory time. In the adult human, inspiratory time is independent of tidal volume until the latter increases to about twice the normal tidal volume, when the inflation reflex appears (Fig. 3-6). In the newborn, particularly the premature newborn, the inflation reflex is present in the eupneic range for a few months (Olinsky et al., 1974).
Apnea, commonly observed in adult patients at the end of surgery and anesthesia with the endotracheal tube cuff still inflated, may be related to the inflation reflex, because the trachea has a high concentration of stretch receptors (Bartlett et al., 1976; Sant’Ambrogio, 1982). Deflation of the cuff promptly restores rhythmic spontaneous ventilation.
Rapidly adapting (irritant) receptors
Rapidly adapting (irritant) receptors (RARs) are located superficially within the airway epithelial cells, mostly in the region of the carina and the large bronchi (Pack, 1981; Sant’Ambrogio, 1982). RARs respond to both mechanical and chemical stimuli. In contrast to SARs, RARs adapt rapidly to large lung inflation, distortion, or deflation, thus possessing marked dynamic sensitivity (Pack, 1981). RARs are stimulated by cigarette smoke, ammonia, and other irritant gases including inhaled anesthetics, with significant interindividual variability (Sampson and Vidruk, 1975). RARs are stimulated more consistently by histamine and prostaglandins, suggesting their role in response to pathologic states (Coleridge et al., 1976; Sampson and Vidruk, 1977; Vidruk et al., 1977; Berger, 2000). The activation of RARs in the large airways may be responsible for various reflexes, including coughing, bronchoconstriction, and mucus secretion. Stimulation of RARs in the periphery of the lungs may produce hyperpnea. Because RARs are stimulated by deflation of the lungs to produce hyperpnea in animals, they are considered to play an important role in the Hering-Breuer deflation reflex (Sellick and Widdicombe, 1970). This reflex, if it exists in humans, may partly account for increased respiratory drive when the lung volume is abnormally decreased, as in premature infants with IRDS and in pneumothorax.
When vagal conduction is partially blocked by cold, inflation of the lung produces prolonged contraction of the diaphragm and deep inspiration instead of inspiratory inhibition. This reflex, the paradoxical reflex of Head, is most likely mediated by RARs. It may be related to the complementary cycle of respiration, or the sigh mechanism, that functions to reinflate and reaerate parts of the lungs that have collapsed because of increased surface force during quiet, shallow breathing (Mead and Collier, 1959). In the newborn, inflation of the lungs initiates gasping. This mechanism, which was considered to be analogous to the paradoxical reflex of Head, may help to inflate unaerated portions of the newborn lung (Cross et al., 1960).
C-Fiber endings
Most afferent axons arising from the lungs, heart, and other abdominal viscera are slow conducting (slower than 2.5 m/sec), unmyelinated vagal fibers (C-fibers). Extensive studies by Paintal (1973) have suggested the presence of receptors supposedly located near the pulmonary or capillary wall (juxtapulmonary capillary or J-receptors) innervated by such C-fibers. C-fiber endings are stimulated by pulmonary congestion, pulmonary edema, pulmonary microemboli, and irritant gases such as anesthetics. Such stimulation causes apnea followed by rapid, shallow breathing, hypotension, and bradycardia. Stimulation of J-receptors also produces bronchoconstriction and increases mucus secretion. All these responses are abolished by bilateral vagotomy. In addition, stimulation of C-fiber endings can provoke severe reflex contraction of the laryngeal muscles, which may be partly responsible for the laryngospasm observed during induction of anesthesia with isoflurane or halothane.
In addition to receptors within the lung parenchyma (pulmonary C-fiber endings), there appear to be similar nonmyelinated nerve endings in the bronchial wall (bronchial C-fiber endings) (Coleridge and Coleridge, 1984). Both chemical and, to a lesser degree, mechanical stimuli excite these bronchial C-fiber endings. They are also stimulated by endogenous mediators of inflammation, including histamine, prostaglandins, serotonin, and bradykinin. Such stimulation may be a mechanism of C-fiber involvement in disease states such as pulmonary edema, pulmonary embolism, and asthma (Coleridge and Coleridge, 1984).
The inhalation of irritant gases or particles causes a sensation of tightness or distress in the chest, probably caused by its activation of pulmonary receptors. The pulmonary receptors may contribute to the sensation of dyspnea in lung congestion, atelectasis, and pulmonary edema. Bilateral vagal blockade in patients with lung disease abolished dyspneic sensation and increased breath-holding time (Noble et al., 1970).
Chest-Wall Receptors
The chest-wall muscles, including the diaphragm and the intercostal muscles, contain various types of receptors that can produce respiratory reflexes. This subject has been reviewed extensively (Newsom-Davis, 1974; Duron, 1981). The two types of receptors that have been most extensively studied are muscle spindles, which lie parallel to the extrafusal muscle fibers, and the Golgi tendon organs, which lie in series with the muscle fibers (Berger, 2000).
Intercostal muscles have a density of muscle spindles comparable with those of other skeletal muscles. The arrangement of muscle spindles is appropriate for the respiratory muscle load-compensation mechanism (Berger, 2000). By comparison with the intercostal muscles, the diaphragm has a very low density of muscle spindles and is poorly innervated by the γ-motoneurons. Reflex excitation of the diaphragm, however, can be achieved via proprioceptive excitation within the intercostal system (Decima and von Euler, 1969).
Golgi tendon organs are located at the point of insertion of the muscle fiber into its tendon and, like muscle spindles, are a slowly adapting mechanoreceptor. Activation of the Golgi tendon organs inhibits the homonymous motoneurons, possibly preventing the muscle from being overloaded (Berger, 2000). In the intercostal muscles, fewer Golgi tendon organs are present than muscle spindles, whereas the ratio is reversed in the diaphragm.
Chemical Control of Breathing
Regulation of alveolar ventilation and maintenance of normal arterial Pco2, pH, and Po2 are the principal functions of the medullary and peripheral chemoreceptors (Leusen, 1972).
Central Chemoreceptors
The medullary, or central, chemoreceptors, located near the surface of the ventrolateral medulla, are anatomically separated from the medullary respiratory center (Fig. 3-7). They respond to changes in hydrogen ion concentration in the adjacent cerebrospinal fluid rather than to changes in arterial Pco2 or pH (Pappenheimer et al., 1965). Since CO2 rapidly passes through the blood-brain barrier into the cerebrospinal fluid, which has poor buffering capacity, the medullary chemoreceptors are readily stimulated by respiratory acidemia. In contrast, ventilatory responses of the medullary chemoreceptors to acute metabolic acidemia and alkalemia are limited because changes in the hydrogen ion concentration in arterial blood are not rapidly transmitted to the cerebrospinal fluid. In chronic acid-base disturbances, the pH of cerebrospinal fluid (and presumably that of interstitial fluid) surrounding the medullary chemoreceptors is generally maintained close to the normal value of about 7.3 regardless of arterial pH (Mitchell et al., 1965). Under these circumstances, ventilation becomes more dependent on the hypoxic response of peripheral chemoreceptors.
Peripheral Chemoreceptors
The carotid bodies, located near the bifurcation of the common carotid artery, react rapidly to changes in Pao2 and pH. Their contribution to the respiratory drive amounts to about 15% of resting ventilation (Severinghaus, 1972). The carotid body has three types of neural components: type I (glomus) cells, presumably the primary site of chemotransduction; type II (sheath) cells; and sensory nerve fibers (McDonald, 1981). Sensory nerve fibers originate from terminals in apposition to the glomus cells, travel via the carotid sinus nerve to join the glossopharyngeal nerve, and then enter the brainstem. The sheath cells envelop both the glomus cells and the sensory nerve terminals. A variety of neurochemicals have been found in the carotid body, including acetylcholine, dopamine, substance P, enkephalins, and vasoactive intestinal peptide. The exact functions of these cell types and the mechanisms of chemotransduction and the specific roles of these neurochemicals have not been well established (Berger, 2000).
The carotid bodies are perfused with extremely high levels of blood flow and respond rapidly to an oscillating Pao2 rather than a constant Pao2 at the same mean values (Dutton et al., 1964; Fenner et al., 1968). This mechanism may be partly responsible for hyperventilation during exercise.
The primary role of peripheral chemoreceptors is their response to changes in arterial Po2. Moderate to severe hypoxemia (Pao2 less than 60 mm Hg) results in a significant increase in ventilation in all age groups except for newborn, particularly premature, infants, whose ventilation is decreased by hypoxemia (Dripps and Comroe, 1947; Rigatto et al., 1975b). Peripheral chemoreceptors are also partly responsible for hyperventilation in hypotensive patients. Respiratory stimulation is absent in certain states of tissue hypoxia, such as moderate to severe anemia and carbon monoxide poisoning; despite a decrease in oxygen content, Pao2 in the carotid bodies is maintained near normal levels, so that the chemoreceptors are not stimulated.
In acute hypoxemia, the ventilatory response via the peripheral chemoreceptors is partially opposed by hypocapnia, which depresses the medullary chemoreceptors. When a hypoxemic environment persists for a few days, for example, during an ascent to high altitude, ventilation increases further as cerebrospinal fluid bicarbonate decreases and pH returns toward normal (Severinghaus et al., 1963). However, later studies demonstrated that the return of cerebrospinal fluid pH toward normal is incomplete, and a secondary increase in ventilation precedes the decrease in pH, indicating that some other mechanisms are involved (Bureau and Bouverot, 1975; Foster et al., 1975). In chronic hypoxemia that lasts for a number of years, the carotid bodies initially exhibit some adaptation to hypoxemia and then gradually lose their hypoxic response. In people native to high altitudes, the blunted response of carotid chemoreceptors to hypoxemia takes 10 to 15 years to develop and is sustained thereafter (Sorensen and Severinghaus, 1968; Lahiri et al., 1978). In cyanotic heart diseases, the hypoxic response is lost much sooner but returns after surgical correction of the right-to-left shunts (Edelman et al., 1970).
Response to Carbon Dioxide
The graphic demonstration of relations between the alveolar or arterial Pco2 and the minute ventilation () is commonly known as the CO2 response curve (Fig. 3-8). This curve normally reflects the response of the chemoreceptors and respiratory center to CO2. The CO2 response curve is a useful means for evaluation of the chemical control of breathing, provided that the mechanical properties of the respiratory system, including the neuromuscular transmission, respiratory muscles, thorax, and lungs, are intact. In normal persons, ventilation increases more or less linearly as the inspired concentration of carbon dioxide increases up to 9% to 10%, above which ventilation starts to decrease (Dripps and Comroe, 1947). Under hypoxemic conditions the CO2 response is potentiated, primarily via carotid body stimulation, resulting in a shift to the left of the CO2 response curve (Fig. 3-8) (Nielsen and Smith, 1951). On the other hand, anesthetics, opioids, and barbiturates in general depress the medullary chemoreceptors and, by decreasing the slope, shift the CO2 response curve progressively to the right as the anesthetic concentration increases (Fig. 3-9) (Munson et al., 1966).
A shift to the right of the CO2 response curve in an awake human may be caused by decreased chemoreceptor sensitivity to CO2, as seen in patients whose carotid bodies had been destroyed (Wade et al., 1970). It may also be caused by lung disease and resultant mechanical failure to increase ventilation despite intact neuronal response to carbon dioxide. In patients with various central nervous system dysfunctions, the CO2 response may be partially or completely lost (Ondine’s curse) (Severinghaus and Mitchell, 1962). In the awake state, these patients have chronic hypoventilation but can breathe on command. During sleep, they further hypoventilate or become apneic to the point of CO2 narcosis and death unless mechanically ventilated or implanted with a phrenic pacemaker (Glenn et al., 1973).
It has been difficult to separate the neuronal component from the mechanical failure of the lungs and thorax, because the two factors often coexist in patients with chronic lung diseases (Guz et al., 1970). Whitelaw and others (1975) demonstrated that occlusion pressure at 0.1 second (P0.1, or the negative mouth pressure generated by inspiratory effort against airway occlusion at FRC) correlates well with neuronal (phrenic) discharges but is uninfluenced by mechanical properties of the lungs and thorax. The occlusion pressure is a useful means for the clinical evaluation of the ventilatory drive.
Milic-Emili and Grunstein (1975) proposed that ventilatory response to CO2 be analyzed in terms of the mean inspiratory flow (Vt/Ti, where Vt is tidal volume and Ti is the inspiratory time) and in terms of the ratio of inspiratory time to total ventilatory cycle duration or respiratory duty cycle (Ti/Ttot) (Fig. 3-10). Because the tidal volume is equal to Vt/Ti × Ti and respiratory frequency (f) is I/Ttot, ventilation can be expressed as follows:
The advantage of analyzing the ventilatory response in this fashion is that Vt/Ti is an index of inspiratory drive, which is independent of the timing element. The tidal volume, on the other hand, is time dependent, because it is (Vt/Ti) × TI. The second parameter, Ti/Ttot, is a dimensionless index of effective respiratory timing (respiratory duty cycle) that is determined by the vagal afferent or central inspiratory off-switch mechanism or by both (Bradley et al., 1975). From this equation, it is apparent that in respiratory disease or under anesthesia, changes in pulmonary ventilation may result from a change in Vt/Ti, Ti/Ttot, or both. A reduction in Ti/Ttot indicates that the relative duration of inspiration decreased or that expiration increased. Such a reduction in the Ti/Ttot ratio may result from changes in central or peripheral mechanisms. In contrast, a reduction in Vt/Ti may indicate a decrease in the medullary inspiratory drive or neuromuscular transmission or an increase in inspiratory impedance (i.e., increased flow resistance, decreased compliance, or both). By relating the mouth occlusion pressure to Vt/Ti, it becomes clinically possible to determine whether changes in the mechanics of the respiratory system contribute to the reduction in Vt/Ti (Milic-Emili, 1977).
Analysis of inspiratory and expiratory durations provides useful information on the mechanism of anesthetic effects on ventilation. Figure 3-11 illustrates the effect of pentobarbital, which depresses minute ventilation, and diethyl ether, which “stimulates” ventilation in newborn rabbits. With both anesthetics the mean inspiratory flow (Vt/Ti) did not change, but Vt decreased because Ti was shortened. With pentobarbital, however, Te was prolonged disproportionately, and Ti/Ttot and frequency decreased; consequently, minute ventilation was decreased. With ether, on the other hand, ventilation increased as the result of disproportionate decrease in Te and consequent increases in Ti/Ttot and frequency (Milic-Emili, 1977).
Control of Breathing in Neonates and Infants
Response to Hypoxemia in Infants
During the first 2 to 3 weeks of age, both full-term and premature infants in a warm environment respond to hypoxemia (15% oxygen) with a transient increase in ventilation followed by sustained ventilatory depression (Brady and Ceruti, 1966; Rigatto and Brady, 1972a, 1972b; Rigatto et al., 1975a) (Fig. 3-12). In infants born at 32 to 37 weeks’ gestation, the initial period of transient hyperpnea is abolished in a cool environment, indicating the importance of maintaining a neutral thermal environment (Cross and Oppe, 1952; Ceruti, 1966; Perlstein et al., 1970). When 100% oxygen is given, a transient decrease in ventilation is followed by sustained hyperventilation. This ventilatory response to oxygen is similar to that of the fetus and is different from that of the adult, in whom a sustained decrease in ventilation is followed by little or no increase in ventilation (Dripps and Comroe, 1947). By 3 weeks after birth, hypoxemia induces sustained hyperventilation, as it does in older children and adults.
The biphasic depression in ventilation has been attributed to central depression rather than to depression of peripheral chemoreceptors (Albersheim et al., 1976). In newborn monkeys, however, tracheal occlusion pressure, an index of central neural drive, and diaphragmatic electromyographic output were increased above the control level during both the hyperpneic and the hypopneic phases in response to hypoxic gas mixture (LaFramboise et al., 1981; LaFramboise and Woodrum, 1985). These findings imply that the biphasic ventilatory response to hypoxemia results from changes in the mechanics of the respiratory system (thoracic stiffness or airway obstruction), rather than from neuronal depression, as has been assumed (Jansen and Chernick, 1983). Premature infants continue to show a biphasic response to hypoxemia even at 25 days after birth (Rigatto, 1986). Thus, in terms of a proper response to hypoxemic challenge, maturation of the respiratory system may be related to postconceptional rather than postnatal age.
Response to Carbon Dioxide in Infants
Newborn infants respond to hypercapnia by increasing ventilation but less so than do older infants. The slope of the CO2 response curve increases appreciably with gestational age as well as with postnatal age, independent of postconceptional age (Rigatto et al., 1975a, 1975b, 1982; Frantz et al., 1976). This increase in slope may represent an increase in chemosensitivity, but it may also result from more effective mechanics of the respiratory system. In adults the CO2 response curve both increases in slope and shifts to the left with the severity of hypoxemia (Fig. 3-8). In contrast, in newborn infants breathing 15% oxygen, the CO2 response curve decreases in slope and shifts to the right (Fig. 3-13). Inversely, hyperoxemia increases the slope and shifts the curve to the left (Rigatto et al., 1975a).
Upper Airway Receptor Responses in the Neonatal Period
Newborn animals are particularly sensitive to the stimulation of the superior laryngeal nerve either directly or through the receptors (such as water in the larynx), which results in ventilatory depression or apnea. In anesthetized newborn puppies and kittens, negative pressure or air flow through the larynx isolated from the lower airways produced apnea or significant prolongation of inspiratory and expiratory time and a decrease in tidal volume, whereas similar stimulation caused little or no effect in 4- to 5-week-old puppies or in adult dogs and cats (Al-Shway and Mortola, 1982; Fisher et al., 1985).
In a similar preparation using puppies anesthetized with pentobarbital, water in the laryngeal lumen produced apnea, whereas phosphate buffer with sodium chloride and neutral pH did not. The principal stimulus for the apneic reflex was the absence or reduced concentrations of chloride ion (Boggs and Bartlett, 1982). In awake newborn piglets, direct electric stimulation of the superior laryngeal nerve caused periodic breathing and apnea associated with marked decreases in respiratory frequency, hypoxemia, and hypercapnia with minimal cardiovascular effects. Breathing during superior laryngeal nerve stimulation was sustained by an arousal system (Donnelly and Haddad, 1986). The strong inhibitory responses elicited in newborn animals by various upper airway receptor stimulations have been attributed to the immaturity of the central nervous system (Lucier et al., 1979; Boggs and Bartlett, 1982).
Active Vs. Quiet Sleep
During the early postnatal period, full-term infants spend 50% of their sleep time in active or REM sleep compared with 20% REM sleep in adults (Stern et al., 1969; Rigatto et al., 1982). Wakefulness rarely occurs in neonates. Premature neonates stay in REM sleep most of the time, and quiet (non-REM) sleep is difficult to define before 32 weeks’ postconception (Rigatto, 1992). Neonates, particularly prematurely born neonates, therefore breathe irregularly.
Neurologic and chemical control of breathing in infants is related to the state of sleep (Scher et al., 1992). During quiet sleep, breathing is regulated primarily by the medullary respiratory centers and breathing is regular with respect to timing as well as amplitude and is tightly linked to chemoreceptor input (Bryan and Wohl, 1986). During REM sleep, however, breathing is controlled primarily by the behavioral system and is irregular with respect to timing and amplitude (Phillipson, 1994).
Periodic Breathing and Apnea
Periodic Breathing
Periodic breathing, in which breathing is interposed with repetitive short apneic spells lasting 5 to 10 seconds with minimal hemoglobin desaturation or cyanosis, occurs normally even in healthy neonates and young infants during wakefulness, REM sleep, and non-REM sleep (Rigatto et al., 1982). Periodic breathing tends to be more regular in quiet sleep than in active sleep and has been observed more often during active sleep (Rigatto et al., 1982) or during quiet sleep (Kelly et al., 1985). Minute ventilation increases during REM sleep due to increases in respiratory frequency with little change in tidal volume (Kalapezi et al., 1981; Rigatto et al., 1982).
An addition of 2% to 4% CO2 to the inspired gas mixture abolishes periodic breathing, probably by causing respiratory stimulation (Chernick et al., 1964). Nevertheless, the ventilatory response to hypercapnia seems to be diminished during periodic breathing (Rigatto and Brady, 1972a). The decreased hypercapnic response appears to result from changes in respiratory mechanics rather than from a reduction in chemosensitivity, because respiratory center output as determined by airway occlusion pressure is greater during REM sleep than during non-REM sleep.
The incidence of periodic breathing was reported to be 78% in full-term neonates, whereas the incidence was much higher (93%) in preterm infants (mean postconceptional age of 37.5 weeks) (Kelly et al., 1985; Glotzbach et al., 1989). The incidence of periodic breathing diminishes with increasing postconceptual age and decreases to 29% by 10 to 12 months of age (Fenner et al., 1973; Kelly et al., 1985).
Apnea of Prematurity and Hypoxia
Central apnea of infancy is defined as cessation of breathing for 15 seconds or longer or a shorter respiratory pause associated with bradycardia (heart rate less than 100 beats/min, cyanosis, or pallor (Brooks, 1982). Apnea is common in preterm infants and may be related to an immature respiratory control mechanism (Jansen and Chernick, 1983). Most preterm infants with a birth weight of less than 2 kg have apneic spells at some time (Spitzer and Fox, 1984). Glotzbach and others (1989) reported a 55% incidence of central apnea in preterm infants, whereas it was rarely found in full-term infants (Kelly et al., 1985). These studies, however, were based on a relatively small number of infants admitted to a single institution.
The report by the Collaborative Home Infant Monitoring Evaluation (CHIME) Study Group has shed a new light on the understanding of the incidence and extent of apnea in infancy (Hunt et al., 1999; Ramanathan et al., 2001). The CHIME study was based on the recordings of respiratory inductive plethysmography, electrocardiography (ECG), and pulse oximetry in normal infants and those with increased risk of sudden infant death syndrome (SIDS), and it involved a total of 1079 infants during the first 6 months after birth (Hunt et al., 1999; Ramanathan et al., 2001). This report has revealed evidence that the control of breathing and oxygenation during sleep in healthy term infants are not as precise as have been assumed. Normal infants, up to 2% to 3%, commonly have prolonged central, obstructive, or mixed apnea lasting up to 30 seconds, which is associated with oxygen desaturation (Ramanathan et al., 2001). With a simple upper respiratory infection, prolonged obstructive sleep apneas were recorded in a few normal full-term infants but were present in 15% to 30% of preterm infants. The risk of having such episodes was 20 to 30 times higher among preterm infants than in full-term infants before 43 weeks’ postconception (Hunt et al., 1999). Healthy term infants had an average baseline SpO2 of 98% throughout the recorded period. However, hypoxemia (SpO2 less than 90%, occasionally in the 70% to 80% range) occurred in 59% of these normal term infants in 0.6% of recorded cases (Hunt et al., 1999). Thus, levels of hypoxemia or hypoxia previously considered pathologic are relatively common occurrences among normal infants.
Apparent life-threatening events (ALTE) are characterized by an episode of sudden onset characterized by color change (cyanosis or pallor), tone change (limpness or rarely stiffness), and apnea, which requires immediate resuscitation to revive the infant and restore normal breathing (National Heart, Lung, and Blood Consensus Development Conference, National Institutes of Health, 1987). The incidence of ALTE is as high as 3% and may occur in previously healthy infants. Overnight polysomnography (PSG) is particularly useful in the evaluation of infants with a history of unexplained apnea. Treatable pathologic conditions, however, were found only in about 30% of infants, and thus normal PSG results are not necessarily diagnostic for the purpose of ruling out ALTE (Ramanathan et al., 2001).
Postoperative Apnea
Life-threatening apnea has been reported postoperatively in prematurely born infants earlier than 41 weeks’ postconception, particularly in those with a history of apneic spells after simple surgical procedures, such as inguinal herniorrhaphy, and can occur up to 12 hours postoperatively (Steward, 1982; Liu et al., 1983). These reports resulted in a general consensus among the pediatric anesthesiologists that infants younger than 44 weeks’ postconception be admitted for overnight observation after inguinal hernia repair for safety. In a subsequent report, including various surgical procedures, apnea was reported in 4 of 18 prematurely born infants who were 49 to 55 weeks’ postconceptional age (Kurth et al., 1987). The authors of this report proposed that premature infants younger than 60 weeks’ postconception should be admitted for overnight observation, which raised a controversy as to what postconceptional age is safe and appropriate for the same-day discharge from the hospital for the prematurely born infant (Kurth et al., 1987). Malviya and others (1993) analyzed the relationship between the incidence of postoperative apnea and maturation. They reported a high incidence of postoperative apnea (26%) in infants younger than 44 weeks’ postconception, whereas the incidence of apnea in those older than 44 weeks was only 3%.
Subsequently, Coté and others (1995) performed a meta-analysis of the data from previously published studies of postoperative apnea in expremature infants after inguinal hernia repairs. They concluded that postoperative apnea was strongly and inversely correlated to both gestational age as well as postconceptual age and was associated with a previous history of apnea. The probability of postoperative apnea in those older than 44 weeks postconceptual age decreases significantly (to 5%) but still exists. Another important finding of this classic paper was that postoperative hypoxemia, hypothermia, and (most importantly) anemia (hematocrit value of less than 30) are significant risk factors regardless of gestational or postconceptual age (see Chapter 13, Induction, Maintenance, and Recovery). Most of these studies occurred in the period when infants were predominantly anesthetized with halothane and without regional (caudal) block to maintain a lighter level of anesthesia with spontaneous breathing during surgery. Postoperative apnea still exists with newer anesthetic agents (e.g., sevoflurane or desflurane), but appears to occur much less often.
Both theophylline and caffeine have been effective in reducing apneic spells in preterm infants (Aranda and Trumen, 1979). Caffeine is especially useful for premature infants during the postanesthetic period (Welborn et al., 1988). Xanthine derivatives are known to prevent muscle fatigue, and their respiratory stimulation in the premature infant may occur via both central and peripheral mechanisms (Aubier et al., 1981).
Maintenance of the Upper Airway and Airway Protective Reflexes
Pharyngeal Airway
The pharyngeal airway, unlike the laryngeal airway, is not supported by a rigid bony or cartilaginous structure. Its wall consists of soft tissues and is surrounded by muscles for breathing and for swallowing and is contained in a fixed bony structure (i.e., the maxilla, mandible, and spine) (Isono, 2006). Anatomic imbalance between the bony structure (the container—micrognathia, facial anomalies) and the amount of the soft tissues (the content—macroglossia, adenotonsillar hypertrophy, obesity) would result in the pharyngeal airway narrowing and obstruction (Fig. 3-14) (Isono, 2006).
Even the normal pharyngeal airway is easily obstructed by the relaxation of the velopharynx (soft palate), posterior displacement of the mandible (and the base of the tongue) in the supine position during sleep, flexion of the neck, or external compression over the hyoid bone. The pharyngeal airway also is easily collapsed by negative pressure within the pharyngeal lumen created by inspiratory effort, especially when airway-maintaining muscles are depressed or paralyzed (Issa and Sullivan, 1984; Reed et al., 1985; Roberts et al., 1985). In neonates, with a relatively hypoplastic mandible, the oropharynx and the entrance to the larynx at the level of the aryepiglottic folds are the areas most easily collapsed (Reed et al., 1985).
Mechanical support to sustain the patency of the pharynx against the collapsing force of luminal negative pressure during inspiration is given by both the sustained muscle tension and cyclic contraction of the pharyngeal dilator muscles, acting synchronously with the contraction of the diaphragm. These include the genioglossus, geniohyoid, sternohyoid, sternothyroid, and thyrohyoid muscles (Fig. 3-15) (Bartlett et al., 1973; Pack et al., 1988; Thach, 1992). Similar phasic activities have been recorded in the scalene and sternomastoid muscles in humans (Onal et al., 1981; Drummond, 1987).
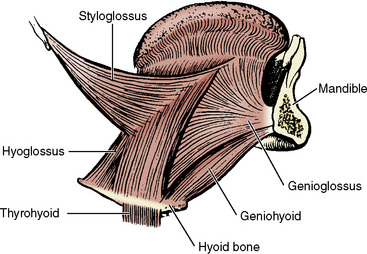
FIGURE 3-15 Lateral view of the musculature of the tongue and its relationship with a mandible and hyoid bone.
(From Kuna ST, Remmers JE: Pathophysiology and mechanisms of sleep apnea. In Fletcher EC, editor: Abnormalities of respiration during sleep, Orlando, FL, 1986, Grune & Stratton.)
A neural balance model of pharyngeal airway maintenance proposed by Remmers et al. (1978) and Brouillette and Thach (1979) and further modified by Isono (2006) is shown in Figure 3-16. In this model, the suction (collapsing) force created in the pharyngeal lumen by the inspiratory pump muscles (primarily the diaphragm) must be well balanced by the activities of pharyngeal airway dilator muscles to maintain upper airway patency. Increased nasal and pharyngeal airway resistance (partial obstruction) exaggerates the suction force. In addition, once pharyngeal closure occurs, the mucosal adhesion force of the collapsed pharyngeal wall becomes an added force acting against the opening of pharyngeal air passages (Reed et al., 1985).
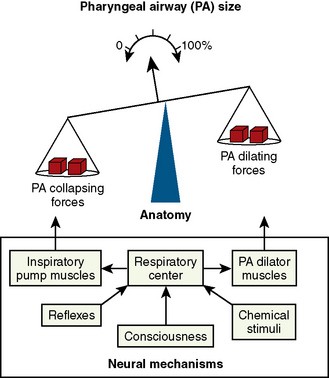
FIGURE 3-16 A neural and anatomic balance model of the pharyngeal airway (PA) maintenance by Remmers et al. (1978) in adults and Brouillette and Thach (1979) in infants illustrating the balance of opposing forces that affect PA size. Airway collapsing forces (suction force created by inspiratory pump muscles) and dilating forces (pharyngeal dilator muscles) are shown on either side of the fulcrum, and neural mechanisms controlling this balance are in the box below the balance.
(Redrawn from Isono S: Developmental changes of pharyngeal airway patency: implications for pediatric anesthesia, Pediatr Anesth 16:109, 2006.)
Several reflex mechanisms are present to maintain the balance between the dilating and collapsing forces in the pharynx. Chemoreceptor stimuli such as hypercapnia and hypoxemia stimulate the airway dilators preferentially over the stimulation of the diaphragm so as to maintain airway patency (Brouillette and Thach, 1980; Onal et al., 1981, 1982). Negative pressure in the nose, pharynx, or larynx activates the pharyngeal dilator muscles and simultaneously decreases the diaphragmatic activity (Fig. 3-17) (Mathew et al., 1982a, 1982b; Hwang et al., 1984; Thach, 1992). Such an airway pressure reflex is especially prominent in infants younger than 1 year of age (Thach et al., 1989). Upper airway mechanoreceptors are located superficially in the airway mucosa and are easily blocked by topical anesthesia (Mathew et al., 1982a, 1982b). Sleep, sedatives, and anesthesia depress upper airway muscles more than they do the diaphragm (Sauerland and Harper, 1976; Ochiai et al., 1989, 1992). The arousal from sleep shifts the balance toward pharyngeal dilation (Thach, 1992).
Laryngeal Airway
The larynx is composed of a group of cartilage, connecting ligaments, and muscles. It maintains the airway, and the glottis functions as a valve to occlude and protect the lower airways from the alimentary tract. It is also an organ for phonation (Proctor, 1977a, 1977b, 1986; Fink and Demarest, 1978). With the exception of the anterior nasal passages, the larynx at the subglottis is the narrowest portion of the entire airway system in all ages (Eckenhoff, 1951). The cricoid cartilage forms a complete ring, protecting the upper airway from compression.
For over half a century, the shape of pediatric larynx was thought to be “funnel shaped,” with the narrowest point at the laryngeal exit (cricoid ring), in contrast to the adult larynx, which is “cylindrical” in shape. This belief was based on the well-referenced classic paper by Eckenhoff in 1951; Eckenhoff quoted the work of Bayeux in 1897, more than half a century before his time, whose description was based on moulages (plaster casting) made from cadaveric larynx from 15 children between the ages of 4 months and 14 years. Indeed, Eckenhoff’s original paper cautioned that “the measurements so derived may not be completely applicable to the living” (Eckenhoff, 1951; Motoyama, 2009). More recently, however, both Litman and colleagues (2003) and Dalal and colleagues (2009) using two entirely different methodologies in living infants and children under general anesthesia, found that the dimensions of the larynx in infants and children are more cylindrical than funnel-shaped, as in adults, and the cylindrical shape does not change significantly with growth. In addition, both Litman and Dalal’s papers confirmed that the cricoid opening is the narrowest point of the larynx; however, in paralyzed children the opening at the vocal cords (lima glottidis) may be narrower than the opening of the cricoid cartilage, but it is expandable beyond the opening of the cricoid ring (Eckenhoff, 1951; Dalal et al., 2009). Perhaps more important clinically, both groups found with statistical significance that the cricoid opening is not circular but mildly elliptic with a smaller transverse diameter. This means that a tight-fitting, uncuffed endotracheal tube or even a “best-fitted” tube in young children with acceptable pressure leak (i.e., 20 cm H2O) would exert more compression, if not ischemia, on the transverse mucosa of the cricoid ring (Motoyama, 2009). This finding provides theoretic evidence and further supports the recent trend of favoring cuffed endotracheal tubes over uncuffed endotracheal tubes in infants and children for their safety.
The glottis widens slightly during tidal inspiration and narrows during expiration, thus increasing laryngeal air flow resistance (Bartlett et al., 1973). Laryngeal resistance is finely regulated in neonates and young infants to dynamically maintain end expiratory lung volume (FRC) well above the small lung volume determined by the opposing elastic recoil forces of the thorax and the lungs, as is discussed in a later part of this chapter (Harding, 1984; England and Stogren, 1986). In infants with IRDS, expiration is often associated with “grunting” caused by narrowing of the glottic aperture. This grunting apparently maintains intrinsic positive end-expiratory pressure (PEEP), also known as PEEPi or autoPEEP, during the expiratory phase and presumably prevent or reduce premature closure of airways and air spaces. In infants with IRDS, when grunting is eliminated by endotracheal intubation, respiratory gas exchange deteriorates rapidly and critically to the point of cardiorespiratory arrest unless continuous positive airway pressure (CPAP) is applied (Gregory et al., 1971).
Airway Protective Reflexes
Upper airway protective mechanisms involve both the pharynx and larynx and include sneezing, swallowing, coughing, and pharyngeal or laryngeal closure. Laryngospasm is a sustained tight closure of the vocal cords caused by the stimulation of the superior laryngeal nerve, a branch of the vagus, and contraction of the adductor muscles that persists beyond the removal of the stimulus. In puppies, it is elicited by repetitive stimulation of the superior laryngeal nerve with typical adductor after-discharge activity. This response is not evoked by the stimulation of the recurrent laryngeal nerve (Suzuki and Sasaki, 1977). Hyperventilation and hypocapnia, as well as light anesthesia, increase the activity of adductor neurons, reduce the mean threshold of the adductor reflex, or increase upper airway resistance (Suzuki and Sasaki, 1977; Nishino et al., 1981). Hyperthermia and decreased lung volume also facilitate laryngospasm produced by stimulation of the superior laryngeal nerve (Sasaki, 1979; Haraguchi et al., 1983). Contrarily, hypoventilation and hypercapnia, positive intrathoracic pressure, and deep anesthesia depress excitatory adductor after-discharge activity and increase the threshold of the reflex that precipitates laryngospasm (Suzuki and Sasaki, 1977; Ikari and Sasaki, 1980; Nishino et al., 1981). Hypoxia below an arterial Po2 of 50 mm Hg also increases the threshold for laryngospasm (Ikari and Sasaki, 1980).
These findings are clinically relevant, suggesting a fail-safe mechanism by which asphyxia (hypoxia and hypercapnia) tends to prevent sustained laryngospasm. In healthy, awake adults, laryngospasm by itself is self-limited and not a threat to life. On the other hand, in the presence of cardiopulmonary compromise, such as may occur during anesthesia (particularly in infants), laryngospasm may indeed become life threatening (Ikari and Sasaki, 1980). Increased depth of anesthesia increases the reflex threshold and diminishes excitatory adductor after discharge in puppies (Suzuki and Sasaki, 1977). This finding is in accord with the clinical experience that laryngospasm occurs most readily under light anesthesia and that it can be broken by deepening anesthesia or awakening the patient. In puppies, positive intrathoracic pressure inhibits the glottic closure reflex and laryngospasm. This supports the clinical observation that during the emergence from anesthesia in infants and young children, maintenance of PEEP and inflation of the lungs at the time of extubation seem to reduce both the incidence and severity of laryngospasm (Motoyama, unpublished observation).
Infants are particularly vulnerable to laryngospasm. Animal studies suggest that during a discrete interval after birth and before complete neurologic maturation, there is a period of transient laryngeal hyperexcitability. This may relate to the transient reduction in central latency and a reduction in central inhibition of the vagal afferent nerve. If these observations in puppies are applicable to human infants, they may explain the susceptibility of infants and young children to laryngospasm and have some causal relation in unexpected infant death such as SIDS (Sasaki, 1979).
Infants, particularly premature neonates, exhibit clinically important airway protective responses to fluid at the entrance to the larynx (Davies et al., 1988; Pickens et al., 1989). This response seems to trigger prolonged apnea in neonates and breath-holding during inhalation induction of anesthesia in children. When a small quantity (less than 1 mL) of warm saline solution is dripped into the nasopharynx in a sleeping infant, it pools in the piriform fossa and then overflows into the interarytenoid space at the entrance to the larynx. This area is densely populated with various nerve endings, including a structure resembling a taste bud. The most common response to fluid accumulation is swallowing. The infant also develops central apnea with either the glottis open or closed; coughing is rare (Pickens et al., 1989). Apneic responses are more prominent with water than with saline solution (Davies et al., 1988).
These findings appear clinically important in pediatric anesthesia. During inhalation induction, pharyngeal reflexes (swallowing) are abolished, whereas laryngeal reflexes remain intact, as Guedel (1937) originally described for ether anesthesia. Secretions would accumulate in the hypopharynx without swallowing and cause breath-holding, resulting from central apnea, a closure of the glottis, or both. Positive pressure ventilation using a mask and bag instead of suctioning the pharynx would push secretions farther down into the larynx, stimulate the superior laryngeal nerve, and trigger real laryngospasm.
Anesthetic Effects on Control of Breathing
Effects of Anesthetic on Upper Airway Receptors
Inhalation induction of anesthesia is often associated with reflex responses such as coughing, breath-holding, and laryngospasm. Volatile anesthetics stimulate upper airway receptors directly and affect ventilation. In dogs spontaneously breathing through tracheostomy under urethane-chloralose anesthesia, an exposure of isolated upper airways to halothane caused depression of respiratory-modulated mechanoreceptors or pressure receptors, whereas irritant receptors and flow (cold) receptors were consistently stimulated in a dose-dependent manner (Nishino et al., 1993). Responses to isoflurane and enflurane were less consistent. Laryngeal respiratory-modulated mechanoreceptors may be a part of a feedback mechanism that maintains the patency of upper airways; the depression of this feedback mechanism may play an important role in the collapse of upper airways during the induction of anesthesia. Furthermore, activation of irritant receptors by halothane and other volatile anesthetics may be responsible for laryngeal reflexes such as coughing, apnea, laryngospasm, and bronchoconstriction seen during inhalation induction of anesthesia (Nishino et al., 1993).
The same group of investigators showed that in young puppies (younger than 2 weeks old), exposure of isolated upper airways to halothane (and to a lesser extent to isoflurane) resulted in a marked depression of ventilation (less than 40% of control) associated with decreases in both tidal volume and respiratory frequency (Sant’Ambrogio et al., 1993). Ventilatory effects caused by the exposure of isolated upper airways to volatile anesthetics were present but only mildly in 4-week-old puppies, whereas adult dogs were not affected. The superior laryngeal nerve section and topical anesthesia of the nasal cavity completely abolished the effects of halothane and isoflurane in the isolated upper airways of puppies (Sant’Ambrogio et al., 1993). Laryngeal receptor output in response to volatile anesthetics was not measured in this study. These findings in puppies appear to be clinically relevant because infants and young children often develop manifestations of upper airway reflexes during inhalation induction.
Effects of Anesthetics on Upper Airway Muscles
The genioglossus, geniohyoid, and other pharyngeal and laryngeal abductor muscles have phasic inspiratory activity synchronous with diaphragmatic contraction, in addition to their tonic activities that maintain upper airway patency in both animals and human neonates (Bartlett et al., 1973; Brouillette and Thach, 1979). The genioglossus and geniohyoid muscles increase the caliber of the pharynx by displacing the hyoid bone and the tongue anteriorly and are the most important muscles for the maintenance of oropharynx patency (Fig. 3-15). They have both phasic inspiratory activity and tonic activity throughout the respiratory cycle in awake humans (Onal et al., 1981). These activities of the genioglossus muscle and presumably other pharyngeal and laryngeal abductor muscles are easily depressed by alcohol ingestion, sleep, and general anesthesia; their depression would result in upper airway obstruction (Remmers et al., 1978; Brouillette and Thach, 1979; Nishino et al., 1984, 1985; Bartlett et al., 1990).
Sensitivity to anesthetics differs among various inspiratory muscles and their neurons. In studies in cats with the use of electromyography, Ochiai et al. (1989) demonstrated that the phasic inspiratory activity of the genioglossus muscle was most sensitive to the depressant effect of halothane at a given concentration, whereas the diaphragm was most resistant; the sensitivity of inspiratory intercostal muscles was intermediate (Fig. 3-18). In addition, phasic genioglossus activity was more readily depressed in kittens than in adult cats. Phasic genioglossus activity was completely abolished with 1.5% halothane or more in all kittens studied, whereas the activity was diminished but present in most adult cats even at 2.5% (Ochiai et al., 1992).
Early depression of the genioglossus muscle and other pharyngeal dilator muscles appears to be responsible for upper airway obstruction in infants and young children, especially during the induction of inhalation anesthesia. Because of the higher sensitivity to anesthetic depression, the upper airway muscles failed to increase the intensity of contraction to keep the pharynx patent while the diaphragm continues to contract vigorously and the negative feedback mechanism to attenuate its contraction may be diminished or lost (Brouillette and Thach, 1979; Ochiai et al., 1989; Isono et al., 2002). Partial upper airway obstruction may occur more often in infants and young children than is clinically apparent during anesthesia by mask without an oral airway. Keidan et al. (2000) found in infants and children breathing spontaneously under halothane anesthesia that the work of breathing (as an index of the degree of upper airway obstruction) significantly increased when breathing by mask without an oral airway than with an oral airway in place, even when partial upper airway obstruction was not clinically apparent. An addition of CPAP (5 to 6 cm H2O) further improved airway patency as evidenced by significant decreases in the work of breathing (Keidan et al., 2000).
Effects of Anesthetic on Neural Control of Breathing
Most general anesthetics, opioids, and sedatives depress ventilation. They variably affect minute ventilation (), its components (Vt, f, Vt/Ti), and respiratory duty cycle (Ti/Ttot). All inhaled anesthetics significantly depress ventilation in a dose-dependent fashion (Fig. 3-9). This subject has been extensively reviewed; information in human infants and children, however, remains limited (Hickey and Severinghaus, 1981; Pavlin and Hornbein, 1986).
Studies in adult human volunteers using the occlusion technique and the timing component analysis have indicated that the reduction in tidal volume with anesthetics results primarily from a reduction in the neural drive of ventilation (Milic-Emili and Grunstein, 1975; Whitelaw et al., 1975; Derenne et al., 1976; Wahba, 1980). Inspiratory time tends to decrease, but the respiratory duty cycle is relatively unaffected. In several studies in children 2 to 5 years of age, breathing was relatively well maintained at a light level of halothane (0.5 minimum alveolar concentration [MAC]) (Murat et al., 1985; Lindahl et al., 1987; Benameur et al., 1993). In deeper, surgical levels of anesthesia (1.0 to 1.5 MAC), breathing was depressed in a dose-dependent manner and hypercapnia resulted. Decreased was associated with reduced Vt and increased respiratory frequency. The neural respiratory drive was depressed as evidenced by reduced Vt/Ti, whereas the duty cycle (Ti/Ttot) either tended to increase without changes in Ti or decreased slightly (Murat et al., 1985; Lindahl et al., 1987; Benameur et al., 1993). In infants younger than 12 months of age, ventilatory depression was more pronounced and the duty cycle did not increase, partly because of high chest-wall compliance and pronounced thoracic deformity (thoracoabdominal asynchrony) compared with older children (Benameur et al., 1993).
When an external load was imposed on the airway system of an awake individual, ventilation was maintained by increased inspiratory effort (Whitelaw et al., 1975). This response was greatly diminished or abolished by the effect of general anesthetics, opioids, and barbiturates (Nunn and Ezi-Ashi, 1966; Isaza et al., 1976; Kryger et al., 1976b; Savoy et al., 1982). In children under light halothane anesthesia (0.5 MAC), an addition of a resistive load initially decreased tidal volume. However, tidal volume returned to baseline within 5 minutes (Lindahl et al., 1987).
Effects of Anesthetic on Chemical Control of Breathing
In the dog, inhaled anesthetics diminish or abolish the ventilatory response to hypoxemia in a dose-dependent manner (Weiskopf et al., 1974; Hirshman et al., 1977). In human adult volunteers, the hypoxic ventilatory response was disproportionately depressed in light halothane anesthesia compared with the response to hypercapnia (Knill and Gelb, 1978). At 1.1 MAC of halothane, the hypoxic ventilatory response was completely abolished, whereas the hypercapnic response was about 40% of control in the awake state. Even at a subanesthetic or trace level (0.05 to 0.1 MAC), halothane, isoflurane, and enflurane attenuated the hypoxic ventilatory response to about 30% of the control group, whereas hypercapnic response was essentially intact (Knill and Gelb, 1978; Knill and Clement, 1984). The site of the anesthetics’ action appears to be at the peripheral (carotid) chemoreceptors, because of the rapid response in humans as well as the direct measurement of neuronal chemoreceptor output in the cat (Davies et al., 1982; Knill and Clement, 1984).
Subsequently, Temp and others (1992, 1994) challenged these findings by demonstrating that 0.1 MAC of isoflurane had no demonstrable ventilatory effect on hypoxia. On the other hand, Dahan and others (1994) confirmed the original findings by Knill and Gelb (1978). The reason for the conflicting results appeared to be related to the contribution of visual and auditory inputs (Robotham, 1994). The study by Temp and others (1994) was conducted while the volunteers were watching television (open-eyed), whereas the volunteers in the study by Dahan and others (1994) were listening to soothing music with their eyes closed (but not asleep).
Pandit (2004) conducted a meta-analysis of 37 studies in 21 publications and analyzed the conflicting response to hypoxia under trace levels of anesthetics. Pandit’s analysis supported the prediction by Robotham (1994) that the study condition has a major impact on the outcome of the study. Bandit concluded that the main factor for the difference in hypoxic response was the anesthetic agent used (p < 0.002). Additional factors included subject stimulation (p < 0.014) and agent-stimulation interaction (p < 0.04), whereas the rate of induction of hypoxia or the level of Pco2 had no effect (Pandit, 2000).
The effect of subanesthetic concentrations of inhaled anesthetics on ventilation in infants and children has not been studied. However, high incidences of postoperative hypoxemia in otherwise healthy infants and children without an apparent hypoxic ventilatory response in the postanesthetic period suggest that the hypoxic ventilatory drive in infants and children may be blunted with the presence of residual, subanesthetic levels of inhaled anesthetics (Motoyama and Glazener, 1986).
Lung volumes
Postnatal Development of the Lungs
In the human fetus, alveolar formation does not begin until about 4 weeks before birth, although development of the airways, including the terminal bronchioles, are completed by 16 weeks’ gestation (Reid, 1967; Langston et al., 1984). The full-term newborn infant has 20 to 50 million terminal airspaces, mostly primitive saccules from which alveoli later develop (Thurlbeck, 1975; Langston et al., 1984). During the early postnatal years, development and growth of the lungs continue at a rapid pace, particularly with respect to the development of new alveoli. By 12 to 18 months of age, the number of alveoli reaches the adult level of 400 million or more; subsequent lung development and growth are associated with increases in alveolar size as well as further structural development (see Development of the Respiratory System) (Dunnill, 1962; Langston et al., 1984).
During the early period of postnatal lung development, the lung volume of infants is disproportionately small in relation to body size. Furthermore, because the infant’s metabolic rate in relation to body weight is nearly twice that of the adult, the ventilatory requirement per unit of lung volume in infants is greatly increased. Infants seem to have far less reserve in lung surface area for gas exchange. Furthermore, general anesthesia markedly reduces the end expiratory lung volume (FRC, or relaxation volume, Vr), especially in young infants, reducing their oxygen reserve severely. Normal values for lung volumes and function in persons of various ages are compiled in Table 3-2.
Total lung capacity (TLC) is the maximum lung volume allowed by the strength of the inspiratory muscles stretching the thorax and lungs. Subdivisions of TLC are shown schematically in Figure 3-19. Residual volume (RV) is the amount of air remaining in the lungs after maximum expiration and is approximately 25% of TLC in healthy children. FRC is determined by the balance between the outward stretch of the thorax and the inward recoil of the lungs and is normally roughly 50% of TLC in the upright posture in healthy children and young adults; it is about 40% when they are in the supine position (Fig. 3-20). The two opposing forces create an average negative average pleural pressure of approximately −5 cm H2O in older children and adults. In the neonate the pleural pressure is only slightly negative or nearly atmospheric.
Functional Residual Capacity and Its Determinants
In infants, outward recoil of the thorax is exceedingly low, and inward recoil of the lungs is only slightly lower than that of adults (Agostoni, 1959; Bryan and Wohl, 1986). Consequently, the FRC (or, more appropriately, Vr) of young infants at static conditions (e.g., apnea, under general anesthesia, or paralysis) decreases to 10% to 15% of TLC, a level incompatible with normal gas exchange because of airway closure, atelectasis, and V/Q imbalance (Fig. 3-21) (Agostoni, 1959). In awake infants and young children, however, FRC is dynamically maintained by a number of mechanisms for preventing the collapse of thelungs, tincluding a sustained inspiratory muscle tension to make the thorax stiffer (Box 3-2) (see Elastic Properties). FRC in young infants is therefore dynamically determined; there is no fixed level of FRC.
Box 3-2 Maintenance of Functional Residual Capacity in Young Infants
All mechanisms of sustaining FRC are lost with anesthesia or muscle relaxant.
In normal children and adolescents, lung volumes are related to body size, especially height. In most instances, the relative size of the lung compartment appears to be approximately constant from school-aged children to young adults (see Table 3-2). A study in anesthetized and paralyzed infants and children indicates that TLC, as measured with a tracer gas washout technique, is relatively small in infants (60 mL/kg) when the lungs are inflated with relatively low inflation pressure (20 to 25 cm H2O; the recruitment of previously collapsed air space with this pressure might not have been complete) (Thorsteinsson et al., 1994). TLC in children older than 1.5 years of age (determined with inflation pressures of 35 to 40 cm H2O) increases with growth until about 5 years of age (body weight, 20 kg), when it reaches that of older children and adolescents (90 mL/kg).
Anesthesia, surgery, abdominal distention, and disease may all alter lung volumes. The patient in the prone or supine position has a smaller FRC than the patient standing or sitting, because the abdominal contents shift. FRC is further decreased under general anesthesia with or without muscle relaxants (Westbrook et al., 1973). (see Effect of General Anesthesia on FRC)
The importance of the air remaining in the lungs at the end of normal expiration is often overlooked. This gas volume (FRC) serves as a buffer to minimize cyclic changes in Pco2 and Po2 of the blood during each breath. In addition, the fact that air normally remains in the lungs throughout the respiratory cycle means that relatively few alveoli collapse. Although alveolar collapse does not occur during normal breathing in healthy, awake infants and children, unusually high pressures are needed to expand the lungs when they are liquid filled at birth, collapsed after open-chest surgery, or during general anesthesia without the maintenance of PEEP, especially in young infants (von Ungern-Sternbery, 2006). Transpulmonary pressure of 30 to 40 cm H2O (and occasionally even more) is needed to reexpand the collapsed lungs. Thereafter, 5 to 7 cm H2O of PEEP appears adequate to prevent airway closure and to maintain FRC.
Mechanics of Breathing
To ventilate the lungs, the respiratory muscles must overcome certain opposing forces within the lungs themselves. These forces have both elastic and resistive properties. Although respiratory mechanics in adults have been studied extensively over the past five decades, most available information on infants and young children has emerged relatively recently (Bryan and Wohl, 1986; ATS/ERS Joint Committee, 1993).
Elastic Properties
Compliance of the Lungs and Thorax
When the lungs are expanded by the contraction of inspiratory muscles or by positive pressure applied to the airways, elastic recoil of the lungs and thoracic structures surrounding the lungs counterreacts to reduce lung volume. This elastic force is fairly constant over the range of normal tidal volumes, but it increases at the extremes of deflation or inflation (Fig. 3-22). The elastic properties of the lungs and respiratory system (lungs and thorax) are measured and expressed as lung compliance (Cl) or respiratory system compliance (Crs) in units of volume change per unit of pressure change. The following equation is derived:
where ΔV is usually the tidal volume and ΔP is the change in transpulmonary pressure (the difference between the airway and pleural pressures [ΔP = Pao− Ppl]) for Cl, and for Crs, ΔP is transrespiratory pressure (the difference between the airway pressure at end-inspiratory occlusion and atmospheric pressure [ΔP = Pao − PB]) necessary to produce the tidal volume. These measurements are made at points of no flow, that is, at the extremes of tidal volume when there is no flow-resistive component (static compliance). Lung compliance may vary with changes in the midposition of tidal ventilation with no inherent alteration in the elastic characteristics of the lungs (Fig. 3-22). The elastic properties of the lungs are described more accurately by measuring pressure-volume relationships over the entire range of TLC.
In normal persons, lung compliance measured during the respiratory cycle (i.e., the dynamic compliance during quiet breathing) is approximately the same as the static compliance. When there is airway obstruction, however, the ventilation of some lung units may be functionally decreased, resulting in decreased dynamic compliance, whereas the static compliance is relatively unaffected. This difference between static and dynamic compliance increases with increasing respiratory frequency (frequency dependence of compliance) and is a sign of airway obstruction (Woolcock et al., 1969).
Quiet, normal expiration occurs passively, resulting from the elastic recoil of the lungs and chest wall and involves little or no additional work. The situation in the infant or in the anesthetized and spontaneously breathing patient may be somewhat different, because expiration may have an active phase (Munson et al., 1966). To consider volume-pressure relationships from another point of view, a normal tidal volume may be obtained using transpulmonary pressures of approximately 4 to 6 cm H2O in persons of all sizes, provided that the lungs are normal, they are normally expanded initially, and the airways are patent. The total transthoracopulmonary pressure needed to ventilate the lungs with positive pressure in a closed chest is, in the adult, approximately twice the required transpulmonary pressure during spontaneous breathing, because the thoracic structures must also be expanded. The chest wall in the newborn is extremely compliant and therefore requires almost no force for expansion (Fig. 3-21). The combined compliance of the chest wall and lungs, or the compliance of the total respiratory system (Crs), is expressed as follows:
where Ers is the elastance of the total respiratory system, El is lung elastance, and Ew is chest wall elastance. Lung compliance in normal humans of different sizes is generally directly proportional to lung size (see Table 3-2). The compliance is expressed per unit of lung volume (e.g., per FRC, vital capacity [VC], or TLC) for comparison (termed specific compliance).
Developmental Changes in the Compliance of the Lungs and Thorax
After the initial period of neonatal adaptation, the compliance of the infant’s lungs is extremely high (elastic recoil is low), probably because of absent or poorly developed elastic fibers (Fig. 3-23) (Fagan, 1976, 1977; Motoyama, 1977). Oddly enough, their functional characteristics resemble those of geriatric, emphysematous lungs with pathologically high compliance caused by the loss of functioning elastic fibers (Fig. 3-24). Thus, at both extremes of human life, the lungs are prone to premature airway closure (Mansell et al., 1972). Elastic recoil pressure of the lungs at 60% TLC increases from about 1 cm H2O in the newborn to 5 cm H2O at 7 years of age and 9 cm H2O at 16 years of age (Fagan, 1976, 1977; Zapletal et al., 1987).
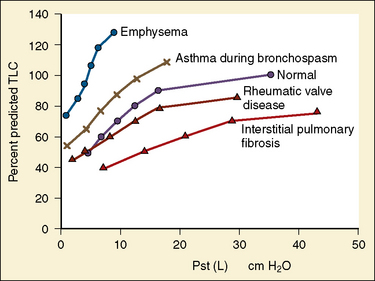
FIGURE 3-24 Static pressure-volume curves (deflation limbs) of the lungs in various conditions as indicated.
(From Bates DV, editor: Respiratory function in disease, ed 3, Philadelphia, 1989, WB Saunders.)
In infants the outward recoil of the chest wall is exceedingly small, because the rib cage is cartilaginous and horizontal, and the respiratory muscles are not well developed, whereas the inward recoil of the lungs is only moderately decreased compared with that in adults (Agostoni, 1959; Gerhardt and Bancalari, 1980). Consequently, the static balance of these opposing forces would decrease FRC to a very low level (Fig. 3-22). Such a reduction in FRC would make parenchymal airways unstable and subject them to collapse. In reality, however, dynamic FRC in spontaneously breathing infants is maintained at around 40% TLC, a value similar to that in adults in the supine position, because of a number of possible mechanisms or their combinations (Bryan and Wohl, 1986).
Maintenance of Functional Residual Capacity in Infants
Infants terminate the expiratory phase of the breathing cycle before lung volume reaches the relaxation volume, or true FRC, determined by the balance of opposing chest wall and lung elastic recoil (Kosch and Stark, 1979). This “premature” cessation of the expiratory phase, which PEEPi with higher FRC, probably results partly from the relatively long time constant of the respiratory system in infants in relation to their high respiratory rate (Olinsky et al., 1974). Additional mechanisms may also help maintain dynamic FRC above the relaxation volume. Glottic closure, or laryngeal braking, during the expiratory phase of the breathing cycle is an important mechanism for the establishment of sufficient air space in the lungs during the early postnatal period (Fisher et al., 1982). Diaphragmatic braking, the diminishing diaphragmatic activity extending to the expiratory phase of breathing, is another important mechanism that extends expiratory time and maintains FRC (Box 3-2).
Among all mechanisms that maintain FRC, tonic contractions of both the diaphragm and the intercostal muscles throughout the respiratory cycle in awake infants appear to be most important. This mechanism effectively stiffens the chest wall and maintains a higher end expiratory lung volume (Muller et al., 1979). Henderson-Smart and Read (1979) have shown a 30% decrease in thoracic gas volume in sleeping infants changing from non-REM to REM sleep. This large reduction in dynamic FRC may result from loss of tonic activity of the respiratory muscles, loss of laryngeal braking, diaphragmatic braking, or all of these factors. All of these important mechanisms for maintaining FRC in infants (and to a lesser extent in older children) are lost with general anesthesia or muscle relaxants, causing marked reductions in FRC, airway closure and atelectasis (Serafini et al., 1999).
When is the FRC no longer determined dynamically but determined by the balance between the recoils of the thorax and the lungs to the opposing direction, as in adults? Colin and others (1989) have shown that, in infants and children during quiet, natural sleep, the transition from dynamically determined to relaxed end expiratory volume or FRC takes place between 6 and 12 months of age. By 1 year of age the breathing pattern is predominantly that of relaxed end-expiratory volume, just as in older children and adults. These findings coincide with the upright posture and development of thoracic tissue and muscle strength in infants.
The breathing pattern of infants younger than 6 months of age is predominantly abdominal (diaphragmatic) and the contribution of the rib cage (external intercostal muscles) to tidal volume is relatively small (20 to 40%), reflecting instability of the thorax or weakness of the intercostal muscles. After 9 months of age, the rib cage component of tidal volume increases to a level (50%) similar to that of older children and adolescents, reflecting the maturation of the thoracic structures (Hershenson et al., 1990). Furthermore, a study by Papastamelos and others (1995) has shown that the stiffening of the chest wall continues throughout infancy and early childhood. By 12 months of age, however, chest wall compliance (which is extremely high in neonates) decreases and nearly equals lung compliance. The chest wall becomes more stable and can resist the inward recoil of the lungs and maintain FRC passively. These relatively recent findings support the notion that the stability of the respiratory system is achieved by 1 year of age.
Effects of General Anesthesia on Functional Residual Capacity
General anesthesia with or without muscle relaxation results in a significant reduction of FRC in adult patients in the supine position soon after the induction of anesthesia, whereas FRC is unchanged during anesthesia in the sitting position (Rehder et al., 1971, 1972b, 1974; Westbrook et al., 1973). A decrease in FRC is associated with reductions in both lung and thoracic compliance, but the mechanism responsible for the reduction in FRC and the sequence of events that changes respiratory mechanics were not understood for many years.
In an excellent study, deTroyer and Bastenier-Geens (1979) showed that when a healthy volunteer was partially paralyzed with pancuronium, the outward recoil of the thorax decreased, whereas lung recoil (compliance) did not. This change altered the balance between the elastic recoil of the lung and thorax in opposite directions, and consequently FRC diminished. The compliance of the lungs decreased shortly thereafter, resulting from the reduced FRC and resultant airway closure. Based on their findings, deTroyer and Bastenier-Geens postulated that, in the awake state, inspiratory muscles have intrinsic tone that maintains the outward recoil and rigidity of the thorax. Anesthesia or paralysis would abolish this muscle tone, reducing thoracic compliance followed by a reduction in FRC, and eventually lung compliance in rapid succession (in a matter of a few minutes).
In healthy young adults, a reduction of FRC during general anesthesia is limited to between 9% and 25% from the awake control levels (Laws, 1968; Rehder et al., 1972; Westbrook et al., 1973; Hewlett et al., 1974; Juno et al., 1978). In older individuals, the average reduction in FRC is more (30%), probably because of lower elastic recoil pressure, increased closing capacity and further airway closure, and eventual atelectasis (Bergman, 1963).
With the more compliant thoraces of infants and young children, general anesthesia and muscle relaxation would be expected to produce more profound reductions in FRC than in adolescents and adults. Henderson-Smart and Read (1979) have shown a 30% reduction in thoracic gas volume (FRC) in infants, changing the sleep pattern from non-REM to REM sleep with increased muscle flaccidity. In children 6 to 18 years of age under general anesthesia and paralysis, Dobbinson and others (1973) found marked reductions in FRC (average reduction, 35%) from their own awake control values, as measured with a helium dilution technique. The average decrease in FRC among those younger than 12 years of age was 46%. Fletcher and others (1990) demonstrated that compliance of the respiratory system (Crs) in infants and children under general anesthesia decreased about 35%, a value comparable with the reduction reported in adults under similar conditions (Westbrook et al., 1973; Rehder and Marsh, 1986). This reduction in Crs occurred both during spontaneous breathing and during manual ventilation with low tidal volume after muscle relaxants were given. When tidal volume was doubled, however, Crs returned to preanesthetic control levels.
These findings are in accord with previous findings in adults and support the notion that anesthesia reduces FRC (deTroyer and Bastenier-Geens, 1979; Hedenstierna and McCarthy, 1980). The finding that a larger tidal volume increases Crs toward control values also indicates that FRC decreases to the lower, flatter portion of the pressure-volume curve, which would lead to airway closure (Fig. 3-22). Motoyama and others (1982a) reported moderate decreases in FRC (−46%) in children as measured with helium dilation and a marked decrease (−71%) in infants under halothane anesthesia and muscle paralysis, approaching the relaxation volume in the newborn infant reported by Agostoni (1959).
Until recently, the possible differential effect on FRC of general anesthesia without muscle paralysis vs. general anesthesia with muscle paralysis was not critically compared. Westbrook et al. (1973) reported a 25% reduction in FRC in healthy young adults in the supine position after anesthesia with intravenous sodium thiopental. They did not find a statistically significant difference in the extent of reductions in FRC with thiopental alone vs. those with an addition of muscle relaxant (d-tubocurarine), although the mean reduction in FRC was somewhat more with the relaxant. A more recent study in anesthetized infants and toddlers clearly demonstrated that addition of muscle paralysis in anesthetized children results in additional marked reductions in FRC (on top of the reduction by the effect of general anesthesia) (von Ungern-Sternberg et al., 2006). Furthermore, the reduction in FRC in infants less than 6 months of age was extreme (FRC, 21.3 to 12.2 mL/kg or −43%) as compared with reductions in toddlers (25.6 to 23.0 mL/kg or −10%) (von Ungern-Sternberg et al., 2006). This marked loss of FRC in infants represents the collapse of their extremely compliant thorax with inspiratory muscle paralysis and massive airway closure that would eventually result in atelectasis, uneven distribution of ventilation, V/Q imbalance, and hypoxemia unless the lungs are reexpanded and supported with PEEP.
Effect of Positive End-Expiratory Pressure Under General Anesthesia
Thorsteinsson and others (1994) reported that the lung volume at FRC (or relaxation volume, Vr) was at a lower, flatter portion of the pressure-volume (P-V) curve in all anesthetized infants and children studied. To restore FRC to the normal or steepest portion of the P-V curve of the respiratory system seen in the awake state (with the highest compliance), a PEEP of 5 to 6 cm H2O had to be added to infants younger than 6 months of age and more than 12 cm H2O in older children (Thorsteinsson et al., 1994). A more recent study in children (aged 2 to 6 years) also showed that PEEP as high as 17 cm H2O is needed to raise the lung volume to the steepest portion (highest compliance) of the P-V curve (Kaditis et al., 2008).
Shortly after the induction of anesthesia and muscle relaxation in adult patients, increased density appearing on computed tomography (CT) scans in the dependent portion of the lung has been described in the literature. This increased density could be reduced or eliminated by adding PEEP (Brismar et al., 1985). Serafini and others (1999) were the first to report evidence of airway closure and atelectasis in young children (aged 1 to 3 years; mean age of 1.8 years) on a CT scan in the dependent portion of the lungs shortly after the inhalation induction of anesthesia and intubation. These patients were given three deep inflations of the lungs (sighs) with 40% oxygen in nitrous oxide and ventilated with 10 mL/kg of tidal volume. Atelectasis-increased density (airway closure or atelectasis) appeared on the CT scan almost immediately when patients were ventilated without PEEP (Fig. 3-25, A). When the patients were ventilated for 5 minutes with an addition of PEEP (5 cm H2O) with the same ventilator settings and end tidal Pco2, the density disappeared from the repeated CT scans in all 10 children studied, indicating the recruitment of atelectic lung segments (Fig. 3-25, B) (Serafini et al., 1999).
Because the stability of the thorax increases during the first year of life, it is likely that the thorax would resist the airway collapse and atelectasis with increasing age (Papastamelos et al., 1995). Motoyama (1996) examined this possibility by measuring respiratory system compliance in infants and young children under 6 years of age who were undergoing halothane-nitrous oxide endotracheal anesthesia. These patients were ventilated either with or without PEEP (6 cm H2O) for 15 minutes preceded by deep sighs. After a period of ventilation with PEEP, respiratory system compliance was consistently higher after PEEP than without PEEP. There were significant age-related differences in the degree of increase in compliance after PEEP (6 cm H2O) vs. no PEEP (Fig. 3-26). The average increase of respiratory system compliance with PEEP was greatest in infants younger than 8 months of age (75% higher with PEEP vs. without PEEP). In contrast, in older infants and toddlers (aged 9 months to 2.5 years), an average increase in compliance with PEEP was 22%; in children (aged 2.5 and 5.5 years), the increase was 9%, the level one would expect in adults. These results reflect greater reductions in FRC (or increases in airway closure and atelectasis) in the younger age groups (Motoyama, 1996).
Persistent airway closure during general anesthesia would result in resorption atelectasis because alveolar gas (mostly oxygen and nitrous oxide) trapped below the occluded airways would be rapidly absorbed. Resultant pulmonary V/Q imbalance and right-to-left shunting of blood in the lung may reduce arterial Po2 in the postanesthetic recovery room. Such an effect would be expected to be more profound in infants. Motoyama and Glazener (1986) studied arterial oxygen saturation (SpO2) with a pulse oximeter in otherwise healthy infants and children before and after general anesthesia for simple, relatively short, surgical procedures (e.g., inguinal hernia repair or myringotomy tube insertions). On arrival at the postanesthetic care unit (PACU), the mean SpO2 was 93% (estimated Pao2, 66 mm Hg), significantly reduced from the preoperative value of 97%. In some children, SpO2 decreased to the low 70s (estimated Pao2 of less than 40 mm Hg). These patients showed no sign of hypoxic ventilatory stimulation with normal cutaneous Pco2 (Motoyama and Glazener, 1986).
A large percentage (20% to 40%) of otherwise healthy infants and children develop oxygen desaturation (SpO2 of less than or equal to 94%) during transport and on arrival at the PACU (Motoyama and Glazener, 1986; Pullertis et al., 1987; Patel et al., 1988). A later study of postoperative hypoxemia involving 1152 patients ranging from infants to adults has demonstrated that hemoglobin desaturation occurs sooner, is more pronounced, and lasts longer in infants than in children and longer in children than in adults (Xue et al., 1996). All children, therefore, should be given oxygen by mask during the transport from the operating room and on arrival at the PACU until they can maintain satisfactory oxygen saturation by pulse oximeter without supplemental oxygen (see Chapter 11, Monitoring).
Closing Volume and Closing Capacity
Whether this closure is anatomic or merely the result of dynamic compression and reduction in flow (see Dynamic Properties) is controversial (Hughes et al., 1970; Hyatt et al., 1973). Because the patency of small airways depends in part on the elastic recoil of the lungs, CC as a percentage of TLC is relatively high in young children and would be even higher, at least theoretically, in infants (Mansell et al., 1972). CC increases with aging as well as with small airway disease, such as chronic bronchitis caused by smoking and emphysema in adults.
Dynamic Properties
Airway Resistance
In tidal breathing, inertance (I) is very small and can be ignored. During normal tidal breathing, approximately 90% of the pressure gradient required is needed to overcome the elastic forces, and the remaining 10% of the pressure is expended to counter the flow resistance (Sly and Hayden, 1998).
Assuming a laminar flow (as seen in small or peripheral airways), it is apparent from this equation (Poiseuille’s law) that the most important factor affecting flow resistance is the change in the radius of the tube (airways), because resistance is inversely proportional to the fourth power of the radius. (When the flow is turbulent, as occurs in large airways, the flow resistance increases approximately with r5.) Therefore, airway resistance in infants with smaller airway diameters is much higher, in absolute terms, than airway resistance in older children and adults. It might also be expected that inflammation or secretions in the airway system would result in exaggerated degrees of obstruction in infants compared with older children and adults (Fig. 3-27). One such example may be the severe and often life-threatening obstruction of upper airways seen only in infants and young children with acute supraglottitis (epiglottitis) and subglottic croup (laryngotracheobronchitis). However, in relation to body size, the caliber of airways in general is wider, and airway resistance is lower in infants and children compared with adults (Motoyama, 1977; Stocks and Godfrey, 1977).
In absolute terms, airway resistance in the newborn is very high (19 to 28 cm H2O/L per second). It decreases to less than 2 cm H2O/L per second in the adolescent and the adult. In relative terms (as expressed per unit of lung volume, usually FRC or specific resistance), specific airway resistance is relatively low, or conductance (Gaw, the reciprocal of resistance; G = 1/R) is very high in the newborn. The specific conductance (sGaw = Gaw/FRC) decreases rapidly during the first year of life, indicating a rapid increase in lung volume (alveolar formation) in relation to airway size (Stocks and Godfrey, 1977, 1978). Between 6 and 18 years of age, Gaw increases linearly with increases in height; However, sGaw stays fairly constant throughout this period at about 0.2 L/sec per cm H2O (Zapletal et al., 1969, 1976, 1987).
Distribution of Airway Resistance
Rohrer’s earlier work (1915) led to the belief that peripheral airways of small caliber were the major contributors to total airway resistance. However, the elegant morphometric studies of Weibel (1963) with airway castings in inflated lungs proved that the total cross-sectional area of each generation of airways increases dramatically toward the periphery, like a cross section of a trumpet, on the cross-sectional area vs. airway-generation plot (Fig. 3-28). Indeed, about two thirds of the total airway resistance exists between the airway opening and the trachea, and most of the remaining resistance is in the large central airways. The airways smaller than a few millimeters in diameter (peripheral airways) contribute only about 10% of total resistance (Macklem and Mead, 1967).
These findings have important clinical implications. If the peripheral airways contribute little to the total airway resistance, disease processes involving small airways—such as emphysema in adults, cystic fibrosis (CF) in children, and bronchopulmonary dysplasia (BPD) in infants—will not be detectable by measurement of the total airway resistance. For instance, complete obstruction of half of the peripheral airways would increase the total airway resistance by only 5% to 6 %, an increase within the usual variation in measurements. For this reason, the peripheral airways used to be called the “quiet zone” of the lung (Mead, 1970). Apparently, the measurement of total airway resistance is not a sensitive clinical test for detecting small airway obstruction.
The airway system extends from the airway opening at the nares or the mouth to the alveolar duct at the periphery of the lung. Functionally, the airway system can be subdivided into the upper (extrathoracic) and lower (intrathoracic) airways. The upper airway begin at the airway opening (the mouth or the nose—normally the mouth in quiet breathing) and include the nasal or oral cavity, the pharynx, the larynx, and the upper most segment of the trachea before it enters into the thorax. The lower airway begins with the thoracic inlet of the trachea, the trachea, bronchi, bronchioli, and alveolar ducts entering the alveoli (Box 3-3).
Upper Airway Resistance
During quiet breathing, air-flow resistance through the nasal passages accounts for approximately 65% of total airway resistance in adults (Ferris et al., 1964). This is more than twice the resistance during mouth breathing. For air warming, humidification, and particle filtration, it is important that one preferentially or instinctively breathe through the nose despite its higher resistance (Proctor, 1977a, 1977b). Stocks and Godfrey (1978) found nasal resistance comprised approximately 49% of the total airway resistance in European infants, whereas it was significantly less in infants of African origin (31%). Overall, upper airway resistance is approximately two thirds of the total airway resistance.
Except when crying, newborn infants are obligatory nose breathers. The cephalad position of the epiglottis and close approximation of the soft palate to the tongue and epiglottis in neonates may be a reason why mouth breathing is more difficult than nose breathing (Moss, 1965; Sasaki et al., 1977a). When the nasal airway is occluded, some infants, especially during REM sleep, do not respond sufficiently to initiate adequate mouth breathing and obstructive apnea ensues. In infants, the insertion of a nasogastric tube significantly increases total resistance by as much as 50% and may compromise breathing (Stocks, 1980).
Lower Airway Resistance
Between the trachea and the alveolar duct are an average of 23 (mean of 17 to 27) airway generations or branchings (Fig. 3-28) (Weibel, 1963). As gas molecules move from the trachea toward the terminal airways during inspiration, the radius of the successive generations of airways becomes smaller and the flow resistance is expected to increase. In reality, however, the total cross-sectional area of the airway segments increases dramatically toward the periphery, although the diameter of successive single airways decreases. This is because the number of airways increases markedly and, consequently, the flow resistance of airways decreases toward the periphery (Weibel, 1963) (Fig. 3-28). Using a retrograde catheter technique, Macklem and Mead (1967) demonstrated that the peripheral airways, less than about 1 mm in diameter (around 14th generation), contribute less than 10% of the lower airway resistance (or 3% of the total airway resistance).
Tissue Viscoelastic Resistance
It has been assumed that airway (frictional) resistance represents the majority of total respiratory system resistance during breathing, and the pressure needed to overcome tissue viscous resistance during inspiration was estimated to be about 35% in adults and 28% in children (Bryan and Wohl, 1986). However, studies since the 1980s in both anesthetized animals and humans on mechanical ventilation have indicated that viscoelastic resistance, or the energy required to counter the hysteresis or viscoelasticity of the lungs and thoracic tissues, contributes a significantly greater proportion of the total resistance than previously assumed (Milic-Emili et al., 1990). Furthermore, both airway resistance (Raw) and viscoelastic resistance (Rvis or ΔR) have been found to be flow and volume dependent (i.e., both Raw and Rvis change with volume and/or flow changes) and to the opposite directions. Airway resistance (Raw) increases with increasing flow as a result of higher turbulence, whereas Raw decreases with increasing lung volume, because airway caliber also increases with volume. The traditional view had been that the total resistance followed the same direction of flow resistance because it was thought to be the majority of total respiratory system resistance. Paradoxically, Rvis decreases with increasing flow, when the volume is kept constant, and when the flow rate is kept constant Rvis increases with increasing lung volume (Fig. 3-29) (D’Angelo et al., 1989). Furthermore, the direction of changes in total resistance followed that of Rvis rather than that of Raw. Studies in children who have been anesthetized and ventilated have shown a similar flow and volume dependence of RV exist in adults; that is, opposite of the direction of changes in Raw, although the total resistance did not necessarily follow the changes in Rvis that occurred in adults (Fig. 3-30) (Kaditis et al., 2008).
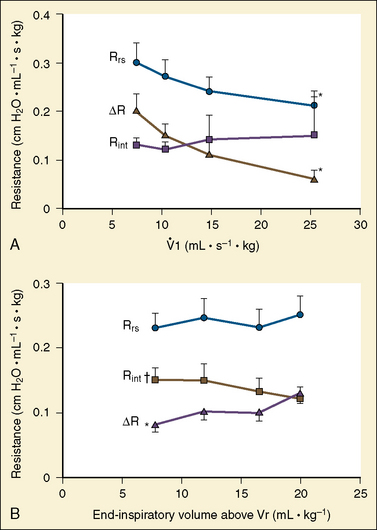
FIGURE 3-30 Flow and volume dependence of respiratory system resistance (Rrs) and its subdivisions, flow-resistive component (Rint or Raw) and viscoelastic component (ΔR or Rvis) (Rrs = Rint + ΔR), in eight healthy children aged 2.3 to 6.5 years under general endotracheal anesthesia. A, Average relationship of Rrs, Rint, and ΔR with increasing inspiratory flow on X-axis at a constant end-inspiratory volume (Vt, 12 mL/kg). ΔR and Rrs decreased significantly with increasing flow as in adults (see Fig. 3-29). B, Average relationship of Rrs, Rint, and ΔR with increasing volume on x-axis while flow was kept constant (15 mL/sec per kg) in the same subjects as in A. Rint decreases with increasing volume as expected, whereas ΔR increased with increasing volume. Unlike in adults, there was no volume dependence of Rrs; Bars, 1 SEM; V.1 inspiratory flow; Vr, relaxation volume or end expiratory volume.
(From Kaditis AG et al.: Effect of lung expansion and PEEP on respiratory mechanics in anesthetized children, Pediatr Anesth 106:775, 2008.)
This evidence and new understanding on the behavior of viscoelastic resistance have important clinical implications. Traditionally, the patient with airway obstruction has been treated with large tidal volumes and a slow respiratory rate to allow complete exhalation to avoid intrinsic PEEPi and air trapping. With the new understanding, it makes more sense to have patients breathe with a smaller tidal volume and higher respiratory rate in order to minimize total respiratory system resistance and decrease work of breathing (Kaditis et al., 1999b, 2008).
Time Constant of the Respiratory System
Under these conditions, the volume-time profile can be represented by an exponential decay and at 1 time constant (1τ), tidal volume is reduced by 63%. It requires 3 × τ to nearly complete exhalation to FRC. In healthy children and adults, τ is 0.4 to 0.5 seconds; it is slightly shorter in neonates (0.2 to 0.3 seconds) (Bryan and Wohl, 1986). In patients with obstructive lung disease, such as bronchial asthma, τ is increased because of an increase in airway resistance; it is also increased markedly in patients breathing through an endotracheal tube under general anesthesia.
The Concept of Flow Limitation and Maximum Expiratory Flow-Volume Curves
During quiet breathing, pleural pressure remains subatmospheric, whereas during forced expiration, pleural pressure increases considerably above atmospheric pressure and in turn increases alveolar pressure. The resultant pressure gradient between the alveoli and the airway opening (atmospheric) produces the expiratory flow. In the periphery of the lungs this pressure within the airways is even higher than the increased pleural pressure by effort because of the additional elastic recoil pressure of the lung. In comparison, in major intrathoracic airways the pressure within the lumen is near atmospheric and lower than the surrounding pleural pressure. At some point along the airways the pressure within the airway lumen should equal the pleural pressure surrounding the airway (equal pressure point [EPP]) (Mead et al., 1967). During forced expiration, the airway between EPP and the trachea is dynamically compressed, and the flow rates consequently become independent of effort (i.e., additional expiratory effort or pressure does not increase flow) (Fig. 3-31). Under these circumstances (dynamic flow limitation), the maximum expiratory flow rate () is determined by the flow resistance of the upstream segment (Rus) between the alveoli and the EPP and the elastic recoil pressure of the lung (Pstl), as follows (Mead et al., 1967):
According to the wave-speed theory of expiratory flow limitation, compliance or collapsibility of lower airways around the EPP (choke point) is an additional determinant of MEF rate (Dawson and Elliott, 1977; Hyatt, 1986).
The maximum expiratory flow volume (MEFV) curve obtained during forced expiration from TLC to residual volume relates instantaneous MEFs to corresponding lung volumes (Fig. 3-32). Clinically, the measurement of MEF rate is an extremely sensitive test for the detection of obstruction of the lower airways toward the periphery (quiet zone) of the lungs because it eliminates the component of upper airway resistance between the mouth and EPP and is independent of the degree of effort or cooperation by the patient (Zapletal et al., 1971) (see Measurements of Pulmonary Function).
Distribution of Flow Resistance
On the basis of physiologic measurements in lungs obtained at autopsy, Hogg and others (1970) reported that airway conductance of the peripheral airways in children younger than 6 years of age was disproportionately low (i.e., resistance was high). They postulated that the diameter of small airways of the same generation was disproportionately smaller in infants than in older children and adults. Although this theory is consistent with the high incidence of severe lower airway disease in infants, it conflicts with later physiologic data obtained from healthy infants. Studies of MEFV curves in anesthetized infants and children, and more recently in sedated infants, showed that at low lung volumes, the MEF normalizes for lung volume and that the conductance of the upstream segment is disproportionately high in infants and decreases with age, indicating that lower airway resistance toward the periphery of the lung parenchyma is relatively lower, rather than higher, in the early postnatal years (Fig. 3-33) (Motoyama, 1977; Lambert et al., 2004).
Ventilation
Ventilation involves the movement of air in and out of the lungs. The diaphragm is the most important muscle for normal inspiration, although the intercostal and accessory respiratory muscles aid in a maximal inspiratory effort. Quiet expiration results from the elastic recoil of the lungs and chest wall and the relaxation of the diaphragm. The expiration of a newborn, even when resting or asleep, appears active rather than passive, as it appears in the older child and adult. A similar active expiration has been observed in anesthetized patients, but the mechanism is unknown (Freund et al., 1964). Forced expiration is accomplished with the aid of the spinal flexors, the intercostal muscles, and especially the abdominal muscles.
The frequency (f) of quiet breathing decreases with increasing age. The exact basis for this change is unknown but may be related to the work of breathing. Humans seem to adjust their respiratory rate and tidal volume so that ventilatory needs are accomplished with a minimum of work (McIlroy et al., 1954). The relatively high rate in newborns (average, 34 breaths/min) as compared with adults (10 to 12 breaths/min) is consistent with this minimum work concept (Fig. 3-34) (Cook et al., 1957). Mead (1960), however, has presented data indicating that in the normal resting state, respiration is adjusted to require a minimum average force of the respiratory muscles. Mead postulated that the principal site of the sensory end of the control mechanism is in the lungs. In certain situations, the minimum work of breathing and minimum average force required would occur at the same frequency of respiration, but this would not invariably be true.
Dead Space and Alveolar Ventilation
In a healthy person, dead space can be estimated as 1 mL/pound of body weight (Radford et al., 1954). In children and young adults, a more exact estimate may be obtained from the relation of dead space to body height (Hart et al., 1963).
The dead space to tidal volume (Vd/Vt) ratio in normal lungs is approximately constant (0.3) from infancy to adulthood (Table 3-2). An absolute increase in dead space, however, whether caused by respiratory abnormalities or external apparatus, is much more critical to the infant than to the adult because of the infant’s small tidal volume and the relatively larger volume of dead space added.
Alveolar ventilation is considerably higher per unit of lung volume in the healthy infant than in the adult. This is expected because the oxygen consumption is also higher per unit of lung volume or body weight in the infant (Cook et al., 1955).
Distribution of Ventilation
The distribution of ventilation is affected by a number of factors. At end expiration with the mouth open and the larynx relaxed, alveolar pressure is zero, or atmospheric. The interpleural pressure is negative, and there is a vertical pressure gradient. The pressure surrounding the apex of the lung is more negative than that at the base. Accordingly, the transmural or distending pressure at the apex is greater and the regional FRC is larger than that at the base (Fig. 3-35, A). At the end of tidal inspiration, a greater proportion of the inspired air is distributed to the base because the regional FRC is at the steepest portion of the pressure-volume curve at the base. In a lateral decubitus position, the lower part of the lung receives a larger tidal volume than the upper part (Kaneko et al., 1966). In adults with unilateral lung disease, pulmonary gas exchange can be improved by positioning with the healthy lung down, or dependent (Remolina et al., 1981).
In infants with unilateral lung disease, however, the opposite seems to be the case. In the lateral decubitus position, oxygenation improves when the healthy lung is uppermost (Heaf et al., 1983; Davies et al., 1985). Furthermore, Heaf and others (1983) have shown by means of a krypton-81m ventilation scan that in infants and children up to 27 months of age, with or without radiologic evidence of lung disease, ventilation is preferentially increased in the uppermost part of the lung and diminished in the dependent lung (Fig. 3-36). This paradoxical distribution of ventilation in young children may be explained by premature airway closure (Davies et al., 1985). Because the infant’s chest wall is extremely compliant, the pleural pressure is near atmospheric. The condition resembles that of adults breathing at extremely low lung volumes (or near RV) (Fig. 3-35, B). Under these circumstances, airway closure occurs and, in the lateral decubitus position, ventilation preferentially shifts to the uppermost part of the lung (Milic-Emili et al., 1966). In paralyzed, mechanically ventilated adults, tidal ventilation is preferentially shifted to the uppermost part of the lung, presumably by a similar mechanism (i.e., reduction of FRC and airway closure) (Rehder et al., 1972).
Clinical Implications
The inspiratory-to-expiratory (I/E) ratio is set to 1:2, a duty cycle (Ti/Ttot) of 0.33. Respiratory frequency should be 10 to 14 breaths/min in adolescents, 14 to 20 breaths/min in children, and 20 to 30 breaths/min in infants. Once the mechanical ventilation is established, it can be decreased and refined with the aid of capnographic monitoring. In patients with obstructive lung disease who have a prolonged respiratory system time constant, expiratory time is increased to allow sufficient time for passive lung deflation. Passive expiration is an exponential function and takes three times the time constant to return to FRC (Lamb, 2000). The addition of a low level of PEEP (5 to 7 cm H2O) restores the volume (FRC) lost from the relaxation of inspiratory muscles and helps prevent airway closure.
Gas diffusion
The ultimate purpose of pulmonary ventilation is to allow the diffusion of oxygen through the alveolar epithelial lining, basement membrane, and capillary endothelial wall into the plasma and red cells and diffusion of CO2 in the opposite direction. The distance for gases to diffuse between the alveolar space and the capillary lumen is extremely small, about 0.3 mcm in humans (Weibel, 1973). Because these processes apparently follow the physical laws of diffusion, without any active participation by the lung tissue, pressure gradients must exist or gas exchange will not occur. On the other hand, if the gradient is increased by changes in gas tension either within the alveoli or in the blood, gas exchange is more rapid. Furthermore, because the blood Po2 affects the blood Pco2, changes in one moiety alter diffusion of the other. CO2 diffuses approximately 20 times faster than oxygen in a gas-liquid environment. Therefore impairment of CO2 diffusion does not become apparent in clinical situations until extremely severe disease is present.
The diffusing capacity of the lungs may be measured with a foreign gas, carbon monoxide (CO), used in small concentrations (less than or equal to 0.3%), or with various concentrations of inspired oxygen (Forster, 1957). The subjects of diffusion and diffusing capacity have been reviewed.
The diffusing capacity of carbon monoxide (Dlco) can be measured with a single breath technique by adding an inert gas to the inhaled gas mixture with a single alveolar gas sample (Ogilve et al., 1957). The Dlco test is not exactly a measure of diffusing capacity, because diffusing implies that the uptake of CO is attributable to diffusion alone and capacity implies it is a maximal limit (Crapo et al., 2001). Indeed, the term transfer factor (Tlco) has become a standard term in most countries outside of North America (Forster, 1983; Cotes et al., 1993). The role of Dlco measurement in lung function testing is to provide information on the transport of gas from alveolar air to hemoglobin in pulmonary capillaries. More specifically, Dlco measures the uptake of CO from the lungs per minute per unit of CO driving pressure, as follows:
Although the diffusion of gases within the lungs is necessary for survival, comparatively few conditions occurring in children affect diffusion per se. Diffusing capacity is decreased in the “alveolar capillary block syndrome” (Bates, 1962). This decrease was considered to result primarily from increased thickness of the alveolocapillary membranes; but it is now believed that uneven distribution of ventilation with a resulting V/Q imbalance is the more important cause of arterial oxygen desaturation (Finley et al., 1962). Diffusing capacity changes with hemoglobin concentrations; it increases as hemoglobin concentration increases. A correction factor has to be used according to the recommendation of American Thoracic Society guidelines (1995). Anemia, on the other hand, is associated with a decrease in diffusing capacity. This is partially explained by the decreased ability of blood to carry the inspired gases. Patients with congenital heart disease and left-to-right shunts often have an increased Dlco caused by increased blood volume and flow in the lungs (Bucci and Cook, 1961). Conversely, diffusing capacity may be reduced when the pulmonary blood flow is markedly decreased, as in pulmonic stenosis.
Pulmonary circulation
Perinatal and Postnatal Adaptation
In prenatal life, pulmonary vascular resistance is high and most of the right ventricular output runs parallel to the left ventricular output, bypassing the lungs and flowing into the descending aorta through the ductus arteriosus. With the onset of ventilation at birth, the pulmonary vascular resistance suddenly decreases and blood flow through the lungs increases, enabling the organism to exchange oxygen and CO2 and sustain independent existence. The principal factors that control this vital adjustment in vascular resistance are chemical changes (i.e., changes in Po2 and Pco2 or pH) in the environment of the pulmonary vessels (Cook et al., 1963). An increase in Po2 also produces constriction and subsequent closure of the ductus arteriosus. The pulmonary arterial pressure, which is slightly higher than the pressure in the ascending aorta in the fetus, suddenly decreases at birth and then continues to decrease, with a gradual decline in pulmonary vascular smooth muscle mass approaching the adult level within the first year of life (Assali and Morris, 1964; Rudolph, 1970). If the lungs do not expand adequately (as in IRDS of the neonate) and Po2 remains low, the pulmonary vascular resistance and pressure may remain high, and there may be prolonged patency of the ductus arteriosus and persistent right-to-left shunting of blood (Strang and MacLeish, 1961) (see Chapter 4, Cardiovascular Physiology).
Both hypoxemia and hypercapnia constrict the pulmonary vascular bed and increase resistance to flow. Chronic hypoxemia is associated with a pulmonary hypertension that returns to or toward normal when the hypoxemia is corrected (Goldring et al., 1964). Pulmonary hypertension that persists for months or years results in right-sided heart failure (cor pulmonale), which then further complicates the existing pulmonary insufficiency.
Nitric Oxide and Postnatal Adaptation
The vascular endothelial cells release various vasoactive factors that affect vascular tone. Nitric oxide (NO) is a unique endogenous regulatory molecule involved in a wide variety of biological activities, including systemic and pulmonary vasodilation, neurotransmission, and immunomodulation (Welch and Loscalzo, 1994). Under physiologic conditions, NO is produced from the amino acid L-arginine catalyzed by constitutive NO synthase (cNOS) with a number of cofactors (nicotinamide adenine dinucleotide phosphate [NADPH], flavoproteins, tetrahydrobiopterin, reduced glutathione, and heme complex) and with the presence of ionized calcium and calmodulin. NO in the vascular endothelial cells diffuses into the adjacent vascular smooth muscle cells, stimulates guanylate cyclase activity, and increases cyclic guanylate monophosphate (cGMP), resulting in controlled smooth muscle relaxation and vasodilation (Furchgott and Vanhoutte, 1989; Moncada et al., 1989). In normal lungs, basal release of endothelium-derived NO contributes to the maintenance of low pulmonary vascular resistance (Celemajer et al., 1994; Stamler et al., 1994).
Certain cytokines and bacterial endotoxins induce a NO synthase isoform (inducible NOS [iNOS]) in macrophages, neutrophils, vascular and airway smooth muscles, and other cell types that normally do not produce cNOS. A massive release of NO by iNOS via activated macrophages and other cell types appears to be the primary cause of profound vasodilation and systemic hypotension in septic shock (Cohen, 1995).
Distribution of Pulmonary Perfusion
As with regional ventilation, gravity results in a nonuniform distribution of pulmonary blood flow in normal lungs. West (1965) divided the characteristics of upright lung perfusion into three zones, which were later modified to four zones, of flow distribution (Fig. 3-37) (Hughes et al., 1968). Perfusion of lung tissue depends on the interrelation among three pressures: alveolar pressure (Pa), pulmonary arterial pressure (Pa), and pulmonary venous pressure (Pv). Because pulmonary circulation normally is a low-pressure circuit, the pulmonary perfusion pressure varies from the top to the bottom of the lung, barely overcoming the hydrostatic pressure to reach the apex of the tall upright adult lung. Both pulmonary perfusion pressure and flow are relatively increased at the lung base (West, 1994).
In zone IV, blood flow is progressively decreased toward the base of the lung, presumably because of increased interstitial pressure surrounding the extraalveolar vessels. This zone increases in size with reduction in the lung volume toward RV (Hughes et al., 1968; West, 1994).
Ventilation/Perfusion Relationships
To achieve normal gas exchange in the lung the regional distribution of ventilation and pulmonary perfusion must be balanced. Without this balance, pulmonary gas exchange is impaired, even when the overall levels of ventilation and perfusion are adequate. The normal value for the ventilation/perfusion (, or simply V/Q) ratio is about 0.8. Studies with radioactive gases have shown that the elastic and resistive properties of various parts of the lung, as well as the pulmonary blood flow, are influenced by gravity. Both components of the V/Q ratio are affected by changes in a patient’s position (West, 1965).
When the patient is in the upright position, blood flow and ventilation are both less in the apex than in the base of the lungs. Because the difference in blood flow between the apex and the base is relatively greater than that in ventilation, the V/Q ratio increases from the bottom to the top of the lungs, as shown in Figures 3-38 and 3-39. The apical regions (high V/Q) have higher alveolar Po2 and lower Pco2 and partial pressure of nitrogen (Pn2), whereas the basal areas (low V/Q) have lower Po2 and higher Pco2 and Pn2. Gravity has a greater effect on the V/Q ratio in hypotensive and hypovolemic patients and may be exaggerated with positive-pressure ventilation. In the supine position, similar differences exist between the anterior and posterior parts of the lung, but they are smaller. During exercise, pulmonary arterial pressure and blood flow, as well as ventilation, are increased and more evenly distributed. In infants and children the distribution of pulmonary blood flow is more uniform than in adults because the pulmonary arterial pressure is relatively high, and the gravity effect in the lungs is less.
The lungs appear to have an intrinsic regulatory mechanism that, to a limited extent, preserves a normal V/Q ratio. In areas with a high V/Q ratio, a low Pco2 tends to constrict airways and dilate pulmonary vessels, and the opposite occurs in areas with a low V/Q ratio. In the latter case, in addition to the effect of Pco2, hypoxic pulmonary vasoconstriction (HPV) decreases regional blood flow and helps to increase V/Q ratios toward normal. The administration of drugs such as isoproterenol, nitroglycerin, theophylline, and sodium nitroprusside diminishes or abolishes HPV and increases intrapulmonary shunting (Goldzimer et al., 1974; Colley et al., 1979; Hill et al., 1979; Benumof, 1994). All inhaled anesthetics depress HPV in vitro, contributing to an increase in venous admixture during general anesthesia (Sykes et al., 1972; Bjertnaes, 1978). The effect of inhaled anesthetics on HPV, however, has not been conclusive in vivo (Marshall and Marshall, 1980, 1985; Pavlin and Su, 1994).
Wagner and others (1974) have developed a quantitative method of studying the continuous spectrum of V/Q mismatch. The technique is based on the pattern of elimination of multiple inert gases infused intravenously. At steady state after intravenous infusion of test gases dissolved in saline solution, arterial, mixed-venous, and expired gas samples are obtained, and minute ventilation and cardiac output are measured. The ratio of arterial to mixed venous concentration (retention) and the ratio of expired to mixed venous concentration (excretion) are computed for each gas, and retention-solubility and excretion-solubility curves are drawn by the computer. The ratio of the two curves represents the distribution of perfusion and ventilation on the spectrum of V/Q ratios (Fig. 3-40) (West, 1974, 1994; Benumof, 1994).
Low Ventilation/Perfusion Ratio and Lung Collapse While Breathing Oxygen
In a lung unit with a low regional V/Q ratio while breathing oxygen, collapse of the lung unit occurs, leading to atelectasis. As alveolar ventilation to the lung unit () decreases, regional expiratory volume (Ve) decreases progressively in comparison with regional inspiratory volume (Vi) as it approaches the amount of oxygen taken up by regional pulmonary blood flow. A point is reached at which the expired alveolar volume falls to zero (West, 1974). This situation occurs at the “critical” inspired V/Q. With inspired ratios less than the critical V/Q value, the lung unit becomes unstable; oxygen may enter rather than leave the lung unit during the expiratory phase or the unit may gradually collapse (Figure 3-41) (West, 1975). Figure 3-42 shows the calculated relationship between the critical inspired V/Q and the concentration of inspired oxygen (assuming mixed venous Po2 of 40 mm Hg and Pco2 of 45 mm Hg and no nitrogen exchange occurring across the whole lung). From Figure 3-42, it can be seen that lung units with V/Q of less than 0.01 become vulnerable when Fio2 is increased above 0.5, whereas lung units with inspiratory
of 0.1 are not at risk even with 100% oxygen (West, 1975). Although a V/Q of less than 0.1 is uncommon in normal awake children, lung units with a V/Q of less than 0.1 may occur in the diseased lung as well as in the normal lung under general anesthesia.