CHAPTER 16 Regional Anesthesia
Regional anesthesia in infants and children has witnessed an increasing popularity over the years that has taken it from a practice of spinal and caudal procedures, to peripheral and truncal nerve blocks taking advantage of sonographic technology (Rochette et al., 2007). The advances that increased its popularity have presumably also improved the safety of blocks in children, which will ultimately encourage further use of this important practice in intraoperative and postoperative pain management. Despite the advances, it is still important to understand the basics of the practice: the pharmacokinetics and toxicities of local anesthetics and their additives, the anatomy of children at different ages, and the indications and complications of specific regional blocks.
Local anesthesia in children
Amide anesthetics are primarily protein bound in the plasma. Bupivacaine, ropivacaine, and levobupivacaine are more than 90% bound to two plasma proteins: α1-acid glycoprotein (high affinity for local anesthetics) and albumin (high volume and relatively low affinity for local anesthetics). It is the free or unbound fraction of the local anesthetic that is physiologically active and is responsible for its effect on the cardiovascular system and the central nervous system (CNS). Infants less than 6 months of age have decreased levels of plasma proteins, resulting in a larger free fraction of local anesthetic, which consequently places this age group at a greater risk for toxicity from these agents (Lerman et al., 1989; Berde, 1992). As an infant matures, the level of plasma proteins increases, and the plasma free-fraction of the drug decreases. Adult levels of protein binding are reached near 1 year of age (Fig. 16-1). Of interest is that α1-acid glycoprotein levels increase in response to surgical stress, and the increased α1-acid glycoprotein ultimately decreases the free fraction of local anesthetic agent. This phenomenon occurs even when total plasma concentration appears to be near toxic levels (Tucker, 1994, 1996; Booker et al., 1996). The liver’s cytochrome P450 system is responsible for the metabolism of amide local anesthetics. These enzymes reach adult activity by the first year of life. The immaturity of liver enzymes in neonates and infants contributes to the decreased clearance of amide local anesthetics seen in this time period.
Ester anesthetics such as chloroprocaine and tetracaine depend on plasma esterases for their elimination. The decreased levels of plasma proteins in neonates and infants are reflected in decreased levels of plasma esterases (Zsigmond and Downs, 1971). However, this has not been shown to be of clinical significance, and tetracaine is commonly used for spinal anesthetics in premature infants for inguinal hernia repairs. Chloroprocaine, although not a commonly used pediatric local anesthetic, has been used for caudal anesthesia. It is thought to afford a greater level of safety than amide anesthetics because of its rapid metabolism (Henderson et al., 1993; Tobias et al., 1996).
Safety Issues
Local Anesthetic Toxicity
Children may be at increased risk for toxicity of local anesthetics because of their relatively increased cardiac output and increased systemic uptake of the agent. This increased systemic uptake may result either in direct CNS toxicity by increasing the amount of local anesthetic available to cross the blood-brain barrier, or in direct cardiac toxicity. Lidocaine at plasma levels of 2 to 4 mcg/mL acts as an anticonvulsant, but at 10 mcg/mL it produces convulsions (Dalens, 1995). Neonates, for example, will manifest symptoms of neurotoxicity such as depressed Apgar scores from lidocaine at umbilical venous blood concentrations of 2.5 mcg/mL, significantly lower than the 5 mcg/mL that is associated with neurotoxicity in adults (Foldes et al., 1960; Shnider and Way, 1968; Ralston and Shnider, 1978; Tucker, 1986).
In unmedicated patients, initial symptoms of neurotoxicity include headache, somnolence, vertigo, and perioral or lingual paresthesia. These symptoms and any objective signs of neurotoxicity such as tremors, twitching, shivering, or even convulsions may not be detected in infants and children under general anesthesia. Diagnosis of local anesthetic toxicity in the child under general anesthesia can be made with indirect signs such as muscular rigidity, hypoxemia without other causes, unexplained tachycardia, dysrhythmias, or cardiovascular collapse. General anesthetics are protective from the CNS effects, but general anesthetics are not protective against cardiac toxicity and may even further contribute to the toxicity (Badgwell et al., 1990). In fact, one study found that in anesthetized ewes, blood concentrations of local anesthetics were doubled because of decreased whole-body distribution and clearance (Copeland et al., 2008a). Net uptake of the local anesthetics in both the heart and brain were greater, with slower efflux, under general anesthesia. Although general anesthesia contributed to local anesthetic–induced cardiovascular depression and changed the overall pharmacokinetics, cardiovascular fatalities occurred only in conscious ewes—there were no fatalities in anesthetized ewes that received intravenous local anesthetics (Copeland et al., 2008b).
The original model that existed was that cardiac toxicity occurs because the local anesthetic prevents the fast inward sodium channels in the myocardium from opening. However, the action leading to toxicity is not at only one specific site, and there is evidence that potassium channels are involved with neuronal excitability and nerve conduction (Kindler and Yost, 2005). Manifestations of toxicity from bupivacaine consist of dysrhythmias with evidence of a high degree of conduction block, widening of the QRS, torsades de pointes, ventricular tachycardia related to reentry phenomena, and major cardiovascular collapse with decreased myocardial contractility (deLaCoussaye et al., 1992). Bupivacaine may produce cardiac and CNS toxicity at serum concentrations of 2 mcg/mL (Tucker, 1986; Dalens and Mazoit, 1998). Although 4 mcg/mL is considered the toxic threshold for bupivacaine in adults, the true toxic concentration of unbound bupivacaine is unknown in humans, particularly children (Knudsen et al., 1997; Luz et al., 1998; Meunier et al., 2001).
Bupivacaine
Bupivacaine is a racemic mixture of equimolar amounts of the enantiomers R(+) bupivacaine and S(−) bupivacaine. For many years, racemic bupivacaine was the only amide local anesthetic with long duration and therefore the most commonly used amide local anesthetic in children. Pharmacokinetic studies of a single dose of racemic bupivacaine (2.5 mg/kg) injected in the caudal space have demonstrated differences between infants and children (Ecoffey et al., 1985; Desparmet et al., 1987; Mazoit et al., 1988). Infants have a greater volume of distribution (3.9 L/kg versus 2.7 L/kg), an increased elimination half-life (7.7 versus 4.6 hours), and decreased clearance (7.1 versus 10.0 mL/kg per minute) compared with older children (Table 16-1). Although side effects from bupivacaine are rare, they can be serious, ranging from CNS excitation to cardiovascular collapse from direct cardiotoxicity. A series of case reports of possible toxicity of racemic bupivacaine secondary to continuous infusions of bupivacaine were reported in the early 1990s (Agarwal et al., 1992; McCloskey et al., 1992). In the report of McCloskey and coworkers, children had continuous caudal infusions of 0.25% bupivacaine at dosages between 1.67 and 2.5 mg/kg per hour. One neonate sustained bradycardia and hypotension, and two older children developed seizures. Bupivacaine serum concentrations at the time of the event ranged between 5.6 and 20.3 mcg/mL. In the cases reported by Agarwal and associates (1992), one child who developed a seizure had an intrapleural catheter with a bupivacaine infusion rate of 0.5 mg/kg per hour. The seizure occurred with a plasma bupivacaine level of 5.6 mcg/mL. The other child in this report had a continuous bupivacaine epidural infusion at 1.25 mg/kg per hour, and seizures occurred with a plasma bupivacaine level of 5.4 mcg/mL. Thus, all children in the reports from the McCloskey and Agarwal groups had plasma bupivacaine levels that exceeded bupivacaine’s toxic threshold of 4 mcg/mL.
These early cases of toxicity prompted an editorial by Berde (1992), who addressed the false generalization that children were more resistant to local anesthetic toxicity than adults. Although earlier studies involving children noted no evidence of toxicity in patients with plasma concentrations of bupivacaine from 1 to 7 mcg/mL, the use of benzodiazepines in those children may have been protective (McIlvaine et al., 1988). Badgwell and coworkers (1990) reported greater resistance to toxicity in 2-day-old piglets compared with older piglets. However, the direct application of this study to neonates is difficult, because neonates have lower plasma protein concentrations and lower bupivacaine clearance. Berde (1992) recommended that maximal allowable dosages of local anesthetics should not be exceeded (Table 16-2), that infusion rates should be reduced in children with risk factors for seizures, and that the maximal allowable dosages should be reduced by at least 30% for infants less than 6 months of age.
Ropivacaine
Ropivacaine has become a commonly used local anesthetic in pediatric patients, with onset times similar to bupivacaine, and durations of actions that are similar or perhaps slightly longer than bupivacaine (Ivani et al., 1998; DaConceicao et al., 1998, 1999; Lonnqvist et al., 2000; Hansen et al., 2001a; Ivani, 2002). Controversy remains as to the potency of ropivacaine when compared with bupivacaine, and adult studies do not correlate with pediatric studies (Ivani, 2002). Although not confirmed, ropivacaine at 0.2% exhibits the same analgesic effect as 0.25% in children, perhaps because of the intrinsic vasoconstrictive activity that is evident at the lower concentrations used in children (Kopacz et al., 1989; Ivani, 2002). Ropivacaine has a lower risk for CNS and cardiac toxicity than bupivacaine. In fact, inadvertent intravenous ropivacaine in a 1-year-old child failed to produce neurotoxic or cardiotoxic signs or symptoms (Thong et al., 2000). In a pharmacokinetic study of ropivacaine in children ages 1 to 8 who were administered 1 mL/kg of 0.2% ropivacaine for caudal block, the free plasma concentrations were well below toxic levels (Lonnqvist et al., 2000). Clearance of the drug was 7.5 mL/kg per minute, and the terminal half-life was 3.2 hours. Hansen and associates (2001a) studied the pharmacokinetics of caudal ropivacaine in infants less than 1 year of age (see Table 16-1). In this study, infants less than 3 months of age were compared with infants 3 months to 1 year. Although the maximum free concentration of ropivacaine was significantly higher in the younger age group (99 mcg/L versus 38 mcg/L), the total and free plasma concentrations in all of the children less than 1 year were within the range of concentrations previously reported for adults and older children (Lonnqvist et al., 2000; Wulf et al., 2000).
Epidural ropivacaine in children aged 1 to 9 years was studied to determine pharmacokinetics and safety of 24- to 72-hour infusions (Berde et al., 2008). After a 2-mg/kg bolus, an infusion of 2 mg/mL at 0.4 mg/kg per hour was delivered and resulted in plasma concentrations that remained stable throughout the infusion and were well below toxic levels.
Levobupivacaine
Levobupivacaine is the S(−) enantiomer of bupivacaine and is less toxic to the CNS or heart than the racemic bupivacaine (Mazoit et al., 1993; Graf et al., 1997; Huang et al., 1998). The decreased risk for cardiotoxicity from levobupivacaine has been shown in healthy adult volunteers after intravenous administration of either levobupivacaine or racemic bupivacaine (Bardsley et al., 1998). Although similar intravenous studies have not been done in children, animal and human studies suggest less toxicity and equipotency of levobupivacaine compared with bupivacaine (Mather and Chang, 2001; McLeod and Burke, 2001; Gristwood, 2002). In pediatric studies, levobupivacaine has resulted in wide ranges of plasma concentrations when given in the caudal space (Chalkiadis et al., 2004, 2005). When levobupivacaine 0.25% was given in a single-shot caudal dose of 2 mg/mL in children less than 2 years of age, Chalkiadis and coworkers (2004) found that the time to peak plasma concentration ranged between 5 and 60 minutes and was reached later in children less than 3 months of age, and the plasma concentration ranged between 0.41 and 2.12 mcg/mL. In a similar study, but at a dosage of 2.5 mg/kg, the range was 0.62 to 2.4 mcg/mL, with the highest maximum concentration found in a 1-month-old infant (Cortinez et al., 2008). The authors suggested using caution when administering levobupivacaine at 2.5 mg/kg in the caudal space in this age group.
Toxicity Comparison among Commonly Used Local Anesthetics
Multiple studies have compared the risk for toxicity of various local anesthetics. Single high-dosage intravenous injection of the local anesthetics in the ewe model revealed that bupivacaine had the most pronounced changes in electrocardiographic and hemodynamic variables compared with ropivacaine, levobupivacaine, and lidocaine (Guinet et al., 2009). In addition, only the bupivacaine ewes experienced ventricular tachycardia. A recent review suggested that overall, ropivacaine has the greatest margin of safety of the long-acting local anesthetics that are currently available, partially because lipophilicity is a major determinant in local anesthetic toxicity (Zink and Graf, 2008).
Reducing Risks of Toxicity
Several safety measures can reduce the risks of toxicity from local anesthetics in children. One primary safety measure is to avoid overdosing by adhering to recommendations for maximal allowable dosages. For a bolus injection, the maximal recommended dosage of lidocaine is 5 mg/kg. Because epinephrine decreases uptake and absorption of local anesthetic, the dosage of lidocaine can be increased to 7 mg/kg when epinephrine (5 mcg/mL) is added to the local anesthetic. The maximal allowable bolus dosage for bupivacaine, ropivacaine, and levobupivacaine is 3 mg/kg, and the addition of epinephrine does not change the maximum (Agarwal et al., 1992; McCloskey et al., 1992). Recommendations for maximal dosing of continuous infusions (see Table 16-2) are the same for bupivacaine, levobupivacaine, and ropivacaine (Berde, 1992). For epidural infusions, the infusion rates should not exceed 0.4 to 0.5 mg/kg per hour for patients older than 6 months, and the recommendation for neonates is not to exceed 0.2 to 0.25 mg/kg per hour. Based on the clearance of bupivacaine and using the guidelines in Table 16-2, the plasma concentrations should remain below 2.5 mcg/mL and therefore below the toxic level of 4 mcg/mL (Tucker, 1986; Desparmet et al., 1987; Mazoit et al., 1988). These infusions assume a loading dosage of 2 to 2.5 mg/kg of bupivacaine and should be reduced by 25% in patients with risk factors for seizures (i.e., past history of febrile seizures, hypomagnesemia, and hyponatremia) (Agarwal et al., 1992; Berde, 1992).
Although there is extensive literature on maximal allowable dosing of local anesthetics, recommendations in children are mostly extrapolated from adults. Additionally, the recommendations for adults are not evidence based and are in turn extrapolated from animal experiences, blood concentration studies, local anesthetic toxicity case reports, and pharmacokinetics (Rosenberg et al., 2004). In the adult literature, it has even been suggested that the idea of maximal blanket dosing be abandoned, as it is not scientifically valid or clinically relevant (Heavner, 2004). This criticism suggests that the maximum dosages that are quoted to be valid in all patients are not truly valid, and one blanket recommendation does not actually cover all types of patients or all types of blocks. Instead, block- and site-specific guidelines are needed so that dosing is tailored to the patient’s situation. In addition, dosing must be based on children’s per-kilogram weight; this is the only way to safeguard the risk of overdosing local anesthetics in different age groups.
In addition to dosing guidelines, the risk for toxicity is also affected by hypothermia, hypoxia, hypercarbia, acidosis, or hyperkalemia (Broadman, 1996). These factors enhance the toxicity of local anesthetics by different mechanisms. Another factor that leads to increased toxicity is the speed of injection of the local anesthetic agent. Rapid injections can cause toxicity by resulting in a rapid high peak that may not allow the sodium channels to adapt for the local anesthetic action.
When local anesthetics are combined, their toxicities are additive. Therefore, if the maximal allowable dosage of one local anesthetic has been reached, another local anesthetic agent should not be delivered (Giaufre et al., 1996). Although combining local anesthetics may be common at some centers, maximal allowable dosing should be calculated for each of the anesthetics used and decreased based on the relative percentage of each.
The choice of local anesthetic is a clinical decision. The risks for potential cardiotoxicity have been discussed and should help guide practitioners in choosing a local anesthetic that they feel has the greatest safety margin. After the local anesthetic is chosen, the concentration must be considered. In general, lower concentrations of local anesthetics such as 0.25% bupivacaine or levobupivacaine and 0.2% ropivacaine may be used in infants and small children, and higher concentrations such as 0.5% bupivacaine, levobupivacaine, or ropivacaine should be reserved for older children. Higher concentrations result in longer duration of action and increased motor block, but the total milligram dosage must be calculated carefully. Additionally, in young children there is a risk for direct local anesthetic toxicity on developing nerves (Selander, 1993; Lambert et al., 1994; Radwan et al., 2002). The age at which this is no longer a concern is unknown.
Test dosing
Before injecting local anesthetics, it is essential to determine that the agent will not be injected into the intravascular space. The absence of blood aspiration when administering a pediatric block is not a reliable indicator that the needle is not in a vessel. Infants, in particular, may be at greatest risk of intravascular injection (Freid et al., 1993; Ved et al., 1993; Flandin-Blety and Barrier, 1995). Although clinical practice has relied on a test dose to elicit a tachycardia when the test drug is inadvertently injected systemically, the test dose was often considered unreliable in the anesthetized patient and its usefulness continues to be questioned (Desparmet et al., 1990; Sethna and McGowan, 2005). In Desparmet’s study of children anesthetized with halothane, only 16 of 21 children who received a test dose of 0.1% lidocaine with epinephrine 1:200,000 had an increase in heart rate that was greater than 10 beats per minute (bpm). Therefore, under halothane anesthesia, an increase of 20 bpm with prior administration of atropine improves the efficacy of the test dose. Sethna and associates (2000) determined that, with the patient under isoflurane anesthesia and after pretreatment of atropine, 0.75 mcg/kg epinephrine was more reliable than 0.5 mcg/kg. All patients in the 0.75-mcg/kg group had heart rate increases of 10 bpm or greater. Isoproterenol has also been used as a test drug, but although it is a more reliable indicator of an intravascular injection in patients anesthetized with halothane (Perillo et al., 1993; Kozek-Langenecker et al., 1996), its safety with neuraxial administration has not been determined, so it is not recommended for this use presently. The validity of test dosing has been studied and reviewed (Tanaka and Nishikawa, 1998, 1999; Tobias, 2001b). With the patient under sevoflurane anesthesia, Tanaka and Nishikawa (1998, 1999) were able to demonstrate that an increase in heart rate of 10 bpm, an increase in T-wave amplitude of 25%, or an increase in blood pressure of 15 mm Hg or more defines the criteria for a positive test dose. Prior administration of atropine did not affect these criteria. Varghese and coworkers (2009) had similar results when 0.1 mL/kg of intravenous 1% lidocaine with 5 mcg/mL epinephrine was injected in children under sevoflurane anesthesia to determine if T-wave amplitude would be visibly detectable on the monitor. An increase in T-wave amplitude in lead II was detected on the electrocardiographic (ECG) monitor in 91% of children and noted on the ECG strip in 94% of children. The amplitude change was considered more reliable for detecting intravascular injection than the increase in heart rate of 10 bpm, which occurred in 64% of children, or the increase of 15 mm Hg or greater in systolic blood pressure, which occurred in 76%. The increase in T-wave amplitude occurred within 30 seconds, as was found in other studies (Tanaka and Nishikawa, 1998; Kozek-Langenecker et al., 2000). Langenecker and associates (2000) delivered an epinephrine solution at 0.5 mcg/kg without being mixed in a local anesthetic and also concluded that the T-wave criterion was more reliable than hemodynamic criteria for a positive test dose.
A test dose can be a useful tool for identifying intravascular injection when the appropriate criteria are used. The test dose should not, however, be a replacement for slow incremental dosing of the total volume of the local anesthetic, with attention to vital sign and ECG monitoring throughout the drug administration. A recommended test dose under sevoflurane anesthesia is 0.1 mL/kg of the chosen local anesthetic with epinephrine 5 mcg/mL added, not to exceed 3 mL. A heart rate increase of 10 bpm, a systolic blood pressure increase of 15 mm Hg, a T-wave amplitude increase of 25% or greater from baseline, or bradycardia should alert the practitioner to possible intravascular injection (Freid et al., 1993; Fisher et al., 1997; Tanaka and Nishikawa, 1998, 1999; Tobias, 2001b).
Although it has not been extensively tested under total intravenous anesthesia (TIVA), there is evidence that the test dose criteria used for determining intravascular injection differ between TIVA and inhaled anesthesia (Polaner et al., 2010). Arterial blood pressure was the most consistent finding, with an increase in all patients of at least 30% within 2 minutes of injection. Heart rate increased by 10 bpm in only 73% of children, and t-wave amplitude was not reliable. Of note in this study, the injectate was 0.1 mL/kg bupivacaine 0.25%, with epinephrine 1:200,000.
Another advantage of epinephrine administration in regional anesthesia is that, in addition to its being a reliable marker for intravascular injection, its use decreases systemic absorption of the local anesthetic (Eyres et al., 1983, 1986). Children may be at increased risk for local anesthetic toxicity from increased systemic absorption because of their relatively higher cardiac output and regional blood flow. In a study comparing bupivacaine with epinephrine 5 mcg/mL to bupivacaine alone in children for fascia iliaca block, Doyle and coworkers (1997) demonstrated that the peak plasma concentration was lower in the bupivacaine-with-epinephrine group (0.35 mcg/mL versus 1.1 mcg/mL), and that the time to peak concentration was more gradual (20 minutes versus 40 minutes from injection). Similarly, the addition of epinephrine to caudal ropivacaine significantly lowers the maximal concentration of ropivacaine and delays the time to maximum concentration, thus improving the safety profile in children (Van Obbergh et al., 2003). In adults, this same delay in systemic absorption and a reduced peak plasma concentration have been shown when epinephrine was added to ropivacaine for paravertebral block (Karmaker et al., 2005). These findings do not suggest that the addition of epinephrine extends the duration of analgesia, but they do demonstrate the effects of epinephrine on the pharmacokinetics of local anesthetics, including those with intrinsic vasoconstrictive properties. Epinephrine (2.5 to 5 mcg/mL) should be added to local anesthetic doses, particularly when large volumes are used, to reduce the risks of local anesthetic toxicity (Rosenberg et al., 2004).
Management of Local Anesthetic Toxicity
Despite all efforts to safely deliver a local anesthetic to a child, it is still possible for local anesthetic toxicity to occur, either by undetected intravascular injection or from inadvertent overdose. When it is apparent that there is local anesthetic toxicity, the practitioner must act quickly to prevent the serious cardiac complications that may ensue. The first steps should focus on the basics, with attention to oxygenation, ventilation, and cardiovascular resuscitation. In particular, hypercarbia must be avoided when resuscitating a patient with local anesthetic toxicity, as an increased Paco2 displaces local anesthetics from their plasma protein binding sites (Burney et al., 1978).
A significant change in the overall management of local anesthetic toxicity has been the introduction of the delivery of a lipid emulsion (Weinberg, 2006). In the clinical arena, lipid emulsion has been used to treat the neurotoxicity and cardiotoxicity that occur from bupivacaine, levobupivacaine, ropivacaine, and mepivacaine (Foxall et al., 2007; Litz et al., 2008; Warren et al., 2008; Marwick et al., 2009). These long-acting local anesthetics are highly soluble in lipid emulsion, and this high binding capacity explains the clinical efficacy of action, although several mechanisms of action have been proposed (Weinberg et al., 2006).
When comparing lipid emulsion (Intralipid) with epinephrine for bupivacaine-induced cardiotoxicity in a rat model, the lipid infusion resulted in better overall resuscitation hemodynamics than epinephrine (Weinberg et al., 2008). In addition, epinephrine resulted in higher lactate levels, hypoxemia, a mixed acidosis, and persistent ventricular ectopy. When compared with saline, epinephrine had similar hemodynamic and metabolic metrics during resuscitation.
Many questions remain regarding the use of Intralipid, and no clear consensus has been reached as to when Intralipid should be delivered (Weinberg, 2009). One school of thought is that the potential risks of administering the high dosages of Intralipid are yet uncertain, and therefore it should be used only after advanced cardiac life support has failed (Corman and Skledar, 2007). Potential risks include infection, intravenous thrombophlebitis, altered inflammatory responses, anaphylaxis, and fat emboli (Brull, 2008). Serum amylase increases and the risk for acute pancreatitis are concerns (Marwick et al., 2009). Certainly for the neonate, who is less likely to be able to handle a large Intralipid load, caution should be used and the risk-benefit ratio weighed prior to dosing. These risks certainly exist, but withholding Intralipid in the event of a local anesthetic–induced arrest would not be advised. The question remains whether it should be the first step in the resuscitation. Intralipid impairs return of cardiac function in the presence of profound hypoxia (Harvey et al., 2009). Likewise, Mazoit and associates (2009) found that a drop in pH, as might occur during resuscitative efforts, decreases the affinity of lipid emulsion for the local anesthetics ropivacaine and bupivacaine. Particularly after the use of epinephrine at dosages of 10 mcg/kg or higher, there is a decrease in successful resuscitation efforts in the presence of bupivacaine toxicity (Hiller et al., 2009). Although epinephrine results in a faster onset of spontaneous circulation than lipid rescue, the effect is not prolonged, and the overall inefficiency in resuscitation in the presence of this dosage of epinephrine probably results from high levels of lactate. These studies would support the early use of Intralipid for improved result.
To effectively use Intralipid, it should be immediately available with clear instructions attached for the practitioner. Dosing guidelines are presented in Box 16-1. As discussed, no specific guidelines have yet been established, and dosing relies only on a series of case reports and animal studies. Nevertheless, having some starting point is essential. A sufficient quantity of Intralipid must be immediately available, as it is possible for a patient to have recurrence of cardiotoxicity after lipid rescue that may require additional support (Marwick et al., 2009). It is important to distinguish that a 20% lipid emulsion has been effective for resuscitation, not propofol, which is 10% Intralipid and 1% propofol and would contribute further to cardiovascular depression in the arrest situation (Weinberg et al., 2004).
Box 16-1 Dosing Guidelines of Intralipid Infusion for Local Anesthetic Toxicity
A website (http://lipidrescue.org) was developed to disseminate information about lipid rescue therapy and to allow practitioners to share their experiences.
Regional Anesthesia in the Anesthetized Child
A significant difference between adult and pediatric regional anesthesia is that children typically receive their regional anesthetic while they are under general anesthesia. This practice remains controversial outside of the pediatric arena (Bromage, 1996; Bromage and Benumof, 1998; Rosenquist and Birnback, 2003). Because of issues regarding patient cooperation, the practice of performing a regional anesthetic in children differs greatly from placing a block in an adult. Performing regional anesthetic blocks during general anesthesia in children, including thoracic epidural blocks, is an accepted practice as long as the individual has the proper training and expertise. Over 50 international pediatric anesthesiologists signed an editorial by Krane and coworkers (1998) to support the placement of blocks in anesthetized children. In fact, “It would be considered malpractice to perform such techniques in patients who were not fully anesthetized” (Dalens, 1999), and “Any performance of a block in an agitated and moving child is not only unethical, but could be dangerous when the needle approaches the delicate nervous structures” (De Negri et al., 2002). A large retrospective study of 4298 adult thoracic surgical patients who underwent epidural catheter placement while under general anesthesia revealed no neurologic complications including radicular symptoms or persistent paresthesias (Horlocker et al., 2003a).
In children who are under general anesthesia or who are heavily sedated, it may be difficult to recognize intravascular injection of a local anesthetic. Even in the awake child, recognizing the signs or symptoms of local anesthetic toxicity is challenging, and it would be optimistic to think that the child would be able to relay this information early in the injection phase. For this reason, the practice of test dosing with a local anesthetic with the addition of epinephrine has been readdressed, and this should be a common practice as it is more reliable than patient report for detecting or preventing intravascular injection (Tobias, 2001b; Bernards et al., 2008). It is also argued that an anesthetized child cannot warn the practitioner of a significant paresthesia and the potential risk for neurologic injury from intraneural placement of a needle or anesthetic. This is a hypothetical risk that has not been supported by reports of large series of pediatric regional anesthetics (Pietrapaoli et al., 1993; Goldman, 1995; Giaufre et al., 1996).
The American Society of Regional Anesthesia and Pain Medicine issued a practice advisory that was based on evidence and expert opinion as it pertains to performing procedures on anesthetized or heavily sedated patients (Bernards et al., 2008). These recommendations (see Box 16-2) differ somewhat from the recommendations set forth for adults.
Box 16-2 Recommendations* for Performing Regional Anesthesia in Anesthetized or Heavily Sedated Children
Modified from Bernards CM, Hadzic A, Suresh S, et al: Regional anesthesia in anesthetized or heavily sedated patients, Reg Anesth Pain Med 33:449, 2008.
An additional concern with regional anesthesia in an anesthetized child is that of “wrong-side block” for a unilateral extremity procedure. Universal protocols must be followed in the conscious child: the operative extremity must be appropriately marked by the surgical team with concurrence of a witness as well as the parent, and the consent must be checked for accuracy regarding the appropriate site. A time-out should occur before blocking the anesthetized child, to again check the consent and marked site. See related video online at www.expertconsult.com.
Direct Nerve Toxicity
The use of local anesthetics and neurotoxicity on the developing nerve is an area that continues to be addressed. Animal data have demonstrated that all local anesthetics are potentially neurotoxic, and this neurotoxicity parallels their anesthetic potency (Selander, 1993). The factors that contribute to the mechanism of the neurotoxicity include the concentration of the local anesthetic and the time of exposure of the nerve to the local anesthetic. This is important in children, particularly in neonates, who may be at greatest risk for direct neurotoxicity during nerve development and therefore should not receive the higher concentrations of local anesthetics. Studies on rabbit nerve fibers have demonstrated an increased sensitivity to the blocking effects of local anesthetics in young nerves (Benzon et al., 1988). Additional in vitro biological investigation has demonstrated that lidocaine, bupivacaine, mepivacaine, and ropivacaine are all capable of producing growth cone collapse and neurite degeneration (Radwan et al., 2002). However, the incidence of growth cone collapse with bupivacaine and ropivacaine is insignificant when compared with lidocaine and mepivacaine. This finding has not been consistent. When looking at cytotoxicity of local anesthetics on peripheral nerves by measuring viability and apoptotic activity, although all local anesthetics decreased cell viability in a concentration-dependent fashion, bupivacaine had the greatest effect (Castro-Perez et al., 2009). The order of the killing potency (based the concentration at which 50% of cells are killed, or the median lethal dose [LD50]), from high to low, was bupivacaine, then ropivacaine, then chloroprocaine, then lidocaine, then mepivacaine, and finally, with much lower effect, procaine. Only bupivacaine and lidocaine killed all cells with increasing concentration and could cause apoptosis with either the increased concentration or time of exposure. The authors of the study concluded that cytotoxicity-induced nerve injury may have different mechanisms for the various local anesthetics, and that the targets may not be neurons, because they found that bupivacaine was the most toxic local anesthetic that they tested, but it has a history of having a low incidence of producing transient neurologic symptoms in the postoperative period.
Risk of Infection
Another safety consideration concerns the risk for infection. An initial way to avoid infection is by preparing the skin prior to a regional block. Before placing any block, sterile preparation of the skin should be performed, but this is particularly important for central blocks, to reduce the risk for meningitis or epidural abscess. Chlorhexidine, despite its labeling, is the agent of choice for preparing the skin prior to regional anesthetic block (Hebl, 2006). It is recommended over the use of povidone iodine (Povidine), as it has been shown to decrease colonization when used in young children for epidural catheter placement (Kinirons et al., 2001; Wagner and Prielipp, 2003). In addition, povidone iodine may be harmful to the very sensitive skin of an infant. If used, the povidone iodine should be allowed to dry and not be carried centrally with the needle into the epidural or subdural spaces. After the block has been placed, the iodine should be washed from the skin to avoid iodine burns.
The actual risk for infection from regional techniques, however, is extremely low. For indwelling caudal catheters, the incidence of catheter tip colonization is 20%, as opposed to 4% for indwelling epidural catheters (McNeely et al., 1997b). No patients with bacterial colonization of the catheters exhibited systemic signs of infection. Strafford and associates (1995) studied 1620 children who received epidural catheters. There were no infections in the children who had the catheters placed for postoperative analgesia, and only one significant infection in an immunosuppressed child who received a long-term catheter for pain secondary to her malignancy (Strafford et al., 1995). Adult review has confirmed that infections are typically limited to long-term indwelling catheters in cancer patients (Ruppen et al., 2007). Giaufre and coworkers (1996), in a prospective study of over 24,000 regional techniques performed in children by members of the French-Language Society of Pediatric Anesthesiologists, reported no infections.
Compartment Syndrome
A concern often cited for failure to perform a regional anesthetic in pediatric patients for orthopedic procedures is the risk of an unrecognized compartment syndrome. Compartment syndrome puts the child at risk for muscle ischemia or loss of limb and must be recognized early so that decompressive fasciotomy may occur if necessary prior to these serious complications. The concern focuses on the possibility that the use of a local anesthetic in a regional block may mask the initial symptoms of the sensation of pressure in the limb that occurs with compartment syndrome (Dunwoody et al., 1997). Case reports in children have demonstrated that a successful epidural block with a low concentration of local anesthetic does not mask the symptoms of compartment syndrome. A literature review to assess the risk for compartment syndrome in children who have received epidurals revealed 12 cases (Johnson and Chalkiadis, 2009). In all cases, pain was the primary finding and was considered more severe than the clinical situation would explain. Five of the patients had compartment syndrome as a result of lithotomy position rather than operative site edema. The authors concluded that warning signs of compartment syndrome are the presence of increasing pain or pain that is remote to the site of surgery, an increase in analgesic requirements, paresthesia not due to analgesia, reduced perfusion, swelling, and pain on passive movement. They suggested that the presence of the epidural catheter did not result in delayed diagnosis but instead contributed to deficiencies in the system. Recommendations made included the following: educate staff as to the signs and symptoms of compartment syndrome, identify which patients are particularly at risk through discussion with the surgeon, use dilute local anesthetic solutions, site epidural catheters as close as possible to involved dermatomes, and assess distribution and density of block frequently. Serial examinations should be performed on children to assess the operated extremity in the presence of good analgesia. For the high-risk child, or if any question exists, measure compartmental pressures postoperatively, particularly in children who would clearly benefit from infusions of local anesthetic, such as those who have undergone microvascular surgery or amputation.
Consent Issues
Performance of a regional block must follow the same guidelines as performance of a surgical procedure with consideration of the risk-benefit ratio, a discussion of complications, and appropriate documentation to support its use. Not only should the more common complications be discussed, but the rare, serious complications should also be part of the preoperative consent process with regard to block placement in a child (Domino, 2007). The manner by which this occurs varies with the country in which the procedure is performed, but some effort must be made to make the parents aware of the purpose, benefits, and risks of regional anesthesia for their child (Lonnqvist et al., 2009).
Reviews of Safety of Regional Anesthesia in Children
Despite the issues of the safety of regional anesthesia in children, studies have shown that the risks and complications of regional anesthesia in children are quite low and often preventable (Dalens and Hasnouai, 1989; Pietropaoli et al., 1993; Giaufre et al., 1996; Dalens and Mazoit, 1998). The largest of these studies was published by the French-Language Society of Pediatric Anesthesiologists (ADARPEF) (Giaufre et al., 1996). This prospective report included 24,409 regional blocks performed over 1 year in children. Central blocks accounted for greater than 60% of the blocks, whereas peripheral nerve blocks and local anesthetic techniques made up the remaining 38%. Only 25 complications occurred in the study, and all the complications occurred in children who received central blocks. Thus, the overall complication rate of regional anesthesia was 0.9 per 1000. The most common complications from regional blockade were inadvertent dural puncture (n = 8), inadvertent intravascular injection of local anesthetic (n = 6), technical problem (n = 3), and overdosing of local anesthesia leading to dysrhythmias (n = 2). In addition, two children had transient paresthesias, one child had apnea after central morphine, and one child had a skin lesion after a caudal anesthetic administration. There were no deaths secondary to any of the complications. The conclusion from this study of regional anesthesia in children was that complications were rare and minor, and that they occurred most often in the operating room where they were readily managed. In addition, when appropriate, a peripheral nerve block may be preferable to a central block.
In a retrospective review of 24,005 regional anesthetics administered over a 10-year period, Flandin-Blety and Barrier (1995) reported 108 events without sequelae (0.45%). However, in this review, five events resulted in severe neurologic injury, including tetraplegia in three children, paraplegia in one child, and one child with cerebral lesions. All five of the children were healthy and less than 3 months of age. Four of the five children had loss of resistance to air used in their technique to identify the epidural space. The true pathophysiologic causes for the neurologic injuries in these children are unknown, but the authors recommended that air not be used to identify the epidural space, and a lower concentration of epinephrine (e.g., 2.5 mcg/mL) be used to avoid possible ischemic injury. In addition, the authors recommended that the indications for regional anesthesia be reconsidered in children less than 18 months of age because of their incomplete myelination of neuronal fibers.
Recent studies have also looked at new technology and whether these advances have improved the practice of regional anesthesia. Rubin and associates (2009) reviewed the literature on ultrasound-guided blocks and suggested that improved safety features included the need for a lower volume of local anesthetic, and better visibility. How these findings extrapolate to whether ultrasound improves overall safety of regional blocks in children is unclear and not adequately demonstrated except for ilioinguinal blocks.
Introduction to ultrasound
Before discussing specific blocks in pediatric anesthesia, the place of ultrasound in the practice of pediatric regional anesthesia should be appreciated. The use of ultrasound for regional anesthesia has experienced immense growth in recent years. The premise of image guidance for nerve localization could not have a better suitor than a developing pediatric anatomy that defies predictable landmarks and has a narrow margin of safety. Despite the demonstrated reduction in the volume of local anesthetic required, the faster onset and longer duration of sensory and motor blocks, and improved quality of sensory block, randomized, controlled studies examining the safety and efficacy of ultrasound-guided nerve blocks in children have been lacking (Marhofer et al.,1997, 1998, 2004; Willschke et al., 2005, 2006a). Therefore, this subject should be approached with the understanding of its evolving nature.
The development of ultrasound technology stems from the principles of piezoelectricity, the ability of crystals and certain other materials to generate an electric potential in response to applied mechanical stress. This effect was first described by brothers Pierre and Jacques Curie in 1880, shortly followed by the demonstration of the converse piezoelectric effect (strain to crystals when an electric field is applied) by Gabriel Lippmann in 1881. However, it took the military innovations of World War I, where ultrasound (sonar) was used to detect submarines, to fuel its use in the medical arena. The first Doppler-assisted nerve block was described in 1978 to locate the subclavian artery for placement of supraclavicular brachial plexus block, but it was not until 1994 that the two-dimensional imaging improved to allow for real-time visualization of the supraclavicular brachial plexus with observation of local anesthetic spread (la Grange et al., 1978; Kapral et al., 1994). Ultrasound guidance has since been used to visualize most remote peripheral and central nerves in both adults and children. This field continues to expand as portable machines with high-resolution capabilities are perfected and three-dimensional ultrasound delineates additional sonoanatomy (Clendenen et al., 2009).
Physics of Sonography
Doppler
When performing regional blocks, it is important to visualize and avoid vascular structures. Color Doppler ultrasonography uses the Doppler effect (a frequency shift that occurs when either a wave source or receiver moves) to assess blood flow and overlays the color velocity mapping on the two-dimensional gray image. The moving red blood cells act as a reflecting medium in the vessels and amplify the perceived signal frequency (red) when moving toward the transducer or diminish it when moving away (blue). The red and blue reflect the direction of the flow to the transducer, not whether the flow is arterial or venous. The image appears black when the wave hits red blood cells perpendicular to the flow. Power color Doppler is a recent alternative that enhances flow detection regardless of the angle of incidence, but it lacks directional information (Rubin et al., 1994).
Artifacts
The complex physics behind ultrasound image derivation frequently creates image distortions with no anatomic basis. Accurate interpretation not only avoids erroneous needle adjustment but also guides corrective action (Sites et al., 2007a, 2007b). Suspect structures can be confirmed by examining them in a second plane.
Types of Ultrasound Transducers
Selection of transducer type, footprint size, and frequency are critical to successful nerve block performance (Fig. 16-2). Linear transducers produce rectangular images and offer superior sonographic views for superficial structures (<4 cm). This ability makes this type of transducer ideally suited for the majority of pediatric examinations. Hockey-stick probe configuration offers the additional advantage of smaller footprint (25 mm), ergonomic shape capable of navigating the pediatric landscape, and a broadband frequency (6 to 13 MHz) adequate to examine even deep pediatric blocks such as the psoas compartment block. Curvilinear probes create a wedge-shaped image capable of reaching deep structures, but they sacrifice lateral resolution as the beam disperses in the far field of the curved array. This type of transducer is useful for working with teenagers and obese children to perform deeper blocks or for epidural guidance. Phased-array transducers have the smallest footprint and electronically guide their beam to diverge distally. They produce deep panoramic views most suited for transthoracic echocardiography and hold little use for regional practice.
Training and Safety
Inexperience is highly correlated with an increased complication rate, and in this age of heightened patient safety, awareness may preclude talented physicians from realizing the benefits of ultrasound-guided regional anesthesia. Recent publications have increasingly focused on optimizing the learning environment and on designing a learner-centered curriculum (Sites et al., 2007c; Smith et al., 2009). The most common novice behavior patterns that were associated with poor block outcomes are as follows: (1) advancement of the needle when the tip was not well visualized, (2) unintentional probe movements, (3) failure to recognize an intramuscular location of the needle tip before injection, (4) poor ergonomics, (5) failure to identify maldistribution of local anesthesia, (6) operator fatigue, (7) failure to correctly correlate ultrasound sidedness to patient’s anatomy, and (8) inappropriate choice of needle insertion site and angle.
Role of the Nerve Stimulator in the Age of Ultrasound
Regional anesthesia took a giant step forward when it abandoned paresthesia and field infiltration for nerve localization and developed a scientifically based, quantitative technique of nerve stimulation (NS). In its 30-year reign, NS has demonstrated a high degree of safety and success rates (Neal et al., 2002). Today, ultrasound guidance offers a qualitative anatomic endpoint, provides the ability to observe local anesthetic spread during injection, and can be used to identify abnormal anatomy. However, it is difficult to be a staunch proponent of solo methodology when both modalities offer complementary evidence of needle-to-nerve proximity and may reduce the time necessary for trainees to successfully reach the desired peripheral nerve block (Orebaugh et al., 2007). Pediatric regional anesthesia presents the additional challenges of working with anesthetized patients unable to report pain on injection, and smaller nerve size that is difficult to locate by NS alone, especially in patients less than 10 years of age (Gurnaney et al., 2007). In addition, NS may yield functional information on proximity to other nerves (phrenic stimulation in parascalene/supraclavicular nerve blocks) and alert to intrafascicular injection when evoked motor response is present with less than 0.2 mA, although this is not a uniform finding (Tsai et al., 2008). Thus, it is reasonable to advocate dual guidance in performing nerve blockade on nerves capable of NS (Gebhard et al., 2008).
Central neuraxial blockade
Central blockade is performed in children for many of the same reasons that it is performed in adults, such as for bilateral lower extremity, abdominal, and thoracic procedures. The risks and benefits to performing central blocks are inherent to the type of block chosen. Central blocks performed in children include spinal blocks and epidural blocks, with the caudal technique being the most commonly used approach to the epidural space. Contraindications to central blockade include a child on anticoagulation therapy, a patient with a preexisting coagulopathy, and a patient or parent who refuses consent for the procedure. The guidelines for regional anesthesia and anticoagulation therapy in adults should be reviewed, as they may be useful for guiding the management of the child (Horlocker et al., 2003b).
Spinal Anesthesia
Spinal anesthesia was a very early form of regional anesthesia that was considered useful for children (Bainbridge, 1901; Tyrell-Gray, 1909). Since that time, spinal anesthetics have become an important anesthetic technique for reducing the incidence of postoperative apnea in premature and expremature infants (Harnik et al., 1986; Welborn et al., 1990; Krane et al., 1995; Somri et al., 1998). Infants who have continuing apnea at home, or a hematocrit of less than 30% are at particular risk for postoperative apnea (Cote et al., 1995; Welborn et al., 1991). Spinal anesthesia may also reduce the need for postoperative mechanical ventilation in infants who are less than 60 weeks of postconceptual age and after hernia repair (Huang and Hirshberg, 2001). The ability of a spinal anesthetic to densely block the dermatomes involved in inguinal hernia repair has kept this regional technique a popular choice in pediatric anesthesia for inguinal surgery. Often, a spinal anesthetic combined with liberal clear liquids until 2 hours before the procedure, and a pacifier intraoperatively, are enough to keep an expremature infant comfortable while he or she undergoes inguinal hernia repair. However, any expremature infant up to 44 weeks of postconceptual age, and up to 60 weeks of postconceptual age if the infant has risk factors such as anemia or ongoing apnea, should be a candidate for overnight monitoring after the surgery, whether or not a pure regional technique was used.
Although spinal anesthesia may be used in any age group, there are relatively few true indications for a spinal anesthetic in older children, who may be at increased risk for post–dural puncture headaches (PDPHs) from the technique (Wee et al., 1996).
Anatomy
The anatomic differences between an infant and a young child or adult are clinically significant and must be taken into consideration when performing a spinal technique. Common teaching has always suggested that the dural sac in a newborn ends at S3, and the conus medullaris may be located at L3 (Fig. 16-3). Willschke and coworkers (2007), however, in a study of 145 neonates using ultrasound for epidural catheter placement, determined that the termination of the spinal cord in neonates actually ended at a median of L2. Likewise, Shin and associates (2009) found that the end of the dural sac was at a median level of the upper S2 in children less than 36 months of age. Even in children up to approximately 6 years of age, the dural sac ascended only slightly on sonographic examination, to remain near the level of S1 or S2 (Shin et al., 2009). This differs significantly from the final position in adults.
Technique
A sterile preparation and a clear plastic drape are used so that the anatomy may be seen (Fig. 16-4). For neonates and infants, a 1½-inch 22-gauge spinal needle is inserted at the L4-5 interspace. The stylet of the needle should be in place when it is passed through the skin. This avoids the remote risk for an epidermoid tumor (Shaywitz, 1972; Barnitzky et al., 1977). Using this size needle in this age group, a pop will be felt as the needle enters the ligamentum flavum, and another pop as the needle enters the dura. The stylet is removed to check for the flow of CSF. In a baby positioned in the lateral position, if no CSF is evident after what seemed to be appropriate needle placement, then the procedure may be repeated with the infant in the sitting position to improve CSF flow.
After confirmation of position into the subarachnoid space as evidenced by free flow of CSF through the needle, the local anesthetic solution is slowly injected. After the injection, the needle is removed and the child is placed in the supine position. It is extremely important that the child remain supine and that the legs not be raised for any reason, including placement of electrocautery pad. Lifting the legs will cause the local anesthetic to migrate and result in an undesirable high spinal blockade (Wright et al., 1990).
Dosing
The total volume of CSF in a neonate is 4 mL/kg, compared with 2 mL/kg in an adult, and the hydrostatic pressure is 30 to 40 mm H2O, lower than that of an adult. In addition, almost half of the total CSF volume is in the spinal subarachnoid space, whereas only one fourth of the total volume in adults is found in the spinal region. These factors play an important part of dosing spinal blocks, as the local anesthetics are quickly diluted by the CSF in a neonate on injection. Infants require higher volumes based on weight, but the duration of action of spinal blocks are shorter than in adults (Rice et al., 1994).
Although many different dosing regimens have been used, tetracaine either with or without epinephrine is a commonly employed local anesthetic for spinal anesthesia. Rice and associates (1994) studied 100 infants to determine the duration of spinal block, and the authors compared three groups: lidocaine 3 mg/kg with epinephrine, tetracaine 0.4 mg/kg plain, and tetracaine 0.4 mg/kg with epinephrine. The duration in the lidocaine with epinephrine group was 56 ± 2.5 minutes, the tetracaine plain group was 86 ± 4 minutes, and the tetracaine with epinephrine group was 128 ± 3 minutes.
When using 1% tetracaine for a spinal block in an infant, a dosage of 0.5 mg/kg mixed in an equivalent amount of 10% dextrose to make the solution hyperbaric should provide at least 90 minutes of surgical analgesia. Yaster and Maxwell (1989) suggested that regardless of the infant’s weight, 1.5 to 2 mg of tetracaine is the minimal effective dosage. To achieve 5 mg/mL of tetracaine, mix 1 mL of 1% tetracaine with 1 mL of 10% dextrose in water (D10W). The delivered volume is then 0.3 to 0.4 mL for infants less than 10 kg in weight. If 0.01 mL/kg of epinephrine is added to 0.75 to 1 mg/kg of hyperbaric tetracaine, surgical analgesia can be extended up to 120 minutes. Similarly, the use of clonidine will extend a spinal block in a former preterm infant, but the risk for apnea may be increased (Rochette et al., 2005). When using bupivacaine for spinal block, 0.5 to 0.6 mg/kg of either isobaric or hyperbaric bupivacaine will provide an average of 80 minutes of surgical analgesia (Dalens, 2000). Dosing of bupivacaine should be reduced for infants and young children who are greater than 5 kg in weight, because of changes in CSF volume. For infants or toddlers 5 to 15 kg, the dosage of hyperbaric bupivacaine or tetracaine is 0.4 mg/kg or 0.08 mL/kg, and for children weighing more than 15 kg, the dosage of bupivacaine or tetracaine is 0.3 mg/kg or 0.06 mL/kg (Dalens, 1995).
Isobaric ropivacaine has been studied in children for spinal anesthesia at a dosage of 0.5 mg/kg up to 20 mg (Kokki et al., 2005). The mean highest sensory level of block was T6 and duration was 96 minutes with a range of 34 to 210 minutes. Additional study on intrathecal ropivacaine is warranted.
Complications
Complications during spinal anesthesia in children are uncommon. In the ADARPEF study, an intravascular injection during spinal anesthesia was the only reported complication in 506 cases (Giaufre et al., 1996). Although considered to be a rare occurrence in children, the incidence of PDPH is actually between 10% and 50% in children aged 10 to 18 years (Oliver, 2002; Wee, 1996). The onset of symptoms from a PDPH typically occurs within 48 hours, with the hallmark symptom being a frontal or occipital headache that is postural. The cause of PDPH is most likely a persistent leakage of spinal fluid that causes a net decrease in CSF volume and intracranial pressure. The supine position helps to alleviate the symptoms of PDPH by decreasing the effect of gravity on the CSF leak. The size of the dural perforation is the primary predictor of the development of PDPH. To reduce the risk for PDPH, smaller-gauge needles are used, and the needle is inserted with the bevel parallel to the dura’s longitudinal fibers (Kokki and Hendolin, 1996). Electron microscopy has revealed that the collagen fibers of the dura are arranged in several layers, and that the thickness of the dura is more predictive of whether a leak will occur than the orientation of the needle’s bevel (Turnbull and Shepherd, 2003). This explains the unpredictability of PDPH.
If a diagnosis of PDPH is made, simple measures such as bed rest and hydration can decrease the volume of CSF loss. In addition, analgesics to reduce the headache and intravenous caffeine may be administered. Caffeine is effective because of its ability to cause cerebrovascular vasoconstriction, resulting in decreased cerebral blood flow. In one study, when caffeine was used prophylactically in adults, visual analog pain scores and analgesic demand after PDPH were lower (Yucel et al., 1999). There is no current pediatric dosage recommendation for caffeine. The oral dosage for adult patients is 300 to 500 mg once or twice a day (Turnbull and Shepherd, 2003). The intravenous formulation of caffeine is administered with sodium benzoate. The adult intravenous dosage is 500 mg. This may be repeated within 2 to 4 hours if the headache is unchanged after the first dose (Janssen et al., 2003).
If conservative measures to treat PDPH are ineffective after 48 hours, an epidural blood patch should be considered. This requires that blood be drawn sterilely from a peripheral vein. The blood is then injected into the epidural space under aseptic technique. An epidural blood patch is most effective 48 to 72 hours after the dural puncture, and it may be ineffective if performed immediately after dural tap because high leakage of CSF may interfere with blood clotting (Oliver, 2002). If a child is awake during the placement of an epidural blood patch, the practitioner should stop the injection once the child feels either discomfort or pressure in the back. If a child is anesthetized during the performance of an epidural blood patch, no more than 0.3 mL/kg of blood should be injected into the epidural space (Ylonen and Kokki, 2002).
Total spinal block with respiratory arrest and bradycardia is another complication of spinal anesthesia (Desparmet, 1990). The preganglionic sympathetic blockade that is commonly seen in adults secondary to a high spinal is not typically seen in children, particularly in infants (Oberlander et al., 1995; Finkel et al., 2003; Somri et al., 2003). Dohi and coworkers (1979) were the first to describe the lack of hemodynamic changes after spinal block induced sympathetic blockade in children. They found that children less than 5 years of age had little or no hemodynamic response to a T3-level tetracaine spinal anesthetic, whereas children older than 8 years had cardiovascular responses that were more similar to adults. The mechanism for this lack of hemodynamic sympathectomy was postulated to be the immaturity of the sympathetic nervous system as well as differences in CSF volume and spinal cord surface area. In addition, it is also possible that the smaller blood volume that is present in the lower extremities of a young child compared with that of an adolescent or adult may account for less venous pooling and therefore less hemodynamic change (Dohi et al., 1979; Dohi and Seino, 1986). Despite the typical lack of cardiovascular compromise, neonates occasionally require ventilatory support or pharmacologic intervention because of a high spinal anesthesia with a resulting blockade of the cardiac accelerator fibers or a decrease in stimulation of the right atrial stretch receptors (Wright et al., 1990). Investigation has shown that even former premature infants in the absence of fluid loading tolerate high spinal anesthesia with minimal autonomic changes (Oberlander et al., 1995).
Caudal Anesthesia
Although regional anesthesia in children has a broader scope with the advent of ultrasound, a commonly employed regional block in pediatric practice remains the caudal epidural block. The reasons for the success of this regional technique include not only its extensive safety record for children but also the ease of performing the block and teaching of the technique. See related video online at www.expertconsult.com. Schuepfer and associates (2000) evaluated the technical skills of residents in anesthesiology to determine the learning curve for performing a caudal block in a child. They found that there was a high success rate after only a limited number of cases.
Caudal blocks have great utility in ambulatory surgical patients and for inpatients. They can be administered as a single injection or as a continuous infusion. A caudal block can be used for any surgery that is performed on the lower abdomen or lower extremities (i.e., procedures involving innervation from the sacral, lumbar, and lower thoracic dermatomes). Commonly performed pediatric surgery such as inguinal hernia repair and orchiopexy with their dermatomal distribution below T10 make the caudal block a useful adjunct to the anesthetic. Caudal blocks result in improved patient pain scores when compared with patients having general anesthesia alone (Londergan et al., 1994). Single-shot caudal anesthesia when combined with postoperative ketorolac administration allows children who have undergone intravesical ureteroneocystostomy to be discharged on the day of surgery (Miller et al., 2002). For outpatient urologic procedures, caudal block with light general anesthesia was superior to local nerve block or general anesthesia alone. Single-shot caudal anesthesia has also been used in expremature infants as the sole anesthetic, to decrease the incidence of postoperative apnea, and to avoid the use of general anesthesia and narcotics (Bouchut et al., 2001b).
The practice of placing a caudal block before the incision would seem likely to improve postoperative care by providing preemptive analgesia. The benefit of providing preemptive analgesia through regional block has not been confirmed in pediatric caudal studies. Holthusen and coworkers (1994) noted that there were no significant differences in cumulative postoperative analgesic requirements or cumulative pain scores between children having caudal blocks placed either before or after their circumcisions. In a separate study comparing two groups of children who received caudal blocks for clubfoot repair, Goodarzi (1996) noted that there were no significant differences in the time to first postoperative analgesic administration or in cumulative analgesic requirements for the first 48 hours between the group that received the block before the incision and the group that received the block after the incision. However, it should be realized that placing the caudal at the end of the procedure does not allow the benefit of lower inhaled anesthetic agent concentrations to be used intraoperatively.
Anatomy
The caudal space is the result of a defect caused by the nonfusion of the fifth sacral vertebral arch. This area of the nonfusion forms the sacral hiatus, the entry into the caudal epidural space. The landmarks around the sacral hiatus are the sacral cornua, the posterior superior iliac spines, and the coccyx (Fig. 16-5). To find the sacral hiatus, one palpates the sacral cornua and the indentation that is immediately caudal and in the midline. If the cornua are difficult to palpate, the general area of the sacral hiatus may be found by palpating the two posterior superior iliac spines, and assuming that a line between these would be the base of an equilateral triangle, the apex should be at the location of the sacral hiatus.
The caudal space itself lies underneath the sacrococcygeal ligament that runs through the sacral hiatus under the skin. At around 7 years of age, the child’s caudal space begins to become more angulated and may be difficult to enter. Although it is possible to perform a caudal block in adolescents and adults, the formation of a presacral fat pad in puberty adds to the difficulty of placing the block. It should be remembered that the distance from the skin to the caudal space in neonates is minimal (Fig. 16-6), and because the dural sac may extend to S3 in neonates, the possibility of entering the dural sac in this age group is increased.
Technique
The choice of needle to be used depends on whether the caudal anesthetic is to be a single shot or whether additional dosing will be required. For a single-shot caudal, it is advantageous to use a short-beveled needle with a stylet. One may also use needles such as a blunt 22-gauge needle or an intravenous catheter that do not have stylets, but there is a remote risk of developing an epidermal inclusion cyst or tumor if the epidermis is carried through the shaft of the needle into the neuraxial space (Shaywitz, 1972). A Crawford needle is similar to an epidural Touhy needle, as it has a stylet and is blunt, but a Crawford needle’s bevel is in alignment with the shaft of the needle so that a catheter will exit the needle in a straight line rather than at an angle, such as with a Touhy. The Crawford needle is ideal for either single-shot local anesthetic injection or for placement of a caudal epidural catheter.
With the child in the lateral position, flex the hips with the dependent leg less flexed than the top leg (Simm’s position). Near the cephalad margin of the gluteal crease, feel for the sacral cornua and the sacral hiatus, a depression immediately inferior to these and in the midline. This is the sacral hiatus (see Fig. 16-5). After sterile preparation and draping, identify the sacral hiatus again with the nondominant gloved hand and place the needle into the skin in the midline at a 45-degree angle or less to the skin, aiming cephalad. Resistance might be felt as the sacrococcygeal ligament is penetrated with a pop once the needle has passed into the epidural space. If using a single-injection technique, local anesthetic may be delivered once the sacrococcygeal ligament has been pierced. Frequent aspiration for blood or CSF should occur, as small movements may result in misplacement of the tip of the needle. If using an intravenous catheter for entry into the caudal space, the angle of the needle must be dropped once the sacrococcygeal ligament has been pierced, to align the needle and intravenous catheter with the epidural space. The needle should then be advanced approximately 2 to 3 mm more, so that the catheter may be directly threaded into the epidural space. After a negative aspiration for blood and CSF, the local anesthetic should inject easily without resistance. A finger should palpate the skin cephalad to the injection to ensure that the agent is not being injected subcutaneously. Although air has been used to check for crepitus after injection, this practice is not recommended because of the risk for air embolism (Guinard and Borboen, 1993; Schwartz and Eisenkraft, 1993).
Technique Using Ultrasound
The superficial and mostly cartilaginous posterior vertebral segments of neonates and infants permit sonographic imaging of the neuraxial structures and have been applied to screen for the presence of spinal dysraphism (Kriss and Desai, 1998; Dick et al., 2002; Deeg et al., 2008). The ultrasound-guided caudal single-shot placement has been described in both children (Park et al., 2006; Roberts, 2006) and adults (Klocke et al., 2003; Chen et al., 2004). It decreases the failure rate by locating the sacral hiatus in the difficult-to-palpate patients (obese or older children), and by confirming the cannula placement in the caudal epidural space, thus limiting the incidence of intraosseous, intrathecal, and intravascular injections (Roberts et al., 2005).
A linear ultrasound transducer set at the highest operational frequency should be used to achieve maximal resolution of the superficial neuraxial anatomy. The child is positioned either prone or in a lateral position, with the operator next to or behind the patient, respectively, and with the ultrasound machine in the operator’s direct line of view. The ultrasound transducer is placed in a longitudinal midline plane between the two sacral cornua, and the sacrococcygeal ligament is identified (Fig. 16-7, A ). The sacral hiatus, visualized between the sacral and dorsal sacra, contains the dural sac and cauda equina. Progressive vertebral ossification limits midline neuraxial imaging in children older than 3 months, but a longitudinal paramedian angle may overcome this problem by scanning through a window lateral to the spinous processes (Marhofer et al., 2005). A needle is advanced in-plane via the sacrococcygeal ligament at approximately a 20-degree angle, and a saline test bolus of 0.1 to 0.3 mL/kg is administered to confirm correct needle position (Park et al., 2006). This injection should produce ventral displacement of the dura, and dilation of the caudal epidural space (see Fig. 16-7, B: Go to www.expertconsult.com to view this image online). In the absence of dural displacement, intrathecal or intravascular needle placement should be considered and the needle replaced. The needle may not be visible under real-time guidance if the insonating angle is misaligned, as may occur with paramedian scanning. If the sacral cornua are not palpable, scanning with the probe in a transverse plane starting at the coccyx and moving cephalad will identify these structures as two hyperechoic mounds connected by a hyperechoic sacrococcygeal ligament.
Dosing
For single-shot caudal anesthesia that will not involve repeat dosing, the goal is to provide the appropriate intraoperative level and a prolonged postoperative analgesia. Although formulas have been developed for determining levels for injection of a single-shot caudal, delivery of 1 mL/kg of local anesthetic with epinephrine (1:200,000) will provide thoracic level anesthesia with a duration of 4 to 6 hours depending on the local anesthetic chosen (Armitage, 1979) (Fig. 16-8). Although 1 mL/kg of local anesthetic would never be injected into the epidural space of an adult, the anatomy of the caudal epidural space in children is such that a high volume is needed to fill the loosely packed space and to spread to reach the appropriate dermatomes. The caudal space communicates freely with the perineural spaces of the spinal nerves, which allows a lower concentration of local anesthetic to be effective. Dosing guidelines are in Table 16-3. Although convention suggests that an increased volume of local anesthetic would be required for adequate block and duration of action, one study found that there was no advantage to increasing the volume of local anesthetic to greater than 0.7 mL/kg (Schrock et al., 2003). In children 1 to 6 years of age undergoing inguinal hernia repair, Schrock and associates (2003) compared three groups, all of whom received 0.175% bupivacaine administered at different volumes (0.7 mL/kg, 1 mL/kg, and 1.3 mL/kg). The durations of action as determined by the time of the first postoperative analgesic were similar for all three groups: 4.2, 3.6, and 4.8 hours, respectively. In addition, there were no differences among the groups with regard to first time to void, ambulate, or discharge. However, in another study, Verghese and coworkers (2002) noted that 1 mL/kg of bupivacaine 0.2% was more effective than a smaller volume (0.8 mL/kg) of 0.25% bupivacaine in blocking the peritoneal response of spermatic cord traction during orchiopexy. The quality of postoperative analgesia was similar in the two groups. When keeping the total dosage of local anesthetic consistent, a higher volume is much more effective than a higher concentration. Hong and associates (2009) demonstrated this effect by comparing a high-volume (1.5 mL/kg), low-concentration (0.15%) ropivacaine solution with a low-volume (1 mL/kg), high-concentration (0.225%) ropivacaine solution and found that the higher volume spread to T6 whereas the lower volume spread to T11. Although there were no differences in postoperative pain scores between the groups, the first time to oral pain medication was at 554 minutes for the high-volume group, compared with 363 minutes for the low-volume group, suggesting a longer analgesic duration when overall dosage is kept constant.
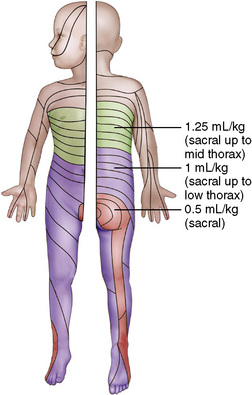
FIGURE 16-8 Dermatomal distribution of different volumes of local anesthetic for single-shot caudal block.
The recommended concentration of bupivacaine for a single-shot caudal is 0.125% to 0.25%, although Gunter and coworkers (1991) in a dose-range study concluded that 0.175% offered the best combination of analgesia and rapid recovery with the least number of side effects.
When performing a single-shot caudal in expremature infants for hernia repair, a combination of agents has been shown to be successful (Bouchut et al., 2001b). A mixture of 0.5 mL/kg of 1% lidocaine, along with 0.5 mL/kg of 0.5% bupivacaine, was used in 25 infants. This combination provided surgical analgesia for 60 minutes. However, one of the 25 infants developed a total spinal block and two children developed postoperative apnea.
Ropivacaine has been evaluated in children for caudal anesthesia and has been found to provide onset and analgesic duration similar to that of bupivacaine (Ivani et al., 1998). In some studies, when compared with bupivacaine, ropivacaine produced less of a motor block at 0.25% and 0.375% concentrations (DaConceicao and Coelho, 1998, 1999). When 0.2% ropivacaine, 0.25% levobupivacaine, and 0.25% bupivacaine were compared in children for caudal anesthesia, postoperative analgesia was not significantly different among the groups, nor was the time for the first postoperative analgesic (Ivani et al., 2002b; Locatelli et al., 2005). The only difference was a slight reduction in the incidence of motor block in the ropivacaine group.
When using 1 mL/kg of 0.2% ropivacaine, free plasma concentrations were well below toxic levels (Lonnqvist et al., 2000). To determine the effective concentration of ropivacaine for single-shot caudal analgesia, Bosenberg and associates (2002b) compared 1 mL/kg of 0.1%, 0.2%, and 0.3% ropivacaine in 110 children aged 4 to 12 years. The median times to first analgesic were 3.3, 4.5, and 4.2 hours in the groups, respectively. Pain scores were significantly higher in the 0.1% group than in the 0.3% group. Motor block was 0%, 13%, and 28% in the 0.1%, 0.2%, and 0.3% groups, respectively. Bosenberg and coworkers concluded that 1 mL/kg of ropivacaine is effective for postoperative pain in children after inguinal surgery, and that the lower concentration of 0.1% is not effective, and the higher concentrations result in a higher rate of motor block.
In other dosing studies with ropivacaine, Koinig and associates (1999) compared 0.75 mL/kg of 0.5% ropivacaine, 0.25% ropivacaine, and 0.25% bupivacaine in children aged 1.5 to 7 years undergoing inguinal hernia repair. The remarkable finding in this study was the duration of analgesia afforded by 0.5% ropivacaine. The duration in the ropivacaine 0.5% group was 1440 minutes, whereas the 0.25% ropivacaine and 0.25% bupivacaine groups were 208 minutes and 220 minutes, respectively.
For neonates and infants up to 12 months, a single dose of 0.2% ropivacaine at 1 mL/kg resulted in plasma levels that were well below toxic dosages and followed a one-compartment open model (Rapp et al., 2004). The mean peak plasma concentration was 0.83 mg/L for total ropivacaine, and 0.042 mg/L for unbound ropivacaine. The time to first analgesic was 3.9 hours.
Levobupivacaine, the S(−) enantiomer of bupivacaine, has also been used clinically as an alternative to bupivacaine. Ivani and coworkers (2002b) compared 1 mL/kg of caudal 0.25% levobupivacaine with 0.2% ropivacaine and 0.25% racemic bupivacaine in children and noted no significant difference in intraoperative or postoperative analgesia among the three groups. In children less than 2 years of age, a dose of 2 mg/kg of 0.25% levobupivacaine had an efficacy similar to that of racemic bupivacaine, and levobupivacaine had a duration of action of 7.3 hours (Taylor et al., 2003). Ivani and associates (2003) noted the optimum concentration of levobupivacaine at 1 mL/kg for a single-shot caudal without any additive was 0.2%. This dosage provided 118 minutes of postoperative analgesia, compared with 60 minutes for 0.125% and 158 minutes for 0.25%. The advantage of using 0.2% instead of 0.25% was the decreased incidence of motor blockade. Dosing guidelines for levobupivacaine are similar to those for bupivacaine (see Table 16-3). The relative potencies of levobupivacaine and ropivacaine have been compared and found to be similar (Ingelmo et al., 2009). At one minimum alveolar concentration (MAC) of sevoflurane, the median effective dose (ED50) for levobupivacaine was 0.069%, and for ropivacaine it was 0.075% in boys aged 1 to 6 years receiving single-shot caudal anesthesia for unilateral groin surgery. The results of the study suggest that the ED95 for the local anesthetics would be 0.2% for levobupivacaine and 0.225% for ropivacaine.
Caudal Additives
One of the disadvantages of single-shot caudal anesthesia is the relatively short duration of postoperative analgesia in children, even when using long-acting local anesthetics. Many agents have been studied in an attempt to find an additive that would prolong the duration of analgesia for single-shot caudal anesthesia. Lonnqvist (2005a) addressed the use of adjuncts to caudal block in an editorial. In particular, the editorial pointed out that there are options such as clonidine and ketamine that enhance postoperative analgesia with acceptable safety profiles, and that anesthetists should adhere to existing literature and evidence-based medicine for the use of additives to the caudal space.
Epinephrine
The use of epinephrine for regional anesthetic techniques in children was discussed earlier. It is recommended that epinephrine be added to single-dose local anesthetics at a dosage of 5 mcg/mL or a concentration of 1:200,000. The hypothetical disadvantage of the use of epinephrine is vasoconstriction and possible cord ischemia from impaired flow to the artery of Adamkiewicz (Cook and Doyle, 1996). Therefore, an epinephrine dosage of 2.5 mcg/mL, or a concentration of 1:400,000 may be used as an additive for central blocks (Flandin-Blety and Barrier, 1995).
Epinephrine will serve as a marker for intravascular injection and decrease systemic absorption of local anesthetic. Additionally, epinephrine may prolong the duration of a regional block. Warner and coworkers (1987) compared children aged 3 months to 17 years receiving single-shot caudal block with 0.5 mL/kg bupivacaine 0.25%. One group received the bupivacaine plain, and for the other group, epinephrine 5 mcg/mL was added to the local anesthetic. Epinephrine prolonged the duration of the caudal block analgesia compared with the blocks that did not include epinephrine. The duration of analgesia decreased with increasing age, with the greatest effect on children less than 5 years of age. Children aged 5 years or less had a mean duration of analgesia 10 to 13 hours longer if epinephrine was in the solution. In children aged 6 to 10 years, epinephrine increased the duration of effect by 2 to 3 hours, whereas in children older than 11 years, epinephrine increased the block by 1 to 2 hours. However, in other studies, epinephrine was not shown to prolong a caudal block with bupivacaine (Fisher et al., 1993; Cook et al., 1995).
Ketamine
Preservative-free ketamine has been described for caudal use in children to prolong a bupivacaine block after hernia surgery. Naguib and associates (1991) compared three groups of children receiving either plain bupivacaine 0.25%, ketamine 0.5 mg/kg, or bupivacaine 0.25% plus ketamine 0.5 mg/kg. The group who received only 0.5 mg/kg ketamine had superior analgesia and longer duration of action than the group who had received plain 0.25% bupivacaine. The ketamine group also had similar analgesia and duration of action to the group who received the combination of ketamine and bupivacaine. No postoperative behavior changes were noted in the ketamine groups. These findings have been confirmed in subsequent studies using 0.5 mg/kg ketamine as an additive to either 0.25% bupivacaine or 0.2% ropivacaine in the caudal space (De Negri et al., 2001a; Weber and Wulf, 2003). Cook and coworkers (1995) studied children aged 1 to 10 years who received caudal anesthesia using 1 mL/kg of 0.25% bupivacaine with the addition of either ketamine 0.5 mg/kg, clonidine 2 mcg/kg, or epinephrine 5 mcg/mL. The ketamine group had a mean duration of analgesia of 12.5 hours compared with 5.8 hours for the clonidine group and 3.2 hours in the epinephrine group. When used alone in the caudal space, ketamine at this dosage of 0.5 mg/kg had a shorter duration of action than bupivacaine 0.25% with 1:200,000 epinephrine, but ketamine 1 mg/kg provided surgical and postoperative analgesia that was equivalent to that of bupivacaine (Marhofer et al., 2000).
Another study using simply ketamine for caudal analgesia without local anesthetic compared a group who received ketamine 1 mg/kg with two other groups who received, in addition to ketamine, clonidine 1 or 2 mcg/kg (Hager et al., 2002). The ketamine group had a mean duration of postoperative analgesia of 13.3 hours. When clonidine 1 mcg/kg or 2 mcg/kg was added to the ketamine, the mean durations were 22.7 hours and 21.8 hours, respectively. This particular study prompted a letter to the editor by Eisenach and Yaksh (2003) that stressed concern over the performance and publications of studies that subjected healthy children to agents that have not had adequate preclinical toxicity studies. Eisenach and Yaksh further discussed the potential risks of neurotoxicity using S(+)-ketamine in the epidural space without significant benefits to the otherwise healthy child. The response to this letter from the authors of the study defended their position with a number of papers on ketamine in the epidural space (Marhofer and Semsroth, 2003). The issue remains controversial, as pointed out in a systematic review of nonopioid additives by Ansermino and associates (2003). This review summarized the findings of randomized control trials performed on children using nonopioid additives in the caudal space and concluded that although clonidine, ketamine, and midazolam increase the duration of analgesia, the potential for neurotoxicity remains a concern with ketamine and midazolam. They also concluded that the routine use of nonopioid adjuvants for elective outpatient surgery had not been shown to improve patient outcome.
Clonidine
Clonidine, an α2-adrenergic agonist, at 1 to 2 mcg/kg, has been used with success and may result in an additional 4 to 6 hours of analgesia when combined with bupivacaine (Jamali et al., 1994; Lee and Rubin, 1994; Constant et al., 1998; Tripi et al., 2005). Clonidine at 1 mcg/kg, when combined with bupivacaine for caudal block, provided a mean duration of 987 minutes compared with 377 minutes in a group of children who received bupivacaine with epinephrine, versus 460 minutes in children who received bupivacaine plain (Jamali et al., 1994). Ivani et al. (2000) demonstrated that 0.1% ropivacaine plus clonidine 2 mcg/mL provided superior analgesic quality to a caudal block compared with 0.2% ropivacaine without clonidine. The true mechanism of analgesic action of clonidine remains unknown, but there is evidence that it has both central and peripheral sites of action (Ivani et al., 2002a). Although clonidine may cause some sedation, particularly at the higher dosages, and although the sedation has not been considered clinically significant in controlled studies, caudal clonidine has been implicated in case reports as a cause of apnea in neonates (Breschan et al., 1999; Bouchut et al., 2001a).
De Negri and coworkers (2001a) compared ketamine with clonidine to determine which agent would more effectively prolong a ropivacaine caudal anesthetic. Children 1 to 5 years of age received 0.2% ropivacaine 2 mg/kg, ropivacaine plus clonidine 2 mcg/kg, or ropivacaine plus ketamine 0.5 mg/kg for caudal anesthesia. Postoperative analgesia was significantly longer in the ropivacaine with ketamine group (701 minutes) than in the ropivacaine with clonidine group (492 minutes) and the ropivacaine plain group (291 minutes). There were no clinically significant side effects in any of the groups. These findings were similar to the study by Cook and associates (1995) that compared clonidine 2 mcg/kg with ketamine 0.5 mg/kg as additives to 0.25% bupivacaine caudal anesthesia.
When clonidine is directly compared with opioids, its use offers comparable analgesia with fewer side effects. To illustrate this, Vetter and coworkers (2007) used single-shot caudal anesthesia for pediatric patients undergoing ureteral reimplantation. Each child received 1 mL/kg of 0.25% ropivacaine with epinephrine with a caudal additive. The three additive groups were 2 mcg/kg clonidine, 10 mcg/kg hydromorphone, or 50 mcg/kg morphine. Caudal morphine afforded the greatest duration of analgesia, although there was not a statistically significant difference overall. Clonidine appeared to be superior to either caudal morphine or hydromorphone because of its similar analgesic profile and lower incidence of postoperative nausea and vomiting.
Tramadol
Tramadol, an analgesic that acts centrally at opioid receptors, has been compared with bupivacaine alone and a tramadol-bupivacaine combination for caudal analgesia (Prosser et al., 1997; Batra et al., 1999; Gunduz et al., 2001). When 1 mg/kg of tramadol was added to bupivacaine, patients had lower pain scores and longer durations of analgesia than with bupivacaine alone (Batra et al., 1999). When 2 mg/kg of tramadol was added to bupivacaine, some children had sedative effects, but this was not considered to be clinically significant (Gunduz et al., 2001). Caudal tramadol 2 mg/kg provides reliable postoperative analgesia similar to caudal morphine 30 mcg/kg for children undergoing herniorrhaphy (Ozcengiz et al., 2001).
Neostigmine
The use of neostigmine in the epidural space in children is not yet common practice but shows promise. Its action may be attributed to either direct action on the spinal cord via inhibition of the breakdown of acetylcholine in the dorsal horn, or by peripheral antinociceptive effect (Shafer et al., 1998; Yang et al., 1998). A study in children compared three groups to determine the effectiveness of neostigmine (2 mcg/kg) as a caudal analgesic for hypospadias repair, either alone or in combination with bupivacaine (Abdulatif and El-Sanabary, 2002). The groups received 1 mL/kg of either 0.25% bupivacaine plain, bupivacaine with neostigmine 2 mcg/kg, or neostigmine plain. The combination of bupivacaine and neostigmine provided superior analgesia to either of the other two groups, with a mean duration of 22.8 hours compared with 8.1 hours in the bupivacaine plain group and 5.2 hours in the neostigmine plain group. This prolonged duration when compared with plain local anesthetic has been demonstrated in other studies as well (Mahajan et al., 2004; Kumar et al., 2005). When compared in boys who received levobupivacaine caudal anesthetics for urologic surgery, the addition of neostigmine 2 mcg/kg compared with 4 mcg/kg provided similar duration of postoperative analgesia (15 hours and nearly 20 hours, respectively) and total analgesic consumption (Karaaslan et al., 2009). The higher dosage offered no advantage, and either dosage improved analgesia beyond levobupivacaine plain.
The combination of bupivacaine with neostigmine has been compared not only with plain bupivacaine, but also with other combinations of additives for single-shot caudal anesthetics (Kumar et al., 2005). Duration of analgesia with the bupivacaine-neostigmine group (19.6 hours) was similar to that of a group with bupivacaine and midazolam (16.8 hours), and longer than the bupivacaine plain group (7.6 hours) and bupivacaine-ketamine group (11.6 hours). The dosage of neostigmine used as an additive in this study was 2 mcg/kg. At dosages of 10 mcg/kg and higher, there may be an increased incidence of postoperative nausea and vomiting, which would make this an unattractive alternative as an adjunct to epidural anesthesia; however, no study has attempted to define whether antiemetics will decrease the incidence of nausea and vomiting in this population (Lonnqvist, 2005a; Almenrader and Passariello, 2006).
Note that neostigmine, which typically contains methylparabens and propylparabens as preservatives, should be used only in a preservative-free form when injected into the epidural space. Investigation as to its effect on neural structures is ongoing, and skepticism remains as to whether neostigmine is a viable option for pediatric caudal use (Lonnqvist, 2005a).
Opioids
Opioids may be used to improve the quality and duration of a block, but there are advantages and disadvantages to spinal axis opioids (Lonnqvist et al., 2002). The major disadvantage of opioid additives is the risk for respiratory depression. In children less than 1 year of age, the risk for respiratory depression from caudal morphine is significantly higher than in children older than 1 year (Valley and Bailey, 1991). In this study of 138 children who had received 70 mcg/kg of caudal morphine, the incidence of clinically significant respiratory depression was 8%. Ten of the 11 children with respiratory depression were less than 1 year of age and weighed less than 9 kg. Seven of the 11 patients also received intravenous opioids. All episodes of respiratory depression occurred within 12 hours of the caudal morphine injection. Spinal axis opioids, because of the risk for delayed respiratory depression, are contraindicated in ambulatory surgical patients. Patients under 1 year of age and patients receiving supplemental intravenous opioids should be carefully monitored postoperatively.
Another disadvantage of neuraxial opioids is the increased incidence of postoperative pruritus, nausea, and vomiting. Although fentanyl 1 mcg/kg may prolong a caudal block, the incidence of pruritus and vomiting also increase (Constant et al., 1998). In one study, the investigation was actually halted because of the unacceptable incidence of postoperative vomiting in a group of children who had received caudal buprenorphine (Khan et al., 2002). Urinary retention is a side effect of caudal morphine and required urinary catheterization in 30% of children in one study using 70 mcg/kg morphine (Irving et al., 1993). Another reason to avoid neuraxial opioids is the availability of alternative additives. As previously discussed, additives such as clonidine and ketamine have been used as adjuncts for central blockade with success and result in fewer side effects compared with opioids.
Despite the pitfalls of using opioids as a component of central blockade, the practice continues because of the relative advantages these agents offer. Preservative-free morphine more than doubles the duration of a single-shot bupivacaine caudal (Krane et al., 1987). In a study of children aged 1 to 16 years, there was a slightly greater incidence of urinary retention, pruritus, and nausea in the group who received caudal morphine, but there was no evidence of delayed respiratory depression even at dosages of 100 mcg/kg in this age group. A caudal morphine dosage of 30 mcg/kg has been recommended for children to provide the advantage of increased analgesic duration with decreased incidence of side effects, particularly respiratory depression (Krane et al., 1989). In children who were undergoing open-heart procedures, a dosage of 70 mcg/kg morphine provided lower pain scores and decreased incidence of atelectatic changes on radiograph compared with a control group who had not received caudal morphine (Rosen and Rosen, 1989). Plasma levels of morphine given via the caudal route peak at 21 ± 4.8 ng/mL approximately 10 minutes after injection (Wolf et al., 1991). These levels are lower than those associated with systemic administration of morphine.
Lipophilic opioids do not offer the same risk for respiratory depression as hydrophilic agents. The disadvantage of the lipophilic agents is the shorter duration of postoperative analgesia than what is provided by morphine, and there may actually be no benefit to the addition of fentanyl to local anesthetic for a single-shot caudal. In most reports, caudal fentanyl 1 mcg/kg has not been shown to increase the duration of analgesia produced by 0.125% bupivacaine, 0.25% bupivacaine, 2% lidocaine, or 0.2% ropivacaine (Jones et al., 1990; Campbell et al., 1992; Joshi et al., 1999; Baris et al., 2003; Kawaraguchi et al., 2006). In contrast, Constant and associates (1998) demonstrated prolongation of analgesia in a study in which fentanyl 1 mcg/kg was added to a mixture of bupivacaine and lidocaine in children aged 6 to 108 months. The mean duration of analgesia in the group was 253 ± 105 minutes compared with 174 ± 29 minutes in the control group without fentanyl. Vomiting occurred in 4 of 15 children who had extradural fentanyl, and in none of the 14 children who had not received fentanyl.
Fentanyl and morphine were compared for efficacy and side effects in children aged 1 to 16 years (Lejus et al., 1994). The children all received a preincision epidural dosage of 0.5% bupivacaine, 0.75 mL/kg, and were then divided into two groups. The morphine group received a preoperative bolus of epidural morphine 75 mcg/kg and the same morphine bolus dose 24 hours later. The fentanyl group received 2 mcg/kg before incision, followed by a continuous infusion of 5 mcg/kg per day. The group who received the fentanyl infusion had comparable analgesia with the morphine group with less pruritus (20% versus 53%), and less nausea and vomiting (0% versus 33%).
Complications
Complications from caudal anesthesia include risks during the performance of the block, risks from injection of local anesthetic, and side effects from the agents used. During the performance of a caudal block, the needle could be accidentally placed into the intravascular space, the subarachnoid space, or the sacral marrow. The incidence of intravascular injection should be decreased with the use of a short-beveled needle, and a test dose should reveal whether the needle is intravascular or in the vascular marrow (Dalens and Hasnaoui, 1989). The detection of a subarachnoid injection may be difficult if CSF is not clearly seen on aspiration prior to local anesthetic injection. In the safety study of Giaufre and coworkers (1996), inadvertent dural puncture was the most frequent complication, but it was still uncommon. In the event the subarachnoid space has been entered, it is possible to reintroduce a needle for a caudal anesthetic; however, the agent should be injected very slowly to avoid the possible migration of local anesthetic solution into the subdural space through any previous puncture sites.
Children differ from adults in that hypotension secondary to centrally delivered local anesthetic is not generally a significant side effect from caudal or neuraxial regional anesthesia. Even without intravascular volume loading before the administration of a central blockade, hypotension is typically not observed in children less than 5 years of age (Dohi et al., 1979; Dohi and Seino, 1986). This may be because of the immature sympathetic nervous system, or because the lower extremities, in proportion to overall body size, do not provide a significant volume for venous pooling.
Studies have been performed in children to investigate the hemodynamic response to caudal analgesia. Doppler studies to investigate hemodynamic changes have demonstrated that cardiac output does not change during caudal anesthesia in infants (Payen et al., 1987). A study to investigate pulmonary Doppler flow revealed that the pulmonary flow velocity changes during caudal anesthesia, presumably secondary to an increase in pulmonary artery resistance (Ozasa et al., 2002). Because the change may have reflected local anesthetic–induced vasoconstriction, the authors concluded that caudal epidural anesthesia is not recommended in children with pulmonary hypertension.
Urinary retention, although a concern, is not considered a frequent side effect in single-shot caudal anesthetics. Fisher and associates (1993) reviewed the postoperative voiding interval in children who received either bupivacaine caudal anesthesia with and without epinephrine, or ilioinguinal hypogastric nerve blocks. They noted no significant difference in the time to micturition among these groups. Although the range of times to first micturition varied widely, from 25 to 630 minutes, no children required any intervention for urinary retention.
Continuous Caudal Catheters
Intraoperatively, an indwelling catheter may be placed in the caudal epidural space. These catheters allow additional dosing of the local anesthetic agents at the end of the procedure or in the recovery area prior to catheter removal. A repeat or second dose of local anesthetic can be safely administered 90 to 120 minutes after the initial dose as long as the maximal allowable dosage is not exceeded in that time period. In addition, continuous caudal block may be used as an alternative to spinal anesthesia in the expremature infant who is at risk for postoperative apnea while undergoing inguinal hernia repair. This technique has been used with success with and without a concomitant general anesthetic (Henderson et al., 1993; Peutrell and Hughes, 1993; Tobias et al., 1996).
The presence of a caudal catheter allows continued postoperative pain management in children. Continuous infusions, although commonly used because of their ability to provide complete postoperative analgesia, have come under scrutiny because of a lack of prospective outcome studies that demonstrate benefit (Chalkiadis, 2003). Audits of postoperative infusions have demonstrated that 17% to 22% of patients require premature termination of the infusions. In 67% of these patients, the termination was because of an unacceptable rate of side effects or complication (Wilson and Lloyd-Thomas, 1993; Wood et al., 1994). This high rate leads one to question the benefit of neuraxial analgesia over intravenous analgesia (Chalkiadis, 2003). This side effect may be greater for lipophilic infused epidural opioids (e.g., fentanyl), because the catheter tip must be positioned at the interspace corresponding to the dermatomes of the surgical procedure. Another issue that argues against placing and maintaining a continuous epidural catheter includes the dissatisfaction of having a motor block in children. There may also be an increased incidence of postoperative urinary retention and pruritus in children who receive infusions of neuraxial opioids (Lloyd-Thomas and Howard, 1994). Although these side effects are not necessarily dangerous, they do result in increased workload for medical staff, additional medications, and child and parent distress. More serious issues with continuous caudal infusions include the rare risks of epidural hematomas, epidural infection and abscesses, and respiratory depression from central opioids. In addition, there is the cost of the epidural catheter kits, the operative time to insert the caudal and urinary catheters, and the postoperative costs for pharmacy, nursing, on-call staff, and a pain service. Therefore, it has been suggested that continuous infusions be reserved for those children who would truly receive a direct benefit.
Technique
The anatomy of and entry into the caudal space for placing a continuous catheter via the caudal route have already been described. In threading a caudal catheter, the space should first be dilated with a push of preservative-free normal saline. The catheter is then threaded through the needle until the tip of the catheter reaches the estimated desired level. The caudal approach to placing a lumbar or thoracic catheter in infants was described by Bosenberg and coworkers (1988). Because of the loosely packed fat in the epidural space, a catheter should be advanced easily. If resistance is felt, it is most likely because the catheter has coiled or doubled back in the epidural space. In older children who may have more densely packed epidural fat, the use of a catheter with a stylet may increase the success rate (Gunter and Eng, 1992). In addition to using a catheter with a stylet, other means to improve success are dilating the space with preservative-free normal saline prior to catheter placement, flexing the hips to straighten the spine, and using fluoroscopy to ensure proper catheter tip position. If an angiocatheter has been used for insertion of the catheter, the catheter may be withdrawn, twisted, and advanced through the plastic introducer until the desired position as determined by radiography is obtained. Catheters should not be withdrawn through metal needles because of the risk of shearing the catheter into the epidural space.
Success in placing a thoracic catheter via the caudal route is variable. In a study of 86 infants who had caudal placement of a thoracic catheter that was confirmed by x-rays, the positions of 28 of these catheters were considered to be inadequate (Valairucha et al., 2002). Of the 28, 10 were determined to be in the high thoracic or cervical regions and were able to be pulled back, 17 were coiled in the lumbosacral area, and one was outside the epidural space in the presacral area. Thus, radiographic confirmation of catheter tip position for thoracic catheters that are threaded from the caudal route is essential, and 0.5 mL Omnipaque 180 (Amersham Health, Princeton, NJ) may be used for this indication. Rather than using radiographic evidence to help determine proper placement of the catheter tip, Tsui and associates (1998) described the use of low-current electrical stimulation during advancement of the catheter, resulting in muscle twitching at the corresponding dermatome. Although the immediate feedback on location of catheter tip helps to identify desired placement, it is unclear whether this method offers advantages over or higher success rate than conventional methods such as cutaneous landmarks and simple catheter measurement (Goobie et al., 2003).
Technique with ultrasound
The advancement of the epidural catheter from the sacral hiatus and tip positioning under the ultrasound guidance has been described (Chawathe et al., 2003; Roberts and Galvez, 2005). This technique avoids patient exposure to radiation and contrast injection and is independent of the neuromuscular blockade or local anesthetics use (Asato and Goto, 1996; Tsui et al., 1998; Valairucha et al., 2002). The catheter is introduced via a previously positioned caudal cannula and is advanced while being sonographically imaged. The catheter is followed cephalad with the ultrasound probe in the longitudinal paramedian plane by direct visualization, which is easiest with styletted catheters, or its level is inferred by the anterior dural displacement with saline injection. Scanning the neuraxial structures in the transverse plane allows direct catheter visualization and is less dependent on bony ossification (Fig. 16-9).
Dosing
Continuous caudal catheters that have their tip in the sacral or lower lumbar areas will be dosed differently from those that have been threaded to the low to mid thoracic levels. Suggestions for dosing are in Table 16-3. Continuous caudal catheters require large volumes of local anesthetic to fill the loose caudal epidural space and to spread the anesthetic cephalad to the desired dermatomal level. It is important to refer to the maximal allowable dosing recommendations so that these rates are not exceeded in an attempt to “drive up” the epidural block from the caudal space (see Table 16-2). Bupivacaine, ropivacaine, and levobupivacaine may be used for continuous caudal anesthesia, with or without additives. It is possible to improve the analgesia of a continuous caudal catheter by adding a hydrophilic opioid such as preservative-free morphine or hydromorphone to the infusion.
In addition to a continuous infusion, children can also benefit from patient-controlled epidural analgesia, which allows the patient to receive analgesia at the press of a button. Its popularity in children began in the late 1980s with intravenous opioids and has expanded to local anesthetic infusions for a variety of blocks. In a study of children who had undergone a total of 132 procedures and who used patient-controlled epidural analgesia postoperatively, more than 90% had satisfactory analgesia with no significant adverse effects (Birmingham et al., 2003). This study confirmed that children as young as 5 years have the cognitive ability to use of patient-controlled analgesia.
Complications
Complications of continuous caudal infusions are typically secondary to the agent that is being delivered. The risk for local anesthetic toxicity has been discussed, and there is ample evidence that the use of bupivacaine at dosages over 0.2 mg/kg per hour in infants and 0.4 mg/kg per hour in children older than 6 months may lead to neurotoxicity or cardiovascular collapse (Agarwal et al., 1992; Berde, 1992, 1993; McCloskey et al., 1992).
Continuous indwelling caudal catheters would seem to offer a risk for fecal contamination and infection. When caudal catheters have been compared with epidural catheters, caudal catheters were found to have a greater incidence of colonization of bacteria (20% versus 4%), with Staphylococcus epidermidis being responsible for the vast majority of colonizations (McNeely et al., 1997b). In addition, four of the nine caudal catheter tips that were colonized involved gram-negative bacteria. Caudal catheter tip colonization was not predicted by duration of catheterization, skin inflammation, or dressing contamination. None of the children developed signs of epidural infection, either in the hospital or during the 3-month follow-up. Another study demonstrated the absence of epidural abscess or clinically significant infection from indwelling catheters for postoperative pain (Strafford et al., 1995). An option for children who are to have prolonged analgesia provided by a continuous caudal or epidural catheter is to tunnel the catheter under the skin (Aram et al., 2001). This should allow a longer-term infusion of agents by decreasing the chance of catheter dislodgment and protecting against colonization and infection.
Epidural Anesthesia
Epidural anesthesia is commonly used for procedures that involve surgery of the mid to upper abdomen and thorax that are less amenable to a continuous caudal anesthetic. Children who have procedures that have higher morbidity rates such as Nissen fundoplication may benefit from perioperative epidural analgesia (McNeely et al., 1997a; Wilson et al., 2001). Postoperative complications and hospital stay may be reduced with the use of an epidural for this procedure compared with opioid analgesia alone (Miller et al., 2002). In addition, the use of epidural anesthesia is associated with a significantly reduced stress response to surgery in children, as determined by lower cortisol level and plasma epinephrine levels (Murat et al., 1988; Wolf et al., 1993).
Although lumbar epidurals are commonly employed, thoracic epidurals should be performed only by practitioners who are experienced in their use and should be reserved for children with pulmonary disease or who are to undergo a surgical procedure in the thoracic or upper abdominal area that is associated with significant postoperative pain. See related video online at www.expertconsult.com. As discussed, under continuous caudal anesthesia, a catheter may be threaded from the caudal space cephalad to the thoracic region to provide more site-specific analgesia without the risks associated with placing a needle in the thoracic spine. However, threaded catheters from the caudal space are frequently positioned poorly. In older children, a thoracic epidural inserted between T4 and T8 should attenuate the stress response associated with thoracic surgery and provide optimal postoperative analgesia (Hammer, 2001). Thoracic epidural catheters that are placed intraoperatively by the surgeon prior to wound closure for anterior spinal fusion and instrumentation for scoliosis have been shown to be safe and effective (Lowry et al., 2001).
Anatomy
The epidural space in children is divided into the sacral, lumbar, thoracic, and cervical levels. The caudal block enters at the sacral level. A lumbar epidural needle or catheter is typically placed at the L3-4 interspace, which in older children is found at the center of a line drawn between the two iliac crests. Although these landmarks are accurate in older children, the intercrestal line may actually cross the L5-S1 interspace in neonates, and the L4-5 interspace in infants up to a year of age because of the lag of the growth of the spinal cord (see Fig. 16-3). Because of the developmental changes that occur with the spinal cord and dural sac positions, placing an epidural catheter below the level of the intercrestal line (e.g., the caudal area) may decrease the risk of a wet tap in a neonate or young infant.
Epidural pressures differ depending on the age of the patient. Infants have narrow epidural spaces and are more likely to exhibit leak around an epidural catheter from a backflow of solution if injected too quickly (Vas et al., 2001). Even at slow injection rates, epidural pressures in infants are higher than in adults.
The depth to the epidural space at the L2-3 level is approximately 10 mm at birth, and this depth increases linearly with age. The approximate expected distance from the skin to the epidural space in children aged 6 months to 10 years is approximately 1 mm/kg body weight (Bosenberg, 1995). See Figure 16-6 for approximate depths from the skin to the lumbar and thoracic epidural spaces at different ages.
Technique
With the child in the lateral position and knees and hips flexed, the line that joins the two iliac crests crosses the body of S1 in neonates, L5 in infants, L4-5 in young children, and L4 in older children and adolescents (Busoni and Messeri, 1989). After sterile preparation and draping, the needle should be placed in the midline between the spinous processes that are closest to the line that crosses the iliac crests. If performing a lumbar epidural puncture, the spinous processes require the needle to be directed slightly cephalad, but mostly perpendicular to the skin. The needle is advanced slowly, with one hand firmly against the child’s back and holding the portion of the needle that is entering the child’s skin. This is to avoid any inadvertent and rapid advancement of the needle. The needle will pass through skin, subcutaneous tissue, supraspinous ligament, interspinous ligament, and then ligamentum flavum before entering the epidural space (Fig. 16-10). Because of the risk for air embolus, a continuous-loss-of-resistance technique using saline rather than air to confirm the epidural space is the recommended approach (Sethna and Berde, 1993). The ability to feel entry into the ligamentum flavum and the loss of resistance as the epidural space is entered is subtler in infants than in adults. Once the catheter is threaded into the epidural space and there is negative aspiration for CSF or blood, the local anesthetic with epinephrine should be injected as an initial test dose before delivering the planned dose of local anesthetic. Compared with adults, epidural pressures differ in children, particularly in infants (Vas et al., 2001). Based on the relationship between the volume and rate of injection of local anesthetics in infants and the epidural pressure, a slow rate of injection is used, perhaps 0.5 mL/min.
Technique with Ultrasound
A preprocedural ultrasound scan of the vertebral column facilitates optimal site selection for the lumbar or thoracic epidural catheter insertion and allows measurement of the depth of the ligamentum flavum, thus estimating the depth at which loss of resistance will occur (Rapp et al., 2005; Willschke et al., 2007) (see Fig. 16-9). The longitudinal median view offers a slightly better correlation with actual depth than the transverse view by ultrasound (Kil et al., 2007). Real-time ultrasound-guided epidural placement has resulted in reduction in the catheter placement time and the frequency of bony contacts (Willschke et al., 2006c). This approach is challenging and requires two anesthesiologists experienced in the ultrasound-guided procedures and neonatal epidural techniques. To simplify the technique, perform a scout ultrasound scan to determine the depth of the epidural space, and then confirm catheter tip position by saline injection test once threading through the needle is completed.
Dosing
Epidural catheters are typically placed so that their use can be continued in the postoperative period. Because the tip of the epidural catheter should be in close proximity to the level of surgery, and because the lumbar and thoracic epidural spaces are more compact than the caudal space, the initial bolus of local anesthetic required for lumbar and thoracic epidurals is smaller (0.3 to 0.5 mL/kg) than the volumes required for a caudal injection. Approximately 90 minutes after the initial bolus, a continuous infusion may be initiated with care to stay within the maximal allowable dose (see Table 16-2). Recommendations for epidural dosing are in Table 16-3.
When using 0.25% bupivacaine at a rate of 0.08 mL/kg per hour in the lumbar epidural space in children aged 11 months to 15 years, Desparmet and coworkers (1987) demonstrated that the terminal half-life ranges from 164 to 270 minutes, and total body clearance is similar to that after single caudal injection. Larsson and associates (1994) studied bupivacaine infusions in 12 infants. In infants, bupivacaine infusions at rates of 0.5 to 0.83 mg/kg per hour for 12 hours resulted in marked increases in plasma bupivacaine concentrations. Larsson also noted possible toxic reactions in three of the 12 infants and suggested these infusion rates were excessive for this age group.
The pharmacokinetics of epidural ropivacaine were assessed after a single injection of 1.7 mg/kg via a lumbar epidural catheter (McCann et al., 2001). The median peak plasma concentrations of ropivacaine were 610 mcg/L in infants aged 3 to 11 months, and 640 mcg/L in children aged 12 to 48 months. In both groups of children, the median peak plasma concentration was reached in 60 minutes. The calculated clearance of ropivacaine was 4.26 mL/kg per minute in infants and 6.15 mL/kg per minute in older children. No ropivacaine toxicity was observed in either group. When ropivacaine 0.2% at 0.4 mg/kg per hour was delivered as a continuous lumbar or epidural infusion for 36 to 96 hours, Hansen and coworkers (2000) noted no clinically significant side effects in 18 pediatric patients aged 4 months to 7 years. In this study, the volume of distribution of epidural ropivacaine was 3.1 L/kg, total clearance was 8.5 mL/kg per minute, and the elimination half-life was 4.9 hours (see Table 16-1). In infants and neonates who received 48 to 72 hours of continuous ropivacaine epidural infusion, Bosenberg and associates (2005) found that plasma concentrations of unbound ropivacaine were not influenced by the duration of the infusion. Although the levels of unbound ropivacaine were higher in the neonates than in the infants, all were below the level of 0.35 mg/L, the threshold that is considered toxic in adults.
Epidural Additives
The advantage of adding clonidine to single-shot caudal blocks has been demonstrated, but there are also advantages to its use for continuous epidural analgesia. In a study of 60 children who received a low lumbar epidural catheter for hypospadias repair, a postoperative infusion of ropivacaine alone was compared with ropivacaine with varying dosages of clonidine. De Negri and coworkers (2001b) evaluated plain ropivacaine 0.1% at 0.2 mg/kg per hour, and ropivacaine 0.08% at 0.16 mg/kg per hour, plus either clonidine 0.04 mcg/kg per hour, 0.08 mcg/kg per hour, or 0.12 mcg/kg per hour. The children who received the ropivacaine with the higher clonidine dosages in the 0.08 to 0.12 mcg/kg per hour range had improved pain scores, longer time to first analgesic, and reduced total supplemental analgesics without increased sedation or other side effects.
The use of neuraxial opioids was discussed (see Caudal Analgesia, earlier). In a study designed to assess the efficacy of preoperative epidural morphine, 21 children were randomized to either a group that received 30 mcg/kg epidural morphine after induction of general anesthesia but prior to surgical incision, or to a group that did not receive an epidural injection (Kiffer et al., 2001). Both groups were given intraoperative infusions of sufentanil and postoperative patient-controlled analgesia with morphine. The group that had received preoperative epidural morphine, in comparison with the control group without epidural morphine, had lower pain scores and decreased total analgesic requirements postoperatively without increased side effects.
Epinephrine is a commonly used additive for single-shot epidural blocks because of its ability to decrease systemic absorption and perhaps prolong the block while adding an additional level of safety. For continuous infusions, these benefits must be weighed against the theoretical risks of vasoconstriction of spinal vessels. Kokki and coworkers (2002) compared a combination of ropivacaine and sufentanil with and without epinephrine 2 mcg/mL in a population of children. The children who had epinephrine in their postoperative epidural infusions had significantly lower infusion requirements and fewer side effects. In adults, the addition of epinephrine to a ropivacaine epidural infusion at 5 mcg/mL reduces the early systemic plasma concentrations of ropivacaine, and thereby may decrease the risk for toxicity from systemic absorption (Lee et al., 2002).
Complications
As discussed for caudal catheters, the risk for infection is very low. Although there have been case reports of epidural abscess, short-term epidural catheterization for postoperative courses do not appear to have the same risk for infection as those placed for chronic pain (Strafford et al., 1995). In 1620 children over a 6-year period who received epidural catheters, there were no infections or epidural abscesses in catheters that had been placed for postoperative pain management. There was only one epidural abscess found in this study, and that was in an immunosuppressed child with terminal malignancy who had Candida colonization of the epidural space that had been invaded by tumor and had an indwelling catheter for control of pain from her malignancy. When catheters have been removed and cultured, the incidence of catheter tip colonization in epidural catheters is 4%, lower than that found in caudal catheters (20%) (McNeely et al., 1997b).
A systematic review and pooled analysis of studies on the risk for infection of long-term epidural catheters estimated that one person in 35 may develop an epidural catheter infection (Ruppen et al., 2007). The populations reviewed were primarily adult cancer patients, and those who developed infections had catheters present for an average duration of 74 days. This population is certainly in a separate risk group from that of the typical pediatric surgical patient.
Peripheral nerve blocks
The use of pediatric peripheral nerve blocks has seen the largest growth in practice and in the literature (Rochette et al., 2007). The goal of placing peripheral nerve blocks is to specifically target analgesia to the location of the surgery so that side effects are kept to a minimum (Ross et al., 2000). The safety of performing such blocks has been established, and it has been recommended by ADARPEF that peripheral blocks be used in place of central blocks when appropriate (Giaufre et al., 1996). In their safety study of regional anesthetics in children, there were no complications in the 9396 children who received peripheral nerve blocks or local anesthesia. Despite these findings, peripheral nerve blocks were often underused in children, probably related to inexperience and the perception that they may be difficult to perform or be hazardous. The use of ultrasound has changed the perception of safety and been responsible for a growth in literature on peripheral nerve blocks in children.
Success of placing peripheral nerve blocks is often a function of knowledge of the anatomy, and use of the appropriate equipment (Sethna and Berde, 1992). Insulated needles with a nerve stimulator have been used for peripheral nerve blockade in past years because the field of current is localized to the needle’s tip. Unsheathed needles may be less expensive and result in successful blocks, but the current is distributed not only to the needle’s tip but also along the shaft of the needle. Thus, the required stimulatory current is greater (up to 2 mA) (Bosenberg, 1995). For upper extremity nerve blocks, a 1- or 2-inch insulated needle will suffice. Lower extremity blocks may require the use of longer needles, particularly for sciatic blocks in adolescents. Another practical consideration in performing a peripheral nerve block with a nerve stimulator is that administration of neuromuscular blockers will abolish the ability to elicit muscle stimulation with a peripheral nerve stimulator. The peripheral nerve stimulator should be capable of delivering 0.1 to greater than 1.5 mA and be set to 2 Hz. The positive electrode of the nerve stimulator should be placed at least 10 cm from the nerve to be blocked and preferentially on the opposite limb. To locate a nerve or plexus, begin with the nerve stimulator set at 1 to 1.2 mA, and advance the needle until the desired motor response is achieved. The voltage may then be decreased. When the voltage is decreased to less than 0.5 mA, the motor response should be diminished but still present. One may need to make further fine adjustments in the needle’s position in order to continue muscle stimulation of the appropriate muscle group at the lower voltage. However, an increase in voltage may be required to relocate the nerve if muscle stimulation is completely lost. Once the nerve stimulator’s voltage is less than 0.5 mA and slight muscle stimulation results, the local anesthetic is injected. If the needle is in correct position, the muscle stimulation should cease immediately. If there is intense muscle stimulation with 0.2 mA, the possibility of intraneural needle placement must be considered. Consequently, the needle should be withdrawn and carefully readvanced. In anesthetized children, the placement of a needle into a nerve would not be detected, so this warning sign of intense muscle stimulation at lower voltage is significant. In animal studies, motor response to less than 0.2 mA occurred only when the needle tip was intraneural (Tsai et al., 2008). Unfortunately, motor response could also be absent with intraneural needle placement at currents up to 1.7 mA.
The ultrasonographic identification of peripheral nerves requires practice. Nerves come in many shapes depending on their anatomic location; however, with systematic examination, patterns emerge. Nerve roots and proximal segments have a hypoechoic core surrounded by a hyperechoic ring. Because nerve roots and trunks are clinically visualized only in the performance of the interscalene, parascalene, and supraclavicular blocks, it is generalized that nerves are dark above the clavicle. Peripheral nerves are hyperechoic with fascicular echotexture and exhibit anisotropy, which is a change in echogenicity depending on imaging angle (Crass et al., 1988; Grechenig et al., 2000). Directing the insonation angle perpendicular to the direction of the nerve fiber will optimize nerve appearance.
Although the practice has been replaced by ultrasound, surface nerve mapping was once recommended for use in children to improve the ability to locate a peripheral nerve or plexus (Bosenberg et al., 2002a). Nerve surface mapping is performed by setting the nerve stimulator at a frequency of 1 to 2 Hz and the current between 3 to 4 mA. The positive electrode should be at least 10 cm from the nerve to be mapped, and the negative electrode or alligator clamp that would normally be attached to the block needle is instead pressed against the skin at right angles across the suspected path of the nerve. Once a point of maximal motor response is found, a mark may be placed to indicate where the insulated needle may be inserted for regional blockade.
Dosing of peripheral nerve blocks will be discussed with regard to the individual block. Regardless of the local anesthetic delivered, the addition of epinephrine to the solution should provide additional safety when performing the block. Not only does the addition of epinephrine to the local anesthetic solution have the potential to serve as a marker for intravascular injection, it may also decrease overall absorption of the local anesthetic. In a study of 20 children who were to undergo unilateral surgery of the thigh, patients were administered a fascia iliaca block using 2 mg/kg bupivacaine either with or without epinephrine 5 mcg/mL (Doyle et al., 1997). The median maximal plasma bupivacaine concentration in the group without epinephrine was 1.1 mcg/mL, compared with 0.3 mcg/mL in the group of patients for whom epinephrine was added to the bupivacaine. Not only did the epinephrine group have a significantly lower peak plasma level, but the onset time to peak plasma concentration was more gradual for the group with epinephrine.
There is little information as to the use of adjuncts to local anesthetics in pediatric peripheral nerve blocks. In a database review of 435 patients at the Children’s Hospital of Philadelphia, the use of clonidine 1 mcg/kg (up to 100 mcg) for peripheral nerve blockade resulted in a longer duration of sensory block of 17.2 hours versus 13.2 hours in the group who received either bupivacaine or ropivacaine with clonidine, and a prolonged motor block of 9.6 hours with clonidine versus 4.3 hours without (Cucchiaro and Ganesh, 2007). This increased duration occurred in children who had received either upper or lower extremity nerve blocks for a variety of surgeries, and it was also independent of which local anesthetic was used. These findings differ from adult studies, where the use of clonidine for peripheral nerve blocks has shown to be of benefit only for upper extremity blocks, particularly axillary block, and with intermediate-acting local anesthetics (McCartney et al., 2007a). The prolongation of sensory analgesia found by Cucchiaro and Ganesh may have resulted from local effect from direct binding at α2-adrenergic receptors. The use of clonidine in children was also in addition to low concentrations of local anesthetics versus higher concentrations that are typically used in adults. Additional investigation is necessary to determine the overall usefulness of clonidine for peripheral nerve blocks in children. Although the early work appears promising, no prolongation of analgesia was found when 1 mcg/kg clonidine was added to bupivacaine for ilioinguinal/iliohypogastric nerve blocks in children (Kaabachi et al., 2005).
Upper Extremity Nerve Blocks
Upper extremity blocks may be used for surgery of the shoulder, arm, and hand. Specifically, brachial plexus blocks below the clavicle including the axillary approach are suitable for surgery on the hand and those blocks above the clavicle are useful for surgery on the shoulder and upper arm. Depending on the approach to the plexus, it is also possible to provide total analgesia to the hand with blocks above the clavicle. See related video online at www.expertconsult.com.
Anatomy
The brachial plexus contains the anterior branches of spinal roots C5 through T1 (Fig. 16-11). In the neck, these branches run between the anterior and middle scalene muscles and are enclosed in a fascial sheath. They then form three trunks (superior, middle, inferior) that exit the interscalene groove and run behind the subclavian artery. As they exit the interscalene groove, these cords form an anterior and posterior division. The divisions then unite to form lateral, posterior, and medial cords, depending on their relation to the axillary artery. There is a natural separation between the supraclavicular and infraclavicular plexus at the coracoid process that ultimately affects spread of local anesthetic (Vester-Andersen et al., 1986).
Understanding of the medial neck anatomy and components at each level of the plexus is crucial to avoiding complications (Fig. 16-12). Because the brachial plexus may be blocked in a number of locations, it is important to know the anatomy at each level in the neck area for successful block (Fig. 16-13). Close anatomic relationship of the carotid artery and internal jugular vein should be observed to select the ideal location for local injection. The proximity of the phrenic nerve overlying the anterior scalene muscle with founding cervical nerve roots C3 to C5 and cervical sympathetic chain explains resulting diaphragmatic paresis and Horner’s syndrome from the excessive spread of local anesthetic (Neal et al., 2009).
At the level of the axilla are the peripheral nerves that innervate the arm. The radial nerve supplies the dorsal aspect of the upper extremity below the shoulder including the thumb and dorsal aspects of the index, middle, and fourth fingers. The musculocutaneous nerve provides innervation to the biceps of the upper arm and cutaneous innervation to the lateral forearm. The median nerve innervates the majority of the forearm, as well as the ventral aspects of the second, third, and lateral portion of the fourth fingers, and the medial portion of the thumb. The ulnar nerve is more limited to the hand and innervates the lateral aspect of the fourth finger and all of the fifth (Fig. 16-14).
Interscalene Block
An interscalene block has been used for surgery of the shoulder and upper arm with success in children. It also anesthetizes the musculocutaneous nerve reliably, but there is less reliability of blocking the ulnar nerve than with the other nerve blocks because the ulnar nerve’s origin is at the lower portion of the plexus. Interscalene blocks are infrequently used in children because of the perceived higher risk for complications and side effects, and they are not recommended for placement in the anesthetized child because of risk for spinal cord injury (Bernards et al., 2008). For this reason, the technique will not be described.
Complications
Complications of interscalene block are not insignificant and include pneumothorax, spinal cord injury, epidural injection, or intrathecal injection. Vertebral artery puncture may also occur, with a risk for local anesthetic delivery directly to the CNS resulting in CNS toxicity. In addition, there is a high risk for phrenic nerve block with paralysis of the hemidiaphragm. Phrenic nerve block is not well tolerated, especially in infants or patients with underlying respiratory compromise (Kempen et al., 2000). Unilateral vocal cord paralysis may also occur and result in airway compromise. Sympathetic blockade with Horner’s syndrome is a common side effect of interscalene blocks.
Parascalene Block
The parascalene approach to the brachial plexus was developed by Dalens (1995) to provide effective analgesia to the shoulder and upper arm (Fig. 16-15, A) while minimizing the risks of vertebral artery puncture, dural puncture, and pneumothorax (i.e., the risks associated with an interscalene block). The parascalene block is similar to the interscalene approach, but by changing the insertion and direction of the needle, major structures in the neck are avoided. This block has been found to be easy to perform, with a 97% success rate in children on the first or second attempt (Dalens et al., 1987). See related video online at www.expertconsult.com
.
Technique
With the child supine and a roll under the shoulders, the arm should be adducted next to the trunk and the head turned to the opposite side. The primary landmarks are Chassaignac’s tubercle (transverse process of C6) and the midpoint of the clavicle. Draw a line between these two structures and insert a needle at the junction of the upper two thirds and lower one third of this line (see Fig. 16-15, B and C). This insertion spot should be at the level of the cricoid process near the external jugular vein. The approximate depth to the parascalene brachial plexus from the skin depends on the age of the child (see Fig. 16-6). Using low voltage, and once appropriate stimulation has been achieved, the local anesthetic solution is injected. Nerve stimulation for a parascalene block may result in muscle response in the biceps or triceps or with finger or hand movement. Shoulder muscle response suggests stimulation of the supraclavicular nerve, indicating that the needle is lateral to the plexus.
Supraclavicular Block
The supraclavicular block has the advantage of providing analgesia to all portions of the arm and is useful for nearly all upper extremity procedures. Despite a pneumothorax-free application of the supraclavicular block in 200 pediatric patients by landmark technique, the close anatomic relationship of pleura to the brachial plexus justifiably deters many pediatric anesthesiologists (Pande et al., 2000). The ultrasound-guided approach was demonstrated to be safe in 40 pediatric patients, had a higher success rate, and was faster to perform than the infraclavicular block (De Jose Maria et al., 2008). The ability of ultrasound to visualize the path and the tip of the needle, as well as the course of local anesthetic spread during injection, holds promise for using these blocks in a pediatric regional practice, and this has resulted in a pneumothorax-free application of supraclavicular block in pediatric patients. Because needle tip visualization is imperative to safe application of this procedure, only experienced ultrasound practitioners should attempt this block.
Technique with Ultrasound
A linear ultrasound transducer with the smallest available footprint, set at the highest operational frequency, should be used to achieve maximal resolution of the superficial supraclavicular structures (Fig. 16-16, A). The ultrasound machine should be positioned on the contralateral side in the direct line of view of the operator. The operator sits or stands by the patient’s shoulder on the ipsalateral side with the ultrasound transducer held in the nondominant hand and positioned parallel to the clavicle in the supraclavicular groove. The subclavian artery is identified in the short axis as a round, pulsatile structure, and the cervical pleura and lung are imaged below. The brachial plexus is located posterosuperior to the artery between the insertion of the anterior and middle scalene muscle onto the first rib and has a cluster-of-grapes appearance (hypoechoic center with hyperechoic rim) (see Fig. 16-16, B). The block needle is inserted in-plane in a lateral to medial direction. Ideally, the needle tip is placed between the first rib and the most inferior aspect of the brachial plexus (see Fig. 16-16, C). Injection at this location prevents superficial image distortion; pushes the brachial plexus up and away from the pleura, thus creating a margin of safety if the needle tip needs to be repositioned; and ensures blockade of the inferior trunk (see Fig. 16-16, D). The needle should be repositioned around the middle and superior nerve structures to achieve complete envelopment of the plexus by local anesthetic. To avoid pleural puncture, the needle tip should be visualized throughout the block placement, and to rule out intravascular injection, separation of tissues with incremental local anesthetic injection should be observed.
Lateral Vertical Infraclavicular Block
A lateral vertical infraclavicular brachial plexus block was introduced for pediatric use because the block can be performed without arm abduction. In addition, the block is more reliable for anesthetizing the musculocutaneous nerve, and this block provides better analgesia of the upper arm than an axillary block (Fleischmann et al., 2003). Although other infraclavicular approaches were considered too hazardous because of the risk for pneumothorax, the more lateral and vertical approach developed by Kapral (1999) and associates provides a safe distance between puncture site and pleura. This technique has been used in adults and children and has been shown to provide a greater spectrum of block than the axillary approach (Kapral et al., 1999; Fleischmann et al., 2003). The indications for a lateral infraclavicular block are the same as those for axillary block. See related video online at www.expertconsult.com.
Technique
With the child in the supine position, the upper arm should remain next to the trunk and the elbow flexed 90 degrees so that the forearm is on the abdomen. Palpate the coracoid process and using a nerve stimulator, insert a 1-inch 24-gauge insulated needle 0.5 cm distal to the coracoid process in a perpendicular or vertical direction while continuously aspirating for blood and/or air (Fig. 16-17). Once appropriate stimulation has been determined and continuous aspiration for blood or air has been negative, local anesthetic solution is injected. Obtaining wrist or finger movement predicts a successful block, whereas if only elbow twitches are seen, a failed block is likely to occur (Ponde and Diwan, 2009).
Infraclavicular Block
The infraclavicular block provides the same analgesia as can be expected from an axillary block, and it is suitable for distal upper extremity procedures. The ultrasound-guided infraclavicular block has been shown in children to offer shorter onset time, longer duration, and decreased discomfort during placement than nerve stimulation (Marhofer et al., 2004). In addition, the volume of local anesthetic needed to produce analgesia may be decreased with the ultrasound guidance, and the block can be successfully performed even in patients with altered response to nerve stimulation such as those with congenital abnormalities, trauma, or distal arm amputation (Sandhu et al., 2006; Ponde and Diwan, 2009). Because of the risk for pneumothorax, ultrasound-guided infraclavicular block is the preferred approach. See related video online at www.expertconsult.com.
Technique with Ultrasound
With the child in supine position and head rotated to the nonoperative side, the operator stands or sits at the head of the bed and positions the ultrasound transducer below and perpendicular (sagittal plane) to the clavicle in the infraclavicular fossa, slightly medial to the coracoid process. A linear transducer with a small footprint should be used for this block, given the narrow anatomic relationship of the infraclavicular fossa. The frequency selection is size dependent: larger children with deeper anatomy benefit from medium frequency range (10 to 7 MHz), but superficial structures ae imaged best with a high frequency (13 to 10 MHz) in young children and toddlers. The ultrasound machine should be positioned in the direct line of view of the operator on the ipsilateral side of the patient. The child’s arm can be kept at the side when block placement is attempted in an awake child with an injured extremity; however, if tolerated or in a sedated child, the arm is abducted to 90 degrees, and external rotation and flexion at the elbow raises the plexus closer to the skin and rotates the clavicle upward, thus creating additional space for probe positioning. Sonographic examination will reveal axillary vessels immediately deep to the pectoralis major and minor muscles, and the three hyperechoic cords surrounding the second part of the axillary artery at the 3-o’clock (medial cord), 6-o’clock (posterior cord), and 9-o’clock (lateral cord) positions (Fig. 16-18, A). The needle insertion site is determined by scanning the infraclavicular fossa in the mediolateral direction, and the ideal site is where the pleura is farthest separated from the plexus (see Fig. 16-18, B). The needle is advanced in-plane from the superior part of the transducer (cranial-caudal direction), and is positioned between the posterior cord of the brachial plexus and the axillary artery. Injection should produce circumferential spread around the axillary artery in young children; however, in older children, the median cord may be missed with a single injection and requires targeted injection to obtain a successful block. Maintaining continuous needle visualization may be challenging because of the acute angle of insertion, and it requires expertise in the ultrasound-guided techniques. Secondary confirmation of the needle tip can be gathered from tissue movement and hydro-locating with injection of 0.5 to 1 mL of normal saline.
Axillary Block
Axillary block is a common approach to the brachial plexus in children, as it is suitable for procedures on the hand such as syndactyly repair and finger reimplantation (Fig. 16-19, A). Advantages of performing an axillary block include the simplicity of the anatomy, ease of placement, and low risk for complications (Tobias, 2001a). Disadvantages include the need for a patient to be able to abduct the arm for access to the axilla, and the inability to block the musculocutaneous nerve 40% to 50% of the time because it branches higher in the axilla than the ulnar, median, and radial nerves. Because the musculocutaneous nerve innervates the lateral side of the forearm, it may need to be blocked separately for surgical procedures that involve that nerve’s distribution. Many approaches for an axillary block have been described and used in children. In a study to compare a single injection versus multiple injection technique in children, unlike in adults, there was no difference in block quality found between the two techniques (Carre et al., 2000). This study also confirmed that using either technique, separate block of the musculocutaneous nerve is still required if necessary for the surgical site.
In a study to assess the efficacy of the timing of an axillary block, 55 children received 2 mg/kg of 0.25% bupivacaine either prior to surgical incision, or immediately after surgery but before emergence (Altintas et al., 2000). In the presurgical group, 32% of the children required no additional analgesics within the first 24 hours, compared with 83% in the postsurgical group who required no additional analgesics. Although cumulative pain scores were higher in the presurgical group, nonetheless both groups had effective analgesia. See related video online at www.expertconsult.com.
Technique
A one-injection technique for axillary nerve block is accomplished by first palpating the axillary artery. The needle is then inserted immediately adjacent and superior to the artery high in the axilla, at a 30- to 45-degree angle aimed toward the midpoint of the clavicle (see Fig. 16-19, B and C). One may feel a pop as the plexus sheath is entered. Using a nerve stimulator, and after evidence of muscle stimulation in the hand is observed, ****local anesthetic is injected. A longitudinal swelling immediately beneath the skin as the local anesthetic fills the sheath may appear, particularly in infants and young children. This swelling disappears quickly as the anesthetic spreads proximally into the sheath, and this swelling should not be confused with a subcutaneous injection, which would have a more circular distribution and not disappear quickly. After the local anesthetic has been delivered and the needle removed, the arm should be adducted, thus releasing the pressure of the head of the humerus from the fossa. This motion along with holding distal pressure at the site of injection will promote proximal spread of the local anesthetic into the sheath. With a single-injection technique, all of the local anesthetic is delivered in one location. With a multiple-injection technique, the nerves are individually anesthetized by locating at least two of them individually using the nerve stimulator or ultrasound. Either technique may miss the musculocutaneous nerve because it exits the sheath proximal to the other three distal nerves. For this reason, a separate block of the musculocutaneous nerve is generally required. The musculocutaneous nerve may be blocked by directly inserting the needle into the belly of the coracobrachialis muscle while looking for stimulation of the biceps. In addition, if a surgical tourniquet is to be used and the patient is not having a general anesthetic, the intercostobrachial nerve should be blocked. This is accomplished by placing a subcutaneous ring of local anesthetic high around the inner aspect of the arm.
Technique with ultrasound
In the adult literature, ultrasound guidance has been demonstrated to improve block success rate (Chan et al., 2007; Lo et al., 2008), diagnose abnormal anatomy (Manickam et al., 2008), and allow a decrease in the required volume of local anesthetic and the number of needle passes (Casati et al., 2007). Although not yet prospectively demonstrated in a pediatric model, and appreciating the degree of anatomic nerve variability in relation to the axillary artery, the sonographic ability to guide the needle direction should prove to be of benefit in children as well.
To use ultrasound for axillary block, the operator stands or sits by the child’s ipsilateral shoulder and positions the ultrasound transducer transverse to the axilla at the crease formed by the pectoralis major and biceps muscles. A linear ultrasound transducer with the smallest available footprint, set at the highest operational frequency should be used to achieve maximal resolution of the superficial axillary plexus structures (Fig. 16-20, A). The ultrasound machine should be positioned in the direct line of view of the operator on the ipsilateral side of the patient. Sonographic examination will reveal a pulsatile axillary artery and a compressible axillary vein (or veins) surrounded by the coracobrachialis and biceps brachii (short head) muscle cranially and teres major and triceps brachii muscle caudally (see Fig. 16-20, B). The nerves of the axillary plexus exhibit fascicular appearance, appear in multiple shapes (round and oval most frequently), occupy varied locations around the axillary artery, and require dynamic distal tracing or neurostimulation to confirm the identity of each individual nerve (Retzl et al., 2001). The radial nerve is most frequently posterior or posteriocaudal (between 2 and 7 o’clock) to the axillary artery, deep to the ulnar nerve, and, when traced distally, it descends behind the triceps muscle into the spiral groove of the humerus accompanied by the radial collateral artery. The ulnar nerve is anterior or anteriocaudal (between 12 and 3 o’clock) to the axillary artery, and when traced distally, it remains superficial and slides medially from the brachial artery on its way to the ulnar nerve sulcus. The median nerve is anterior or anterocranial (9 to 1 o’clock) to the axillary artery, and it remains close to the brachial artery as it is traced distally. The musculocutaneous nerve has a hyperechoic appearance, changes in shape from oval to triangular, is located posterocranial to the axillary artery adjacent to the median nerve in the proximal axilla, and separates cranially to lie in a fascial plane between the coracobrachialis and the short head of the biceps brachii muscles in the distal axilla. The needle is advanced in-plane from the superior part of the transducer and is repositioned to sequentially block the radial, ulnar, medial, and musculocutaneous nerves. Injection of local anesthetic around the radial nerve first prevents the distortion of the deep anatomy and may help to localize the ulnar nerve. Median and musculocutaneous nerves can often be surrounded by local anesthetic with minimal needle adjustment when performing the block proximally in the axilla.
Complications
There should be few complications when performing an axillary block. There is the rare risk for hematoma and nerve compression, and for this reason a transarterial approach may not be recommended in children. If inadvertent axillary artery puncture occurs, firm pressure should be held for at least 5 minutes to avoid formation of hematoma and subsequent vascular insufficiency (Merril et al., 1981). Other complications may include relative distortion of anatomy after the first injection of local anesthetic in the axillary region, or inadvertent overdose of local anesthetic when multiple injection techniques are used (Dalens, 1995). However, these complications were not reported in the pediatric study by Carre and coworkers (2000).
Dosing of Upper Extremity Blocks
Various local anesthetics either alone or in combination have been used for upper extremity blocks. For prolonged analgesia, bupivacaine, levobupivacaine, or ropivacaine should be used. Because the brachial plexus is not highly vascular, the uptake of local anesthetic is less than that of pleural or central blocks, but the maximal allowable dosages of local anesthetic must be determined and the block dosed accordingly. When concentrations were compared, bupivacaine 2 mg/kg versus 3 mg/kg delivered for axillary block in children resulted in plasma levels of 1.35 mcg/mL and 1.84 mcg/mL, respectively (Campbell et al., 1986). These values are well below the toxic range. To compare 0.2% ropivacaine with 0.25% bupivacaine, Thornton and associates (2003) administered 0.5 mL/kg to children for axillary block. There was no significant difference between the two groups in pain scores, time to first analgesic, or total analgesic in 24 hours. The median time to first dose of analgesic was 7.25 hours in the ropivacaine group and 9.3 hours in the bupivacaine group. In general, if using 0.25% to 0.5% bupivacaine or levobupivacaine, or 0.2% to 0.5% ropivacaine, the lower concentration should be used in children 5 years of age or younger at a volume of 0.5 mL/kg. Using this volume under ultrasound guidance will result in a faster onset (Marhofer et al., 2004). Epinephrine 5 mcg/mL should be added to the solution to assist in identifying intravascular injection and to decrease the absorption of the local anesthetic. Using these dosing guidelines, approximately 4 to 12 hours of analgesia should be achieved (Table 16-4).
TABLE 16-4 Dosage Recommendations* for Peripheral Nerve Blocks
Regional Technique | Bolus Dose† | Continuous Infusion |
Axillary | 0.2-0.5 mL/kg | 0.1-0.2 mL/kg/hr |
Parascalene | 0.2-0.4 mL/kg | 0.1-0.2 mL/kg/hr |
Femoral or LFC | 0.3-1 mL/kg | 0.15-0.3 mL/kg/hr |
Fascia iliaca | 0.5-1 mL/kg | 0.15-0.3 mL/kg/hr |
Lumbar plexus | 0.5-1 mL/kg | 0.15-0.3 mL/kg/hr |
Sciatic | 0.3-1 mL/kg | 0.15-0.3 mL/kg/hr |
ILIH | 0.25 mL/kg | NA |
Penile block | 0.1 mL/kg | NA |
Paravertebral | 0.5 mL/kg | 0.2-0.25 mL/kg/hr |
TAP | 0.3-0.5 mL/kg | 0.25 mL/kg/hr |
ILIH, Ilioinguinal/iliohypogastric; LFC, lateral femoral cutaneous nerve; NA, not applicable; TAP, transversus abdominis plane.
* Bupivacaine, levobupivacaine, or ropivacaine may be used. For bolus dosing, lower concentrations, such as 0.2% to 0.25%, should be used for infants and young children, whereas concentrations of 0.375% to 0.5% should be used in children older than 5 to 8 years. For continuous infusions, lower concentrations such as 0.1% to 0.2% of all agents are acceptable.
† Epinephrine 1:200,000 should be added to single-shot peripheral nerve blocks except for penile block.
Clonidine 1 mcg/kg may be added to extend the duration of block and was used successfully in a large series of children (Cucchiaro and Ganesh, 2007). The use of clonidine may allow lower concentrations of local anesthetic to be used with durations similar to those expected with higher concentrations, with less risk for local anesthetic toxicity.
Peripheral Nerve Blocks in the Forearm
Individual blockade of the peripheral nerves in the distal upper extremity offers selective analgesia for minor surgeries, avoids excessive motor blockade, and provides rescue for failed brachial plexus blocks. When performed using a landmark-only technique, these blocks are restricted to the superficial sites at the elbow or wrist. This may result in nerve ischemia from local anesthetic injection into a tightly bound space such as the ulnar nerve at the elbow or median nerve at the wrist, or vascular complications such as brachial artery puncture at the elbow and ulnar artery at the wrist, and thus these blocks have been reported to have a failure rate of 10% to 30% in adults (Delaunay and Chelly, 2001). Ultrasound imaging allows tracking of each nerve along its course in the arm and selection of the optimal block location (McCartney et al., 2007b). The aim is to visualize the target nerve at a site distant from the vascular structures and to accommodate local anesthetic without increased compartment pressures. Use of a linear ultrasound transducer with the smallest available footprint, set at the highest operational frequency, is advised for performance of these blocks.
(See www.expertconsult.com to view the complete text for the median, ulnar, and radial nerve blocks in the forearm, and to view Figs. 16-21 through 16-23.)
Median Nerve Block
The median nerve is consistently found overlying the brachialis muscle and medial to the brachial artery in the antecubital fossa. It is hyperechoic and of approximately the same size as the artery (Fig. W16-21, A). As the nerve leaves the fossa, it splits from the brachial artery and angles downward between the pronator teres and flexor digitorum superficialis muscles (Fig. W16-21, B). In the mid forearm, the median nerve is clearly visualized between the flexor digitorum superficialis muscle superiorly and flexor digitorum profundus muscle inferiorly (Fig. W16-21, C). The nerve assumes a superficial position at the wrist under the flexor retinaculum, and where it clusters with and between the flexor carpi radialis tendon laterally and the flexor digitorum superficialis tendon medially (Fig. W16-21, D). Distinguishing the median nerve from the tendons can be challenging at this location, but tracing the structures proximally can be helpful.
The nerve blockade at the antecubital fossa and wrist are typically performed with the needle in an out-of-plane orientation, given the superficial location of the nerve, and an in-plane approach is used for the proximal and mid forearm (Fig. W16-21, E). To determine where to block the median nerve, the following should be considered: the ease of nerve localization (clearly visualized at the antecubital fossa and mid forearm), rates of complication (e.g., arterial hematoma at the elbow and tightly bound space at the wrist), sensory innervation (the palmar branch may be missed with the blockade at the wrist), motor blockade (forearm muscle sparing when anesthetizing the median nerve below the antecubital fossa), and tourniquet location (avoid injecting at a location intended for the tourniquet). The mid forearm is therefore a common location for this block.
Ulnar Nerve Block
The ulnar nerve emerges out of the ulnar nerve sulcus at the medial epicondyle and is bounded by the flexor carpi ulnaris (superior), flexor digitorum superficialis (lateral), and flexor digitorum profundus (medial) (Fig. W16-22, A). In the mid forearm, the ulnar artery joins the ulnar nerve (Fig. W16-22, B) and maintains this association into the wrist (Fig. W16-22, C). The ulnar nerve becomes progressively smaller as it travels toward the wrist and is found superficial and medial to the ulnar artery.
The ulnar nerve blockade is performed at the proximal and mid-forearm level, with the arm abducted and flexed at the elbow and the hand supinated. The needle is introduced in-plane with the ultrasound view at these locations to avoid inadvertent vascular injury (Fig. W16-22, D). At the wrist level, an out-of-plane needle orientation is easiest given the superficial landscape (Fig. W16-22, E). The proximal forearm is a preferred location as it minimizes the risk of vascular injury and ensures blockade of the dorsal branch of the ulnar nerve, which separates above the wrist.
Radial Nerve Block
The radial nerve snakes its way down the lateral humerus (Fig. W16-23, A) until it enters the antecubital fossa between the brachioradialis (lateral) and brachialis (medial) muscles (Fig. W16-23, B) and bifurcates into the sensory and motor branches. The sensory branch (i.e., the superficial radial nerve) continues in the forearm between the extensor muscles over the radius, but it is difficult to image below the mid forearm (McCartney et al., 2007). The motor branch (i.e., the deep radial nerve) descends in the proximal forearm to become the posterior interosseous nerve, and it then crosses into the extensor compartment.
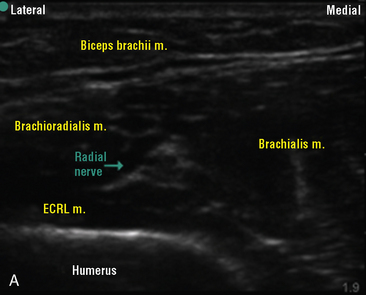
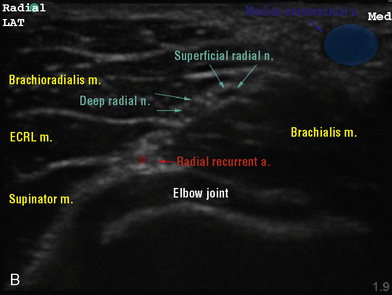
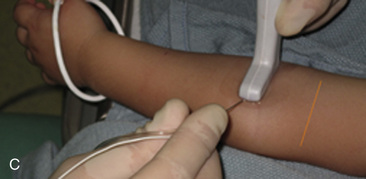
FIGURE W16-23 Ultrasound radial nerve block. A, At lateral humerus. B, At antecubital fossa. C, Needle and transducer near fossa.
The radial nerve blockade is performed with the needle in-plane and the arm abducted (Fig. W16-23, C). Block placement at the antecubital fossa may allow selective anesthesia of the sensory branch of the radial nerve and sparing of the motor component. The recurrent proximity of the radial artery at this level increases the risk of vascular puncture and justifies an in-plane approach. The antecubital fossa is a preferred location for a radial nerve block.
Lower Extremity Nerve Blocks
Although central axial blocks are commonly performed pediatric regional anesthetic techniques, lower extremity nerve blocks often provide analgesia to the lower limbs with a more direct effect (McNicol, 1986; Dalens, 1995; Ross et al., 2000; Tobias, 2003). Lower extremity blocks are performed by anesthetizing the lumbar or sacral plexus, or both. See related video online at www.expertconsult.com.
The lumbar plexus is located in the psoas compartment that lies in the paravertebral space (Fig. 16-24). The union of the anterior rami of lumbar nerves L1 to L4 constitutes the primary input of the lumbar plexus with a small portion of the 12th thoracic nerve. As the plexus emerges from the paravertebral space, it divides into three nerves, the femoral, the lateral femoral cutaneous, and the obturator. Although the iliac vessels run anterior to the iliac fascia, these three nerves remain posterior to the fascia. The femoral nerve is a mixed nerve with motor innervation to the quadriceps muscles and sensory innervation to the anterior and medial thigh. A branch of the femoral nerve, the saphenous nerve, provides innervation below the knee to the medial aspect of the lower leg and foot near the saphenous vein. The lateral femoral cutaneous nerve is a sensory nerve with innervation to the lateral thigh, and the obturator nerve is primarily motor to the leg adductors with some sensory to the lower medial thigh and knee.
The sacral plexus is derived from the anterior rami of L4, L5, and S1 to S3, and it gives rise to the sciatic nerve and the posterior cutaneous nerve of the thigh (Fig. 16-25). The sciatic nerve is a mixed nerve that provides motor and sensory innervation to the posterior aspect of the thigh and most of the lower leg. As the sciatic nerve travels down the posterior thigh, it branches into the common peroneal and posterior tibial nerves.
Specific blocks of the lower extremity will be described.
Lateral Femoral Cutaneous Nerve Block
Although an isolated block of the lateral femoral cutaneous nerve (LFC) is rarely needed, it may be blocked to provide analgesia for muscle biopsy of the vastus lateralis muscle during malignant hyperthermia testing (Wedel, 1989). An LFC block may also be used in combination with a femoral nerve block in high-risk children in place of a general anesthetic for complete analgesia for muscle biopsies of the thigh (Rosen and Broadman, 1986; Maccani et al., 1995). See related video online at www.expertconsult.com
.
Anatomy
The lateral femoral cutaneous nerve arises from second and third lumbar nerves and travels deep to the iliacus fascia toward the anterior superior iliac spine until it emerges under the fascia lata in the upper thigh. It is a pure sensory nerve that innervates the lateral thigh to the knee, including some terminal branches at the patellar plexus (Fig. 16-26, A).
Technique
No nerve stimulator is required to block the LFC, as it is purely a sensory nerve. In the infrainguinal approach, a blunt 22-gauge needle is inserted perpendicular to the skin, aiming in the direction of the nerve inferolaterally 0.5 to 1 cm below the inguinal ligament and medial to the anterior superior iliac spine (see Fig. 16-26, B). A pop will be felt as the needle pierces the fascia lata. Local anesthetic is then injected in a fanlike manner (McNicol, 1986).
Femoral Nerve Block
A femoral nerve block may be used for any above-the-knee surgery of the lower extremity that requires analgesia of the majority of the thigh (Fig. 16-27, A). This includes analgesia for femur fracture (Ronchi et al., 1989). The block is simple to perform, either with or without a nerve stimulator, but a nerve stimulator should not be used in an awake child with a femur fracture because of the pain that may occur with muscle contraction from nerve stimulation. See related video online at www.expertconsult.com.
Anatomy
The femoral nerve, derived from lumbar nerves 1 to 3, enters the thigh in the femoral triangle below the inguinal ligament. The approximate depth to the femoral nerve from the skin should be reviewed (see Fig. 16-6). The nerve is immediately lateral to the femoral artery and is covered by the fascia lata and fascia iliaca (see Fig. 16-27, B).
Technique
With the child supine and the feet rotated outward, the femoral artery is palpated immediately below the inguinal ligament. The needle is inserted at a slight cephalad angle to the skin at 0.5 to 1 cm below the inguinal ligament and 0.5 to 1 cm lateral to the artery (see Fig. 16-27, C). As the needle pierces the fascia lata, a distinct pop is felt. If a nerve stimulator is used, the desired muscle response should be contraction of the mid quadriceps with a “patellar kick” If there is muscle stimulation medial to the mid patella at the thigh adductors, the needle is slightly adjusted laterally. If there is lateral muscle stimulation, the needle is adjusted slightly medially. Because of the close proximity of the femoral vessels, continuous aspiration for blood should be performed to detect intravascular entry. Once the desired location of the needle is achieved, local anesthetic is then injected.
A 3-in-1 block is a modification of a femoral nerve block. This technique anesthetizes the lateral femoral cutaneous and obturator nerves, which lie more proximal in the sheath. The 3-in-1 technique is accomplished by performing a femoral nerve block and promoting proximal spread. The landmarks and needle insertion are exactly the same as for a simple femoral block, except that the volume of local anesthetic is increased and distal pressure is used to promote cephalad spread to the lumbar plexus with the femoral sheath as a conduit. When compared with a fascia iliaca compartment block (see later), a 3-in-1 approach can result in a higher failure rate (Dalens et al., 1989). Although it is effective in anesthetizing the femoral nerve 100% of the time, the LFC and obturator were successfully blocked in only 20% of the children. Dalens noted that the successful blocks of the LFC and obturator nerves in the 3-in-1 group were not the result of proximal spread of the local anesthetic but possibly of a fascia iliaca–like spread of the local anesthetic.
Technique with ultrasound
Ultrasound-guided femoral nerve block has been shown to improve the quality and lengthen the duration of the block while decreasing the required dose of the local anesthetic as compared with the nerve stimulation technique (Marhofer et al., 1997, 1998; Oberndorfer et al., 2007).
A linear ultrasound transducer set at the highest operational frequency should be used to achieve maximal resolution of the superficial inguinal anatomy. The patient is positioned supine with the leg slightly bent at the knee and externally rotated. The ultrasound machine should be positioned on the contralateral side in the direct line of view of the operator. The operator sits or stands by the patient’s hip on the ipsalateral side, with the ultrasound transducer held in the nondominant hand and positioned transverse to the femoral artery, inferior to the inguinal ligament (Fig. 16-28, A). Femoral vessels are a readily recognized starting point for locating the nerve (see Fig. 16-28, B). The femoral nerve often has a triangular, hyperechoic appearance and is located lateral to the femoral artery, superficial to the iliopsoas muscle, and beneath the fascia iliaca. The block needle is inserted in-plane in a lateral-to-medial direction (see Fig. 16-28, A) and should be seen transversing the superficial fascia lata and immediately adherent fascia iliaca before the test dose is administered. Local anesthetic injection should produce circumferential spread around the nerve bundle below the fascia iliaca (see Fig. 16-28, C). Out-of-plane needle insertion is possible for the performance of this block once the inguinal sonoanatomy is appreciated, and for the placement of continuous catheters.
Fascia Iliaca Compartment Block
The fascia iliaca block provides analgesia of the femoral, lateral femoral cutaneous, and obturator nerves. A fascia iliaca block may be effective in more than 90% of children, compared with 20% effectiveness of the 3-in-1 technique (Dalens et al., 1989). Fascia iliaca blocks are useful for all above-the-knee lower extremity surgeries because of their ability to anesthetize this region in its entirety (Fig. 16-29, A). In addition, the fascia iliaca block reliably anesthetizes the femoral branch of the genitofemoral nerve, the sensory nerve supply to Scarpa’s triangle. Because of its ability to block the upper leg, the fascia iliaca compartment block can be used in combination with intravenous sedation or nitrous oxide for children who are undergoing muscle biopsy of the thigh. See related video online at www.expertconsult.com.
Anatomy
The three distal nerves of the lumbar plexus, the femoral, lateral femoral cutaneous, and obturator nerves, all emerge from the psoas muscle and run along the inner surface of the fascia iliaca. A fascia iliaca compartment block delivers local anesthetic between the fascia iliaca and iliacus muscles where it spreads to bathe the three nerves (see Fig. 16-29, B).
Technique
With the child in the supine position, the inguinal ligament is located by drawing a line from the pubic tubercle to the anterior superior iliac spine. Divide the inguinal ligament into thirds. At the junction of the lateral third and the medial two thirds of the inguinal ligament, drop a line inferiorly 0.5 to 2 cm and perpendicular to the ligament. This is the point of needle insertion (see Fig. 16-29, C). A blunt needle is inserted perpendicular to the skin. There is no need for a nerve stimulator, because the goal is to find the area behind the iliacus fascia for anesthetic injection, not to locate a specific nerve. Two pops are felt as the needle first pierces the fascia lata, then the fascia iliaca. If light pressure is held on the plunger of the syringe, a loss of resistance is felt as the fascia iliaca is pierced. With the needle in the correct position, local anesthetic solution is injected.
Lumbar Plexus Block
Like the fascia iliaca compartment block, a lumbar plexus block provides analgesia to the three major nerves of the lumbar plexus. This block is useful for any surgery that may occur on the upper leg because it can completely anesthetize that region. This block also anesthetizes the distal branches of the lumbar plexus, including the iliohypogastric, ilioinguinal, and genitofemoral nerves that innervate the groin area applicable to many pediatric surgical procedures (Fig. 16-30, A). See related video online at www.expertconsult.com.
Anatomy
The lumbar plexus lies in the psoas compartment between the two masses of the psoas muscle that attach to the vertebrae, and it is surrounded by fascia derived from fascia iliaca (see Fig. 16-30, B). The approximate depth from the skin to the lumbar plexus at different ages is noted in Figure 16-6.
Technique
In a study of 50 children aged 6 months to 16 years undergoing hip and upper lower extremity procedures, Dalens and coworkers (1988) compared two techniques of lumbar plexus block. In group 1, a modification by Chayen of a psoas compartment block was used. A needle was inserted at the midpoint of a line connecting the spinous process of the fifth lumbar vertebra and the posterior superior iliac spine. There were difficulties with needle insertion in 7 of 25 children, and 23 of the 25 had epidural spread of local anesthesia. In the second group, a modification of Winnie’s approach was used. In this technique, a needle was inserted at the intersection of the line drawn to connect the iliac crests and a line drawn through the posterior superior iliac spine parallel to the spinous processes (Fig. 16-30, C). There were no problems with needle insertion, and all 25 patients exhibited a unilateral lumbar plexus block distribution. Sacral distribution occurred in 23 of the 25 children, as these two plexuses are found in the same anatomic planesee. Although both techniques provided effective analgesia to the lumbar plexus, the Chayen approach resulted in epidural spread rather than being isolated to the lumbar plexus. Because of the greater ease of performance of the modified Winnie technique, this technique will be further described for use in children.
Modified Winnie approach to the lumbar plexus
With the child in the lateral position, block side up, the knees and thighs are flexed. Two lines are drawn: (1) to connect the two iliac crests, and (2) from the ipsilateral posterosuperior iliac spine running cephalad and parallel to the spinous processes. The needle is inserted perpendicular to the skin at the intersection of the two lines (see Fig. 16-30, C) and then advanced through the quadratus lumborum. If contact is made with a transverse process, the needle is directed slightly more cephalad until a strong contraction of the mid (not lateral or medial) quadriceps with a patellar kick is apparent. If hamstring contractions are observed, the needle is directed slightly more laterally. If there is isolated hip movement, the psoas has been directly stimulated. If the quadriceps and hamstrings are contracting simultaneously, the needle should be directed more cephalad to stimulate the lumbar rather than sacral plexus.
The needle is placed in the same location as in the landmark technique for ultrasonographic-guided lumbar plexus block (see Fig. 16-30, D). Sonoanatomy shows the relationship to the structures before (see Fig. 16-30, E) and after (see Fig. 16-30, F) local anesthetic injection.
Complications
Although complications are rare, they may be serious if the needle is advanced too deeply into the retroperitoneum. Retroperitoneal hematoma is a significant risk, and continuous aspiration for blood should be done while performing the block. The highest incidence of major bleeding after peripheral regional anesthetic techniques has been found to occur after a psoas compartment block (Horlocker et al., 2003a).
Saphenous Nerve Block
Technique with Ultrasound
Multiple approaches to the saphenous nerve block have been described, but visualization of this nerve in a child can be challenging, so the transsartorial perifemoral artery approach is preferred (Benzon et al., 2005; Tsui and Ozelsel, 2009). A linear ultrasound transducer set at the highest operational frequency should be used. The patient is positioned supine with the leg slightly bent at the knee and externally rotated. The ultrasound machine should be positioned on the contralateral side in the direct line of view of the operator. The operator sits or stands by the patient’s knee on the ipsalateral side with the ultrasound transducer held in the nondominant hand and positioned transverse to the longitudinal axis of the extremity at the mid thigh (Fig. 16-31, A). The femoral artery in the adductor canal is a reliable landmark for locating the nerve (see Fig. 16-31, B). The saphenous nerve appears hyperechoic and is located anterior to the artery below the sartorius muscle. The block needle is inserted in-plane in a lateral-to-medial direction toward the nerve, and circumferential envelopment by local anesthetic is the goal. If the nerve is not clearly visualized in the adductor canal, a perivascular injection will be adequate. As the practioner gains expertise in identifying and tracing the saphenous nerve, it may be advantageous to perform saphenous nerve blockade distally, as this will avoid motor blockade of the vastus medialis muscle because the nerve to the vastus medialis is located in the adductor canal.
Sciatic Nerve Block
A sciatic nerve block is indicated for surgical procedures that involve the lower extremity below the knee. When used in combination with blocks of the lumbar plexus, the lower extremity can be blocked in its entirety. See related video online at www.expertconsult.com.
Anatomy
The sciatic nerve is derived from the anterior rami of L4 to S3 and is the largest nerve in the body (see Fig. 16-25). It emerges through the greater sciatic foramen to run between the greater trochanter of the femur and the ischial tuberosity before taking its position in the thigh posterior to the quadriceps femoris. If the sciatic nerve is blocked in its proximal position, this will also anesthetize the posterior femoral cutaneous nerve (a branch of ventral rami of S1 to S3). This nerve innervates the posterior thigh above the knee and the hamstring muscles. The sciatic nerve primarily consists of two nerves, the tibial and common peroneal nerves, which travel in a common sheath in the posterior upper portion of the leg. These nerves typically divide near the popliteal fossa and innervate the leg below the knee.
Approaches to the Sciatic Nerve
Several approaches to the sciatic nerve have been described in children. The posterior, anterior, and lateral approaches have been compared with respect to ease of performance, efficacy of block, and rate of complications (Dalens et al., 1990). The overall success rate of all three approaches exceeded 90%. However, there were fewer difficulties reported with the posterior approach. The posterior approach resulted in an 88% success rate on first attempt, compared with 78% for the lateral approach and only 62% on the first attempt for the anterior approach. In addition, vascular punctures occurred only in children who underwent an anterior approach. Because of the higher success rate of the posterior approach and the completeness of analgesia of the sciatic nerve and posterior branches (Fig. 16-32, A), the posterior approach is described here. However, the reader is prompted to review the lateral approach, especially for use in children who are unable to be positioned for other approaches to the sciatic nerve (Dalens, 1995).
To block the sciatic nerve using the posterior approach, a modification of Labat’s technique was developed by Dalens and associates (1990). The child is placed in the lateral position with the side to be blocked uppermost and the upper leg flexed at the hip and knee. Using a nerve stimulator and insulated needle, the point of needle insertion is at the midpoint of the line that extends from the tip of the coccyx to the greater trochanter of the femur. The needle should be perpendicular to the skin with slight angulation toward the lateral ischial tuberosity (see Fig. 16-32, B and C). The approximate depth to the sciatic nerve using the posterior approach depends on the age of the patient (see Fig. 16-6). Using a nerve stimulator, the motor response is a movement in the patient’s foot. Plantar flexion indicates stimulation of the tibial nerve. Dorsiflexion or eversion at the ankle indicates stimulation of the peroneal nerve. Once the appropriate response is elicited, local anesthesia is injected.
The Raj block was developed in 1975 and is similar to the posterior approach (Raj et al., 1975). This approach anesthetizes the sciatic nerve slightly more distal than in the classic posterior approach (Fig. 16-33, A). This block is performed in the supine child with the leg to be blocked lifted and flexed at the hip and knee. The needle is inserted at the midpoint between ischial tuberosity and greater trochanter in the sciatic groove (see Fig. 16-33, B and C). Once appropriate muscle stimulation with less than 0.5 mA is seen at the foot, local anesthetic is injected. The advantage to this block is the reliability of the landmarks and simplicity of the block itself. By flexing the hip, the Raj technique brings the sciatic nerve closer to the skin. This improves the likelihood of a successful block, especially in obese children and adolescents.
To use some of the principles of the posterior and Raj approaches, the ultrasound-guided subgluteal approach may be performed. A linear, high-frequency probe can be used in children, but as the depth of the imaged neural structure increases with age or obesity, use of the lower-frequency, curvilinear probe is required to achieve sufficient penetration. The child is positioned in a lateral decubitus position with the operative side uppermost and the knee flexed. The operator faces the patient with the ultrasound machine across in the direct line of view. A line connecting the greater trochanter and ischial tuberosity represents a starting point for the sonographic examination (Fig. 16-34, A). The sciatic nerve is visualized between the anterior surface of the gluteus maximus and the posterior surface of the quadratus femoris muscle. It is bounded medially by the ischial tuberosity and laterally by the greater trochanter. It has hyperechoic appearance and may be oval or triangular (see Fig. 16-34, B). Tracing the nerve cranially or caudally may allow for improved sonographic visualization. The posterior cutaneous nerve of the thigh is frequently observed medial to the sciatic nerve at this location (Karmakar et al., 2007). An in-plane approach is used to guide needle advancement, and circumferential spread of local anesthetic is sought. Medial envelopment of the sciatic nerve by local anesthetic ensures blockade of the posterior cutaneous nerve of the thigh and may require needle repositioning when thigh incision or tourniquet use is planned. An out-of-plane approach is used for continuous catheter placement because it facilitates threading and fixation of the catheter (van Geffen and Gielen, 2006).
A popliteal fossa block may be used for procedures of the distal lower extremity and will anesthetize the sciatic nerve more distally in the leg and just proximal to the knee (Kempthorne and Brown, 1984) (Fig. 16-35, A). See related video online at www.expertconsult.com. Near the popliteal fossa, the sciatic nerve divides into the common peroneal nerve and the posterior tibial nerve (see Fig. 16-35, B). The common peroneal nerve runs anteriorly to wrap around the head of the fibula, and the posterior tibial nerve travels down the posterior lower leg. In approximately 10% of the population, the branching of the sciatic nerve occurs more proximal to the popliteal fossa and high in the posterior thigh. Therefore, there may be a variable success rate in blocking the sciatic at this level, but both nerves are usually blocked by this approach because a common epineural sheath envelops the two nerves (Vloka et al., 1997). Advantages to the popliteal approach include the relatively superficial location of the sciatic nerve (i.e., near to the skin) and the decreased risk of intraneural injection, as the sciatic nerve is not fixed against any bony structures in this location. To easily access the popliteal fossa for a single-shot block, the patient may remain in the supine position and the leg to be blocked is lifted with the knee and thigh flexed. The child may also be turned to the lateral position and the leg to be blocked positioned uppermost. The superior triangle of the popliteal fossa has as its boundaries the semimembranosus and semitendinosus tendons medially, the biceps femoris tendon laterally, and the popliteal crease inferiorly. The needle is inserted 45 degrees to the skin, aiming cephalad and just lateral to the midline of the popliteal triangle (see Fig. 16-35, C). The distance from the popliteal fold to needle insertion is estimated based on weight. If the weight is less than 10 kg, the distance is 1 cm; if the weight is 10 to 20 kg, the distance is 2 cm (Konrad and Johr, 1998). Each 10 kg of body weight should move the needle cephalad in the triangle approximately 1 cm. Muscle stimulation of the foot in either the common peroneal or the posterior tibial distribution is a confirmation of an acceptable needle position, but stimulation of both branches suggests that the sciatic nerve has not yet separated into the two branches at this level. Local anesthetic is injected once appropriate stimulation is apparent at less than 0.5 mA.
Konrad and Johr (1998) sought to determine a system for standardization of popliteal fossa block. They performed the block in 50 children between the ages of 2 months and 18 years. They determined that the minimal distance to the sciatic nerve in the popliteal fossa was 13 mm, and the depth did not vary significantly in patients weighing less than 35 kg but did increase for children weighing more than 35 kg. All blocks in the study were successful, and there were no complications. Vascular puncture was avoided because of the lateral position of the needle in relation to the popliteal vessels.
Tobias and Mencio (1999) provided analgesia in 20 children for foot and ankle surgery by performing popliteal fossa blocks at the completion of the surgical procedure. When using 0.75 mL/kg of 0.2% ropivacaine, the duration of analgesia was between 8 and 12 hours.
The ultrasound-guided popliteal approach can also be performed with the linear ultrasound transducer set at the highest operational frequency. The child can be positioned supine with the leg elevated on a footrest or held by an assistant (Fig. 16-36, A) or lateral with the operative side uppermost and the knee flexed (see Fig. 16-36, B). The operator stands or sits by the child’s ipsilateral limb with the ultrasound machine in the direct line of view. The sonographic examination begins by positioning the transducer over the popliteal fossa in an axial plane and locating the popliteal artery and vein. The tibial nerve is located superficial to the vein and has a round or fasciculated appearance. Dynamic cephalad tracing will identify the point of merger of the tibial and common peroneal components into the sciatic nerve (see Fig. 16-36, C and D). The level of the local anesthetic placement should be proximal to the division of the sciatic nerve to achieve complete blockade of the foot. The sciatic nerve exhibits a significant degree of anisotropy and is best visualized when the insonating ultrasound angle is directed caudally. An in-plane, lateral-to-medial approach is used to guide needle advancement for single-shot blocks, and the needle tip is guided posterior to the sciatic nerve to achieve the circumferential spread of local anesthetic (see Fig. 16-36, E and F). An out-of-plane approach is used for continuous catheter placement, because it facilitates threading and fixation of the catheter.
Ankle Block
An ankle block is a simple block that provides analgesia to the foot for procedures such as toe removal or simple reconstructive surgery. See related video online at www.expertconsult.com.
Anatomy
Five nerves that innervate the foot must be blocked for analgesia of the foot in its entirety (Fig. 16-37). The saphenous nerve is found near the saphenous vein on the medial side of the dorsum of the foot and is somewhat superficial in its location. It innervates the skin surrounding the medial malleolus. Following the dorsum of the foot, and near the anterior tibial artery, runs the deep peroneal nerve, which is responsible for the innervation of the web space between the first and second toes. As the name implies, this nerve runs deep and is found near the tibia and between the extensor hallucis longus and anterior tibial artery. Immediately lateral to the deep peroneal nerve, but found superficially, is the superficial peroneal nerve. This nerve innervates the medial and lateral aspects of the dorsum of the foot. The plantar innervation of the foot is supplied by the tibial and sural nerves. The tibial nerve is found immediately posterior to the posterior tibial artery and medial malleolus, and the sural nerve is located posterior to the lateral malleolus.
Dosing of Lower Extremity Blocks
The volumes of local anesthesia depend on the nerves to be blocked. For femoral nerve blocks, 0.2 to 0.75 mL/kg is administered, whereas for plexus blocks, volumes of greater than 1 mL/kg are frequently needed (see Table 16-4). The duration of action of local anesthesia depends on the anesthetic concentration and age of the patient. When used in combination, local anesthetics are additive. It is important not to exceed maximal allowable dosing (see Table 16-2).
Continuous Peripheral Nerve Catheters
There is little information on the continuous infusions of local anesthetics in upper extremity catheters in children, but dosing of an indwelling catheter for brachial plexus anesthesia should adhere to maximal allowable dosing guidelines (see Table 16-2). Typical dosages should start at 0.1 to 0.2 mL/kg per hour of either bupivacaine or levobupivacaine (0.125% to 0.25%) or ropivacaine (0.1% to 0.2%) (see Table 16-4). Increases in the infusion rate may be made as needed as long as the maximal rate does not exceed 0.2 mg/kg per hour in infants less than 6 months, or 0.4 mg/kg per hour in children older than 6 months. It would be unusual, however, for peripheral nerve catheter infusions to reach maximal limits.
Continuous catheters have been used for the lower extremity in children, with early cases describing its use for femur fractures of patients in the intensive care unit (ICU) (Johnson, 1994; Tobias, 1994). Johnson used an epidural catheter in the femoral sheath and delivered 0.125% bupivacaine at 0.3 mg/kg per hour. In these patients, plasma bupivacaine concentrations were well below toxic levels. Using a Seldinger technique, and a 3-French, 8-cm, single-lumen central line catheter (Cook Critical Care, Bloomington, IN), Tobias administered continuous infusions at 0.15 mL/kg per hour of 0.2% bupivacaine to four children with femur fractures and closed head trauma. The catheters provided adequate analgesia in the ICU setting for 4 to 6 days and there were no complications. To provide more complete analgesia of the upper portion of the leg, catheters may also be placed in the lumbar plexus or fascia iliaca compartments. Sciard and coworkers (2001), using nerve stimulation, placed 20-gauge plexus catheters (Pajunk, Albany, NY) in the lumbar plexus of children and administered continuous infusions of 0.2% ropivacaine at 0.33 to 0.4 mg/kg per hour. Paut and associates (2001) inserted 20-gauge catheters (Contiplex, B. Braun, Melsungen, Germany) through 55-mm cannulae into the fascia iliaca compartment and administered continuous infusions of 0.1% bupivacaine at a rate of 0.5 mL/kg per year of age. After 48 hours, the mean delivered dosage of bupivacaine was 0.135 ± 0.03 mg/kg per hour, and the plasma bupivacaine levels at 24 and 48 hours were not significantly different at 0.71 mcg/mL and 0.84 mcg/mL, respectively. The authors concluded that the bupivacaine plasma concentrations at the rates used in their study for a continuous fascia iliaca block are within safety margins.
When compared with a continuous epidural catheter, a continuous popliteal catheter provides similar excellent postoperative analgesia without the side effects of urinary retention, nausea, and vomiting (Dadure et al., 2006). Children in this study received a popliteal catheter infusion of 0.2% ropivacaine at 0.1 mL/kg per hour for 48 hours and received a 100% parent satisfaction score. The subgluteal approach to the sciatic nerve has been used for continuous catheters by employing an ultrasound-guided approach to placement of a stimulating catheter (van Geffen and Gielen, 2006). These children received bupivacaine 0.25% at a rate of 0.1 mL/kg per hour and had excellent pain relief, with no children receiving morphine other than those who had undergone amputation. The children who had undergone limb amputation received intravenous morphine despite pain scores of less than 4 as part of their multimodal therapy.
The use of disposable pumps for continuous delivery via a peripheral catheter of local anesthetic solutions provides a great option for postoperative pain control after outpatient orthopedic surgery. Dadure and coworkers (2003) described the use of the disposable elastomeric pumps in 25 children aged 1 to 15 years receiving continuous infusion via catheters in the popliteal, femoral, or axillary sheaths. A continuous infusion of 0.2% ropivacaine was used at a rate of 0.1 mL/kg per hour. The median pain score for all children was zero up to 48 hours, and there were no adverse events. A larger study at Children’s Hospital of Philadelphia reported on the use of 226 continuous peripheral nerve catheters in children aged 4 to 18 years (Ganesh et al., 2007). There was a 2.8% incidence of complications. Three patients had prolonged numbness that resolved spontaneously, one patient had cellulitis that was treated with antibiotics, one patient developed tinnitus that was reversed with clamping of the local anesthetic catheter, and one patient had difficulty removing the catheter at home. The conclusion of this report was that it is feasible to implement a program that provides continuous peripheral nerve catheters in children when appropriate expertise is available. Education of the patient and parents as well as frequent follow-up are important to recognize potential complications in a timely fashion.
Patient-controlled regional analgesia was investigated in 30 children who had undergone lower limb orthopedic surgery (Duflo et al., 2006). When comparing 0.2% ropivacaine delivered as a continuous infusion or by patient control, both groups had adequate analgesia, but the latter group achieved it with significantly less local anesthetic and, therefore, lower total plasma levels of ropivacaine.