25 Radiosurgery
Stereotactic radiosurgery (SRS) is most often defined as the delivery of a single high dose of ionizing radiation to an intracranial target defined using stereotactic imaging.1 Although the definition has been expanded by some to include up to five fractions delivered using stereotactic technology, this chapter is limited to a discussion of the single-dose approach and results. Extracranial SRS is discussed in Chapter 75.
The use of accelerated particles for highly conformal treatment of brain tumors developed following the proposal of physicist Robert Wilson and coworkers at the University of California, Berkeley.2 The initial clinical trial using protons to treat pituitary disorders was begun in 1954 with 840 patients subsequently treated with protons or helium ions.3,4 These focal-radiation delivery methods were further developed and the term radiosurgery was coined by Swedish neurosurgeon Lars Leksell and radiobiologist Borje Larsson of the Karolinska Institute in Stockholm, initially for treatment of functional disorders.5 Several different delivery techniques result in a dose distribution that is highly conformal to the target. Dose fall-off outside the target is precipitous and therefore only the target and immediately adjacent normal tissue are included in the high-dose region. After more than 40 years of development, radiosurgery has entered the mainstream of medical care. Since the 1960s, more than 1,000,000 patients have been treated with some form of radiosurgery, most within the past 10 years. Radiosurgery is now practiced at more than 250 facilities in the United States.
In North America, patients who undergo radiosurgery are usually selected, treated, and followed by a dedicated multidisciplinary radiosurgery team of experts that includes radiation oncologists, neurosurgeons, physicists, nurses, and technologists; the same radiosurgery team provides quality assurance. This multidisciplinary approach to therapy has received support from the radiosurgery task forces of societies representing both neurosurgery and radiation oncology, and is considered the standard of care in the United States.1,6–8
Techniques
Stereotaxis
The term stereotactic (from the Greek stereo and taxis, ordered in three dimensions) refers to techniques in which intracranial targets are localized precisely in three-dimensional space for introduction of a probe for biopsy or for delivery of localized radiotherapy. The basis for construction of the stereotactic space is the rigid application of a head frame to the patient’s skull. Stereotactic imaging is then performed with a localizer fitted over the patient’s head and attached to the frame. Fiducial markers in the localizer box define x, y, and z coordinates for any location in the defined space above the frame and appear on each image obtained. Imaging may be done with either computed tomography (CT) or magnetic resonance imaging (MRI) technology, or both with “fusion” of the two imaging modalities (Fig. 25-1).
Stereotactic Radiosurgery Delivery Systems
At present, radiosurgery is performed by using one of three methods of delivery of high-energy radiation. The radiosurgery technology most commonly available around the world uses high-energy x-rays generated from a clinical linear accelerator, or “linac.” Gamma ray radiosurgery with the gamma knife, although not as widely available as linac radiosurgery, is performed at an increasing number of centers and has been used more often than the other radiosurgery technologies. Radiosurgery with charged particles (e.g., protons or heavy ions) produced by a cyclotron or synchrotron is the technology least frequently used, but may be most suitable for very large target volumes.9 Any radiosurgery treatment-planning system should provide rapid, three-dimensional visualization of the target and isodose distribution superimposed on CT scans, MRIs, or angiograms.
Gamma Knife
More than 250 gamma knife units are now located around the world, including more than 100 in the United States. As of the year 2006, more than 400,000 patients had been treated with this technology. The gamma knife unit consists of a central core containing either 192 or 201 cobalt 60 (60Co) sources surrounded by cast-iron or tungsten shields and a steel entrance door (Fig. 25-2). The sources are situated in individual beam channels in the central core directed convergently toward a single target point (isocenter) at the hollow center of the radiation unit (Fig. 25-3). Collimation of the beams is provided by collimators projecting to 4, 8, 14, or 18 mm diameter (Model 4C) or 4, 8, 16 mm (Perfexion) at isocenter (Fig. 25-4).
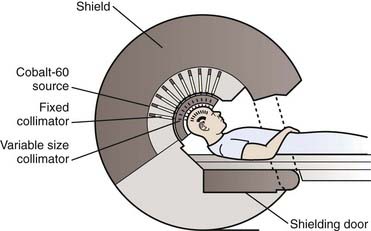
(From Lutz W: Radiation physics for radiosurgery. In Alexander E, Loeffler JS, Lunsford LD (eds): Stereotactic Radiosurgery. New York, McGraw-Hill, 1993.185)
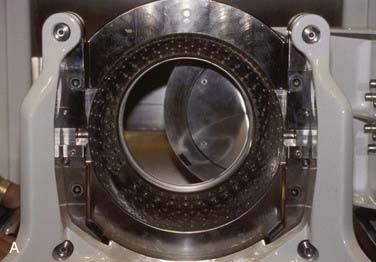
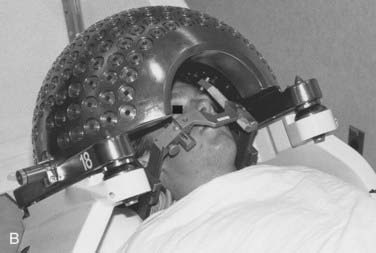
FIGURE 25-4 • A, Close-up of gamma knife helmet. The “8” designates this helmet as having collimators projecting to 8 mm at isocenter.
B, A patient positioned in a gamma knife helmet prior to entry into the gamma knife core.
After stereotactic frame placement and imaging, treatment planning consists of selection of a number of individual “shots” or radiation doses to small volumes within the target, each with a unique isocenter coordinate. Treatment plans are evaluated and optimized using dose-volume histograms (DVH). Once treatment planning is completed, the patient lies on the treatment couch, the position of which is adjusted so that the patient’s head, fixed within the stereotactic frame, is moved into the treatment position in the helmet (see Fig. 25-4). The frame is oriented within the helmet in the x, y, and z directions so that the radiation beams align with the target site according to the treatment plan. Several different isocenters may be treated in sequence during the treatment session to make the isodose surface conform closely to any irregular three-dimensional target contour (Fig. 25-5). Historically, it has required 10 to 15 minutes to place the patient in position and treat each isocenter. Modifications of the gamma knife have shortened these times considerably. Because 60Co has a half-life of 5 years, treatment times must progressively be increased.
Figs. 25-6 to 25-8 demonstrate examples of gamma knife plans for arteriovenous malformation (AVM), metastatic disease, and a benign acoustic schwannoma, respectively. In each, the dose was prescribed at the 50% isodose line (yellow), which was required to conform tightly to the target configuration (red or blue). The 25% isodose line (green) is also shown. The crosses represent projections of isocenter locations onto the MRI plane.
Linear Accelerator Radiosurgery
Like the gamma knife, linacs have a defined isocenter. The linac isocenter is the point of the intersection of the axis of rotation of the gantry and the treatment couch and the central axis of the photon beam (Fig. 25-9). Targets may be treated with single or multiple isocenter plans. Initial linac systems used special circular collimators of diameters from 5 to 60 mm to reduce the penumbra of the beam and the radiation dose to normal tissues. However, the use of circular collimators greatly limits the potential for isodose shaping, especially when using single-isocenter plans. Excellent conformity is now possible using micro-multileaf collimation (Fig. 25-10) and either multiple fixed fields or dynamic-arc radiosurgery. In the latter, the field shape is constantly changing to conform to the beam’s-eye view outline of the target volume (see Fig. 25-10). This treatment strategy has been shown to produce isodose distributions with conformity indices similar to those reported for multiple isocenter gamma knife plans.10
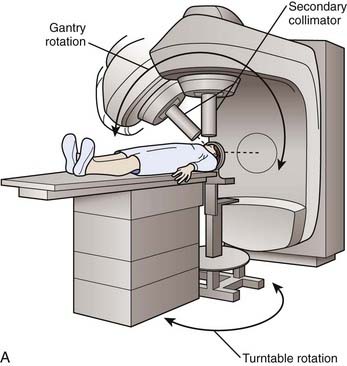
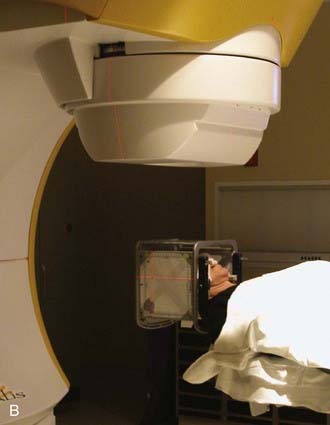
(A, From Lutz W: Radiation physics for radiosurgery. In Alexander E, Loeffler JS, Lunsford LD (eds): Stereotactic Radiosurgery. New York, McGraw-Hill, 1993.185)
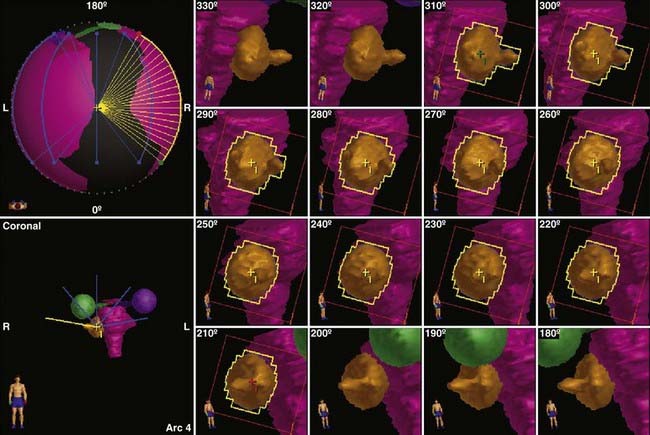
FIGURE 25-10 • Dynamic conformal arc (DCA) technique for linac radiosurgery. Right acoustic schwannoma was treated with 5 DCA. The micro-multileaf collimation (mMLC) conforms to the tumor periphery without a margin. The shape of the mMLC changes with each degree of arc. The resulting plan is shown in Fig. 25-11.
Figs. 25-11 to 25-13 demonstrate linac-based radiosurgery plans for acoustic neuroma, recurrent pituitary adenoma, and AVM. All plans employed a single isocenter.
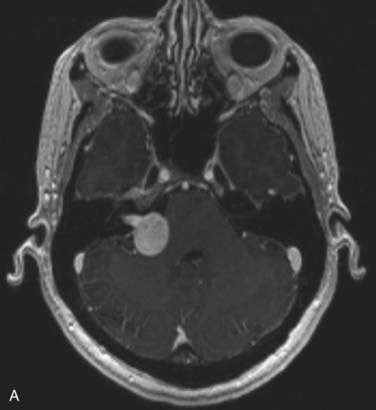
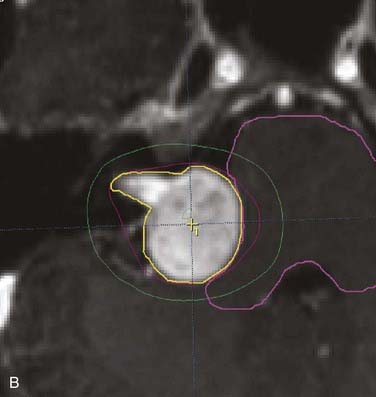
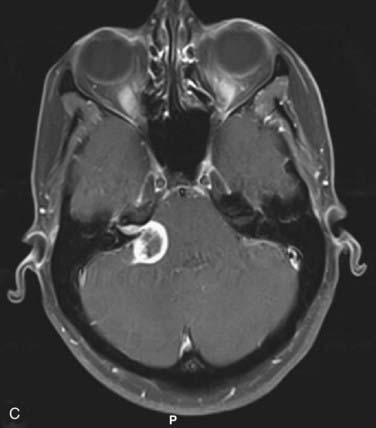
FIGURE 25-11 • A, Acoustic neuroma treated as shown in Fig. 25-10. Pretreatment magnetic resonance imaging (MRI) scan (left). The tumor volume was 4.1 cm3. B, A dose of 13 Gy was prescribed to the tumor margin corresponding to the 85% isodose line (red). The prescription isodose volume also contained 0.96 cm3 of normal tissue resulting in a conformity index of 1.3. Also shown is the isodose line corresponding to 50% of the prescription dose (green). C, A follow-up MRI scan 18 months after stereotactic radiosurgery shows smaller tumor volume and loss of central enhancement.
Further improvement in conformity of linac radiosurgery plans may be obtained using intensity-modulated delivery methods. Coupled with inverse treatment planning systems, avoidance of normal structures adjacent to the target volume is also possible.11 Clearly the future of linac-based stereotactic radiotherapy and radiosurgery lies in the ability to produce conformal treatment plans entirely covering target while using intensity modulation to avoid adjacent critical structures.12 Such treatment may often be delivered through a single isocenter and with prescription to the 80% isodose line or higher, thereby reducing dose inhomogeneity within the target.10
To align the patient’s head with respect to the linac beam, some groups have the patient lie on the treatment couch with the head supported and positioned on a separate, floor-mounted, stereotactic fixture, whereas others use only the treatment couch together with the standard alignment lasers associated with linac technology. Most groups recommend that target positioning be verified radiographically before each individual treatment. With most systems, patients are treated while they lie supine. In most cases, beam-entrance patterns trace a series of non-coplanar arcs on the scalp, one arc for each of several positions to which the table is turned.13
Particle-Beam Radiosurgery
Charged particle-beam radiosurgery has been used predominantly in North America, Europe, and the former Soviet Union during the previous 40 years, and during that time more than 6000 patients have been treated, some receiving therapy in as many as four fractions rather than one.14 In contrast to x-ray or gamma knife techniques, which require a large number of convergent radiation beams to produce a highly focal dose distribution, charged-particle beams produced by cyclotrons or synchrotrons produce a highly focal dose distribution with only a few beams because of the Bragg ionization peak produced by each individual beam. The width of the Bragg peak and its depth in relation to the scalp are adjusted to match the target’s dimensions and depth in brain. Patients usually are treated with two to six stationary, intersecting beams, each with its own irregularly shaped collimator that conforms to the contour of the target (Fig. 25-14). To ensure an acceptable three-dimensional dose distribution, compensators may be interposed in the beams to compensate for skull curvature and tissue inhomogeneities. As in linac or gamma knife radiosurgery, the three-dimensional dose distribution produced by a few intersecting particle beams falls off rapidly with distance from the target periphery, but it is usually more homogeneous within the target. Although proton radiosurgery is used to treat the common intracranial targets of photons techniques, a disproportionate number of patients with large irregular shaped lesions are referred to proton centers to achieve a greater conformality of dose. For example, at the Massachusetts General Hospital more than 50% of the AVMs treated are larger than 10 cc. This experience has provided unique data concerning dose-volume analyses for larger targets that is otherwise not available from photon radiosurgery experiences.15
Radiobiologic Effect
Usually, radiosurgery delivers little or no clinically important radiation dose beyond the target volume, in part because of the steep dose gradient at the target’s margins and in part because the minimum target isodose contour (usually 50% to 80%) conforms closely to the three-dimensional configuration of the target. Therefore, radiosurgery doses are high and inhomogeneous. The biologic effectiveness of radiation depends on the total dose of radiation, the dose per fraction, and the type of tissue irradiated. For late-responding tissues like normal central nervous system or vasculature tissue, there is a strong dependence on dose per fraction. With radiosurgery, total dose and dose per fraction are high, and variations in both according to isodose distributions produce sharply changing biologic effects. Within the target periphery, dose inhomogeneity (sharply rising dose from target margin to isocenter) has a disproportionate radiobiologic effect and may substantially elevate the potential for a cure or complication. Beyond the target’s perimeter, the radiobiologic effect declines rapidly as a result of decreasing dose and dose per fraction, a characteristic that may spare normal tissue, but that may permit the survival of potential infiltrative disease just outside the radiologically apparent target.16
Intracranial Target Lesions
Intracranial targets for radiosurgery fall into one of four categories based on two criteria: (1) whether the target tissue’s radiobiologic response to radiosurgery is early or late; and (2) whether the target volume contains any normal tissue.16
Category D
For small targets in categories A and B, it is to be anticipated that the ratio of patients cured to those with complications (therapeutic ratio) will bear little relation to the fractionation scheme used, whether single or multiple. For AVMs (category A), clinical data on fractionated treatments to a dose sufficient to test this prediction are not available. The clinical data available so far on radiosurgery for meningioma (category B) are not dissimilar to the best reported results of fractionated radiation therapy planned with CT or MRI.17–19 For metastases (category D), it might be thought that fractionated treatments would be preferable to single-fraction treatments because hypoxic tumor cells might reoxygenate and become more radiosensitive between fractions. The therapeutic ratio appears to be better with radiosurgery for metastatic lesions than with fractionated treatment, although a valid comparison is not possible because no studies have used sufficiently high fractionated doses. Why fractionation is not more important for these targets is not entirely understood, although the explanation may relate to the treatment volume. Usually, for small targets, a single fraction does not produce enough damage to normal tissue to have clinical consequences. In any case, most patients with lesions in category C and many in category D, except those with recurrent tumors, undergo fractionated irradiation as part of their therapy.
Single-fraction SRS results in tumor control rates for malignant tumors higher than predicted by modeling based on in vitro and in vivo killing of tumor cells by radiation.20 It has been proposed that, in the setting of single, high doses of radiation, the vascular endothelial cells become an additional important therapeutic target. Radiation-induced apoptosis of the endothelial cells may only become significant in single doses larger than 8 to 11 Gy.21 Similar effects likely come into play in the treatment of benign neoplasms with SRS.
Target Volume Definition
The hallmark of SRS is conformity of the prescription dose volume to the target volume. In conventional radiotherapy the gross target volume (GTV) defines radiographically visible tumor; there is often a “margin” added to this for microscopic extension, lymph nodes at risk, and so on, to form the clinical target volume (CTV); a further expansion is made to account for small, day-to-day errors in patient set-up to form the planning target volume. In SRS, the GTV is defined on stereotactic CT or MRI scan. In principle there is no need for expansion to cover subclinical disease or for set-up error. Set-up errors for all radiosurgery delivery systems are in the sub-millimeter range. Therefore, target volume definition is a crucial first step in the SRS planning process. Definition of AVM nidus or tumor volume requires the use of the appropriate imaging modality or modalities. Definition of normal structures at risk requires equal care. DVH analysis is highly recommended for evaluation of SRS plans in terms of target coverage and dose to normal structures. Various indices have been described to evaluate the degree conformity of the prescription isodose to the target volume.10,22
Radiation Dose
In all radiosurgery techniques, the volume of normal brain tissue beyond the target that receives a moderate to high dose of radiation increases approximately as the cube of the average diameter of the target volume. As complications of irradiation affecting normal tissue may correlate with the volume of normal tissue receiving a dose of a specified magnitude, at least to some extent, isoeffect dose levels may be expected to follow a power law, by which a log-log plot of isoeffective dose versus target diameter approximates a straight line with negative slope. The empirical power law dose-diameter isoeffect curve developed by Kjellberg and colleagues23 for proton radiosurgery is thought to correspond to a 1% risk for radiation necrosis of human brain tissue. The theoretical integrated logistic model dose-diameter curve developed by Flickinger24—obtained from a mathematical model that predicts the relationship of dose to volume for a 3% risk for radiation necrosis—closely resembles Kjellberg’s 1% curve for both gamma knife and linac radiosurgery. In each case, a log-log plot of isoeffective dose versus diameter approximates a straight line with negative slope. Although insufficient clinical data are available to confirm the level or slope of these curves, no doubt several variables, in addition to dose and volume, influence the probability of radiation damage. Still, Flickinger’s model can be helpful in comparing treatment plans to determine, for each particular patient, which plan has the least potential to cause complications (Fig. 25-15).
With the possible exception of AVMs and trigeminal neuralgia (see following), there are no clear dose-response data for targets treated with SRS. Most centers base doses on tumor volume and any restriction based on adjacent normal structures such as optic chiasm. Radiation Therapy Oncology Group (RTOG) 90-05 determined the maximum tolerated dose (MTD) of SRS in previously irradiated patients being treated for recurrence.25 Dose escalation was performed with stratification of patients into three categories based on maximum tumor diameter: less than 20 mm, 21 to 30 mm, and 31 to 40 mm. As expected, patients with smaller tumors tolerated higher doses. For the group with tumors smaller than 20 mm, 24 Gy was established as safe. Further dose escalation in this group was not possible because of lack of patient accrual at the 27-Gy level (presumably because of the reluctance of treating physicians to treat patients at this dose.) MTDs of 18 Gy and 15 Gy were established for patients with tumors between 21 and 30 mm and between 31 and 40 mm in diameter, respectively.
Although these results are extremely valuable and may serve as guidelines, several factors should be kept in mind when choosing SRS dose.26 First, patients in RTOG 90-05 were previously irradiated patients. Patients receiving SRS as a boost, immediately following whole-brain radiation therapy (WBRT), for example, for metastatic disease, may neither require nor tolerate the doses established as MTD in the protocol. Some clinicians decrease doses by approximately 10% when treating as a boost compared with treatment with SRS alone. Other special circumstances may also apply. For example, it is clear that acoustic neuroma is well treated with doses well below the RTOG MTDs, and that these doses would result in unnecessary toxicity (see the following text).
As complications of radiosurgical treatment have been documented more thoroughly, there has been a general reduction in dose levels prescribed. Central to the practice of radiosurgery are four general principles: (1) Slowly proliferating tissues may not manifest a response for months or years after treatment; (2) a slow response does not necessarily indicate that the lesion is radiation-resistant; (3) the latent interval can be decreased by increasing the dose delivered; and (4) response often reflects proliferative activity rather than intrinsic radiation sensitivity.27 Radiosurgical targets that are slowly proliferative include AVMs and benign tumors (meningioma, pituitary adenoma, and acoustic neuroma). Those lesions and other slowly growing tumors usually do not require a radiation dose that unduly risks damage to normal tissue to produce a rapid response or tumor necrosis.
Results of Radiosurgical Treatment
Arteriovenous Malformations
The natural history of AVMs represents a potentially life-threatening intracranial process, as is evident from the retrospective study of 160 patients with untreated AVMs (mean follow-up, 23.7 years) in which Ondra and associates28 found an annual rate of spontaneous hemorrhage of 3.9% and annual morbidity and mortality rates of 2.4% and 1.0% respectively. It appears that AVMs are cured, with little further risk of hemorrhage, when total microsurgical resection or total radiosurgical obliteration can be angiographically demonstrated.29 Because there seems to be a persistent risk of hemorrhage during the latent interval following radiosurgery, complete microsurgical excision of the AVM is the preferred therapy whenever it can be accomplished safely.30 When this is not possible, radiosurgery can obliterate both superficial AVMs and deep-seated, relatively inaccessible AVMs, with low morbidity and mortality rates and minimal hospitalization (Table 25-1). Radiosurgery induces a progressive thickening of the vascular wall and luminal thrombosis, which take months to years to complete. Reported obliteration rates are 74% to 92% at 2 to 3 years after radiosurgery (see Table 25-1).31–35 In most series, the obliteration rates at 2 years after radiosurgical treatment of small AVMs are higher (80% to 100%) than those for larger AVMs (40% to 70%).36 This has been shown to be due to the higher minimum doses delivered to smaller AVMs.37,38 Minimum doses of 13 and 25 Gy have been associated with in-field obliteration rates of 50% and 98%, respectively.38,39 The “gold standard” for success following SRS for AVM is angiographic evidence of complete obliteration. Patients may initially be followed with MRI until the nidus is no longer evident, at which time complete obliteration may be confirmed on angiogram.40 Repeat SRS for residual nidus demonstrated 2 to 3 years after SRS may result in subsequent obliteration in up to 85% of cases with low rates of morbidity.35,41,42 Likewise, an approach of repeat SRS for large AVMs may lead to improvement in obliteration rates, compared with a single session of low dose SRS.43
The rate of permanent residual neurologic injury after radiosurgery for AVM is 3% to 4%.35,42,44–46 In about one third of patients, follow-up MRIs show transient increased T2 signal changes surrounding the target that develop about 6 to 18 months after radiosurgery and resolve a year or so thereafter. These changes depend on the location and size of the target.47 Approximately one third of patients who develop these T2 signal changes also have transient neurologic symptoms.
Series treating patients younger than 18 years of age with radiosurgery for AVM have shown results comparable with those found in adults when similar doses are used. Smyth and colleagues48 reported on 40 children treated with SRS for AVM. Results were significantly correlated to marginal dose. Obliteration rates were 7% and 63% for marginal dose of less than 18 Gy and more than 18 Gy, respectively. Similarly, others have reported obliteration rates of 77% to 80% for small AVMs (<3 cc) and 61% to 65% for AVMs 3 to 10 cc in volume.49,50
Other related vascular lesions such as dural arteriovenous (AV) fistulae and cavernous malformations have also been treated with SRS. Dural AV fistulas treated in a fashion similar to AVMs (dose 10 to 28 Gy based on volume) demonstrated a 68% to 77% obliteration rate at 2-year follow-up. An additional 24% of shunts had significantly reduced flow, indicating a potential obliteration rate of up to 90% with further follow-up.51,52 Cavernous malformations have also been treated with SRS in selected “high risk” patients. Hasegawa and colleagues53 reported on 82 such patients who had experienced at least one major hemorrhage. During an average observation period of 4.3 years, the annual risk of hemorrhage was 33.9%. Following SRS (median dose 16.2 Gy), the risk of hemorrhage was reduced to 12.3% in the 2-year period after SRS and to 0.76% for years 3 through 12. Of these patients, 13% had new neurologic symptoms unrelated to hemorrhage following SRS; half of these were mild and transient. The authors recommend SRS for “high-risk” patients who have suffered at least one major hemorrhage.
Benign Tumors
Benign tumors commonly treated by radiosurgery are acoustic neuromas, meningiomas, and nonsecreting pituitary adenomas or those secreting somatotropin, adrenocorticotropin, or prolactin. Clinical observations on the radiosurgery of these lesions have provided fundamental information about the tolerance to single-fraction treatment on the part of the pituitary gland, the cranial nerves of the cavernous sinus, and the mesial temporal lobes.54–58
Acoustic Neuroma
The role of radiosurgery for acoustic neuroma as an alternative to microsurgery is a topic of lively debate. The radiosurgical progression-free survival (PFS) rate with acoustic neuromas has been reported to be more than 90%.59 The early use of high doses in the range of 20 Gy at the tumor margin resulted in high rates of transient cranial nerve V and VII neuropathies. There was also hearing loss in approximately half of patients with useful hearing at the time of SRS. Because of these toxicities and the high rate of tumor control, doses have decreased during the past 10 years (Table 25-2). Chopra and colleagues recently reported a 98.3% 10-year control rate in more than 216 patients treated with the gamma knife to marginal doses of 12 to 13 Gy.60 No patient in this group developed a new facial neuropathy. Eight patients (3.7%) developed some degree of trigeminal neuropathy At 3 years there was a 78% probability of maintaining initial Gardner-Robertson hearing level in 110 patients with testable hearing. Similar results have been obtained by other institutions,61–64 and marginal doses in the range of 12 to 13 Gy have become the standard.
Follow-up imaging following SRS for acoustic schwannoma may show early enlargement of the tumor. Pollock reported a 14% rate of enlargement at a median of 9 months following SRS.65 The majority of these tumors (86%) did not subsequently demonstrate serial progression.
Fractionated stereotactic radiotherapy or staged radiosurgery has been used to treat acoustic neuromas with very similar results as those reported for SRS. Tumor control rates of more than 90% for treatment regimens of 50 to 54 Gy in standard fractions or 20 to 35 Gy in fractions of 4 to 5 Gy have been reported with virtually no incidence of cranial neuropathy and excellent preservation of hearing rates similar to low-dose SRS.66–69
Meningioma
SRS is increasingly becoming the sole treatment or a component of the treatment of intracranial meningioma. During the previous decade there have been a number of reported series with substantial follow-up demonstrating excellent local control of benign meningioma following SRS (Table 25-3).
Stafford and colleagues reported on 184 World Health Organization (WHO) grade-1 meningiomas in 168 patients treated with SRS.70 Local control at 5 years was 93% following marginal doses of 12 to 36 (median 16 Gy). These authors found no correlation between marginal dose and tumor control. Kreil and colleagues reported on 200 patients treated with a median dose of 12 Gy (7-25 Gy) SRS for meningiomas ranging in volume from 0.38 cc to 89.8 cc.71 Local control probability was 97.2% at 10 years with a complication rate of 2.5%, including temporary symptoms relating to peritumoral edema. Kollova and colleagues from the Czech Republic recently published their series of 325 patients with a median meningioma volume of 4.4 cc (0.11-44.9 cc).72 The median marginal tumor dose was 12.6 Gy (6.5-24 Gy). Five-year actuarial tumor control was 97.9%, with 69.7% of tumors showing some regression and 27.8% remaining stable in size. A strict dose limit was set for the optic pathways at 8 Gy for previously unirradiated patients and 3 Gy for previously irradiated patients. Temporary treatment-related toxicity was reported in 10.3% of patients and permanent effects were suffered by 5.7%. However, no patient was reported to have an optic nerve-related complication. Tumor control was significantly compromised when the marginal dose was less than 12 Gy. Patients treated with marginal doses in excess of 16 Gy had a significantly increased risk of peritumoral edema, temporary or permanent sequelae of radiosurgery. The authors conclude a dose range of 12 to 16 Gy for meningiomas is optimal. The University of Florida group treated 181 patients with WHO grade I meningioma with linac-based radiosurgery. A median marginal dose of 13.14 Gy (10-20 Gy) was delivered to a median treatment volume of 7 cc (0.3-48.6 cc). The local control rate was 96% at 5 years.73
Often meningiomas are treated without biopsy, based on characteristic appearance on MRI scan. Flickinger and colleagues74 reported on a series of 219 meningiomas diagnosed by imaging criteria. Marginal doses of 8.9 to 20 (median 14) Gy were prescribed. The actuarial local control rate was 93.2% at 5 and 10 years. The probability of misdiagnosis was 2.3% at 10 years. The historical trend toward using lower marginal doses after 1991 (14 Gy versus 17 Gy) did not decrease the local control rate. However, in the later period, when stereotactic MRI was used for planning, the rate of complication was significantly lower: 5.3% versus 22.9%, when CT alone was used. This confirmed a previously reported excellent local control of 93% for meningiomas diagnosed by imaging compared with 60% for those previously resected.75
Cavernous sinus involvement with meningioma, in which complete surgical resection is not usually considered an option, presents a special situation because the optic nerve or chiasm may lie very close to tumor and patients are at high risk for either tumor- or treatment-related morbidity. The Gamma Knife group in Milan recently reported on their experience in treating 123 patients with cavernous sinus meningiomas with a mean tumor volume of 7.99 cc (0.7-30.5 cc). Tumor control was achieved in 121 (98.4%) patients with a median follow-up of 3 years. Of 73 patients with cranial nerve deficits prior to SRS, 23 (31%) improved following treatment. Five patients (4.1%) were reported to have treatment-related adverse effects. Of these, only one was permanent (CN VI palsy) occurring 8 months after radiosurgery. Optic-nerve dose was limited to 10 Gy and no patient experienced visual deterioration.76 The Pittsburgh Gamma Knife group reported on 155 patients with benign cavernous sinus meningioma, most treated with marginal doses of 12 to 14 Gy. Local control was 93.1% at 10 years with 34% of tumors regressing on follow-up imaging. Eight patients experienced adverse effects, including three patients with visual deterioration who received 12 Gy to the optic nerve.77
Pollack compared radiosurgical to surgical outcomes for patients with small to medium (<35 mm) meningiomas.78 SRS resulted in a probability of local control at 7 years of 95%, equivalent to that found following a Simpson grade 1 resection (96%). Less extensive surgery resulted in poorer outcome—82% and 34% 7-year local control for Simpson grade 2 and grades 3 to 4 resections, respectively. Complications were lower in the SRS group (10%) than in the surgical group (22%). It was suggested that SRS will likely become the preferred treatment for patients with meningiomas in this size range and without mass effect.
Based on findings available to date, radiosurgery appears to be safe and effective in producing a durable arrest of growth of small, inoperable, recurrent, or residual meningiomas. Early experience indicated that treatment of large-volume tumors or the use of high marginal doses resulted in excessive risk of treatment-related sequelae.24,79,80 Marginal doses in the range of 12 to 16 Gy appear to afford an excellent probability of tumor control and a low risk of complication. When the optic nerve or chiasm receive doses of less than 10 Gy, the risk of neuropathy is low. The risk of optic neuropathy when dose is limited to less than 8 Gy is likely less than 1%.72 Leber and colleagues reported that higher doses carry substantial risk of visual impairment that may not manifest for many years after treatment. A group of patients receiving optic nerve doses of 10 to 15 Gy had a 26.7% incidence of optic neuropathy, which increased to 77.8% in a group receiving more than 15 Gy.58 Conformal radiotherapy delivered in standard fractionation (54 Gy in 30 fractions) has been shown to result in excellent tumor control, with low rates of complications and, in particular, an extremely low risk of optic neuropathy.81,82 Fractionated therapy should be considered when optic-nerve tolerance is likely to be exceeded with SRS.83 We do not recommend SRS for the treatment of optic-nerve-sheath meningioma.
Tumor volume has been shown to strongly correlate with local control and overall survival (OS) of patients with WHO grade 1 meningioma treated with SRS. DiBiase and colleagues found 5-year disease-free survival (DFS) and OS of 86.2% and 91.0%, respectively, for 162 patients treated with SRS.84 Both DFS and OS were significantly better for patients with tumors smaller than 10 cm3 compared with those with larger tumors: 91.9% versus 68.0% (DFS) and 100% versus 59.7% (OS), respectively. Milker-Zabel and colleagues reported a 5-year local control rate of 95.7% for meningiomas of up to 60 cm3 in volume following fractionated stereotactic radiotherapy and standard fractionation (57.6 Gy in 32 fractions) compared with 84.5% for larger tumors.85 A subset of patients undergoing neuropsychiatric testing showed no decrement in function following fractionated treatment.86 So existing data indicate that, although control rates for SRS and fractionated radiotherapy are similar for smaller meningiomas, larger tumors may be better suited to fractionated radiotherapy.
Pituitary Adenoma
Nonfunctioning Tumors
Pituitary adenomas have been treated with radiosurgery for approximately 50 years using a variety of doses and techniques with excellent long-term local control.87,88 Recent large series of nonfunctioning pituitary adenomas treated with SRS report control rates of more than 90% (Table 25-4).89–91 Response rates are 60% to 90% and may continue to increase with follow-up longer than 3 years.90 Marginal doses used in these studies ranged from 5 to 35 Gy. Mingione and colleagues90 demonstrated a significant reduction in tumor-control rates for marginal doses less than 12 Gy, compared with more than 12 Gy (68.7% versus 97.3%, respectively, P < 0.05). There was no apparent benefit when marginal dose exceeded 20 Gy.
Tumor-control rates for nonfunctioning adenomas reported for SRS are equivalent to those reported following fractionated radiotherapy. Brada and colleagues reported PFS of 97% and 92% at 10 and 20 years, respectively, for 252 patients treated with conventional radiotherapy of 45 to 50 Gy.92 Milker-Zabel and colleagues93 reported a 93% 5-year PFS in 60 patients with nonfunctioning pituitary adenomas treated to a median dose of 52.2 Gy in 29 fractions with stereotactic radiotherapy. The choice of one modality over the other should be based on patient and tumor characteristics. SRS may be the preferred treatment option for patients with discrete residual tumor located at least 5 mm from the optic apparatus. Fractionated radiotherapy, used successfully for more than 50 years to treat pituitary adenomas, should be recommended for tumors abutting optic nerve or chiasm and for diffuse or large tumors.
The actuarial risk of SRS-related hypopituitarism is dose- and axis-related.89 Vladyka and coworkers reported that the mean pituitary dose is the best correlate to risk of hypopituitarism following SRS. A mean pituitary dose of 15 Gy was found to pose little risk of subsequent thyrotropic, gonadotropic, or adrenocorticotropic function. Mean doses of more than 17 Gy for gonadotropic and thyrotropic function and more than 20 Gy for adrenocorticotropic function resulted in a 50% rate of hypopituitarism at 5 years.94 Dose to the pituitary stalk and hypothalamus may also contribute to hypopituitarism following SRS.
Secretory Tumors
Pituitary adenoma hypersecreting growth hormone (GH) and causing acromegaly is a serious, disabling disease associated with reduced life expectancy. The risk of death resulting from cardiovascular and respiratory disease and malignancy have been reported to be increased two- to threefold in the acromegalic patient population. Reduction of GH levels to near-normal levels is associated with reduced risk of death. Surgery is nearly always offered as initial management of these patients, but results in a biochemical cure in only 50% to 70% of patients.95 A postoperative serum GH level in excess of 2 ng/ml is associated with a nearly threefold risk of death compared with lower levels.96
SRS for residual GH-secreting adenoma to reduce GH levels to near normal has been reported in several recent studies (Table 25-5). The Mayo Clinic group reported on 46 patients treated for acromegaly with a median pretreatment GH level of 8.4 ng/ml (0.5–82 ng/ml) and a insulin-like growth factor-I (IGF-I) level 2.25 times the upper limit of normal.97 Biochemical control was defined as a GH level of 2 ng/ml and a normal age- and sex-adjusted IGF-I with no suppressive medications. The median marginal dose was 20 Gy (14.4-30 Gy). At a median of 36 months (6-63 months) following SRS, 50% of patients achieved biochemical control. The actuarial rates of biochemical remission were 11% and 60% at 2 and 5 years, respectively. The rate of remission was strongly correlated with a pretreatment IGF-I of less than 2.25 times normal and the absence of pituitary suppressive medications at the time of SRS.
Attanasio and colleagues98 reported on 30 acromegalic patients treated with gamma knife SRS to a median dose of 20 Gy (15-35 Gy). The 5-year probability of normalized GH (<2.5 ng/ml) was 50%. IGF-I levels were normal for age and sex in 30% at 5 years. Strict biochemical cure (both GH and IGF-I normalized) was achieved in 30% of patients at 5 years. There was no case of tumor growth and 79% of tumors were decreased in size at 4 years.
Jezkova and colleagues99 reported on 96 patients with acromegaly treated with 10 to 42 Gy (35 Gy, median) SRS. For the strictest criteria of control (GH < 1.0 ng/ml on oral glucose suppression and normal IGF-I for sex and age), control rates were 14.6%, 28.6%, and 44.2% at 1, 2, and 5 years, respectively. Corresponding probabilities of reducing GH to a “safe” level (<2.5 ng/ml) were 23.9%, 47.1%, and 67.4%, respectively.
It has been suggested that SRS results in more rapid normalization of hormone hypersecretion in patients with pituitary tumors.100 Minniti and colleagues101 treated 47 acromegalic patients with fractionated radiotherapy of 45 to 50 Gy in 25 to 28 fractions. Normal suppression of GH during oral glucose tolerance test (<1 ng/ml) was demonstrated in 9%, 29%, 52%, and 77% of patients at 2, 5, 10, and 15 years, respectively. Likewise Powell and colleagues102 reported on 32 acromegalic patients treated with 45 to 54 Gy of “conventional” radiotherapy. A normal IGF-I level was found in 43.7% of these patients with a mean follow-up of 5.6 years. Long-term follow-up of 884 acromegalic patients treated with conventional radiotherapy was reported by Jenkins and colleagues and the UK Acromegaly Register Group.103 GH normalization (<2.5 ng/ml) was achieved in 22%, 36%, and 60% of patients at 2, 5, and 10 years, respectively. Corresponding IGF-I normalization was seen in 38%, 50%, and 63% of patients, respectively. These data suggest a similar rate of normalization with fractionated radiotherapy compared with SRS.
Cushing syndrome is a constellation of signs and symptoms secondary to an overproduction of adrenocorticotropic hormone (ACTH). The most common cause of Cushing syndrome is an ACTH hypersecreting pituitary microadenoma, or Cushing disease. Features of Cushing syndrome include fatigue, muscle weakness, hypertension, depression, cognitive impairment, purplish skin, striae, easy bruising, hyperpigmentation, loss of libido, diabetes, hirsutism, acne, and menstrual disorders.104 Transphenoidal resection of the microadenoma is the treatment of choice leading to remission in 65% to 90% of patients.105 Morning serum cortisol levels of greater than 5 mcg/dl requires careful monitoring. The recurrence rate in these patients is up to 25% at 10 years, and may be higher in patients younger than 25 years of age.106 For patients with persistent or recurrent disease, based on serum cortisol or urine-free cortisol levels, treatment options may include repeat surgery, bilateral adrenalectomy, medical therapy with suppressive drugs, or radiation therapy.105
Radiosurgery has been used to treat patients who have failed surgery for Cushing disease (Table 25-6).107–110 Jagannathan and colleagues reported on 90 patients treated with gamma knife radiosurgery for Cushing disease after failed surgery. Of these, 49 patients (54%) achieved remission, defined as a normal 24-hour urine-free cortisol. New hormonal deficits appeared in 20 patients (22.3%) following SRS. The Gamma Knife group in Marseilles reported on 40 patients with Cushing disease.107 At a median follow-up of 52 months, 42% of patients were in remission and 15% were reported to have new endocrinopathies. The Massachusetts General Hospital proton group recently reported their experience with single fraction SRS for 33 patients with Cushing disease.110 At a median follow-up time of 62 months, 52% of patients had achieved remission. No second tumors, optic neuropathy, or necrosis was noted. However, 52% of patients developed new pituitary deficits. Devin and colleagues108 treated 35 patients with Cushing disease with linac-based SRS. Initially 17 (49%) of patients achieved remission. However, 4 patients subsequently relapsed 17 to 64 months following SRS. Of these patients, 40% developed new endocrine deficiency.
Results treating Cushing disease with conventionally fractionated radiotherapy are strikingly similar to those published for SRS.111 Minniti and colleagues112 treated 40 patients with 45 to 50 Gy in 25 to 28 fractions for Cushing disease. Normalization of plasma cortisol was achieved in 28%, 73%, 78%, and 84% of patients at 1, 3, 5, and 10 years following radiotherapy, respectively. Hypopituitarism was observed in 62% and 76% of patients at 5 and 10 years, respectively. Estrada and colleagues113 treated 30 patients with Cushing disease with 48 to 54 Gy (1.8-2.0 Gy daily). At a median follow-up of 42 months (18-114 months), 25 of 30 patients achieved remission with an average time to remission of 18 months. The probability of remission was 44% and 83% at 1 and 3 years. No patient who achieved remission subsequently recurred. No patient suffered visual impairment, second tumor or brain necrosis. Hypopituitarism was seen in 57% of patients following radiotherapy.
Radiosurgery is an effective adjunct to surgery or in the setting of recurrence for Cushing disease. Data does not seem to indicate a more rapid response nor a higher rate of response than that reported for conventionally fractionated radiotherapy.111 The choice of radiotherapeutic modality should be made on the basis of tumor size and location. In the case of larger tumors or those abutting optic nerve or chiasm, fractionated radiotherapy is likely to be the therapy with the higher therapeutic ratio.
Studies reporting on SRS for prolactinomas report less successful outcomes. Landolt and Lomax114 treated 20 patients with prolactinoma with gamma knife radiosurgery and achieved normalized prolactin levels in only 5 (20%). They found that the rate of normalization was higher (45%) in patients who were not receiving dopamine agonists at the time of SRS, compared with those who were (0%). Pouratian and colleagues115 recently reported on 23 patients treated with gamma knife SRS for prolactinoma. At a median follow-up time of 55 months, 26% had achieved normalized prolactin levels.
Brain Metastases
The median survival for patients with symptomatic metastases to the brain is about 1 month if they remain untreated and about 3 to 6 months if they undergo conventional WBRT, irrespective of the fractionation scheme used.116 As compared with patients receiving only WBRT, those treated with surgery and radiation therapy for solitary brain metastases have a significantly longer survival (40 weeks versus 15 weeks, p < 0.01) and improved quality of life, and significantly fewer patients have recurrence at the initial metastatic site (20% versus 52%, p < 0.02).117 Systemic chemotherapy has not been shown to influence the outcome to any great extent. When patients with extracranial metastases are considered for promising therapies with biologic response modifiers, those with brain metastases are usually excluded. Therapies such as SRS aimed at control of intracranial metastases are therefore of great potential value.
Because of their biologic and physical characteristics, metastatic lesions are well suited for radiosurgery. They are usually relatively small when diagnosed, and they are often nearly spherical and radiographically distinct. They are minimally invasive and displace normal brain parenchyma circumferentially beyond the target volume, reducing the risk of radiation injury to normal tissue. Although surgery for metastases has certain advantages in that it provides immediate resolution of mass effect, provides diagnostic information if needed, and entails no risk of radiation necrosis, radiosurgery has the substantial advantage that the entire extent of disease can usually be encompassed within the treatment volume because treatment is linked directly to three-dimensional visualization of the tumor. Moreover, radiosurgery avoids the risks of hemorrhage, infection, and tumor seeding, and, as it requires minimal or no hospitalization, the costs of therapy are less than those of surgery.118 Local control can be expected in 73% to 98% of patients treated with radiosurgery. However, because many patients with metastatic cancer may have limited survival, it is more informative to refer to actuarial local control following SRS in such patients. The results of radiosurgical treatment for single brain metastases appear to be at least equivalent to those of surgery followed by WBRT (Table 25-7).
Table 25-7 Freedom from Local and Distant Failure Following Stereotactic Radiosurgery With or Without Whole-Brain Radiation Therapy for Brain Metastases
The target volume for brain metastases treated with radiosurgery is most often the enhancing tumor defined on CT or MRI scan. It has been suggested, based on pathologic examination, that brain metastases infiltrate at least 1 mm beyond gross tumor borders.119 Noel and colleagues reported a significant increase in local control when a 1-mm CTV margin was added.120 These authors routinely used a relatively low prescription dose of 14 Gy to the margin of the CTV. Therefore, the addition of a margin would have increased the dose to the GTV, likely at a steep portion of the dose-response curve. Recently, Nataf and colleagues121 found that the addition of a 2-mm margin did not improve local control or survival, but resulted in a significant increase in parenchymal complications.
Many centers use the RTOG 90-05 guidelines to assign prescription dose for brain metastases.25 These guidelines recommend “safe” doses of 15 Gy, 18 Gy, and 24 Gy for tumors smaller than 20 mm, 21 to 30 mm, and 31 to 40 mm diameter, respectively. It is unclear if doses larger than 20 Gy for small metastases result in superior local control.122,123
Actuarial local control rates 1 year following SRS alone in treating single or multiple metastases range from 68% to 91%.122,124–128 In a multicenter study reported by investigators from several gamma knife facilities, 116 patients were treated with gamma knife radiosurgery for single brain metastases (mean minimal dose, 17.9 Gy).128 Local control of tumor was achieved in 99 patients (85%). Among all patients, the 1- and 2-year actuarial tumor control rates were 75% and 67%, respectively, with a plateau in the curve at 18 months. These findings suggested that, for most patients treated, local control rates were durable. Multivariate analysis showed that the patients with better local control were those who underwent WBRT in addition to radiosurgery. Local control rates at 1 year were 81.2% and 68% for patients receiving WBRT and those receiving SRS alone, respectively. Paradoxically, 42 patients with brain metastases of histologies reputed to be resistant to fractionated radiation therapy (melanoma and renal cell carcinoma) also had improved local control: 100% at 2 years compared with 51.5% for other histologies, a finding also reported by others.129 Median survival from the time of radiosurgery was 11 months. Patients with breast cancer had a significantly superior median survival of 18 months.
In a single-institution, randomized, prospective trial Kondziolka and colleagues130 examined the relative efficacy of WBRT (30 Gy in 12 fractions) alone compared with the same WBRT plus SRS. Patients with two to four brain metastases with known histology were eligible. Twenty-seven patients were accrued before stopping rules were met. Local failure at 1 year was 100% for WBRT alone versus 8% with the addition of SRS. There was no significant difference in OS between the groups.
RTOG 95-08 has also studied the benefit of WBRT (37.5 Gy in 15 fractions) compared with WBRT plus a boost with SRS in patients with one, two, or three brain metastases.131 At 1 year the local control rates were 82% and 71% for WBRT and SRS combined and WBRT alone (p = 0.01), respectively. For patients with single metastases, a significant survival benefit was demonstrated for the addition of SRS (6.5 versus 4.9 months). Similar significant survival advantages of 1.6 to 2 months were demonstrated for patients in RTOG recursive partitioning analysis (RPA) class I,132 patients younger than 50 years of age, and those with non–small cell lung cancer, regardless of number of metastases. Karnofsky performance status (KPS) was improved or stable at 6 months in 43% versus 27% in SRS-treated patients versus those receiving WBRT alone (p = 0.03), respectively. There was no statistical difference in the rate of grade 3 or 4 toxicities between the groups.
The addition of WBRT to SRS for treatment of brain metastases has universally been shown to improve local control122,124,126,128,133 and to decrease the risk of new metastases developing elsewhere in the brain.124,136 Fuller and associates133 reported significantly improved local control within the radiosurgical target region or elsewhere in the brain for patients undergoing SRS plus WBRT as compared with those who received only SRS, although there was no significant difference in survival rates for the two groups. Similarly, Flickinger and associates128 showed that significantly fewer patients receiving whole-brain irradiation plus radiosurgery had recurrence at the radiosurgical target site.
The Japanese Radiation Oncology Group randomized 132 patients with one to four brain metastases smaller than 3 cm in diameter to SRS alone or WBRT (30 Gy in 10 fractions) and SRS.124 SRS dose was determined by tumor size and treatment arm: 22 to 25 Gy for tumors smaller than 2 cm and 18 to 20 Gy for tumors 2 to 3 cm in the SRS alone arm and 30% less dose for the WBRT and SRS arm. OS was 8.0 months in the SRS alone group and 7.5 months in the group receiving WBRT (p = 0.42). Recurrence rates anywhere in the brain at 1 year were 46.8% for those receiving WBRT and 76.4% following treatment with SRS alone (p = 0.02).
These results are parallel to those found for the addition of WBRT to surgery for single brain metastases. Patchell and colleagues134 found that the addition of high-dose WBRT (50.4 Gy) decreased failure at the surgical site from more than 75% to less than 20% at 1 year and decreased failure elsewhere in the brain from more than 50% to less than 20%. However, the addition of WBRT to surgery or SRS has not been demonstrated to increase OS, given that effective salvage therapies are available (WBRT, further SRS or surgery or combinations thereof).126,134 Lively debate continues as to whether the demonstrated benefits of WBRT outweigh the potential associated toxicity.135 WBRT has been reported to reduce the risk of neurologic death following surgical resection of single brain metastases.134 Patients with brain metastases have been shown to have impaired neurocognitive function before receiving WBRT.136 A prospective study from Germany found similar results in patients studied prior to WBRT and also reported that after minimum follow-up of 9 months after WBRT, these patients showed no significant decline in any tested neurocognitive function.137 Although Regine and colleagues138 reported no significant change in neurocognitive testing among a cohort of patients with controlled brain metastases following WBRT; there was a dramatic and significant decline in patients treated on the same study in whom brain metastases were uncontrolled. Aoyama and colleagues124 found no difference between the arms receiving SRS alone or with WBRT in survival, death from neurologic causes, neurocognitive function, or treatment-related toxicities.
However, it is argued that WBRT may be omitted without compromising patient survival or control of intracranial disease with close follow-up and salvage therapy.125,126,139 Using this approach, more than 70% of patients initially managed with SRS alone may be spared WBRT.
In a series of 421 metastatic lesions in 248 patients who were treated at one center during a 7-year period, Alexander and colleagues140 reported that control rates for lesions with radiation-resistant histologic findings were statistically equal to those of other lesions. Several studies have found that “radioresistant” histologies are less well controlled with SRS than other histologies. A recent Eastern Cooperative Oncology Group Study of 31 patients with melanoma (14), renal cell carcinoma (14), or sarcoma (3) found a local control rate of 67.8% at 6 months following SRS. Chang and colleagues141 reported 1-year local control rates of 64%, 47%, and 0% for metastases from renal cell carcinoma (n = 77), melanoma (n = 103), and sarcoma (n = 9), respectively. Recently reported control rates at 1 year for melanoma range from 47% to 81%.141–144 For renal cell carcinoma, 1-year local control rates of 64% to 93% have been reported.141,145–147 Differences seen among studies may be due to SRS dose used or differences in definition of local failure in these histologies prone to hemorrhage.
Within the range of doses commonly used for radiosurgery, there appears to be no clear-cut dose-response relationship, and the range of doses used does not appear to correlate with outcome. Most radiosurgical teams prefer to use a lower dose for larger lesions to minimize the risk of complications. Therefore, the response rate can be expected to correlate with target size. Among reported series, complete response was achieved in 78% of patients with lesions with tumor volumes less than 2 cm3 and in 50% of those with tumor volumes 10 cm3 or greater, and subsequent progression occurred in only 4% of complete responders versus 20% of partial responders.148 In another series,140 tumor volume greater than 3 cm3 was significantly associated with higher rates of local failure in univariate analysis, although in multivariate analysis the association was not significant (p = 0.06).
Many groups omit WBRT and use SRS alone in many circumstances. Sneed and colleagues retrospectively reviewed the outcome of 62 patients treated with SRS alone versus 43 patients treated with SRS plus WBRT for single or multiple brain metastases.126 Patients receiving SRS alone were at significantly greater risk for developing new brain metastases. Salvage therapy in these patients was either repeat SRS or WBRT. Overall, 74% of patients in the SRS-alone group were spared WBRT. There was no difference in OS between the groups. In general, most patients who undergo radiosurgery for lesions metastatic to the brain experience clinical improvement and reduced steroid requirements, and only 11% to 25% eventually die of neurologic causes.124,131,133,148
Glioblastoma Multiforme
Although surgical resection followed by irradiation and chemotherapy lengthens the survival of patients who have glioblastoma multiforme (GBM), most patients die as a result of persistence or local recurrence of their tumor.149,150 Reirradiation of focally recurrent malignant gliomas using stereotactic brachytherapy has resulted in durable local control and median survival of 12 months.151,152 Radiosurgery for focally recurrent GBM has resulted in median survival times of 8 to 10.2 months (Table 25-8).152–155
For highly selected patients with small primary glioblastomas—a minority of the patients affected with this tumor—a highly conformal brachytherapy boost was shown to provide a longer than expected median survival of 20 months following conventional fractionated radiation therapy in a single-institution trial.156 Because conformal dose distributions of radiosurgery and brachytherapy are similar, it was proposed that radiosurgery may provide an effective therapeutic boost and may have a similar potential for improving survival. Single-institution studies have reported longer-than-expected survival in highly selected patients with glioblastoma treated with standard surgery and radiotherapy followed by a boost with SRS to the enhancing tumor volume (Table 25-9).157–159 These studies reported median survivals of 16 to 19.9 months and 2-year survivals of 36% to 40%. A large multi-institutional review found a median survival of 22.1 months and 2-year survival of 45%.160 These findings were especially striking when comparisons were made based on RTOG RPA class.161
However, a larger prospective RTOG study failed to find any survival benefit for patients treated with SRS boost before standard postoperative radiotherapy. The ROTG 95-03 randomized patients with unifocal GBM smaller than 4 cm in greatest dimension to standard postoperative radiotherapy and chemotherapy or SRS treatment to residual enhancing tumor followed by standard radiotherapy and chemotherapy.162 Median survival times were not different between these two randomizations: 13.6 months versus 13.5 months, respectively. No subgroup analyzed demonstrated a survival benefit from upfront SRS. Of first failures, 62% were local only and 25% of patients randomized to radiotherapy alone received SRS or reirradiation for salvage at the time of failure.
Larson and colleagues163 performed multivariate analysis of 189 patients with WHO grades 1 through 4 glioma treated with the gamma knife. These investigators explored a broad spectrum of criteria for patient selection and treatment. The main endpoints of their study were survival and complications, and the analysis was designed to determine factors that were associated with better or worse outcomes. Multivariate analysis identified five variables significantly associated with decreased survival: higher pathologic tumor grade, older age of the patient, lower KPS score, larger tumor volume, and multifocal versus unifocal tumor. It is likely that variations in published reports regarding survival rates after radiosurgery or brachytherapy for malignant glioma can be attributed, in part, to substantial differences in patient populations with respect to these five variables. Patients with unfavorable constellations of these variables are unlikely to benefit from radiosurgery.
Radiosurgery for Functional Disorders
Radiosurgery has been used for the treatment of functional disorders for nearly 5 decades.164 Such treatment usually requires extremely high doses (up to 180 Gy) to small, anatomically defined volumes. Treatment of Parkinson disease–related tremor or dystonia, intractable chronic pain, epilepsy, or obsessive-compulsive disorder are areas of current investigation.165
SRS for trigeminal neuralgia is by far the most commonly practiced and accepted form of functional radiosurgery. A multi-institutional gamma knife study demonstrated that doses of more than 70 Gy of SRS delivered to the trigeminal nerve root entry zone adjacent to the pons resulted in a significantly greater probability of complete pain relief than lower doses, 72% versus 9%, respectively.166 Goss and colleagues167 reported on 25 patients treated with 90 Gy using a linac-based system. All 25 patients treated experienced excellent or good pain relief at a median of 2 months from SRS. Relapse was experienced in 32% of patients 4 to 13 months following SRS. Longhi and colleagues168 recently reported on a series of 160 patients treated with gamma knife SRS for trigeminal neuralgia. Of these patients, 90% experienced good or excellent pain relief at a median time of 45 days. Recurrence was noted in 18% of patients at a median time of 14.2 months. Complications were more common in patients treated with doses in excess of 90 Gy and durable pain relief was significantly associated with doses of more than 80 Gy.
Normal-Tissue Tolerance to Radiosurgery
With increasing international experience with SRS, guidelines for reducing the risk of normal-tissue toxicity have emerged (Table 25-10). In general, normal tissues at risk depend on the location of the target volume. These include the brain parenchyma (edema, necrosis), brainstem (edema, necrosis, neuropathy), cranial nerves (neuropathy), and hypothalamic-pituitary axis (hypopituitarism). The interaction of dose and volume irradiated has not been clearly defined for most structures at risk.
Table 25-10 Recommended Normal Structure Dose Constraints to Avoid Complications Using a Single Fraction
Structure | Single-Fraction Dose Constraint | Reference # |
---|---|---|
Intraparenchymal brain lesion ≤ 2 cm | ≤24 Gy (MTD unknown) | 25 |
Brain lesion > 2 cm and ≤ 3 cm | ≤18 Gy | |
Brain lesion > 3 cm and ≤ 4 cm | ≤15 Gy | |
Brainstem | ≤16 Gy Dmax | 176 |
Pituitary | ≤15 Gy (mean) | 94 |
Optic chiasm and optic nerves | ≤8-10 Gy | 57, 58, 172 |
Cranial nerves III, IV, VI |
MTD, Maximum tolerated dose; WBRT, whole-brain radiation therapy.
Dose constraint is a maximum unless otherwise specified.
The risk of treatment-related edema and necrosis following radiosurgery has been correlated with SRS dose and volume irradiated. Kjellberg and Abe169 first introduced the concept of the risk of postradiosurgery necrosis being related to dose and volume. They reported a log-log relationship of dose and volume that could define levels of risk between 1% and 99%. This model did not account for dose-volume effects outside the treatment volume. Flickinger170 described the integrated logistic formula, which accounted for the contribution of all isodose volumes to the risk for complication. Subsequently Flickinger and colleagues correlated the risk of asymptomatic or symptomatic T2 MRI changes following SRS for AVM to the volume having received 12 Gy.171
The RTOG Protocol 90-05 was designed to determine the MTD of radiosurgery for patients with malignant brain tumors or brain metastases who had failed prior conventional radiotherapy. Recommended dose levels found to result in less than 20% risk of serious complication were 15 Gy, 18 Gy, and 24 Gy for tumors smaller than 20 mm, 21 to 30 mm, and 31 to 40 mm, respectively.25
Cranial nerve damage following SRS can result in blindness, ophthalmoplegia, facial weakness, numbness, or hearing loss. The special sensory cranial nerves II and VIII are apparently more sensitive to radiation than the motor nerves. Tishler and colleagues57 found that 24% of patients whose optic nerves received more than 8 Gy suffered visual loss, compared with none who received lower doses. These results were largely supported by Leber and colleagues58 who demonstrated a dose response for optic neuropathy following SRS, finding the actuarial risk to be 0%, 26.7%, and 77.8% for doses of less than 10 Gy, 10 to 15 Gy, and more than 15 Gy, respectively. Stafford and colleagues172 reported on patients treated for cavernous sinus meningioma and found a less than 2% risk of optic neuropathy for nerves receiving less than 10 Gy and 6.9% for those receiving more than 12 Gy.
Early experience with SRS for acoustic neuroma using high-prescription doses of 18 to 20 Gy resulted in a high incidence of hearing loss (49%), facial weakness (21%), and trigeminal neuropathy (27%).59 The subsequent change in practice to reduce the marginal tumor dose to 12 to 13 Gy has resulted in no compromise of tumor control but much lower rates of hearing loss (26%), facial weakness (0%), and trigeminal neuropathy (5%).60 Increased incidence of hearing loss has been associated with relatively low doses (5.3 Gy) to the cochlea.173
Lower cranial nerves are less well studied. Zhang and colleagues174 reported on 27 patients treated for jugular foramen schwannoma using marginal doses of 9.8 to 20 Gy and maximum doses of 25.4 to 50 Gy. No patient suffered a new cranial neuropathy of cranial nerves IX through XII. Martin and colleagues175 treated 36 patients with jugular foramen schwannomas with marginal SRS doses of 12 to 18 Gy (maximum dose 21.4-36 Gy). No patient suffered an SRS-related neuropathy.
Meeks and colleagues176 modeled the risk of cranial nerve dysfunction and brainstem DVH. They found that a maximum brainstem dose in excess of 16 Gy was the most important risk factor for delayed cranial nerve deficit. The model predicted a risk of cranial nerve deficit less than 5% if one third or less of the brainstem received less than 16 Gy. Tolerance is diminished for larger volumes.
Leber and colleagues58 reported no cranial neuropathies related to cranial nerves III through VI in 210 nerves receiving up to 30 Gy with long-term follow-up. Tishler and colleagues57 reported an incidence of 12% neuropathy for cranial nerves III through VI receiving doses of 15 to 40 Gy without a demonstration of a dose response.
Pituitary tolerance to SRS has been described by Vladyka and colleagues.94 A strong dose-response was demonstrated. For the gonadotropic axis, dysfunction was found in 60% of patients for mean pituitary doses of more than 17 Gy. No patient receiving less than 15 Gy demonstrated dysfunction with follow-up of up to 90 months. Similarly, thyrotropic dysfunction was found in 85% of patients with long-term follow-up following mean doses of more than 17 Gy and no patients receiving less than 15 Gy. For adrenocorticotropic function, the risk was found to be 85% for doses larger than 20 Gy, compared with 10% when the mean pituitary dose was less than 20 Gy. No incidence of adrenocorticotropic dysfunction was found when the mean pituitary dose was 18 Gy or less. In general, pituitary function was maintained in all axes when the mean dose did not exceed 15 Gy.
Summary
Radiosurgical facilities have been established in many community hospitals and most academic medical centers, and the number of patients treated with radiosurgery is increasing every year. The number of cooperative group trials of radiosurgical applications is also increasing, and changes in practice patterns are occurring and are likely to continue to evolve. Radiosurgery is being incorporated into staged treatment protocols, together with embolization, surgery, and radiation therapy; and it is being used as immediate adjuvant therapy to prevent recurrence, in preference to observation followed by treatment at recurrence.177
Also resulting from such work are certain generalizations that define radiosurgery. It has been established that the larger the target volume, the smaller the dose that can be delivered safely,178 and the lower the probability of achieving control or obliteration of the target lesion. It is known that the development of symptomatic radiation necrosis is related to the dose, volume, site, and histology of the target lesion, and that cranial nerves II and VIII are particularly vulnerable to damage by radiation. Radiosurgery is also especially damaging to late-responding tissue such as abnormal blood vessels, which accounts for the high overall response to radiosurgical treatment of vascular tumors and AVMs. Tentatively, certain conclusions seem justified:





1 Barnett GH, Linskey ME, Adler JR, et al. Stereotactic radiosurgery—an organized neurosurgery-sanctioned definition. J Neurosurg. 2007;106:1-5.
2 Wilson RR. Radiological use of fast protons. Radiology. 1946;47:487-491.
3 Tobias CA, Lawrence JH, Born JL, et al. Pituitary irradiation with high-energy photon beams: A preliminary report. Cancer Res. 1958;18(2):121-134.
4 Levy RP, Fabrikant JI, Frankel KA, et al. Heavy-charged-particle radiosurgery of the pituitary gland: Clinical results of 840 patients. Stereotact Funct Neurosurg. 1991;57:22-35.
5 Leksell L. The stereotaxic method and radiosurgery of the brain. Acta Chir Scand. 1951;102:316.
6 Larson DA, Bova F, Eisert D. Consensus statement on stereotactic radiosurgery and quality improvement. Int J Radiat Oncol Biol Phys. 1994;28:527.
7 Larson DA, Bova F, Eisert D, et al. Current radiosurgery practices: results of an ASTRO survey. Int J Radiat Oncol Biol Phys. 1994;28:523.
8 Lunsford LD, Alexander EIII, Chapman P. Consensus statement on stereotactic radiosurgery. Neurosug. 1994;34:193.
9 Silander H, Pelletieri L, Montelius A, et al. Fractionated, stereotactic proton beam treatment of cerebral arteriovenous malformations. Acta Neurol Scand. 2004;109:85-90.
10 Hazard L, Wang B, Skidmore TB, et al. Conformity of LINAC-based stereotactic radiosurgery using dynamic conformal arcs and micro-multileaf collimator. Int J Radiat Oncol Biol Phys. 2009;73(2):562-570.
11 Jensen RJ, Wendland MM, Chern S, et al. Novalis intensity-modulated radiosurgery: Methods for pretreatment planning. Neurosurgery. 2008;62:A2-A9.
12 Nakamura JL, Pirzkall M, Carol M, et al. Comparison of intensity-modulated radiosurgery to gamma knife for challenging skull base lesions. Int J Radiat Oncol Biol Phys. 2001;51:125.
13 Kooy HM. Linear accelerator in stereotactic radiosurgery. In: Alexander EIII, Loeffler JS, Lunsford LD, editors. Stereotactic Radiosurgery. New York: McGraw-Hill; 1993:67-76.
14 Levy RP, Fabrikant JI, Frankel KA, et al. Charged particle radiosurgery of the brain. Neurosurg Clin N Am. 1990;1:955.
15 Barker FG, Butler WE, Lyons S, et al. Dose-volume prediction of radiation-related complications after proton beam radiosurgery for cerebral arteriovenous malformations. J Neurosurg. 2003;99:254-263.
16 Larson DA, Flickinger JC, Loeffler JS. The radiobiology of radiosurgery. Int. J. Radiation Oncology Biol Phys. 1993;25(3):557-561.
17 Goldsmith BJ, Wara WM, Wilson CB, et al. Postoperative irradiation of subtotally resected meningiomas. J Neurosurg. 1994;80:195.
18 Hakim R, Alexander EIII, Loeffler JS, et al. Results of linear accelerator-based radiosurgery for intracranial meningiomas. Neurosurgery. 1998;42:446-454.
19 Kondziolka D, Mathieu D, Lunsford LD, et al. Radiosurgery as definitive management of intracranial meningiomas. Neurosurgery. 2008;62:53-60.
20 Brown JM, Koong AC. High-dose single fraction radiotherapy: exploiting new biology? Int J Radiat Oncol Biol Phys. 2008;71(2):324-325.
21 Garcia-Barros M, Paris F, Cordon-Cardo C, et al. Tumor response to radiotherapy regulated by endothelial cell apoptosis. Science. 2003;300:1155-1159.
22 Paddick I. A simple scoring ratio to index the conformity of radiosurgical treatment plans. J Neurosurg. 2000;93:219-222.
23 Kjellberg RN, Hanamura T, Davis KR, et al. Bragg-peak proton-beam therapy for arteriovenous malformations of the brain. N Engl J Med. 1983;309:269-274.
24 Flickinger JC. An integrated formula for prediction of complications from radiosurgery. Int J Radiat Oncol Biol Phys. 1989;17(4):879-885.
25 Shaw E, Scott C, Souhami L, et al. Single dose radiosurgical treatment of recurrent previously irradiated primary brain tumors and brain metastases: final report of RTOG protocol 90-05. Int J Radiat Oncol Biol Phys. 2000;47:291-298.
26 Flickinger JC, Kondziolka D, Lunsford LD. Dose selection in stereotactic radiosurgery. Neurosug Clin N Am. 1999;10:271-280.
27 Larson DA, Flickinger JC, Loeffler JS. Stereotactic radiosurgery: techniques and results. In: DeVita VT, Hellman S, Rosenberg SA, editors. Cancer: principles and practice of oncology updates. Philadelphia: Lippincott; 1993:1.
28 Ondra SL, Troupp H, George ED, et al. The natural history of symptomatic arteriovenous malformations of the brain: a 24-year follow-up assessment. J Neurosurg. 1990;73:387-391.
29 Stein BM, Sisti MB, Kader A. Microsurgery and radiosurgery in small AVMs. J Neurosurg. 1993;79:795.
30 Karlsson B, Lax I, Soderman M. Risk for hemorrhage during the 2-year latency period following gamma knife radiosurgery for arteriovenous malformations. Int J Radiat Oncol Biol Phys. 2001;49:1045-1051.
31 Fabrikant JI, Levy RP, Steinberg GK, et al. Charged particle radiosurgery for intracranial arteriovenous malformations. Neurosug Clin N Am. 1992;3:99.
32 Friedman WA, Bova FJ. Linear accelerator radiosurgery for arteriovenous malformations. J Neurosurg. 1992;77:832-841.
33 Bollet MA, Anxionnat R, Buchheit I, et al. Efficacy and morbidity of arc-therapy radiosurgery for cerebral arteriovenous malformations: A comparison with natural history. Int J Radiat Oncol Biol Phys. 2004;58(5):1353-1363.
34 Flickinger JC, Kondziolka D, Maitz AH, et al. An analysis of dose-response for arteriovenous malformation radiosurgery and other factors affecting obliteration. Radiother Oncol. 2002;63:347-354.
35 Liscak R, Vladyka V, Simonova G, et al. Arteriovenous malformations after Leksell Gamma Knife radiosurgery: rate of obliteration and complications. Neurosurgery. 2007;60:1005-1016.
36 Miyawaki L, Dowd C, Wara W, et al. Five year results of linac radiosurgery for arteriovenous malformations: outcome for large AVMs. Int J Radiat Oncol Biol Phys. 1999;44(5):1089-1106.
37 Loeffler JS, Alexander E, Siddon RL, et al. Stereotactic radiosurgery for intracranial arteriovenous malformations using a standard linear accelerator. Int J Radiation Oncology Biol Phys. 1989;17(3):673-677.
38 Karlsson B, Lax I, Soderman M. Can the probability for obliteration after radiosurgery for arteriovenous malformations be accurately predicted? Int J Radiat Oncol Biol Phys. 1999;43(2):313-319.
39 Flickinger JC, Pollack BE, Kondziolka D, et al. A dose-response analysis of arteriovenous malformation obliteration after radiosurgery. Int J Radiat Oncol Biol Phys. 1996;36:873-879.
40 Nagaraja S, Lee KJ, Coley C, et al. Stereotactic radiosurgery for brain arteriovenous malformations: Quantitative MR assessment of nidal response at 1 year and angiographic factors predicting early obliteration. Neuroradiology. 2006;48:821-829.
41 Karlsson B, Kihlstrom L, Lindquist C, et al. Gamma knife radiosurgery for previously irradiated arteriovenous malformations. Neurosurgery. 1998;42(1):1-6.
42 Pollack BE, Gorman DA, Coffey RJ. Patient outcomes after arteriovenous malformation radiosurgical management: results based on a 5- to 14-year follow-up study. Neurosurgery. 2003;52:1291-1297.
43 Karlsson B, Jokura H, Yamamoto M, et al. Is repeated radiosurgery an alternative to staged radiosurgery for very large arteriovenous malformations? J Neurosurg. 2007;107:740-744.
44 Lindquist C, Steiner L. Stereotactic radiosurgical treatment of arteriovenous malformations of the brain. In: Lunsford LD, editor. Modern Stereotactic Neurosurgery. Boston: Martinus Nijhoff; 1988:491.
45 Steiner L, Lindquist C, Adler JR, et al. Clinical outcome of radiosurgery for cerebral arteriovenous malformations. J Neurosurg. 1992;77:1-8.
46 Lunsford LD, Kondziolka D, Flickinger JC, et al. Stereotactic radiosurgery for arteriovenous malformations. J Neurosurg. 1991;75:517.
47 Flickinger JC, Lunsford LD, Kondziolka D, et al. An analysis of neurodiagnostic imaging changes following gamma knife radiosurgery for arteriovenous malformations. Int J Radiat Oncol Biol Phys. 1992;23:19.
48 Smyth MD, Sneed PK, Ciricillo SF, et al. Stereotactic radiosurgery for pediatric intracranial arteriovenous malformations: The University of California at San Francisco experience. J Neurosurg. 2002;97:48-55.
49 Levy EI, Niranjan A, Thompson TP, et al. Radiosurgery for childhood intracranial arteriovenous malformation. Neurosurgery. 2000;47:834-842.
50 Reyns N, Blond S, Gauvrit JY, et al. Role of radiosurgery in the management of cerebral arteriovenous malformations in the pediatric age group: Data from a 100-patient series. Neurosurgery. 2007;60:268-276.
51 Soderman M, Edner G, Ericson K, et al. Gamma knife surgery for dural arteriovenous shunts: 25 years of experience. J Neurosurg. 2006;104:867-875.
52 O’Leary S, Hodgson TJ, Coley SC, et al. Intracranial dural arteriovenous malformations: Results of stereotactic radiosurgery in 17 patients. Clin Oncol (R Coll Radiolo). 2002;14(2):97-102.
53 Hasegawa T, McInerney J, Kondziolka D, et al. Long-term results after stereotactic radiosurgery for patients with cavernous malformations. Neurosurgery. 2002;50(6):1190-1198.
54 Mehta MP, Kinsella TJ. Cavernous sinus cranial neuropathies: is there a dose-response relationship following radiosurgery? Int J Radiat Oncol Biol Phys. 1993;27:477-480.
55 Morita A, Coffey RJ, Foote RL, et al. Risk of injury to cranial nerves after gamma knife radiosurgery for skull base meningiomas: experience in 88 patients. J Neurosurg. 1999;90:42-49.
56 Sheehan JM, Vance ML, Sheehan JP, et al. Radiosurgery for Cushing’s disease after failed transsphenoidal surgery. J Neurosurg. 2000;93:738-742.
57 Tishler RB, Loeffler JS, Lunsford LD, et al. Tolerance of cranial nerves of the cavernous sinus to radiosurgery. Int J Radiation Oncology Biol Phys. 1993;27(2):215-221.
58 Leber KA, Bergloff J, Pendl G. Dose-response tolerance of the visual pathways and cranial nerves of the cavernous sinus to stereotactic radiosurgery. J Neurosurg. 1998;88:43-50.
59 Kondziolka D, Lunsford LD, McLaughlin MR, et al. Long-term outcomes after radiosurgery for acoustic neuromas. N Engl J Med. 1998;339:1426-1433.
60 Chopra R, Kondziolka D, Niranjan A, et al. Long-term follow-up of acoustic schwannoma radiosurgery with marginal tumor doses of 12 to 13 Gy. Int J Radiat Oncol Biol Phys. 2007;68(3):845-851.
61 Miller RC, Foote RL, Coffey RJ, et al. Decrease in cranial nerve complications after radiosurgery for acoustic neuromas: prospective study of dose and volume. Int J Radiat Oncol Biol Phys. 1999;43:305-311.
62 Friedman WA, Bradshaw P, Myers A, et al. Linear accelerator radiosurgery for vestibular schwannomas. J Neurosurg. 2006;105:657-661.
63 Petit JH, Hudes RS, Chen TT, et al. Reduced-dose radiosurgery for vestibular schwannomas. Neurosurgery. 2001;49:1299-1307.
64 Hasegawa T, Fujitani S, Katsumata S, et al. Stereotactic radiosurgery for vestibular schwannomas: analysis of 317 patients followed more than 5 years. Neurosurgery. 2005;57(2):257-265.
65 Pollock BE. Management of vestibular schwannomas that enlarge after stereotactic radiosurgery: treatment recommendations based on a 15 year experience. Neurosurgery. 2006;58:241-248.
66 Varlotto JM, Shrieve DC, Alexander EIII, et al. Fractionated stereotactic radiotherapy for the treatment of acoustic neuromas: Preliminary results. Int J Radiat Oncol Biol Phys. 1996;36:141-145.
67 Williams JA. Fractionated stereotactic radiosurgery for treatment of acoustic neuromas, Stereotact Funct. Neurosurg. 1999;73:45-49.
68 Koh ES, Millar BA, Michaels H, et al. Fractionated stereotactic radiotherapy for acoustic neuroma. Cancer. 2007;109:1203-1210.
69 Fuss M, Debus J, Lohr F, et al. Conventionally fractionated stereotactic radiotherapy (FSRT) for acoustic neuromas. Int J Radiat Oncol Biol Phys. 2000;48(5):1381-1387.
70 Stafford SL, Pollack BE, Foote RL, et al. Meningioma radiosurgery: tumor control, outcomes, and complications among 190 consecutive patients. Neurosurgery. 2001;49:1029-1038.
71 Kreil W, Luggin J, Fuchs I, et al. Long term experience of gamma knife radiosurgery for benign skull base meningiomas. J Neurol Neurosurg Psychiat. 2005;76:1425-1430.
72 Kollova A, Liscak R, Novotny J, et al. Gamma knife surgery for benign meningioma. J Neurosurg. 2007;107:325-336.
73 Friedman WA, Murad GJ, Bradshaw P, et al. Linear accelerator surgery for meningiomas. J Neurosurg. 2005;103:206-209.
74 Flickinger JC, Kondziolka D, Maitz AH, et al. Gamma knife radiosurgery of image-diagnosed intracranial meningioma. Int J Radiat Oncol Biol Phys. 2003;56(3):801-806.
75 Kondziolka D, Flickinger JC, Perez JC. Judicious resection and/or radiosurgery for parasagittal meningiomas: outcomes from a multicenter review. Neurosurgery. 1998;43(3):405-413.
76 Frankel A, Vimercati A, Medone M, et al. Neurophthalmological evaluation after gamma knife surgery for cavernous sinus meningioma. Neurosurg Focus. 2007;23(6):E10.
77 Lee JYK, Niranjan A, McInerney J, et al. Stereotactic radiosurgery providing long-term tumor control of cavernous sinus meningiomas. J Neurosurg. 2002;97:65-72.
78 Pollack BE, Stafford SL, Utter A, et al. Stereotactic radiosurgery provides equivalent tumor control to Simpson grade 1 resection for patients with small- to medium-size meningiomas. Int J Radiat Oncol Biol Phys. 2003;55(4):1000-1005.
79 Engenhart R, Kimmig BN, Hover K, et al. Stereotactic single high dose radiation therapy of benign intracranial meningiomas. Int J Radiation Oncology Biol Phys. 1990;19(4):1021-1026.
80 Kondziolka D, Lunsford LD, et al. Stereotactic radiosurgery for meningiomas. J Neurosurg. 1991;74:552-559.
81 Debus J, Wuendrich M, Pirzkall A, et al. High efficacy of fractionated stereotactic radiotherapy of large base-of-skull meningiomas: Long-term results. J Clin Oncol. 2001;19:3547-3553.
82 Goldsmith BJ, Rosenthal SA, Wara WM, et al. Optic neuropathy after irradiation of meningioma. Radiology. 1992;185:71-76.
83 Shrieve DC, Hazard L, Boucher K, et al. Dose fractionation in stereotactic radiotherapy for parasellar meningiomas: radiobiological considerations of efficacy and optic nerve tolerance. J Neurosurg. 2004;101(Supp 3):390-395.
84 DiBiase SJ, Kwok Y, Yovino S, et al. Factors predicting local tumor control after gamma knife stereotactic radiosurgery for benign intracranial meningiomas. Int J Radiat Oncol Biol Phys. 2004;60(5):1515-1519.
85 Milker-Zabel S, Zabel A, Schulz-Ertner D, et al. Fractionated stereotactic radiotherapy in patients with benign or atypical meningioma: Long-term experience and prognostic factors. Int J Radiat Oncol Biol Phys. 2005;61(3):809-816.
86 Steinvorth S, Welzel G, Fuss M, et al. Neuropsychological outcome after fractionated stereotactic radiotherapy (FSRT) for base of skull meningiomas: a prospective 1-year follow-up. Radiother Oncol. 2003;69:177-182.
87 Lawrence JH. Heavy particle irradiation of intracranial lesions. In: Wilkens RH, Rengachary SS, editors. Neurosurgery. New York: McGraw-Hill; 1985:1113-1132.
88 Sheehan JP, Niranjan A, Sheehan JM, et al. Stereotactic radiosurgery for pituitary adenomas: An intermediate review of its safety, efficacy, and role in the neurosurgical treatment armamentarium. J Neurosurg. 2005;102:678-691.
89 Pollack BE, Cochran J, Natt N, et al. Gamma knife radiosurgery for patients with nonfunctioning pituitary adenomas: results for a 15-year experience. Int J Radiat Oncol Biol Phys. 2008;70(5):1325-1329.
90 Mingione V, Yen CP, Vance ML, et al. Gamma surgery in the treatment of nonsecretory pituitary macroadenoma. J Neurosurg. 2006;104:876-883.
91 Liscak R, Vladyka V, Marek J, et al. Gamma knife radiosurgery for endocrine-inactive pituitary adenomas. Acta Neurochir (Wien). 2007;149(10):999-1006.
92 Brada M, Rajan B, Traish D, et al. The long-term efficacy of conservative surgery and radiotherapy in the control of pituitary adenomas. Clin Endocrin (Oxf). 1993;38(6):571-578.
93 Milker-Zabel S, Debus J, Thilman C, et al. Fractionated stereotactically guided radiotherapy and radiosurgery in the treatment of functional and nonfunctional adenomas of the pituitary gland. Int J Radiat Oncol Biol Phys. 2001;50(5):1279-1286.
94 Vladyka V, Liscak R, Novotny J, et al. Radiation tolerance of functioning pituitary tissue in gamma knife surgery for pituitary adenomas. Neurosurgery. 2003;52:309-317.
95 Laws ER. Surgery for acromegaly: evolution of the techniques and outcomes. Rev Endocr Metab Disord. 2008;9:67-70.
96 Ayuk J, Clayton RN, Holder G, et al. Growth hormone and pituitary radiotherapy, but not serum insulin-like growth factor-I concentrations, predict excess mortality in patients with acromegaly. J Clin Endocrinol Metab. 2004;89(4):1613-1617.
97 Pollack BE, Jacob JT, Brown PD, et al. Radiosurgery of growth hormone-reducing pituitary adenomas: factors associated with biochemical remission. J Neurosurg. 2007;106:833-838.
98 Attanasio R, Epaminondia P, Motti E, et al. Gamma-knife radiosurgery in acromegaly: a 4-year follow-up study. J Clin Endocrinol Metab. 2003;88(7):3105-3112.
99 Jezkova J, Marek J, Hana V, et al. Gamma knife radiosurgery for acromegaly—long-term experience. Clin Endocrin. 2006;64:588-595.
100 Mitsumori M, Shrieve DC, Alexander EIII, et al. Initial clinical results of linac-based stereotactic radiosurgery and stereotactic radiotherapy for pituitary adenoma. Int J Radiat Oncol Biol Phys. 1998;42:573-580.
101 Minniti G, Traish D, Ashley S, et al. Fractionated stereotactic conformal radiotherapy for secreting and nonsecreting pituitary adenomas. Clin Endocrinol. 2006;64:542-548.
102 Powell JS, Wardlaw SL, Post D, et al. Outcome of radiotherapy for acromegaly using normalization of insulin-like growth factor I to define cure. J Clin Endocrinol Metab. 2000;85:2068-2071.
103 Jenkins PJ, Bates P, Carson MN, et al. Conventional pituitary irradiation is effective in lowering serum growth hormone and insulin-like growth factor-I in patients with acromegaly. J Clin Endocrinol Metab. 2006;91(4):1239-1245.
104 Newell-Price J, Bertagna X, Grossman AB, et al. Cushing’s Syndrome. Lancet. 2006;367:1605-1617.
105 Biller BMK, Grossman AB, Stewart PM, et al. Treatment of adrenocorticotropin-dependent Cushing’s syndrome: a consensus statement. J Clin Endocrinol Metab. 2008;93(7):2454-2462.
106 Sonino N, Zielezny M, Fava GA, et al. Risk factors and long-term outcome in pituitary-dependent Cushing’s Disease. J Clin Endocrinol Metab. 1996;81(7):2647-2652.
107 Castinetti F, Nagai M, Dufour H, et al. Gamma knife radiosurgery is a successful adjunctive treatment in Cushing’s disease. Eur J Endocrinol. 2007;156:91-98.
108 Devin JK, Allen GS, Cmelak AJ, et al. The efficacy of linear accelerator radiosurgery in the management of patients with Cushing’s disease. Stereotact Funct Neurosurg. 2004;82:254-262.
109 Jagannathan J, Sheehan JP, Pouratian N, et al. Gamma knife surgery for Cushing’s disease. J Neurosurg. 2007;106:980-987.
110 Petit JH, Biller BMK, Yock TI, et al. Proton stereotactic radiotherapy for persistent adrenocorticotropin-producing adenomas. J Clin Endocrinol Metab. 2008;93:393-399.
111 Minniti G, Brada M. Radiotherapy and radiosurgery for Cushing’s disease. Arq Bras Endocrinol Metab. 2007;51(8):1373-1380.
112 Minniti G, Osti M, Jaffrain-Rea ML, et al. Long-term results of postoperative radiation therapy for Cushing’s disease. J Neuroncol. 2007;84(1):79-84.
113 Estrada J, Boronat M, Mielgo M, et al. The long-term outcome of pituitary irradiation after unsuccessful transsphenoidal surgery in Cushing’s disease. N Eng J Med. 1997;336:172-177.
114 Landolt AM, Lomax N. Gamma knife radiosurgery for prolactinomas. J Neurosurg. 2000;93(Supp 3):14-18.
115 Pouratian N, Sheehan J, Jagannathan J, et al. Gamma knife radiosurgery for medically and surgically refractory prolactinomas. Neurosurgery. 2006;59:255-266.
116 Shaw E, Scott C, Suh J, et al. RSR13 plus cranial radiation therapy in patients with brain metastases: Comparison with the radiation therapy oncology group recursive partitioning analysis brain metastases database. J Clin Oncol. 2003;21:2364-2371.
117 Patchell RA, Tibbs PA, Walsh JW, et al. A randomized trial of surgery in the treatment of single metastases to the brain. N Engl J Med. 1990;322(8):494-500.
118 Noyes WR, Auchter RM, Craig B, et al. Cost analysis of radiosurgery versus resection for single brain metastases. In: Kondziolka D, editor. Radiosurgery 1995. Basel: Karger; 1995:172-179.
119 Baumert BG, Rutten I, Dehing-Oberije C, et al. A pathology-based substrate for target definition in radiosurgery of brain metastases. Int J Radiat Oncol Biol Phys. 2006;66(1):187-194.
120 Noel G, Simon JM, Valery CA, et al. Radiosurgery for brain metastasis: impact of CTV on local control. Radiother Oncol. 2003;68:15-21.
121 Nataf F, Schlienger M, Zhihua L, et al. Radiosurgery with or without a 2-mm margin for 93 single brain metastases. Int J Radiat Oncol Biol Phys. 2008;70(3):766-772.
122 Shehata MK, Young B, Reid B, et al. Stereotactic radiosurgery of 468 brain metastases <2 cm: implications for SRS dose and whole brain radiation therapy. Int J Radiat Oncol Biol Phys. 2004;59(1):87-93.
123 Vogelbaum MA, Angelov L, Lee SY, et al. Local control of brain metastases by stereotactic radiosurgery in relation to dose to the tumor margin. J Neurosurg. 2006;104:907-912.
124 Aoyama H, Shirato H, Tago M, et al. Stereotactic radiosurgery plus whole-brain radiation therapy vs stereotactic radiosurgery alone for treatment of brain metastases. JAMA. 2006;295:2483-2491.
125 Hasegawa T, Kondziolka D, Flickinger JC, et al. Brain metastases treated with radiosurgery alone: An alternative to whole brain radiotherapy? Neurosurgery. 2003;52:1318-1326.
126 Sneed PK, Lamborn KR, Forstner JM, et al. Radiosurgery for brain metastases: is whole-brain radiotherapy necessary? Int J Radiat Oncol Biol Phys. 1999;43:549-558.
127 Lutterbach J, Cryson D, Henne K, et al. Radiosurgery followed by planned observation in patients with one to three brain metastases. Neurosurgery. 2003;52:1066-1074.
128 Flickinger JC, Kondziolka D, Lunsford LD, et al. A multi-institutional experience with stereotactic radiosurgery for solitary brain metastasis. Int J Radiat Oncol Biol Phys. 1994;28(4):797-802.
129 Loeffler JS, Alexander EIII. Radiosurgery for the treatment of intracranial metastases. In: Alexander EIII, Loeffler JS, Lunsford LD, editors. Stereotactic Radiosurgery. New York: McGraw-Hill; 1993:197.
130 Kondziolka D, Patel A, Lunsford LD, et al. Stereotactic radiosurgery plus whole-brain radiotherapy versus radiotherapy alone for patients with multiple brain metastases. Int J Radiat Oncol Biol Phys. 1999;45:427-434.
131 Andrews DW, Scott CB, Sperduto PW, et al. Whole brain radiation therapy with or without stereotactic radiosurgery boost for patients with one to three brain metastases: phase III results of the RTOG 9508 randomised trial. Lancet. 2004;363:1665-1672.
132 Gaspar LE, Scott C, Rotman M, et al. Recursive partitioning analysis (RPA) of prognostic factors in three Radiation Therapy Oncology Group (RTOG) brain metastases trials. Int J Radiat Oncol Biol Phys. 1997;37:745-751.
133 Fuller BG, Kaplan ID, Adler J, et al. Stereotaxic radiosurgery for brain metastases: The importance of adjuvant whole brain radiotherapy. Int J Radiat Oncol Biol Phys. 1992;23:413-418.
134 Patchell RA, Cirrincione C, Thaler HT, et al. Single brain metastases: surgery plus radiation or radiation alone. Neurology. 1986;36:447-453.
135 Brown PD, Asher AL, Farace E. Adjuvant whole brain radiotherapy: Strong emotions decide but rational studies are needed. Int J Radiat Oncol Biol Phys. 2008;70(5):1305-1309.
136 Meyers CA, Smith JA, Bezjak A, et al. Neurocognitive function and progression in patients with brain metastases treated with whole-brain radiation and motexafin gadolinium: results of a randomized phase III trial. J Clin Oncol. 2004;22(1):157-165.
137 Penitzka S, Steinvorth S, Sehlleier S, et al. Assessment of cognitive function after preventive and therapeutic whole brain irradiation using neuropsychological testing. Strahlenther Onkol. 2002;178(5):252-258.
138 Regine WF, Scott C, Murray K, et al. Neurocognitive outcome in brain metastases patients treated with accelerated-fractionation vs. accelerated hyperfractionated radiotherapy: an analysis from Radiation Therapy Oncology Group Study 91-04. Int J Radiat Oncol Biol Phys. 2001;51(3):711-717.
139 Chitapanarux I, Goss B, Vongtama R, et al. Prospective study of stereotactic radiosurgery without whole-brain radiotherapy in patients with four or less brain metastases: incidence of intracranial progression and salvage radiotherapy. J Neurooncol. 2003;61(2):143-149.
140 Alexander EIII, Moriarity T, Davis R, et al. Stereotactic radiosurgery for definitive, noninvasive treatment of brain metastases. J Nat Can Inst. 1995;87:34.
141 Chang EL, Selek U, Hassenbusch SJIII, et al. Outcome variation among “radioresistant” brain metastases treated with stereotactic radiosurgery. Neurosurgery. 2005;55(5):936-945.
142 Gaudy-Marqueste C, Regis JM, Muracciole X, et al. Gamma-knife radiosurgery in the management of melanoma patients with brain metastases: a series of 106 patients without whole-brain radiotherapy. Int J Radiat Oncol Biol Phys. 2006;65(3):809-816.
143 Mathieu D, Kondziolka D, Cooper PB, et al. Gamma knife radiosurgery in the management of malignant melanoma brain metastases. Neurosurgery. 2007;60:471-482.
144 Samlowski WE, Watson GA, Wang M, et al. Multimodality treatment of melanoma brain metastases incorporating stereotactic radiosurgery (SRS). Cancer. 2007;109:1855-1862.
145 Goyal LK, Suh JH, Reddy CA, et al. The role of whole-brain radiotherapy and stereotactic radiosurgery of brain metastases from renal cell carcinoma. Int J Radiat Oncol Biol Phys. 2000;47(4):1007-1012.
146 Noel G, Valery CA, Boisserie G, et al. LINAC radiosurgery for brain metastases of renal cell carcinoma. Urol Oncol. 2004;22(2):25-31.
147 Samlowski WE, Majer M, Boucher KM, et al. Multidisciplinary treatment if brain metastases derived from clear cell renal cancer incorporating stereotactic radiosurgery (SRS). Cancer. 2008;133(9):2539-2548.
148 Mehta MP, Rozental JM, Levin AB, et al. Defining the role of radiosurgery in the management of brain metastases. Int J Radiat Oncol Biol Phys. 1992;24:619.
149 Leibel SA, Scott CB, Loeffler JS. Contemporary approaches to the treatment of malignant gliomas with radiation therapy. Semin Oncol. 1994;21(2):198-219.
150 Wallner KE, Galicich JH, Krol G, et al. Patterns of failure following treatment for glioblastoma multiforme and anaplastic astrocytoma. Int J Radiat Oncol Biol Phys. 1989;16(6):1405-1409.
151 Gutin PH, Leibel SA, Wara WM, et al. Recurrent malignant gliomas: survival following interstitial brachytherapy with high-activity iodine-125 sources. J Neurosurg. 1987;67:864-873.
152 Shrieve DC, Alexander EI, Wen PY, et al. Comparison of stereotactic radiosurgery and brachytherapy in the treatment of recurrent glioblastoma multiforme. Neurosurgery. 1995;36(2):275-284.
153 Combs SE, Widmer V, Thilman C, et al. Stereotactic radiosurgery (SRS): treatment option for recurrent glioblastoma multiforme (GBM). Cancer. 2005;104:2168-2173.
154 Hall WA, Djalilian HR, Sperduto PW, et al. Stereotactic radiosurgery for recurrent malignant gliomas. J Clin Oncol. 1995;13(7):1642-1648.
155 Chamberlain MC, Barba D, Kormanik P, et al. Stereotactic radiosurgery for recurrent gliomas. Cancer. 1994;74(4):1342-1347.
156 Scharfen CO, Sneed PK, Wara WM, et al. High activity iodine-125 implant for gliomas. Int J Radiat Oncol Biol Phys. 1992;24:583-591.
157 Shrieve DC, Alexander EI, Black PM, et al. Treatment of patients with primary glioblastoma multiforme with standard postoperative radiotherapy and radiosurgical boost: Prognostic factors and long-term outcome. J Neurosurg. 1999;90:72-77.
158 Nwokedi EC, DiBiase SJ, Jabbour S, et al. Gamma knife stereotactic radiosurgery for patients with glioblastoma multiforme. Neurosurgery. 2002;50:41-47.
159 Ulm AJIII, Friedman WA, Bradshaw P, et al. Radiosurgery in the treatment of malignant gliomas: the University of Florida experience. Neurosurgery. 2005;57:512-517.
160 Sarkaria JN, Mehta MP, Loeffler JS, et al. Radiosurgery in the initial management of malignant gliomas: Survival comparison with RTOG recursive partitioning analysis. Int J Radiat Oncol Biol Phys. 1995;32:931-941.
161 Curran WJ, Scott CB, Horton J, et al. Recursive partitioning analysis of prognostic factors in three Radiation Therapy Oncology Group malignant glioma trials. J Nat Cancer Inst. 1993;85:704-710.
162 Souhami L, Sieferheld W, Brachman D, et al. Randomized comparison of stereotactic radiosurgery followed by conventional radiotherapy with carmustine to conventional radiotherapy with carmustine for patients with glioblastoma multiforme: report of Radiation Therapy Oncology Group 93–05 Protocol. Int J Radiat Oncol Biol Phys. 2004;60(3):853-860.
163 Larson DA, Gutin PH, McDermott MW, et al. Gamma knife for glioma: selection factors and survival. Int J Radiat Oncol Biol Phys. 1996;36(5):1045-1053.
164 Leksell L. Cerebral radiosurgery. I. Gammathalanotomy in two cases of intractable pain. Acta Chir Scand. 1968;137:311-314.
165 Friehs GM, Park MC, Goldman MA, et al. Stereotactic radiosurgery for functional disorders. Neurosurg Focus. 2007;23(6):E3.
166 Kondziolka D, Lunsford LD, Flickinger JC, et al. Stereotactic radiosurgery for trigeminal neuralgia: a multi-institutional study using the gamma knife. J Neurosurg. 1996;84:940-945.
167 Goss BW, Frighetto L, DeSalles AAF, et al. Linear accelerator radiosurgery using 90 Gray for essential trigeminal neuralgia: results and dose volume histogram analysis. Neurosurgery. 2003;53:823-830.
168 Longhi M, Rizzo P, Nicolato A, et al. Gamma knife radiosurgery for trigeminal neuralgia: results and potentially predictive parameters—Part I: Idiopathic trigeminal neuralgia. Neurosurgery. 2007;61:1254-1261.
169 Kjellberg RN, Abe M. Stereotactic Bragg peak proton beam therapy. In: Lunsford LD, editor. Modern Stereotactic Neurosurgery. Boston: Martnus Nijhoff; 1988:463.
170 Flickinger JC. An integrated logistic formula for prediction of complications from radiosurgery. Int J Radiat Oncol Biol Phys. 1989;17:879-885.
171 Flickinger JC, Kondziolka D, Pollack BE, et al. Complications from arteriovenous malformation radiosurgery: multivariate analysis and risk modeling. Int J Radiat Oncol Biol Phys. 1997;38(3):485-490.
172 Stafford SL, Pollack BE, Leavitt JA, et al. A study of the radiation tolerance of optic nerves and chiasm after stereotactic radiosurgery. Int J Radiat Oncol Biol Phys. 2003;55(5):1177-1181.
173 Massager N, Nissim O, Delbrouck C, et al. Irradiation of the cochlear structures during vestibular schwannoma radiosurgery and associated hearing outcome. J Neurosurg. 2007;107:733-739.
174 Zhang N, Pan L, Zhong J, et al. Gamma knife radiosurgery for jugular foramen schwannomas. J Neurosurg. 2002;97:456-458.
175 Martin JJ, Kondziolka D, Flickinger JC, et al. Cranial nerve preservation and outcomes after stereotactic radiosurgery for jugular foramen schwannomas. Neurosurgery. 2007;61:76-81.
176 Meeks SL, Buatti JM, Foote KD, et al. Calculation of cranial nerve complication probability for acoustic neuroma radiosurgery. Int J Radiat Oncol Biol Phys. 2000;47(3):597-602.
177 Lunsford LD. Contemporary management of meningiomas: radiation therapy as an adjuvant and radiosurgery as an alternative to surgical removal? J Neurosurg. 1994;80:187-190.
178 Shaw E, Scott C, Souhami L, et al. Radiosurgery for the treatment of previously irradiated recurrent primary brain tumors and brain metastases: initial report of Radiation Therapy Oncology Group Protocol 90-05. Int J Radiation Oncology Biol Phys. 1996;34(3):647-654.
179 Larson DA, Gutin PH, McDermott M, et al. Gamma knife for glioma: selection factors and survival. Int J Radiation Oncology Biol Phys. 1996;36(5):1045-1053.
180 Shrieve DC, Alexander EIII, Wen PY, et al. Comparison of stereotactic radiosurgery and brachytherapy in the treatment of recurrent glioblastoma multiforme. Neurosurgery. 1995;36:275-284.
181 Flickinger JC, Kondziolka D, Niranjan A, et al. Acoustic neuroma radiosurgery with marginal doses of 12 to 13 Gy. Int J Radiat Oncol Biol Phys. 2004;60:225-230.
182 Beegle RD, Friedman WA, Bova FJ. Effect of treatment plan quality on outcomes after radiosurgery for vestibular schwannoma. J Neurosurg. 2007;107(5):913-916.
183 Lunsford LD, Niranjan A, Flickinger JC, et al. Radiosurgery of vestibular schwannomas: summary of 829 cases. J Neurosurg. 2005;102S:195-199.
184 Flickinger JC, Kondziolka D, Niranjan A, et al. Results of acoustic neuroma radiosurgery: an analysis of 5 year’s experience using current methods. J Neurosurg. 2001;94:1-6.
185 Lutz W. Radiation physics for radiosurgery. In: Alexander E, Loeffler JS, Lunsford LD, editors. Stereotactic Radiosurgery. New York: McGraw-Hill; 1993:7-15.