Chapter 45 Principles of Antimicrobial Use
Abbreviations | |
---|---|
CNS | Central nervous system |
CSF | Cerebrospinal fluid |
DNA | Deoxyribonucleic acid |
IM | Intramuscular |
IV | Intravenous |
MBC | Minimal bactericidal concentration |
MIC | Minimal inhibitory concentration |
MRSA | Methicillin-resistant Staphylococcus aureus |
PBP | Penicillin binding proteins |
PO | Oral |
RNA | Ribonucleic acid |
Viruses, bacteria, other unicellular organisms, and multicellular organisms in the environment can also live in the human body. This relationship can produce desirable and undesirable responses within the host. In a healthy person, normal bacteria in the gastrointestinal tract have beneficial effects, assisting in the production of vitamins and the breakdown of foods. However, when nonbeneficial, pathogenic organisms enter the body through oral ingestion, inhalation, trauma, surgical procedures, or any body opening, the result is an unwanted host-pathogen response, termed an infection. The responses to an infection can occur either directly through the release of toxins or antigens by the invading organism or indirectly through tissue invasion or generation of an inflammatory response by the body. Many infections develop as a result of noninfectious primary diseases that suppress the patient’s natural immune response. However, on a global scale, parasitic infections are the greatest cause of morbidity and mortality worldwide.
Table 45-1 lists the types of pathogenic organisms that invade the human body and cause unwanted biological responses. Treatment of the problems generated by these invasions is approached in two ways: (1) destruction or removal of invading organisms, and (2) alleviation of symptoms. The availability of drugs for successful eradication of invading organisms varies considerably with the type and location of the organisms within the human host.
TABLE 45–1 Pathological Organisms that Can Live in a Parasitic Invader-Host Relationship in Humans, Listed in Order of Increasing Complexity
Cell Type | Organism | Typical Size (nm) |
---|---|---|
Acellular | Viruses | 20-200 |
Unicellular | Chlamydia (P) | 1000 |
Mycoplasma (P) | 1000 | |
Rickettsia (P) | 1000 | |
Bacteria (P) | 1000 | |
Fungi: yeasts (E) | 3000-5000 | |
Protozoa (E) | ||
Multicellular | Fungi: molds (E) | 2000-10,000 and larger |
Helminths (E) |
P, Prokaryotes (no nuclear membrane); E, eukaryotes (with a nucleus).
The only acellular organisms known to induce infectious diseases in humans are viruses and proteinaceous agents lacking nucleic acids called prions. Other small molecular weight, acellular nucleic acids called viroids can exist in humans but probably do not contribute to disease. Bacteria are unicellular, nonnuclear organisms. Higher orders of size and complexity are found in unicellular, nucleated fungi (including yeast and filamentous forms) and protozoa. Major differences include the addition of a membrane-enclosed nucleus and mitochondria within the cell. More complex fungi are found in the multicellular, nucleated molds (see Chapter 50). Still higher orders of parasitic organisms are helminths (worms), which are estimated to infect 40% to 60% of the world’s population and are a medical problem in both industrialized and developing countries (see Chapter 52).
drugs available to halt proliferation of, or eliminate, clinically important viruses, although this situation is improving. Vaccines have provided the most effective protection against viruses; however, prophylactic use of some antiviral drugs has also proven effective in preventing certain viral infections (see Chapter 51).
The development of antimicrobial therapy is considered by many to be one of the most important advances in the history of medicine. Major historical events leading to the development of antimicrobial therapy are listed in Box 45-1. The term antibiotic traditionally refers to substances produced by microorganisms to suppress the growth of other microorganisms. The term antimicrobial agent is broader in meaning, because it encompasses drugs synthesized in the laboratory and those natural antibiotics produced by microorganisms. Some natural antibiotics produced originally by microbial fermentation are now produced by chemical synthesis. Many agents are semisynthetic; that is, the key portion of the compound is produced by microbial fermentation, and various moieties are attached synthetically. Thus the distinction between the terms antibiotics and antimicrobial agents is somewhat blurred and has little meaning today.
BOX 45–1 History of Antimicrobial Therapy
Early 17th Century | The first recorded successful use of antimicrobial therapy involving the use of an extract from cinchona bark for the treatment of malaria. |
1909 | Paul Ehrlich’s quest for a “magic bullet” that would bind specifically to particular sites on parasitic organisms leads to an arsenic derivative, salvarsan, with modest activity against syphilis. He also suggested that antimicrobial drugs would be most useful if the sites of action were not present in the organs and tissues of the human host. |
1929 | Alexander Fleming discovers penicillin. |
1935 | Discovery of prontosil, a forerunner of sulfonamides. |
1940 | Florey and Chain first use penicillin clinically. |
The efficacy and relative safety of these drugs has led to their widespread use and overuse, with up to 50% of antimicrobials consumed in the United States considered unnecessary. Because of their ability to alter microbial flora and lead to antibiotic-resistant microorganisms, antimicrobials are fundamentally different from other types of drugs. It is important to always remember that the pathogenicity of a bacterial species may mutate with time as new strains appear, existing strains develop resistance, old strains disappear, and new problems emerge with old strains thought to be relatively benign. This complicates planning drug treatment and requires the clinician to stay informed and use these agents wisely.
Antimicrobial agents can be classified into major groups according to the point in the cellular biochemical pathways at which they exert their primary mechanism of action (Fig. 45-1). These are:
Agents that inhibit the synthesis of cell walls include the β-lactams, such as penicillins, cephalosporins, monobactams, and carbapenems, and others, such as vancomycin. Inhibitors of cytoplasmic membranes include the polymyxins and daptomycin. In fungi the cell wall is damaged by the polyene antifungal drugs or by the azoles. Inhibitors of nucleic acid synthesis include the quinolones, which inhibit deoxyribonucleic acid (DNA) gyrase, and the ribonucleic acid (RNA) polymerase inhibitor rifampin. Protein synthesis is inhibited by aminoglycosides, tetracyclines, chloramphenicol, erythromycin, clindamycin, linezolid, and streptogramins. These agents are usually bacteriostatic, except the aminoglycosides and sometimes streptogramins, which can be bactericidal. Finally, folate antagonists such as sulfonamides and trimethoprim interfere with cell metabolism. These antimicrobial drugs are listed by their mechanisms of action in Table 45-2.
TABLE 45–2 Classification of Antimicrobial Agents by Mechanism of Action
Mechanism of Action | Agent | Discussed in Chapter |
---|---|---|
Inhibition of synthesis or damage to cell wall | Penicillins | 46 |
Cephalosporins | 46 | |
Monobactams | 46 | |
Carbapenems | 46 | |
Bacitracin | 46 | |
Vancomycin | 46 | |
Inhibition of synthesis or damage to cytoplasmic membrane | Polymyxins | 48 |
Amphotericin B | 50 | |
Modification of synthesis or metabolism of nucleic acids | Quinolones | 48 |
Rifampin | 49 | |
Nitrofurans | 48 | |
Inhibition or modification of protein synthesis | Aminoglycosides | 47 |
Tetracyclines | 47 | |
Chloramphenicol | 47 | |
Erythromycin | 47 | |
Clindamycin | 47 | |
Streptogramins | 47 | |
Linezolid | 47 | |
Mupirocin | 47 | |
Modification of energy metabolism | Sulfonamides | 48 |
Trimethoprim | 48 | |
Dapsone | 49 | |
Isoniazid | 49 |
DRUG SELECTION FOR INDIVIDUAL PATIENTS
Major factors to be considered when selecting an antimicrobial agent are outlined in Box 45-2. An understanding of these factors assists with rational selection of an appropriate compound. It is important to keep in mind that each factor is important, but their relative significance may vary from case to case.
Identification of the Pathogen
For many infections an attempt should be made to identify the possible pathogen or pathogens before initiating antimicrobial therapy. Specimens for direct inspection and culture should be obtained before therapy is started, because drug therapy can decrease the yield of culture. Gram staining is the fastest, simplest, and most inexpensive method to identify bacteria and fungi. Because a Gram stain can be accomplished quickly, the results can be used to guide the initial antimicrobial choice. Body fluids that are normally sterile should be Gram stained. In addition, stains of wound exudates, sputum, and fecal material can sometimes yield information about the infecting microorganisms.
Susceptibility of Infecting Microorganisms
Susceptibility testing is often performed by automated systems based on the broth dilution method in which antibiotics are tested in serial dilutions that encompass the concentrations normally achieved in humans. This method detects the lowest concentration of antimicrobial agent that prevents visible growth after incubation for 18 to 24 hours, referred to as the minimal inhibitory concentration (MIC). The technique is shown in Figure 45-2, A. Deciding whether the results demonstrate that the organisms are susceptible requires an understanding of the pharmacokinetics of the antimicrobial agent, correlations between clinical outcomes and MIC data, and knowledge of the relationship between resistance mechanisms and MICs. Determining the MIC “cutoff” for susceptibility is often not straightforward and is the purview of an international committee. This same test procedure can be extended further to determine the minimal bactericidal concentration (MBC), or minimal concentration that kills 99.9% of cells. In this test samples are removed from the antibiotic-containing tubes in which there is no visible microbial growth and plated on agar that contains no additional antibiotic. The MBC is the lowest concentration of antibiotic in the original tube from which bacteria do not grow on the agar (see Fig. 45-2, B). MBC determinations are no longer used regularly in most clinical laboratories.
Another method for determining bacterial susceptibility to antibiotics is the disk diffusion method, in which disks impregnated with the drugs to be tested are placed on an agar plate freshly inoculated with the bacterial strain in question. After the plates have been incubated for 18 to 24 hours, bacterial growth occurs everywhere except near the disks, where a “zone of inhibition” may be present. If sufficiently large, the zone indicates that the bacterial strain is susceptible to the antibiotic in the disk. This test is simple to perform but is only semiquantitative and not useful for determining the susceptibility of many slow-growing or fastidious organisms; it has been replaced by automated broth dilution systems in many laboratories. The test procedure and typical results are shown in Figure 45-3. A newer and related method is the E-test, which uses a strip containing the antibiotic in a concentration gradient along with a numerical scale instead of a disk. The point where the zone of inhibition touches the strip indicates the MIC (Fig. 45-4).
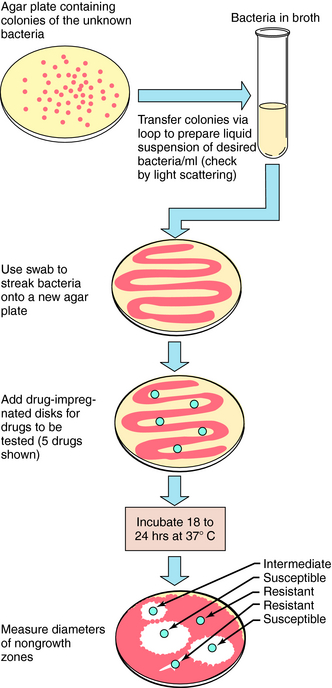
FIGURE 45–3 Disk diffusion method for testing bacteria for susceptibility to specific antimicrobial agents.
Need for Bactericidal versus Bacteriostatic Agents
Antimicrobial agents can be bactericidal (i.e., the organisms are killed) or bacteriostatic (i.e., the organisms are prevented from growing) (Fig. 45-5). A given agent may show bactericidal actions under certain conditions but bacteriostatic actions under others, depending on the concentration of drug and the target bacteria. A bacteriostatic agent often is adequate in uncomplicated infections, because the host defenses will help eradicate the microorganism. For example, in pneumonococcal pneumonia, bacteriostatic agents suppress the multiplication of the pneumococci, and the pneumococci are destroyed by interaction with alveolar macrophages and polymorphonuclear leukocytes. For a neutropenic individual, such a bacteriostatic agent might prove ineffective, and a bactericidal agent would be necessary. Thus the status of the host influences whether a bactericidal or bacteriostatic agent is selected.
Pharmacokinetic and Pharmacodynamic Factors
The amount of antimicrobial agent that reaches the extravascular tissues and fluids where the infection is usually present depends on basic pharmacokinetic principles (see Chapters 2 and Chapter 3). Considerable variation exists among the many antimicrobial agents, and the pharmacokinetic profile of the antimicrobials is an important factor in selecting the proper drug.
Meningitis is also a difficult infection because many antimicrobial agents do not cross the blood-brain or blood-CSF barriers very well (Table 45-3). Therefore infections of the central nervous system (CNS) often require the use of lipid-soluble agents, which easily enter the CSF, such as chloramphenicol, rifampin, and metronidazole. Aminoglycosides are ineffective against CNS infections because they do not enter the CSF even in the presence of inflammation. Penicillins, aztreonam, cephalosporins, and carbapenems enter the CSF to variable degrees in the presence of meningitis. Quinolones such as ciprofloxacin also enter the CSF at concentrations adequate to kill some microorganisms. Vancomycin is active against all pneumococci, and because of possible resistance to other agents, it is used routinely as part of empiric therapy for pneumococcal meningitis. Yet microbiologic failures on vancomycin have been reported, because this agent does not penetrate into the CSF sufficiently to guarantee acceptable bactericidal levels.
TABLE 45–3 Ability of Antibiotics to Enter the Cerebrospinal Fluid in Effective Concentrations
Readily Enter CSF | Enter CSF When Inflammation Present | Do Not Enter CSF Adequately to Treat Infection |
---|---|---|
Chloramphenicol | Penicillin G | Cefazolin |
Sulfonamides | Ampicillin | Cefoxitin |
Trimethoprim | Piperacillin | Erythromycin |
Rifampin | Oxacillin | Clindamycin |
Metronidazole | Nafcillin | Tetracycline |
Cefuroxime | Gentamicin | |
Cefotaxime | Tobramycin | |
Ceftriaxone | Amikacin | |
Ceftazidime | ||
Aztreonam | ||
Ciprofloxacin | ||
Vancomycin | ||
Meropenem | ||
Cefepime |
A history of an adverse reaction to an antibiotic is important, because a similar reaction to other members of the same drug class may occur. It is important to characterize the reaction to distinguish intolerance such as gastrointestinal upset from a true allergy and to recognize potentially life-threatening allergic reactions such as anaphylaxis or exfoliative dermatitis. When these serious reactions occur, administration of chemically related compounds should be avoided. Significant allergy appears to be more common with β-lactams, particularly penicillins, and sulfonamides. In anaphylactic reactions to penicillins, the immunoglobulin E antibody is usually directed at the penicillin nucleus, so the potential for allergic reactions to other penicillins is high. For many other allergic reactions, the potential for cross-allergy to related compounds is not known.
Almost all antimicrobial agents cross the placenta to some degree and may affect the fetus. With most agents, the greatest risk of teratogenic and toxic effects on the fetus is in the first trimester (Table 45-4). Metronidazole is teratogenic in lower animals, but it is not clear if this drug poses a risk to human fetuses. Other agents such as rifampin and trimethoprim may have a teratogenic potential and should be used only when alternative agents are unavailable. Quinolones cause cartilage abnormalities in animal models. As with some other agents, it is not clear if these effects seen in animals translate to significant human health risks.
TABLE 45–4 Antimicrobial Agents to be Used with Caution or Avoided During Pregnancy
Agent | Potential Toxicity |
---|---|
Aminoglycosides | Damage to cranial nerve VIII |
Chloramphenicol | Gray baby syndrome |
Erythromycin estolate | Cholestatic hepatitis in mother |
Metronidazole | Possible teratogenicity |
Nitrofurantoin | Hemolytic anemia |
Sulfonamides | Hemolysis in newborn with glucose-6-phosphate dehydrogenase deficiency; increased risk of kernicterus |
Tetracyclines | Limb abnormalities, dental staining, inhibition of bone growth |
Trimethoprim | Altered folate metabolism |
Quinolones | Abnormalities of cartilage |
Vancomycin | Possible auditory toxicity |
An absence of white blood cells predisposes a patient to serious bacterial infection, and bacteriostatic agents are often ineffective in treating serious infections in neutropenic hosts. The critical white blood cell count is between 500 and 1000 mature polymorphonuclear cells/mm3. Bactericidal agents are also required in the setting of other host defects, such as agammaglobulinemia or asplenia. The latter predisposes patients to pneumococcal or Haemophilus infection. The absence of complement components C7 to C9 predisposes patients to serious infection with Neisseria species. Knowledge of the organisms most frequently causing infections in patients with defects of white blood cells, complement, T cells, or immunoglobulin production aids in the selection of bactericidal agents when fever develops.
The reasons for using antibiotic combinations are listed in Box 45-3. Although combination therapy is necessary sometimes, it tends to be overused. Some of the reliance on antibiotic combinations is due to a failure to identify the etiologic agent, forcing continuation of broad empiric therapy.
Antimicrobial combinations directed at a single organism are considered to elicit indifferent effects if the combined activity equals the sum of the separate activities. Synergism is present if the activity of the combined antimicrobial agents is greater than the sum of the independent activities. Combinations of antibiotics are antagonistic when the activity of the combination is less than could be achieved by using the agents separately. The “kill curves” illustrating these interactions are shown in Figure 45-6.
A synergistic effect has been documented for three combinations of antimicrobials:
A classic example of synergy is the use of penicillin or ampicillin plus an aminoglycoside to treat enterococcal endocarditis. Although penicillins are usually bactericidal, they affect enterococci in a bacteriostatic fashion, with a large difference between the inhibitory and bactericidal concentrations. Aminoglycosides alone are inactive against enterococci because they cannot get inside the cell to reach their ribosomal target site. Penicillins alter the cell wall of enterococci, allowing the aminoglycoside to enter the bacterial cell when both drugs are administered (Fig. 45-7). The combination is bactericidal, and this synergistic effect is critically important in the treatment of enterococcal endocarditis in humans.
ANTIBIOTIC DECISION MAKING AFTER THERAPY HAS BEEN INITIATED
The need for ongoing antimicrobial therapy should be reassessed regularly as the patient’s clinical course evolves. Because empiric therapy is often broad, it should always be reevaluated as soon as initial culture results are available (typically within 2 days). The misdemeanor of using an overly broad empiric regimen can be forgiven if, when culture and sensitivity results return, the regimen is refined accordingly. Switching from parenteral to oral therapy is cost effective, and, depending on the infection, should be initiated when the patient’s condition allows. The optimal duration of therapy for many infections is not known; however, some approximate durations are listed in Table 45-5.
TABLE 45–5 Approximate Duration of Antimicrobial Therapy for Common Infections
Infection | Duration (days) |
---|---|
Streptococcal pharyngitis | 10 |
Otitis media | 5-10 |
Sinusitis | 10 |
Uncomplicated urinary tract infection | 3 |
Pyelonephritis | 14 |
Cellulitis | 3 days after inflammation resolves |
Pneumococcal pneumonia | 3-5 days after fever resolves |
Other pneumonia | Variable, often 14 days |
Bacteremia | Variable, often 10-14 days without endocarditis |
Endocarditis | 28-42 |
Meningitis | 7-14 |
Osteomyelitis | 42 |
Septic arthritis | 21 |
MONITORING ANTIMICROBIAL THERAPY
Because of the favorable safety profile of most antimicrobial agents, predictable pharmacokinetics, and the ability to achieve serum levels well above MICs, it is usually not necessary to monitor serum antibiotic concentrations. Agents for which serum levels are routinely obtained are those that have serious toxicity or narrow margins of safety, such as aminoglycosides and vancomycin. Measuring serum concentrations of these agents can help ensure that therapeutic and not toxic levels are attained. This is particularly important in patients with diminished renal function as these agents undergo renal elimination and have nephrotoxic potential.
PROPHYLAXIS WITH ANTIMICROBIAL AGENTS
The efficacy of prophylaxis is well established in situations such as perioperative antibiotic administration before certain surgical procedures, exposure to invasive meningococcal disease, and prevention of recurrent rheumatic fever. To prevent postoperative wound infections, the antimicrobial agent must be present at the surgical site when the area is exposed to the bacteria. The antibiotic should be given immediately preoperatively and should inhibit the most common and important bacteria likely to produce infection. Prophylaxis can be effective without eradication of all bacteria, so it is not essential to administer a broad-spectrum drug.
The importance of antibiotic resistance cannot be overstated. The ability of bacteria to develop resistance to all drugs in our armamentarium threatens many of the chemotherapeutic advances of the antimicrobial era. There are several consequences of antibiotic resistance; the most obvious is that treating a patient with an ineffective drug will lead to therapeutic failure or relapse. The development of resistance also forces physicians to use newer, more costly, and sometimes more toxic agents. Resistant bacteria have a competitive advantage over other flora, and under the pressure of heavy antibiotic use can spread in the hospital environment. Most ominously, because of resistance, we now face the prospect of untreatable infections more than any time in the last several decades. The drugs of choice for treating many infections have changed over the years, in large part because of expanding resistance. Knowledge of the resistance patterns of organisms in the community and in the hospital setting helps direct empiric therapy. In addition, recognizing that some bacteria have the propensity to develop resistance to certain antibiotics during a course of treatment should influence antibiotic selection even after the susceptibility profile is known.
Antibiotic resistance can be intrinsic or acquired. For example, Pseudomonas aeruginosa is intrinsically resistant to many antibiotics because of the inability of antibiotics to cross its outer membrane or bind to target sites. Acquired resistance can be due to mutation of existing genetic information or acquisition of new genes. Exchange of genetic information among bacteria is very common and occurs by several mechanisms (Fig. 45-8). Conjugation is the process by which two physically apposed bacteria exchange genetic information, usually contained on plasmids (extrachromosomal pieces of DNA). Resistance-conferring plasmids have been identified in virtually all bacteria. Resistance is also present on transposable genetic elements (transposons or “jumping genes”) that can “jump” to plasmids or become integrated into chromosomes. Certain bacteria are naturally transformable, meaning that they can pick up exogenous DNA from the environment. A third means of genetic exchange is transduction or exchange of DNA via phages or viruses that have tropism for a particular bacterial species. This mechanism is thought to be important in resistance to Staphylococcus aureus.
The basic mechanisms of resistance to antimicrobial agents include:
These mechanisms are depicted in Figure 45-9; examples of each mechanism are listed in Table 45-6. Microorganisms can possess one or more of these mechanisms simultaneously.
TABLE 45–6 Resistance Mechanisms of Different Bacteria to Various Antibiotics
Antibiotic(s) | Mechanisms | Pathogens with Potential for Resistance Development |
---|---|---|
β-Lactams |
Data from Neu HC. The crisis in antibiotic resistance. Science 1992; 257:1064
Resistance Based on Altered Receptors for Drug
With the exception of vancomycin, resistance to every major class of antibiotics was recognized before or within a few years of introduction for clinical use. Resistance to vancomycin took three decades to develop. The mechanism is complex but involves a change in the target site for the drug on the side chains of cell wall peptidoglycan. This mechanism likely evolved from the Streptomyces that produce glycopeptide antibiotics and is an example of resistance in pathogenic bacteria borrowed from the self-protection mechanism used by the antibiotic-producing organism.
A change of only one amino acid in the β-subunit of the DNA-directed RNA polymerase can confer resistance to rifampin. For this reason rifampin is almost always used in combination with other agents to prevent development of resistance. Resistance to quinolones is usually attributable to an altered DNA gyrase.
Destruction or Inactivation of Drug
The most widely recognized example of bacterial drug resistance is that of the β-lactamases. These enzymes catalyze the hydrolysis of penicillins, cephalosporins, and other β-lactams, yielding compounds that are unable to bind to bacterial transpeptidases and other enzymes needed for cell wall synthesis and repair. The β-lactamases are discussed in Chapter 46. Other enzymes that inactivate aminoglycosides have also been described.
SELECTION OF AN ANTIMICROBIAL AGENT
The extensive variety of pathogenic bacteria, the numerous antibiotics available, and the significant list of factors to be considered in rationally selecting antibiotic therapy can be confusing for students as well as nonspecialists in infectious diseases. At the risk of oversimplification, an overview of the clinical utility of specific agents and drug classes in treatment of common pathogens and clinical syndromes is listed in Table 45-7. Again, it is important to remember that the drugs and drug classes and susceptible bacteria listed are a simplification and are meant to serve as basic guidelines, not absolute therapeutic choices. For example, not all cephalosporins are active against all gram-positive or gram-negative bacteria, and several gram-negative species are not inhibited by any of the cephalosporins.
TABLE 45–7 Overview of Antibacterial Activity of Major Antibiotics
Antibiotics | Effective Against |
---|---|
Penicillins | Many gram-positive cocci, some gram-negative |
Penicillin/β-lactamase inhibitor | More gram-positive, gram-negative, anaerobes |
Cephalosporins | |
First generation | Gram-positive, some gram-negative |
Second generation | More gram-negative, similar gram-positive |
Third generation | More gram-negative, less gram-positive; some inhibit Pseudomonas |
Fourth generation | Better gram-positive; more gram-negative (more β-lactamase stable), inhibit Pseudomonas |
Carbapenems | Broad gram-positive, gram-negative, anaerobes |
Aztreonam | Aerobic gram-negative only |
Vancomycin | Gram positive only |
Quinolones | Variable gram-positive, most gram-negative, Mycoplasma, Chlamydia, Legionella |
Aminoglycosides | Aerobic gram-negative bacilli |
Tetracyclines | Aerobic and anaerobic gram-positive and gram-negative Mycoplasma, Chlamydia |
Macrolides | Gram-positive, Mycoplasma, Chlamydia, Legionella |
Clindamycin | Many gram-positive cocci, many anaerobes |
Sulfonamides | Some gram-positive and gram-negative |
Rifampin | Gram-positive (in combination with other agents) |
Streptogramins | Gram-positive |
Oxazolidinones | Gram-positive |
Metronidazole | Anaerobes |
The relative activities of some representative antibiotics against individual microbial species are given in greater detail in Table 45-8. Because the development of antibiotic resistance occurs at variable rates, the relative activities are approximate and subject to change.
Bacteria and Anatomical Location
It is estimated that initial antimicrobial therapy is initiated in 75% of cases of bacterial infections before the pathogenic microorganisms have been identified, and that the specific pathogenic organism is never identified in approximately 50% of treated infections. As discussed previously, because empiric therapy is often used in making treatment choices, it is important to be aware of the bacterial strains that may be present at selected anatomical sites. Many times this may be the only meaningful way of guiding the selection of an antibiotic. Table 45-9 lists the common organisms that infect specific anatomical sites, and Table 45-10 groups organisms with the anatomical locations of the infection and the drugs often used for treatment.
Otitis Media | Intraabdominal Sepsis |
---|---|
Most infections can be treated successfully with different antibiotics, and there may be more than one drug that results in successful therapy. When choosing a drug regimen, it is always important to consider the rates of local antibiotic resistance.
Anonymous. Choice of antibacterial drugs. Treat Guidel Med Lett. 2007;5:33-50.
Bratzler DW, Houck PM. Antimicrobial prophylaxis for surgery: An advisory statement from the National Surgical Infection Prevention Project. Clin Inf Dis. 2004;38:1706-1715.
Moellering RCJr, Graybill JR, McGowan JEJr, Corey L. Antimicrobial resistance prevention initiative—an update: Proceedings of an expert panel on resistance. Am J Med. 2007;120:S4-S25.