Chapter 7 Ocular Embryology
With the explosion of information from improved technology and human genome study, new information is reported daily about the processes that control cellular development, structure, and function. A number of growth factors have been identified that bind to receptor sites on target cells to control normal development by modulating proliferation, migration, and differentiation.1 These processes are at the basis of structure development. Cells from germ layers migrate to a specific location and then proliferate, forming the population of cells that will differentiate into the specific cell type necessary.
Development of Ocular Structures
By the third week of embryonic development, the three primary germ layers—ectoderm, mesoderm, and endoderm—have formed the embryonic plate.2 (Of these three, only ectoderm and mesoderm will take part in the developing ocular structures.) A thickening in the ectoderm, visible on the dorsal surface of the embryo, forms the neural plate, which will give rise to the central nervous system, including ocular structures. A groove forms down the center of this plate at approximately day 18 of gestation, and the ridges bordering the groove grow into neural folds. As the groove expands, these folds grow toward one another and fuse to form the neural tube along the dorsal aspect of the embryo. Just before fusing, an area of cells on the crest of each of the neural folds separates from the ectoderm; these are neural crest cells. They form islands of cells within the mesoderm, which now surrounds the neural tube. The neural tube is formed on or near day 22.2 The tissue of the neural tube is now called neural ectoderm and the surface layer is now called surface ectoderm. Neural and surface ectoderm differ in anatomic location and in differentiation potentials (Box 7-1). Figure 7-1 illustrates these events.
BOX 7-1 Embryologic Derivation of Ocular Structures
Surface ectoderm gives rise to:
Optic Pits
Indentations form in the inner surface of the neural tube on both sides of the forebrain region even before the tube is completely closed. These indentations are the optic pits. On approximately day 25, after the neural tube has closed, the optic pits expand forming lateral sac-shaped extensions, the optic vesicles.3 The cavity within the optic vesicle is continuous with the lumen of the neural tube. The surface of each vesicle expands until it comes in contact with surface ectoderm then gradually becomes separated from it by cells of neural crest origin and mesoderm.4
Neural crest cells and mesoderm collectively make up the mesenchyme, from which the connective tissue of the globe and orbit develop. Although most orbital connective tissue is derived from neural crest, determining whether a structure is of neural crest or mesodermal origin sometimes is difficult because mesodermal cells and neural crest cells appear similar cytologically.5 If the origin is uncertain, mesenchyme is cited as the germ layer.
As the optic vesicle evaginates, the tissue joining the vesicle to the neural tube constricts, forming the optic stalk (Figure 7-2). The cells lining the inner surface of this entire formation are ciliated, and the outer surface is covered by a thin basal lamina.3 The cavity of the optic stalk, as well as that of the optic vesicle, is continuous with the space that will become the third ventricle.
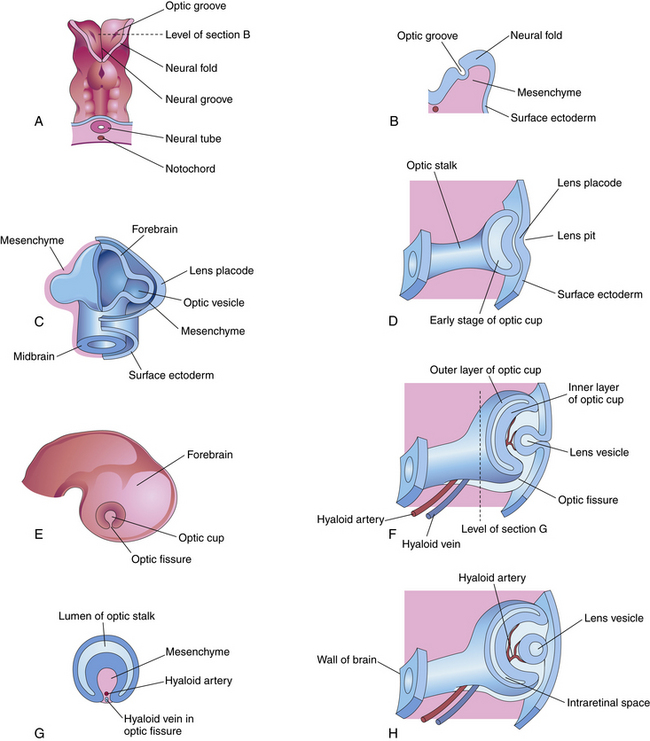
FIGURE 7-2 Early eye development.
(From Moore KL: Before we are born: essentials of embryology and birth defects, ed 5, Philadelphia, 1998, Saunders.)
While the wall of the optic vesicle is in contact with surface ectoderm, it thickens and flattens to form the retinal disc.5 The lower wall of the optic vesicle and optic stalk begins to buckle and move inward toward the upper and posterior walls. This invagination forms a cleft, variously called the optic fissure, embryonic fissure, or fetal fissure. (It has also been called the “choroidal fissure,” but this name will be avoided because it may imply that the choroid is involved in the fissure, which it is not.) The inferior wall continues to move inward, pulling the anterior wall of the optic vesicle with it and placing the retinal disc in the approximate location of the future retina. The edges of the fissure grow toward one another and begin to fuse at 5 weeks; fusion starts at the center and proceeds anteriorly toward the rim of the optic cup and posteriorly along the optic stalk. Closure is complete at 7 weeks, forming the two layers of the optic cup and optic stalk6 (Figure 7-3). Mesenchyme enters the fissure and moves into the cavity of the developing optic cup.
Optic Cup, Lens, and hyaloid vessels
Optic Cup
The optic cup at this stage of development is composed of two layers of cells (both neuroectodermal in origin) that are continuous with each other at the rim of the cup. The cells of the inner and outer layers of the optic cup are positioned apex to apex and are separated by the intraretinal space (see Figure 7-2, H), which, as the two layers approach each other, finally will become only a potential space. The outer layer of the optic cup will become the retinal pigment epithelium (RPE), the outer pigmented epithelium of the ciliary body, and the anterior iris epithelium. The inner layer will become the neural retina, the inner nonpigmented ciliary body epithelium, and the posterior iris epithelium.
Mesenchyme proliferates and migrates around the optic cup, and once the cells reach their destination, they proliferate and differentiate, contributing to the connective tissue of the eye and orbit. Neural crest cells will form corneal stroma and endothelium, uveal stroma and melanocytes, ciliary muscle, much of the sclera, the connective tissue and meningeal sheaths of the optic nerve, and the connective tissue of the lids, conjunctiva, and orbit. Vascular endothelium and striated muscle cells are formed by mesoderm.1
Clinical Comment: Coloboma
Incomplete closure of the optic fissure may affect the developing optic cup or stalk and the adult derivations of these structures, resulting in an inferior nasal defect in the optic disc, retina, ciliary body, or iris. This defect is called a coloboma and can vary from a slight notch to a large wedgelike defect. A large iris coloboma produces a keyhole-shaped pupil, although the remainder of the iris develops normally (Figure 7-4, A). When the coloboma is unilateral, the affected iris may have denser pigmentation than the opposite normal iris.7 Colobomas affecting the sensory retina and RPE also involve the choroid because its differentiation depends on an intact RPE layer.8 Bare sclera is seen in the area affected, with retinal vessels passing over the defect (Figure 7-4, B).
Induction
During embryologic development, formation and growth of structures depend on tissue differentiation and interactions among these tissues. Some structures will not develop unless they are near another developing area at a specific time. In some cases the two structures must actually come in contact; in others the structures must just be in proximity to each other, allowing biochemical signals to pass between them.9 The influence that one developing structure has on another is termed induction. It is likely that the mechanism of induction is not a single event but a series of separate steps that presumably occur on a biochemical level.10
Lens
Induction occurs between the developing optic cup and the developing lens, apparently through a reciprocal relationship.11 As the surface ectoderm comes in contact with the optic vesicle, the invagination of the optic cup begins (approximately day 27), and the surface ectoderm adjacent to the vesicle begins to thicken, forming the lens plate (lens placode) (Figures 7-5 and 7-6, A). This thickening is caused by an elongation of the ectodermal cells and by a regional increase in cell division.5 If the area of contact between the optic vesicle and surface ectoderm is less than normal, a perfectly formed but microphthalmic eye can result.12 Some investigators believe that transformation of the lens plate into the lens vesicle might be independent of direct contact with the optic vesicle.13 In addition to signals from the developing optic vesicle, complete lens differentiation might also depend on factors that inhibit lens formation in the ectoderm adjacent to the lens plate.14
Cell division ceases in the center of the lens plate, forming a pit, and cell division accelerates in the periphery such that the lens plate invaginates rapidly.15 As invagination continues, the lens vesicle is formed and then separates from the surface ectoderm at approximately day 33.3,9 The lens vesicle is a hollow sphere composed of a single layer of cells, the apical surface of the cell lines the lumen, and the basal aspect is covered by a thin basal lamina; with the addition of more material, the basal lamina will become the lens capsule (Figure 7-6, B and C).
Once the lens vesicle is formed, the posterior epithelial cells adjacent to the future vitreous cavity elongate to fill in the lumen within the lens vesicle (Figure 7-6, D, E). In chick embryos if the lens is turned 180 degrees the epithelium that was once at the anterior of the lens vesicle is now adjacent to the developing optic cup and this is the epithelium that elongates filling in the lumen.16 The developing retina secretes biochemical mediators and is apparently the inducing factor for this elongation.15 The orientation of the lens is also influenced by the developing vitreous.12,17 The posterior epithelial cells become the primary lens fibers and form the embryonic nucleus at the center of the lens. This nucleus has no sutures. The fact that the posterior epithelium was used to form the embryonic nucleus accounts for the lack of an epithelial layer beneath the posterior lens capsule in the fully formed lens. The anterior epithelial cells remain in place, and the cells near the equator begin to undergo mitosis. Each new cell elongates anteriorly and posteriorly, forming secondary lens fibers that are laid down around the embryonic nucleus. The first layer of secondary fibers is completed by week 7.9 Secondary lens fibers continue to form and each layer surrounds the previous layer. The ends of the fibers meet in an upright Y-suture immediately posterior to the anterior epithelium and in an inverted Y-suture immediately anterior to the posterior capsule. These sutures are visible during the third month.18 The fetal nucleus contains the Y-sutures and all fibers formed before birth. If a line were drawn to connect the cellular nuclei within a lens fiber layer, an arcuate shape would be revealed. This configuration is called the lens bow (Figure 7-7).
Mitosis, cell elongation, and lens fiber formation continue throughout development and throughout life. The lens is initially spherical in shape but becomes more ellipsoid with additional fibers.16 The lens capsule is evident at 5 weeks, evolving from the basement membrane of the invaginating surface ectoderm and from secretions of the lens epithelium.18
Clinical Comment: Congenital Cataract
The spectrum of lens opacities that can result from problems during lens development range from pinpoint densities having no effect on vision to significant opacities causing extensive loss of vision.19 If the tissue near the developing lens fails to induce the lens fibers to elongate and pack together in an orderly way, the lens fibers will be misaligned, forming a cataract of the primary fibers. Interference with secondary lens fibers can lead to sutural cataracts.20
Viral infection affecting the mother during the first trimester often causes congenital malformations, including a cataract. The developing lens is vulnerable to the rubella virus (German measles) between the fourth and seventh week of development, when the primary fibers are forming. After this period the virus cannot penetrate the lens capsule and thus will not affect the lens. The cataract usually is present at birth but may develop weeks to months later because the virus can persist within the lens for up to 3 years. The opacity may be dense and opaque or it may be diffuse; the cataract may affect the nucleus only or may involve most of the lens.21
Hyaloid Arterial System
A branch of the internal carotid artery enters the optic cup through the fetal fissure to become the hyaloid artery during week 5.1 This vessel produces a highly branching network that fills the vitreous cavity and forms the posterior vascular tunic of the lens (posterior tunica vasculosa lentis), a vascular network covering the posterior lens (Figure 7-8). By the end of the second month, the hyaloid vasculature is fully formed.1 Branches near the lens equator anastomose with the annular vessel at the margin of the optic cup. The annular vessel sends loops forward onto the anterior surface of the lens to form the anterior vascular tunic of the lens (anterior tunica vasculosa lentis) during the seventh week1,22,23 (Figure 7-9). These vascular networks carry nutrients to the developing lens until production of the aqueous humor occurs. These vessels drain into a network located in the region that will become the ciliary body.3 The vessels of the hyaloid system cannot be identified as arterial or venous on the basis of their histologic makeup.22
Glial cells on the surface of the optic cup form a conelike mass of tissue around the base of the hyaloid artery. These cells proliferate, forming a glial mantle around the arterial system. The hyaloid vasculature reaches its peak development during the third month1 and begins to atrophy during the fourth month, at the same time that the retinal vasculature is developing.1,24 By the seventh month, no blood flow is present in the hyaloid vasculature, which normally should be completely reabsorbed by birth.3 The extent of the degeneration of the glial tissue mass defines the extent of the adult physiologic optic cup.25
Clinical Comment: Bergmeister’s Papilla and Mittendorf’s Dot
Remnants of the hyaloid system often are seen clinically during examination of a patient’s ocular health. Glial tissue that persists on the nerve head is called Bergmeister’s papilla (Figure 7-10, A), and a pinpoint remnant of the hyaloid artery on the posterior surface of the lens is called Mittendorf’s dot. Rarely, a remnant of the entire hyaloid artery will be seen coursing through the vitreous from its attachment at the disc to the posterior lens (Figure 7-10, B).
Retinal Pigment Epithelium
Apposition of the two layers of the optic cup is essential for development of the retinal pigment epithelium,12 the first retinal layer to differentiate.25 Cellular structures and melanosomes begin to appear in the outer layer of the optic cup, and pigmentation of the retinal epithelium occurs at approximately week 3 or 4; this is the earliest pigmentation evident in the embryo3,4,26 (see Figure 7-8). After week 6, the RPE is one cell thick. The cells are cuboidal to columnar in shape, and the base of each cell is external toward the developing choroid and the apex internal toward the inner layer of the optic cup.27,28
Neural Retina
Between weeks 4 to 6, the cells of the inner layer of the optic cup (in the area that will become the neural retina) proliferate, and two zones are evident.1 The cells accumulate in the outer region, the proliferative zone or germinating zone. The inner marginal zone (of His) is anuclear. A thin lamina—the basement membrane of the inner layer of the optic cup and the precursor of the internal limiting membrane—separates the marginal zone from the vitreal cavity.18 At approximately week 7, cell migration occurs, forming the inner and outer neuroblastic layers, between which lies the transient fiber layer of Chievitz, a nucleus-free area.27,29 The formation of these two neuroblastic layers is complete during the third month. Differentiation of the neural retinal cells begins in the central retina and proceeds to the periphery.1
Ganglion cells and amacrine cells differentiate in the vitread portion of the inner neuroblastic layer.30 The ganglion cells migrate, forming a layer close to the basement membrane, and almost immediately send out their axonal processes, which become evident by week 8.18 Biomolecular agents guide axonal growth toward termination in the lateral geniculate nucleus.31,32 Müller cells, located rather centrally in the inner neuroblastic layer, develop at the same time. The bodies of the Müller and amacrine cells remain in the inner neuroblastic layer but move slightly sclerad.30
Bipolar cells migrate from the outer neuroblastic layer and settle near the Müller and amacrine cells; the horizontal cells follow.1 The fiber layer of Chievitz is gradually obliterated by this move of the prospective bipolar and horizontal cells.18 The photoreceptor cells remain in the outer neuroblastic layer. By week 12 the photoreceptors are aligned along the outer side of the inner layer of the optic cup and adhering junctions appear between them. These junctions form the precursor of the external limiting membrane. The photoreceptor cells are the last cells of neural retina to differentiate; this occurs during the fifth month.1 Cones differentiate first and rods begin to differentiate during the seventh month. The early inner segment produces a protuberance that becomes embedded in the RPE and continues to grow, forming the cilium and outer segment by week 24 or 25.18,33
The horizontal, bipolar, amacrine, and Müller cells are developing in the inner nuclear layer, and the inner and outer plexiform layers are filling with neuronal processes.3 The fibers of the Müller cells appear and extend to the basal lamina, forming the primitive internal limiting membrane, and external processes extend between the rods and cones.3,27 The Müller cell provides a scaffolding for cell development and appears to be involved with guiding the direction of axonal fiber growth.34 (The developing interplexiform neuron has not yet been identified.) Figures 7-11 and 7-12 show the development of the neural retina.
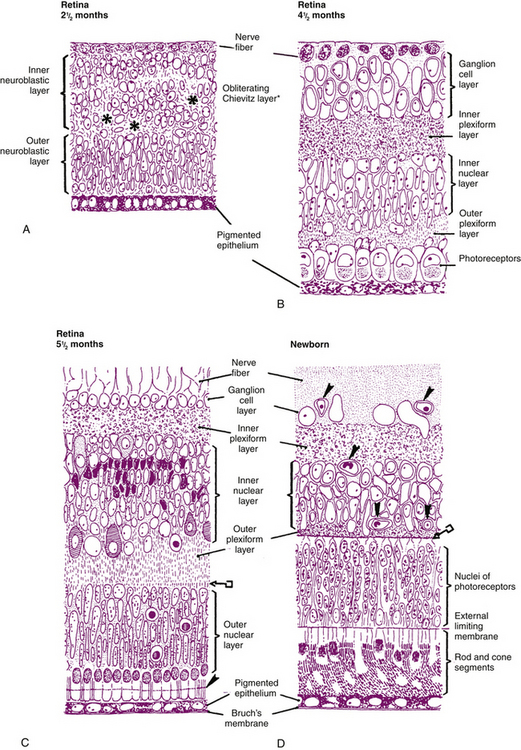
FIGURE 7-11 Developing retina.
(From Cook CS, Ozanics V, Jakobiec FA: Prenatal development of the eye and its adnexa. In Tasman W, Jaeger EA, editors: Duane’s foundations of clinical ophthalmology, vol 1, Philadelphia, 1994, Lippincott.)
Synaptic complexes begin to appear at about the same time as the plexiform layers, with the inner plexiform layer preceding the outer layer.27,35 Cone pedicles develop earlier than rod spherules, and photoreceptor synapses with bipolar cells are established before the outer segments are completed.27
By month 5 the ganglion cell layer is well established.36 Because retinal development is more advanced centrally than peripherally, the ganglion axons from the periphery must take an arched route above and below the macular area to reach the nerve head.33,37 This line of deviation at the horizontal temporal meridian is termed the horizontal raphe.3 During the fifth month a reduction of retinal cells by apoptosis begins. By month 6 there is no further mitosis, and retinal growth continues because of cell differentiation, growth, and maturation.38
During the sixth month cones begin to differentiate, and a dense accumulation of nuclei in the macular area makes this region thicker than the rest of the retina;25 up to nine rows of ganglion cells are evident.36 Foveal development consists of three stages: (1) displacement of inner retinal components to form the depression; (2) migration of photoreceptors toward the center, which increases cone packing; and (3) maturation of the photoreceptors.39 During the seventh month the ganglion cells and the cells of the inner nuclear layer begin to move to the periphery of the macula. By birth, however, there still is a single layer of ganglion cells and a thin inner nuclear layer across the now-depressed foveal area (Figure 7-13). By 4 months postpartum, both these layers are displaced to the sloping walls of the fovea, leaving the cones of the outer nuclear layer as the only neural cell bodies in the center of the depression.4,6 The foveal depression continues to deepen until about age 15 months as cells continue to move toward the macular periphery.39
The foveola, the retinal area of sharpest visual acuity, is the last to reach maturity.39 Before birth the rod-free area is large compared with that in the adult, but it becomes narrower with migration of the cones centrally, increasing cone density. When examining an area with a diameter of 100 μm at the center of the rod-free zone, the cone population is 18 in the 1-week-old infant. The cone population increases to 42 by age 4 to 5 years, which is the same count as seen in the adult.39 The cone inner fibers elongate and adopt an oblique orientation (forming Henle’s fiber layer) in order to synapse with the cells of the inner nuclear layer, which have been displaced to the sloping walls. During the first few years, the outer segment continues to develop and the inner fiber lengthens.
Clinical Comment: Ocular Albinism
Melanocytes that derive their pigment from neural crest (i.e., those located in the choroid, skin, and hair) show a variance that is related to race. Melanocytes that are neuroectodermal in derivation (i.e., retinal pigment, iris, and ciliary body epithelia) are densely pigmented in all races.36 Melanin production is gene regulated, and in an individual with albinism either or both types of melanocytes can be affected. Because normal development of sensory retina is influenced by a melanin-related agent produced in the RPE, when pigment is absent from this layer, as occurs in ocular albinism, a number of retinal abnormalities are present at birth in addition to the absence of pigmentation. The macula is underdeveloped and the fovea may be absent. The number of rods may be decreased.40 Abnormal optic nerve projection to the lateral geniculate nucleus occurs, with more crossed fibers than normal, often resulting in binocular problems.40
Retinal Vessels
The fetal fissure along the optic stalk closes around the hyaloid artery, and the portions of the vessel within the stalk become the central retinal artery.3 A branch of the primitive maxillary vein located within the optic stalk is the likely precursor of the central retinal vein.26 Early in the fourth month of development, primitive retinal vessels emerge from the hyaloid artery near the optic disc and enter the developing nerve fiber layer. Signals from biomolecular agents called guidance molecules guide the growth and pathway of neurons and likely also guide the growth of these retinal vessels.41,42 The vessels of the retina continue to develop, gradually forming the arterioles, venules, and capillary beds, but all vessel structure is not completed until approximately 3 months after birth, with the vessels to the nasal periphery completed before those to the temporal periphery.3
Clinical Comment: Retinopathy of Prematurity
Premature infants who are exposed to a high concentration of oxygen can develop retinopathy of prematurity (also called retrolental fibroplasia). The immature retinal blood vessels respond to the high concentration of oxygen with vasoconstriction and cease to develop. On removal of the oxygen, vasoproliferation occurs; however, the new vessel growth is composed of “leaky” vessels with poorly formed endothelial tight junctions. Potential serious complications include neovascular invasion of the vitreous and development of vitreoretinal adhesions, which may be followed by hemorrhage and retinal detachment.43
Cornea
Induction by the developing anterior epithelium of the lens signals multiple steps in corneal development, at about the time the lens vesicle separates from the surface ectoderm (day 33).12,17,44 One or two layers of epithelial cells from surface ectoderm become aligned and will form the corneal epithelium. During the sixth week, zonula occludens are evident.1 The first component of the anchoring system is the basal lamina, which is evident by week 9, and hemidesmosomes are present by week 13.45 By the fifth or sixth month, all the cellular layers of the corneal epithelium are present.46 Corneal endothelium, formed from the first wave of mesenchyme that migrates into the space between the corneal epithelium and the lens, is one to two cells thick by week 6.3 At 4 months the endothelium is a single row of flattened cells with a basal lamina, the first evidence of Descemet’s membrane.3,47 By the middle of the fourth month, tight junctions are apparent in the endothelium, coinciding with the beginning of aqueous formation.48 The material comprising Descemet’s membrane before birth has a banded appearance, whereas the tissue secreted by the endothelium after birth (which has a more posterior position) has a homogeneous, unbanded appearance.49,50
By week 8, a second wave of mesenchyme proliferates, migrates between the developing epithelium and endothelium, and gives rise to the fibroblasts, collagen, and ground substance of the stroma.3,51 A third wave of mesenchyme migrates into the area between the developing endothelium and lens, giving rise to the pupillary membrane. These three waves of mesenchyme, as well as that giving rise to the sclera, are of neural crest origin (see Box 7-1).3
At 3 months all layers of the cornea are present (Figure 7-14) except Bowman’s layer, which appears during the fourth month45 and is presumably formed by fibroblasts of the anterior stroma and secretions of the epithelial cells;52,53 whatever the stage, Bowman’s layer is always acellular.49 Fibroblast arrangement and subsequent production of collagen fibrils begins in posterior corneal stroma and proceeds anteriorly. Rapid growth of the corneal stroma causes an increase in curvature relative to the rest of the globe.5 At birth the cornea is circular and steep (55D); the curvature decreases to 44D at age 6 months1.
Sclera
The sclera first develops anteriorly from condensations in the mesenchyme near the limbus. Growth continues posteriorly until the sclera reaches the optic nerve, and by the end of the third month the sclera has surrounded the developing choroid.26 During the fourth month connective tissue fibers cross the posterior scleral foramen, running through the optic nerve fibers and producing the first connective tissue strands of the lamina cribrosa.3 By the fifth month, the sclera (including the scleral spur) is well differentiated.4
UVEA
Choroid
The mesenchyme that forms the choriocapillaris must be in contact with the developing pigment epithelium to differentiate.4,6 The vessels appear during the second month, and the diaphragm-covered fenestrations are evident by week 12.28 Bruch’s membrane develops during month 4. During the fifth month the layers of the large and medium vessels are evident, as are the vessels that will become vortex veins.54 The short posterior ciliary arteries also are evident and begin to anastomose to form the circle of Zinn.3
At midterm in fetal development the elastic sheet of Bruch’s membrane is present, the basement membrane of the RPE is developing, and the collagenous layers are thickening. The basement membrane of the choriocapillaris is the last component to appear.3 By term the choroidal stroma is pigmented.28
Ciliary Body
The region of the outer layer of the optic cup, which will become the outer pigmented epithelium of the ciliary body, begins to form ridges late in the third month.55 The inner nonpigmented epithelium, from the inner optic cup layer, grows and folds with it. These folds, almost 70 in number, become the ciliary processes. Zonula occludens are evident in the nonpigmented epithelium during the third month.1 Neural crest cells differentiate into stromal elements; the fenestrations in the capillaries in the processes are visible in week 14.3,55 During the fourth month the major arterial circle of the iris is formed by the anastomosing long ciliary arteries and replaces the annular vessel.56 Gap junctions and desmosomes appear, joining the apices of the two epithelial layers during the fourth month. The ciliary muscle begins to develop from neural crest44 during the fifth month. However, the annular muscle (of Müller) remains incomplete at birth.3,4 Aqueous humor production begins at 4 to 6 months of gestation.55
Iris
By the end of the third month, the lip of the optic cup begins to elongate and grows between the lens and the developing cornea. The outer layer of the optic cup becomes the anterior iris epithelium and the inner layer forms the posterior iris epithelium; these layers remain separated from each other for a time by the marginal sinus. The proliferation of myofilaments in the basal aspect of the anterior epithelium adjoining the stroma transforms the layer into myoepithelium.57 The group of cells that will become the iris sphincter breaks away from the pupillary zone of this epithelial layer during the fifth month and develops into smooth muscle within the iris stroma.6,57 During the sixth gestational month the fibers of the dilator muscle continue to develop within the epithelial layer, and both muscles are completed by birth. That the sphincter and dilator come from neural ectoderm is unusual because most muscle tissue is derived from mesenchyme. Pigmentation in the anterior and posterior epithelium begins to appear at approximately week 10 and is complete during the seventh month. The marginal sinus disappears and the two epithelial layers are joined at their apices by intercellular junctions.3
Mesenchymal cells line up, leaving large gaps between them, to form the anterior border layer. The stromal components are of neural crest origin and are said to migrate from the second wave of mesenchyme.1,58 A sparse distribution of collagen fibers begins to accumulate to form the iris stroma.3 Stromal melanocytes continue to produce more pigment, and the color of the iris can continue to darken for the first 6 postnatal months, with some stromal organization not complete until age 7 years.1
Pupillary Membrane
As the lens thickens, its anterior vascular tunic disconnects from the annular vessel, and its constituents are incorporated into the iris stroma; remnants contribute to the minor circle of the iris.3 During the third month, the pupillary membrane forms between the lens epithelium and the corneal endothelium to replace the vascular tunic. This transitory membrane contains components from the third wave of mesenchyme and branches from the major circle of the iris.1,59 (The pupillary membrane can be seen in Figure 7-15 just anterior to the lens.) Three or four arcades of thin-walled blood vessels separated by a thin mesodermal membrane are completed by the end of the fifth month.59 The vessels of the pupillary membrane cannot be identified as arterial or venous on the basis of their histologic makeup.22
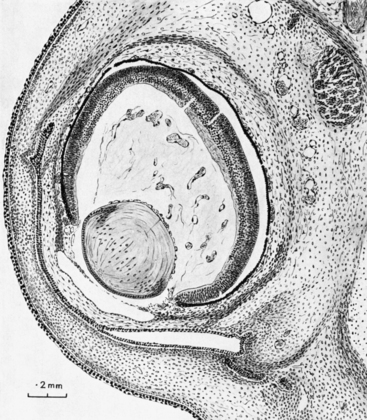
FIGURE 7-15 Section through eye and surrounding structures in 35-mm human embryo (approximately 8 weeks).
(From Mann I: The development of the human eye, New York, 1964, Grune & Stratton. Copyright 1964, British Medical Association.)
During gestational month 6, the central vessels atrophy and become bloodless. The more peripheral vessels contribute to the minor circle of the iris, and by 8½ months the central vessels have fragmented and disappeared.6,59 As reabsorption of the central pupillary membrane occurs, the loops of the midregion form the ridge of the collarette, with other components incorporated into the anterior border layer.3
Clinical Comment: Persistent Pupillary Membrane
Remnants of the central portion of the pupillary membrane that do not reabsorb may be seen with a biomicroscope and appear similar to strands of a spider web attached to the surface of the iris. A persistent pupillary membrane may have a variety of presentations, from a single strand of connective tissue (anchored at one or both ends) to several interconnecting strands; pigment cells also might be incorporated. A persistent pupillary membrane is present in 17% to 32% of the population.59
Anterior Chamber
A mass of cells of neural crest origin and from the first wave of mesenchyme accumulates adjacent to the ciliary body and the iris root in the anterior chamber angle area.50,60 The method whereby this mass is eliminated to expose the angle remains controversial. The mass may atrophy,4 the structure may split between the iris and the meshwork, with some tissue contributing to each,61 or the intercellular spaces may enlarge and the cells reorganize into the surrounding tissue.62
The trabecular meshwork is visible as a triangular mass of mesenchymal cells during the fourth month; at least part of this tissue is of neural crest origin (see Box 7-1).63–65 The tissue progressively becomes more organized, and by 9 months the trabecular beams and pores are well developed, with the intratrabecular spaces and pores likely formed by programmed cell death.
Schlemm’s canal is derived from the deep scleral plexus.63,64 During the fourth month, tight junctions are evident in the canal’s endothelial lining.62 During the seventh month, Schlemm’s canal is fully formed in some quadrants; during the eighth month, giant vacuoles are seen in the endothelial lining, and the complete circular canal is present during the ninth month.
Once formed, the anterior chamber is lined by a continuous endothelium that covers the trabecular meshwork and the iridocorneal angle.66 This membrane appears continuous at gestational month 7 but is discontinuous in the region of the meshwork by month 9.62,67 During the last few weeks before birth, splits occur between cells in the membrane, and the size and number of these splits increase rapidly because of the increase in the size of the anterior ocular structures.67 The loss of continuity in this membrane over the trabecular meshwork correlates significantly with an increase in the facility of aqueous outflow.63 Persistence of the uninterrupted endothelial membrane over the meshwork (Barkan’s membrane) can be a causative factor in congenital glaucoma.3,8,67
Vitreous
The presence of the developing lens is essential for normal accumulation of vitreous.68 The primary vitreous fills the vitreous space early in development (see Figure 7-8) and has both mesenchymal and ectodermal origins. Fibrils derived from the developing lens and from the developing retina and components from the degenerating hyaloid system will form the primary vitreous.62
As the secondary vitreous develops, apparently produced by neural retina and hyalocytes from the primary vitreous,1 it encloses the primary vitreous within the region of the atrophying hyaloid vessels, thus forming the funnel-shaped Cloquet’s canal. This zone, with its apex at the optic disc and its base at the posterior lens, is well formed by the fourth month. It persists in the adult. The secondary vitreous contains a fibril network and primitive hyalocytes.3 During the third month, a thickening of secondary vitreous in the anterior peripheral area occurs (the marginal bundle of Druault); it forms attachments at the vitreous base and at the hyaloideocapsular ligament.1
The zonule fibers develop in the area between the lens equator and the ciliary body. They have been called tertiary vitreous because they do arise in the vitreous and were assumed to be collagenous, but now are believed to be noncollagenous.1 Questions remain about whether the tertiary vitreous is mesenchymal or epithelial in origin.3 The fibers forming the zonules pass through the marginal bundle of Druault at right angles. Early zonule fibers appear to be a continuation of a thickening of the internal limiting membrane of the ciliary body, formed by the ciliary epithelium; the fibers run from a zone near the ora serrata and from the valleys between the processes to the lens capsule.5 The zonules are well formed during the seventh month.
Optic Nerve
The optic stalk, the precursor of the optic nerve, joins the optic vesicle to the forebrain. As the optic fissure develops along the inferior stalk invagination, a two-layered optic stalk is created. The outer layer of the optic stalk becomes the neuroglial sheath that surrounds the optic nerve; it also gives rise to the glial components of the lamina cribrosa.3 Programmed cell death occurs in the cells of the inner layer, providing an avenue for passage of the axons from ganglion cells entering the optic stalk; other cells of the inner wall become the glial cells of the optic nerve. As apoptosis occurs in the ganglion cell population, the number of axons in the optic nerve decreases from 2.6 million during the second month to 1.1 million in the eighth month1. This decrease makes room for the increase in glial and connective tissue processes that enter the optic nerve.
A band of glial tissue forms around the optic disc, at the junction of the inner and outer layers of the optic cup, thus separating the potential intraretinal space from the fibers of the optic nerve; this tissue will become the intermediary tissue of Kuhnt.1 Ganglion cell axons fill the lumen of the optic nerve (Figure 7-16) and grow toward their termination in the lateral geniculate nucleus. Myelination of the axon begins during the fifth month of gestation once the fiber reaches the lateral geniculate nucleus. Myelination reaches the chiasm during the sixth month, and the lamina cribrosa 1 to 3 months after birth.3,69 Normally no myelin continues into the retina past the lamina cribrosa.
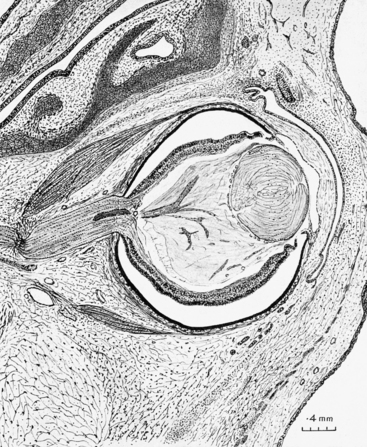
FIGURE 7-16 Section through eye and orbit of 48-mm human embryo (approximately 9.5 weeks).
(From Mann I: The development of the human eye, New York, 1964, Grune & Stratton. Copyright 1964, British Medical Association.)
Clinical Comment: Emmetropization
The globe continues to grow after birth, and the eye will become emmetropic as long as there is coordination between the length of the eye and the power of the refractive components. Although this growth is under genetic control, visual experience that provides feedback for normal growth may influence this process.1
Development of Ocular Adnexa
Eyelids
Early in the second gestational month, folds of surface ectoderm filled with mesenchyme begin to grow toward one another anterior to the developing cornea; these folds will become the eyelids. Early formation of these folds can be seen in Figure 7-8. The upper fold is from the frontonasal process, and the lower fold is from the maxillary process.1 The eyelid margins meet and fuse during the third month of development and remain fused until the lid structures have developed (Figure 7-17).25 Two layers of epithelium cover the anterior surface to become epidermis and one layer lines the inner surface to become conjunctiva.70 The tarsal plates are the first structures evident and then epithelial buds from the margins of the eyelid folds grow into the developing tarsal plates to form meibomian glands.1 The epithelial layers of the skin and conjunctiva, the hair follicles and cilia, and the meibomian glands, Zeis glands, and glands of Moll all develop from surface ectoderm; the tarsal plates, the orbicularis, the levator, and the tarsal muscle of Müller develop from mesenchyme.3 The fusion of the eyelids isolates the developing eye from the amniotic fluid71 and “probably prevents the cornea and conjunctiva from keratinizing.”3 The desmosomes joining the margins of the fused lids break down during the fifth and sixth month, allowing the eyelids to separate; the lipid secretions of the meibomian glands may be responsible for this disjunction.3,5
Orbit
Orbital fat and connective tissue are derived from neural crest cells. The first evident orbital bone is the maxilla at 6 weeks; the frontal, zygomatic, and palatine bones are apparent at week 7. The lesser wing of the sphenoid bone and the optic canal are present at week 7, the greater wing is evident at week 10, and the wings join at week 16.72 Most of the orbital bones ossify and fuse between the sixth and seventh months.3 The angle between the orbits early in development is approximately 180 degrees, decreases to 105 degrees at 3 months, and is 71 degrees at birth and 68 degrees in adulthood (Figure 7-18). The globe reaches its adult size by age 3 years, but the orbit is not of adult size until age 16 years.3
Extraocular Muscles
The extraocular muscles are of mesenchymal origin (Figure 7-19). The muscle cells are derived from mesoderm, whereas the connective tissue components originate in neural crest.1,44 Extraocular muscles once were thought to develop in stages, first posteriorly near the orbital apex and then grow forward,4 but recent investigation suggests that muscle origin, belly, and insertion develop simultaneously.73 The muscles innervated by cranial nerve III are derived from the first pair of somites at approximately day 26. The lateral rectus muscle, innervated by cranial nerve VI, develops from the mesenchyme of the maxillomandibular area at about day 27. The superior oblique muscle, innervated by cranial nerve IV, is derived from the second pair of somites at day 29.1 The tendinous sheaths at the scleral insertions are not fully formed until 18 months of age.1 The newborn exhibits poorly coordinated eye movements during the early years, indicating that the extraocular muscles are not fully developed and early visual experience can influence the development of normal binocular eye movements.74
Nasolacrimal System
The main lacrimal gland has long been thought to develop from epithelial buds that arise from the temporal portion of the conjunctiva of the superior fornix.3 Some investigators question this origin and suggest a neural crest origin.75 The lacrimal gland continues to develop after birth and is not fully developed until age 3 or 4 years.3
The nasolacrimal drainage system develops from a cord of surface ectodermal cells that becomes buried below the maxillary mesenchyme. This cord may fragment and form the canaliculi, the nasolacrimal duct, and the lacrimal sac, or the canaliculi might be formed from a later epithelial cord.3
Blood Vessel Permeability and Barriers
The blood-retinal and blood-aqueous barriers are recognizable early in development in the tight junctions formed in the RPE, the non pigmented ciliary epithelium, and the capillaries of the iris and retina. Fenestrations that establish vessel permeability in the capillaries of the ciliary processes and the choriocapillaris also are evident early in the gestational period.
Genetic Implications
With the current interest in the human genome, the field is growing exponentially and numerous studies are exploring and identifying genes expressed by ocular structures and the mechanisms by which cellular characteristics and processes are governed by those genes. The PAX6 gene is considered the master control gene and is necessary for normal development of ocular structures.76,77 An increase in PAX6 in mice is associated with multiple lens defects, including abnormal fiber shape and fiber-to-fiber and fiber-to-cell interactions.53 The PAX6 gene is expressed in the corneal and conjunctival epithelium and may regulate and maintain cell structure; it may also have a role in the proliferation and maintenance of corneal and conjunctival stem cells.76,77
Myriad speculations surround genes and the proteins they encode. Some interesting theories concerning ocular structures include: clusterin might be the factor essential for preserving the nonkeratinized state of corneal epithelial cells and may also provide some protection against apoptosis;78 the gene, ALDH3, may provide some protection against UVR damage to corneal epithelium;78 there may be a connection between atherosclerosis and drusen formed in AMD through the same or similar extracellular matrix genes;79 and genes identified in the aqueous outflow tissues are usually associated with lymphatic tissue, perhaps suggesting additional function for the trabecular meshwork.79
Marfan’s syndrome is a disease affecting connective tissue structures throughout the body. Several genetic mutations have been identified, which lead to faulty production or a reduction in the secretion or assembly of fibrillin molecules.80 Marfan’s related disorders are also associated with abnormalities in extracellular matrix formation. An individual with Marfan’s often has tall and thin body stature and can be at increased risk for aortic enlargement. Ocular structures can be affected; high myopia is often present and can increase the risk of retinal detachment; zonular malformation or dysfunction can result in a dislocated lens.
The gene called RAX is thought to be a major factor in the early stages of ocular development and mutations in RAX have been identified as causative in some cases of anophthalmia.81 Mutations in the PAX2 gene have been implicated in papillorenal syndrome, which is characterized by renal hypoplasia and optic nerve head coloboma.82
1. Barishak Y.R. Embryology of the eye and its adnexae. New York: Karger; 2001.
2. Moore K.L. Before we are born: essentials of embryology and birth defects, ed 5. Philadelphia: Saunders; 1998.
3. Cook C.S., Ozanics V., Jakobiec F.A. Prenatal development of the eye and its adnexa. Tasman W., Jaeger E.A., editors. Duane’s foundations of clinical ophthalmology, vol 1. Philadelphia: Lippincott, 1994.
4. Mann I. Development of the human eye. New York: Grune & Stratton; 1964.
5. Barishak Y.R. Embryology of the eye and its adnexae. In: Straub W., editor. Developments in ophthalmology. New York: Karger, 1992.
6. Barber A.N. Embryology of the human eye. St Louis: Mosby; 1955.
7. Morrison D.A., FitzPatrick D.R., Fleck B.W. Iris coloboma with iris heterochromia: a common association. Arch Ophthalmol. 2000;118(11):1590.
8. Trachimowicz R.A. Review of embryology and its relation to ocular disease in the pediatric population. Optom Vis Sci. 1994;71(3):154.
9. Smelser G.K. Embryology and morphology of the lens. Invest Ophthalmol. 1965;4:398.
10. Matsuo T. The genes involved in the morphogenesis of the eye. Jpn J Ophthalmol. 1993;37(3):215. (abstract)
11. Harrington L., Klintworth G.K., Seror T.E., et al. Developmental analysis of ocular morphogenesis in alpha A-crystallin/diphtheria toxin transgenic mice undergoing ablation of the lens. Dev Biol. 1991;148(2):508.
12. Coulombre A.J. Regulation of ocular morphogenesis. Invest Ophthalmol. 1969;8:25.
13. McKeehan M.S. Induction of portions of the chick lens without contact with the optic cup. Anat Rec. 1958;132:297.
14. Grainger R.M., Henry J.J., Saha M.S., et al. Recent progress on the mechanisms of embryonic lens formation. Eye. 1992;6(pt 2):117.
15. Marshall J., Beaconsfield M., Rothery S. The anatomy and development of the human lens and zonules, Trans Ophthalmol Soc UK. 1982;102:423-440.
16. Coulombre J.L., Coulombre A.J. Lens development: fiber elongation and lens orientation. Science. 1963;142:1489.
17. Coulombre J.L., Coulombre A.J. Lens development. IV. Size, shape and orientation. Invest Ophthalmol. 1969;8:251.
18. O’Rahilly R. The prenatal development of the human eye. Exp Eye Res. 1975;2:93.
19. Amaya L., Taylor D., Russell-Eggitt I.R., et al. The morphology and natural history of childhood cataracts. Surv Ophthalmol. 2003;48(2):125.
20. Nelson L.B., Folberg R. Ocular development anomalies. Tasman W., Jaeger E.A., editors. Duane’s foundations of clinical ophthalmology, vol 1. Philadelphia: Lippincott, 1991.
21. Kanski J.J. Clinical ophthalmology, ed 2. Oxford, UK: Butterworth-Heinemann; 1989. p 238
22. Mutlu F., Leopard I.H. The structure of the fetal hyaloid system and tunica vasculosa lentis. Arch Ophthalmol. 1964;71:102.
23. Jack R.L. Ultrastructural aspects of hyaloid vessel development. Arch Ophthalmol. 1972;87:427.
24. Zhu M., Madigan M.C., van Driel D., et al. The human hyaloid system: cell death and vascular regression. Exp Eye Res. 2000;70:767.
25. Warwick R. Eugene Wolff’s anatomy of the eye and orbit, ed 7. Philadelphia: Saunders; 1976. p 418
26. Duke-Elder S., Cook C. Normal and abnormal development. Duke-Elder S., editor. System of ophthalmology. Embryology. vol 3. St Louis: Mosby; 1963.
27. Hollenberg M.J., Spira A.W. Early development of the human retina. Can J Ophthalmol. 1972;7:472.
28. Mund M.L., Rodrigues M.M., Fine B.S. Light and electron microscopic observations on the pigmented layers of the developing human eye. Am J Ophthalmol. 1972;73:167.
29. Smelser G.K., Ozanics V., Rayborn M., et al. The fine structure of the retinal transient layer of Chievitz. Invest Ophthalmol. 1973;12:504.
30. Uga S., Smelser G.K. Electron microscopic study of the development of retinal Müllerian cells. Invest Ophthalmol. 1973;12:295.
31. Isenmann S., Kretz A., Cellerino A. Molecular determinants of retinal ganglion cell development, survival, and regeneration. Prog Retin Eye Res. 2003;22(4):483. (abstract)
32. Oster S.F., Sretavan D.W. Connecting the eye to the brain: the molecular basis of ganglion cell axon guidance. Br J Ophthalmol. 2003;87:639. (abstract)
33. Narayanan K., Wadhwa S. Photoreceptor morphogenesis in the human retina: a scanning electron microscopic study. Anat Rec. 1998;252:133.
34. Willbold E., Layer P.G. Müller glia cells and their possible roles during retina differentiation in vivo and in vitro. Histol Histopathol. 1998;13(2):531.
35. Smelser G.K., Ozanics V., Rayborn M., et al. Retinal synaptogenesis in the primate. Invest Ophthalmol. 1974;13:340.
36. Sharma R.K., Ehinger E.J. Development and structure of the retina. In: Kaufman P.L., Alm A., editors. Adler’s physiology of the eye. St Louis: Mosby, 2003. p 319
37. Vrabec F. The temporal raphée of the human retina. Am J Ophthalmol. 1966;62:926.
38. O’Connor A.R., Wilson C.M., Fielder A.R. Ophthalmological problems associated with preterm birth Eye. 2007;21:1254-1260.
39. Yuodelis C., Hendrickson A. A qualitative and quantitative analysis of the human fovea during development. Vision Res. 1986;26(6):847.
40. Jeffery G. The retinal pigment epithelium as a developmental regulator of the neural retina. Eye. 1998;12:499.
41. Dorrell M.I., Aguilar E., Friedlander M. Retinal vascular development is mediated by endothelial filopodia, a preexisting astrocytic template and specific R-cadherin adhesion. Invest Ophthalmol Vis Sci. 2002;43(11):3500.
42. Provis J.M. Development of the primate retinal vasculature. Prog Retin Eye Res. 2001;20(6):799. (abstract)
43. Patz A., Payne J.W. Retrolental fibroplasia. Duane T.D., Jaeger E.A., editors. Clinical ophthalmology, vol 3. Philadelphia: Harper & Row, 1982.
44. Gage J.E., Rhoades W., Prucka S.K., et al. Fate maps of neural crest and mesoderm in the mammalian eye. Invest Ophthalmol Vis Sci. 2005;46:4200-4208.
45. Tisdale A.S., Spurr-Michaud S.J., Rodrigues M., et al. Development of the anchoring structures of the epithelium in rabbit and human fetal corneas. Invest Ophthalmol Vis Sci. 1988;29(5):727.
46. Zinn K.M., Mockel-Pohl S. Fine structure of the developing cornea. Int Ophthalmol Clin. 1975;15(1):19.
47. Wulle K.G. Electron microscopy of the fetal development of the corneal endothelium and Descemet’s membrane of the human eye. Invest Ophthalmol. 1972;11:897.
48. Wulle K.G., Ruprecht K.W., Windrath L.C. Electron microscopy of the development of the cell junctions in the embryonic and fetal human corneal endothelium. Invest Ophthalmol. 1974;13:923.
49. Lesueur L., Arne J.L., Mignon-Conte M., et al. Structural and ultrastructural changes in the developmental process of premature infants’ and children’s corneas. Cornea. 1994;13(4):331.
50. Bahn C.F., Falls H.F., Varley G.A., et al. Classification of corneal endothelial disorders based on neural crest origin. Ophthalmology. 1984;91:558.
51. Ozanics V., Rayborn M., Sagun D. Some aspects of corneal and scleral differentiation in the primate. Exp Eye Res. 1976;22:305.
52. Wulle K.G., Richter J. Electron microscopy of the early embryonic development of the human corneal epithelium. Albrecht Von Graefes Arch Klin Exp Ophthalmol. 1978;209(1):39.
53. Duncan M.K., Kozmik Z., Cveklova K., et al. Overexpression of PAX6(5a) in lens fiber cells results in cataract and upregulation of (alpha)5(beta)1 integrin expression. J Cell Sci. 2000;113:3173.
54. Heinmann K. The development of the choroid in man: choroidal vascular system. Ophthalmol Res. 1971;3:257.
55. Wulle K.G. The development of the productive and drainage system of the aqueous humor in the human eye. Adv Ophthalmol. 1972;26:296.
56. Loewenfeld I.E. The pupil: anatomy, physiology, and clinical applications. Boston: Butterworth-Heinemann; 1999.
57. Tamura T., Smelser G.K. Development of the sphincter and dilator muscles of the iris. Arch Ophthalmol. 1973;89:332.
58. Carlson B.M. Human embryology and developmental biology. St Louis: Mosby; 1994. p 265
59. Matsuo N., Smelser G.K. Electron microscopic studies on the pupillary membrane: the fine structure of the white strands of the disappearing stage of the membrane. Invest Ophthalmol. 1971;10:108.
60. Edelhauser H.F., Ubels J.L. Cornea and sclera. In: Kaufman P.L., Alm A., editors. Adler’s physiology of the eye. St Louis: Mosby; 2003:p 47.
61. Burian H.M., Braley A.E., Allen L. A new concept of the development of the angle of the anterior chamber of the human eye. Arch Ophthalmol. 1956;53:439.
62. Smelser G.K., Ozanics V. The development of the trabecular meshwork in primate eyes. Am J Ophthalmol. 1971;71:366.
63. Rodrigues M.M., Katz S.I., Foidart J.M. Collagen factor VIII antigen, and immunoglobulins in the human aqueous drainage channels. Ophthalmology. 1980;87:337.
64. Tripathi B.J., Tripathi R.C. Neural crest origin of human trabecular meshwork and its implications for the pathogenesis of glaucoma. Am J Ophthalmol. 1989;107:583.
65. Tripathi B.J., Tripathi R.C. Embryology of the anterior segment of the human eye. In: Ritch R., Shields M.B., Krupin T., editors. The glaucomas. St Louis: Mosby, 1989.
66. Kupfer C., Ross K. The development of outflow facility in human eyes. Invest Ophthalmol. 1971;10:513.
67. Hansson H.A., Jerndal T. Scanning electron microscopic studies on the development of the iridocorneal angle in human eyes. Invest Ophthalmol. 1971;10:252.
68. Coulombre A.J., Coulombre J.L. Mechanisms of ocular development. Int Ophthalmol Clin. 1975;15(1):7.
69. Sadun A.A., Glaser J.S. Anatomy of the visual sensory system. Tasman W., Jaeger E.A., editors. Duane’s foundations of clinical ophthalmology, vol 1. Philadelphia: Lippincott, 1994.
70. Kikkawa D.O., Lucarelli M.J., Shovlin J.P., et al. Ophthalmic facial anatomy and physiology. In: Kaufman P.L., Alm A., editors. Adler’s physiology of the eye. St Louis: Mosby; 2003:p 16.
71. Anderson H., Ehlers N., Matthiessen M.E., et al. Histochemistry and development of the human eyelids. II. A cytochemical and electron microscopical study. Acta Ophthalmol (Copenh). 1967;45:288.
72. Sires B.S., Gausas R., Cook B.E., et al. Orbit. In: Kaufman P.L., Alm A., editors. Adler’s physiology of the eye. St Louis: Mosby, 2003.
73. Sevel D. Reappraisal of the origin of human extraocular muscles. Ophthalmology. 1981;88:1330.
74. Porter J.D., Andrade F.H., Baker R.S. The extraocular muscles. In: Kaufman P.L., Alm A., editors. Adler’s physiology of the eye. St Louis: Mosby; 2003:p 787.
75. Tripathi B.J., Tripathi R.C. Evidence of neuroectodermal origin of the human lacrimal gland. Invest Ophthalmol Vis Sci. 1990;31:393.
76. Koroma B.M., Yang J.M., Sundin O.H. The PAX6 homeobox gene is expressed throughout the corneal and conjunctival epithelia. Invest Ophthalmol Vis Sci. 1997;38(1):108.
77. Secker G.A., Daniels J.T. Corneal epithelial stem cells: deficiency and regulation, Stem Cell Res. 2008;4:159-168.
78. Kinoshita S., Adachi W., Sotozono C., et al. Characteristics of the human ocular surface epithelium. Prog Retin Eye Res. 2001;20(5):639.
79. Wistow G., The N.E.I. Bank project for ocular genomics: data-mining gene expression in human and rodent eye tissues. Prog Retin Eye Res. 2006;25:43-77.
80. Bonetti M.I. Microfibrils: a cornerstone of extracellular matrix and a key to understand Marfan syndrome. Ital J Anat Embryol. 2009;114:201-224.
81. Lequeux L., Rio M., Vigouroux A., et al. Confirmation of RAX gene involvement in human anophthalmia, Clin Genet. 2008;74:392-395.
82. Martinovic-Bouriel J., Benachi A. PAX2 mutations in fetal renal hypodysplasia. Am J Med Genet. 2010;152A:830-835.