Chapter 101 Neurologic Disorders in Children with Heart Disease
Neurologic Conditions Associated with Congenital Heart Disease
Children with congenital heart disease (CHD) and its complications comprise one of the largest inpatient populations in major pediatric centers, and neurologic dysfunction is one of the most common extracardiac complications of CHD. The mortality of CHD has decreased markedly over the years, especially among the most critically ill infants at greatest risk of brain dysfunction. As neurodevelopmental integrity is a major factor determining quality of life, the prevention of neurologic dysfunction is central to the management of these children. Reliable estimates of both the incidence of CHD and the prevalence of neurologic dysfunction are difficult to obtain and vary widely across studies [Reller et al., 2008; Rosenthal, 1998]. This variation is due to differences in the diagnostic criteria and classification for both CHD and its neurologic complications. A recent review of 62 studies reported CHD incidences ranging from 4 to 75 per 1000 live births; the lower rates apply to moderate and severe CHD lesions, while the higher rates include even trivial CHD anomalies, such as small muscular ventricular septal defects [Hoffman and Kaplan, 2002]. The most reliable data are those for infants with “critical CHD” (i.e., requiring an interventional procedure during the first year of life) [Benson, 1989; Castaneda et al., 1974, 1989], which have an incidence around 1.5 per 1000 of all births [Reller et al., 2008].
Trends in Cardiac Surgery and Associated Neurologic Complications
The birth of pediatric cardiac surgery was in 1938, when Frey in Düsseldorf and Gross in Boston performed the first successful ligations of patent ductus arteriosus [Gross and Hubbard, 1984; Kaemmerer et al., 2004]. Over the subsequent seven decades, advances in CHD management have been associated with changes in the etiologic and clinical profile of neurologic dysfunction in children with CHD. In the years prior to cardiopulmonary bypass (CPB), neurologic complications of CHD were related, in large part, to the effects of right-to-left shunting and chronic cyanosis [Berthrong and Sabiston, 1951; Cottrill and Kaplan, 1973; Martelle and Linde, 1961; Phornphutkul et al., 1973; Terplan, 1976; Tyler and Clark, 1957a, 1957b]. The clinical application of CPB by Kirklin and Lillehei in 1955 [Moller et al., 2009] provided the extracorporeal circulatory support necessary for open-heart surgery. However, repair of complex CHD lesions in the newborn and young infant remained a major surgical challenge for almost two decades, due to the small heart size and the constricted surgical field. During this period, surgical options for complex CHD lesions were limited to repeated palliative procedures while awaiting sufficient physical growth to permit definitive corrective procedures. This delay to definitive cardiac repair exposed infants and children to the risk of cumulative brain injury from chronic hypoxia, polycythemia, and right-to-left shunts, as well as from the effects of repeated CPB and surgery [Newburger et al., 1984].
Primary neonatal correction of many complex CHD lesions became possible in the early 1970s, when Barrat-Boyes in Auckland and Castaneda in Boston began using intraoperative deep hypothermia to suppress cellular metabolism and oxygen demand. In this manner, deep hypothermia enabled maneuvers such as low-flow CPB and circulatory arrest to clear the surgical field of blood and cannulae, optimizing exposure during critical phases in the repair of small and complex CHD lesions. As a result of these techniques, neonatal heart repair has become commonplace in many major centers since the 1980s, significantly increasing the survival of infants with complex CHD lesions. In fact, the hospital mortality for neonatal cardiac procedures in most large centers has fallen to less than 3 percent [Wernovsky, 2006]. Unfortunately, these intraoperative support techniques were not without neurologic risk and, as a result, the prevalence of perioperative neurologic morbidity of infant cardiac surgery did not parallel the decrease in mortality. Although neonatal cardiac repair reduced exposure to earlier risk factors, such as chronic hypoxia, a paradoxical increase in other forms of neurologic morbidity was noted. First, severe and previously lethal CHD lesions often were associated with significant perioperative hemodynamic shock and acidosis, the long-term neurologic consequences of which were now becoming evident in the growing population of CHD survivors. Second, the intraoperative support techniques responsible for increasing survival had their own inherent risk for neurologic injury. Consequently, in a significant minority of infants, the increase in survival and decrease in cardiac morbidity associated with severe CHD was replaced by chronic, often lifelong, neurodevelopmental dysfunction. This unacceptable situation triggered a period of intense clinical and research focus on advancing intraoperative neuroprotection during these procedures. Based on a number of randomized clinical trials over the past two decades (discussed below), substantial changes have occurred in the perioperative management of CHD. Whether due directly to these clinical changes or to more general advances in the field, the incidence of major neurologic complications following neonatal cardiac surgery appears to be decreasing in recent years.
Neurologic Complications of Congenital Heart Disease Prior to Anatomic Intervention
Brain Anomalies of Fetal Onset Associated with CHD
Primary brain dysgenesis in congenital heart disease
In earlier autopsy studies of children with CHD, the prevalence of brain malformations ranged from 10 to 29 percent [Glauser et al., 1990b; Jones, 1991; Miller and Vogel, 1999; Terplan, 1976] (Figure 101-1). The prevalence of dysgenetic brain lesions appears to be increased particularly in certain cardiac lesions [Glauser et al., 1990b; Jones, 1991; Terplan, 1976]. For example, a range of cerebral malformations, from microdysgenesis to gross malformations, such as holoprosencephaly, has been described at autopsy in infants with hypoplastic left heart syndrome (HLHS) [Glauser et al., 1990b]. Clinically, these malformations may present in the newborn period with seizures, alterations in level of consciousness, and abnormalities in motor tone, or may remain clinically silent until later infancy and childhood, when they present with developmental delay, epilepsy, and cerebral palsy. The in vivo diagnosis of cerebral dysgenesis is best confirmed by brain magnetic resonance imaging (MRI). Given the decreasing availability and inherent bias of autopsy studies, the increasing sophistication of MRI will be invaluable for the more precise delineation of the relationship between CHD and brain dysgenesis.
Children with certain chromosomal syndromes, particularly aneuploidy, are at increased risk for combined malformations of the heart and brain. Approximately 40 percent of children with trisomy 21 have CHD, typically endocardial cushion defects [Eskedal et al., 2004; Vergara et al., 2006]. The most common cardiac lesions in both trisomies 13 and 18 are ventricular septal defects and patent ductus arteriosus. Conversely, the spectrum of brain malformations in these trisomy syndromes is broad.
With a prevalence of 1:4000–5000, chromosome 22 deletion syndromes are the most common microdeletion disorders in man [Aggarwal and Morrow, 2008], and commonly have features of both cardiac anomalies and neurologic dysfunction.[Morrow et al., 1995] The phenotypic similarities across these syndromes have led to the acronym CATCH-22 spectrum (cardiac defect, abnormal facies, thymic hypoplasia, cleft palate, hypocalcemia, chromosome 22q11 deletions) [Demczuk et al., 1995; Driscoll, 1994; Lindsay et al., 1995; Morrow et al., 1995; Shprintzen et al., 1992]. This spectrum includes the DiGeorge’s and velocardiofacial (Shprintzen’s) syndromes. A second genetic locus for DiGeorge’s syndrome and velocardiofacial syndrome (DGS2) has also been identified on chromosome 10p [Bartsch et al., 2003; Daw et al., 1996].
The most common cardiac defects in DiGeorge’s syndrome are conotruncal lesions, including interrupted aortic arch type B, truncus arteriosus, and tetralogy of Fallot. Features typical of the velocardiofacial syndrome include cleft palate or velopharyngeal insufficiency and a typical facial appearance (Figure 101-2), with a broad prominent nose and retrognathia. Ventricular septal defects and tetralogy of Fallot are the most common cardiac defects in the velocardiofacial syndrome. A range of brain anomalies has been reported [Zinkstok and van Amelsvoort, 2005], the more common of which are midline defects in corpus callosum, septum pellucidum, and vermis development, cerebral and cerebellar “atrophy,” and white-matter abnormalities, including hyperintensities and frontal periventricular cysts [Chow et al., 1999; van Amelsvoort et al., 2001]. In addition, under-opercularization and polymicrogyria (especially of the insula and adjacent cortex) also have been described in chromosome 22 deletion syndromes [Bingham et al., 1997, 1998; Ghariani et al., 2002; Sztriha et al., 2004; Worthington et al., 2000]. The neurologic dysfunction in these syndromes is broad and may evolve over time. These children may present initially with hypocalcemic seizures, and subsequently they develop neurologic or cognitive disturbances, including impaired attention, executive and visuospatial function, and memory [Antshel et al., 2008; Bearden et al., 2001; Moss et al., 1995; Simon et al., 2005]. Early life oromotor dysfunction and expressive language delay is common; however, over time, the most consistent developmental pattern is that of significant discrepancy between verbal IQ and significantly worse performance IQ [Moss et al., 1995]; the mean IQ is around 70. This nonverbal learning disability [Swillen et al., 1999] is in keeping with significant visuoperceptual deficits in many of these children. During childhood, a unusual and inappropriately blunt affect emerges [Golding-Kushner et al., 1985], evolving in some cases into an autistic spectrum disorder, and in adulthood into psychosis [Gothelf et al., 2004; Pulver et al., 1994; Shprintzen et al., 1992; Vorstman et al., 2006].
The most common cardiac lesions seen in Williams’ syndrome are supravalvar aortic stenosis, peripheral pulmonary stenosis, and ventricular/atrial septal defects. These patients are prone to neurologic dysfunction [Ardinger et al., 1994; Chapman et al., 1995; Kaplan, 1995; Soper et al., 1995] and usually have obvious cognitive impairment; the average IQ is around 55. This is compounded further by learning and social disabilities. Gross motor, fine motor, and oromotor dysfunction is common, as are visuospatial and constructional difficulties [Chapman et al., 1995]. Children with Williams’ syndrome also may develop an intracranial arteriopathy with subsequent risk of stroke [Ardinger et al., 1994; Kaplan, 1995; Soper et al., 1995].
Acquired brain lesions in the fetus with congenital heart disease
Recent studies have explored the impact of CHD on fetal brain development. In a retrospective review of aortic stenosis-HLHS spectrum cases [Hinton et al., 2008], midgestation (19–22 weeks) fetal ultrasound measurements were compared with neonatal measurements. Several important observations were made in these subjects. First, more than half of these infants had a birth head circumference below the 10th percentile. Second, there was significant decrease in head circumference percentile over the third trimester. Third, fetuses with conditions associated with greater limitation of aortic arch blood flow documented greater impairment of head growth. Finally, there was a tendency for greater restriction of head growth than somatic growth [Hinton et al., 2008]. The only prospective MRI study to date to examine in vivo brain growth in fetuses with CHD versus control fetuses used volumetric measures of brain size and measures of fetal brain metabolism using 1H-MR spectroscopy [Limperopoulos et al., 2010]. This study revealed a progressive impairment in third-trimester brain growth and metabolism in fetuses with CHD (Figure 101-3). Reduced brain volume and brain N-acetyl aspartate (NAA)/choline ratios were most common in fetuses with HLHS and transposition of the great arteries (TGA); these CHD diagnoses were also responsible for the 20 percent of fetuses with elevated cerebral lactate, suggestive of anaerobic cerebral metabolism. The effects of these CHD diagnoses on cerebral volumetric growth also may underlie the increased incidence of disturbed late-gestation developmental processes in brain development, seen in children with HLHS and TGA. For example, incomplete insular closure, sometimes with polymicrogyria, both late-gestation events, has been described in close to 90 percent of newborns with TGA and HLHS [Licht et al., 2009]. In addition, a high prevalence of disturbances in the third-trimester events of cortical organization has been described in HLHS autopsy studies [Glauser et al., 1990a]. In summary, the findings of these studies suggest that brain growth might be most impaired in those fetuses with CHD resulting in decreased cerebral blood flow and/or hypoxemia. Other studies in newborns with CHD have described decreased pyramidal tract maturation (by diffusion tensor MRI tractography) [Partridge et al., 2006] and biochemical immaturity of the developing white matter (i.e., lower NAA/choline ratio using 1H-MR spectroscopy) [Miller et al., 2007], suggesting a fetal origin for these brain anomalies.
Acquired Neurologic Injury Between Birth and Anatomic Intervention
The increase in antenatal diagnosis of CHD has been associated with an improved overall outcome [Montana et al., 1996; Tworetzky et al., 2001; Verheijen et al., 2001], including a decreased rate of neonatal acidosis [Eapen et al., 1998; Kumar et al., 1999] and improved short-term neurologic outcome. This increase is, in large part, due to planned delivery and immediate neonatal care, especially for serious lesions such as HLHS [Mahle et al., 2001]. Furthermore, the advances in neonatal heart repair have reduced the delay to correction, thereby decreasing the brain’s exposure to the deleterious effects of an uncorrected cardiovascular anatomy (e.g., chronic hypoxia). However, while enabling surgical repair in increasingly smaller infants with immature cerebrovascular structure and function, these advances paradoxically have increased the risk of prematurity-related hemorrhagic (e.g., germinal matrix-intraventricular hemorrhage) and ischemic (e.g., periventricular leukomalacia) brain injury.
Preoperative Neurologic Complications
Preoperative neurologic dysfunction has been described in newborns with CHD, in some studies involving more than half of cases [Clancy et al., 2001; Fuller et al., 2009b; Limperopoulos et al., 1999a, 2000, 2001; Newburger et al., 1993; Robertson et al., 2004]. Clinical abnormalities described include muscle tone abnormalities, jitteriness, motor asymmetries, abnormal behavioral state regulation, decreased sensory (visual and auditory) responsiveness, and feeding difficulties. In one study, neurologic compromise was more prominent among newborns with acyanotic CHD [Limperopoulos et al., 1999a]. Congenital microcephaly, despite appropriate birth weight, has been reported in one-third or more of neonates with CHD, suggesting fetal-onset impaired brain growth [Licht et al., 2004; Limperopoulos et al., 1999b; Manzar et al., 2005; Shillingford et al., 2007]. Likewise, preoperative electroencephalographic (moderate, diffuse, background disturbances) and evoked potential abnormalities have been reported in over 40 percent of newborns with CHD [Limperopoulos et al., 1999b, 2000].
Neuroimaging studies in newborns with CHD, using either cranial ultrasound or MRI, have depicted a significantly increased prevalence of brain injury, largely clinically silent. Cranial ultrasound studies performed preoperatively and postoperatively in a cohort of infants undergoing cardiac surgery identified a 24 percent incidence of new lesions after cardiac surgery, with new hemorrhagic lesions in 6 percent of cases [Krull et al., 1994]. Preoperative cranial ultrasound abnormalities, including cerebral atrophy (41 percent), enlarged cerebrospinal fluid (CSF) spaces (26 percent), linear deep gray-matter echodensities (20 percent), intraventricular hemorrhage (16 percent), and parenchymal echodensities (16 percent), have been reported in 40–60 percent of newborns with CHD [Te Pas et al., 2005; van Houten et al., 1996]. The incidence of these cranial ultrasound abnormalities is greatest in newborns with aortic coarctation (especially with ventricular septal defect) or HLHS, ranging from 63 percent [Te Pas et al., 2005] to 71 percent [van Houten et al., 1996].
Recent MRI studies have confirmed this high prevalence of preoperative brain injury [Mahle et al., 2002; McConnell et al., 1990; McQuillen et al., 2006, 2007; Miller et al., 2004; Tavani et al., 2003], especially in infants with certain CHD lesions, including HLHS and TGA, as well as those with low preoperative oxygenation and longer time to surgery [Cheng, 2006; McQuillen et al., 2006]. In an MRI study of infants with HLHS, 23 percent documented preoperative evidence of focal or diffuse brain injury [Dent et al., 2005]. Procedures such as preoperative balloon-atrial septostomy also have been associated with evidence of infarction on MRI in some [Cheng, 2006; McQuillen et al., 2006], but not all, studies [Beca et al., 2009; Petit et al., 2009]. Prospective MRI studies have also increased the detection of periventricular leukomalacia (PVL) [Mahle et al., 2002; McConnell et al., 1990; McQuillen et al., 2006, 2007; Miller et al., 2004; Tavani et al., 2003], with some studies reporting a prevalence as high as 20 percent [Galli et al., 2004; Mahle et al., 2002]. PVL characteristically is a lesion of the premature infant, mediated by an interplay between the vulnerability of immature oligodendrocytes and insults such as cerebral ischemia. The fact that the preoperative PVL described in infants with CHD has been in term or near-term newborns has led to speculation that oligodendrocyte maturation is delayed in infants with CHD [du Plessis, 1997; Licht et al., 2009; Miller et al., 2007]. The notion of cerebral hypoperfusion in the newborn with CHD has been supported by studies indicating decreased cerebral perfusion (by arterial MRI spin-labeling) [Licht et al., 2004], as well as elevated cerebral lactate (by preoperative 1H-MR spectroscopy) in up to 53 percent of newborns with CHD – most commonly, those with TGA and single ventricle physiology [Ashwal et al., 1996, 2003; Mahle et al., 2002; Miller et al., 2004, 2007]. Table 101-1 summarizes the prevalence and type of preoperative brain injury in infants with CHD.
Neurologic Complications Following Anatomic Intervention
Two decades ago, the frequency of neurologic complications developing in the postoperative period following deep hypothermic cardiac surgery began to draw increasing attention. At that time, the prevalence of such complications was estimated to be as high as 25 percent in some centers [Ferry, 1987]. This finding led to a period of intense basic and clinical research into the mechanisms of intraoperative brain injury and its prevention, including several large clinical trials. Over the intervening years, the incidence of these complications appears to be decreasing in some [Menache et al., 2002], but not all, reports [Fallon, 1995; Kirkham, 1998]. In fact, it has been suggested that intraoperative (“procedure-specific”) factors are no longer major determinants of long-term outcome [Gaynor et al., 2007]. There is a clear need for a comprehensive and multicenter review of neurologic complications in the current era.
Mechanisms of Neurologic Injury during Deep Hypothermic Cardiac Surgery
Regardless of its frequency, intraoperative brain injury remains a major concern for children undergoing surgery for severe CHD. Intra- and perioperative hypoxic-ischemic/reperfusion (HI/R) insults generally are considered the principal cause of postoperative neurologic dysfunction. During open-heart surgery, a multitude of complex, dynamic, and interrelated phenomena may impact simultaneously on cerebral oxygen delivery and demand (Box 101-1) during the core cooling, low or no-flow bypass, and rewarming phases of deep hypothermic cardiac surgery. In individual infants sustaining intraoperative brain injury, the precise role and contribution of each of these phenomena are often unclear. The potential mechanisms for the development of HI/R are multiple and include both global (Box 101-1) and focal insults (Box 101-2). In adults undergoing cardiac surgery, the predominant form of brain injury is focal embolic ischemia, resulting when atheromatous debris dislodged from the aorta enters the arteriosclerotic cerebrovascular bed. Conversely, in the young infant undergoing open-heart surgery, the cerebral HI/R insults are mediated primarily by global hypoperfusion. Normally, the delicate balance between cerebral oxygen supply and utilization is controlled by intrinsic cerebral autoregulatory systems. These systems are likely overwhelmed by the major hemodynamic and metabolic changes induced during infant open-heart surgery. During the intraoperative period, it becomes the responsibility of the anesthesiologist-perfusionist team to maintain a positive balance of cerebral energy supply and demand. Guidelines for this extrinsic regulation of cerebral oxygenation remain based largely on theoretical safety parameters of aspects, such as duration of circulatory arrest, as well as rate and depth of cooling. The complex and dynamic intraoperative changes in both oxygen substrate demand and supply, as well as the lack of reliable and validated intraoperative monitoring techniques, make it challenging in the individual patient to identify imminent cerebral hypoxia-ischemia before injury occurs [du Plessis et al., 1995b; Sakamoto et al., 2001, 2004; Wardle et al., 1998].
Box 101-1 Intraoperative Determinants of Global Cerebral Oxygen Delivery and Use
Over the last two decades, there has been intense research into techniques that reduce the risk of intraoperative hypoxia-ischemia. Clinical trials have focused on strategies for optimizing intraoperative cerebral oxygen availability, which is most tenuous during periods of low-flow cardiopulmonary bypass (LFB) or deep hypothermic circulatory arrest (DHCA). Since longer periods of both DHCA and LFB have their own attendant risks, the neurologic outcome associated with LFB and DHCA was compared in a randomized clinical trial of 171 infants with TGA undergoing neonatal cardiac repair. Based on the findings of this trial, in which DHCA was associated with higher perioperative morbidity [Newburger et al., 1993] and worse developmental outcome at age 1 year, most centers adopted a strategy that minimized DHCA. The longer-term outcomes of this study are discussed below [Bellinger et al., 1997, 1999b, 2003].
Deep hypothermia has significant acid–base effects, and different intraoperative management strategies have been used to manipulate these acid–base changes with the goal of optimizing tissue metabolism. Both the more alkalotic alpha-stat strategy and the more acidotic pH-stat strategy have theoretical advantages. The alpha-stat approach is based on the natural alkaline shift in tissue that accompanies hypothermia and is thought to optimize enzyme function at low temperatures. Conversely, the pH-stat technique utilizes the addition of CO2 to counter this alkaline shift and is used to enhance tissue oxygen delivery. The more hypercarbic perfusate increases cerebral perfusion and increases oxygen release in tissues by countering the increased oxygen-hemoglobin affinity associated with hypothermia. A randomized clinical trial comparing these two pH management strategies suggested that infants managed by the pH-stat strategy had less perioperative morbidity and a shorter time to recovery of electrocortical activity [du Plessis et al., 1997]. As a result, the pH-stat strategy has become incorporated into the intraoperative acid–base management protocols of many centers, particularly during the induction of hypothermia on CBP.
Hemodilution is used widely in CBP to improve blood flow in the microcirculation. However, the benefits of improved perfusion are countered by the risks of reduced oxygen-carrying capacity of the circulation. A randomized clinical trial of different hematocrit levels in infants undergoing hypothermic CBP found that hemodilution was associated with greater perioperative hemodynamic instability and worse psychomotor development at age 1 year [Jonas et al., 2003]. However, at longer-term follow-up, the neurodevelopmental outcomes were similar in both groups [Newburger et al., 2008].
Besides global HI/R insults, several other mechanisms have been implicated in intraoperative brain injury. Compared with those in adults, vaso-occlusive insults play a lesser role in intraoperative brain injury during cardiac surgery in infants. CPB and the surgical field may generate particulate (e.g., platelet, synthetic, or fat) (Figure 101-4) or gaseous (e.g., air) emboli [Boyajian et al., 1993; Fish, 1988; Moody et al., 1990; Padayachee et al., 1987]. Inflammatory mechanisms triggered during CPB also have been investigated for their role in intraoperative brain injury, an interest stimulated by the growing body of evidence for cytokine-mediated neurotoxicity in animal studies [Back, 2006; Back et al., 2007; Den et al., 2009]. A post-bypass systemic inflammatory syndrome [Kirklin et al., 1983; Westaby, 1987], with systemic vascular injury manifesting with marked edema, is common, particularly in young infants. This syndrome is related to the relatively large blood volume required for priming bypass pumps and the prolonged exposure of this blood to artificial surfaces. The elevated levels of cytokines (interleukins-6 and 8, tumor necrosis factor-α, and endothelin-1) measured in bypass blood [Bando et al., 1998; Elliott, 1999; Elliott and Finn, 1993; Jansen et al., 1992; Journois et al., 1996; Kirklin et al., 1983; Millar et al., 1993; Steinberg et al., 1993] potentially may mediate vascular, as well as cellular, injury. Ultrafiltration of blood during or after CPB reduces both cytokine levels and excess free plasma water [Gaynor, 2003]. Ultrafiltration has been associated with improved postoperative hemodynamics [Bando et al., 1998] and improved myocardial function [Chaturvedi et al., 1999; Davies et al., 1998]. It is not known whether the beneficial effects of ultrafiltration result primarily from a reduction in postoperative cytokine levels or from hemoconcentration. To date, the potential contribution of this systemic inflammatory response to intraoperative brain injury has not been demonstrated convincingly in clinical trials with long-term follow-up.
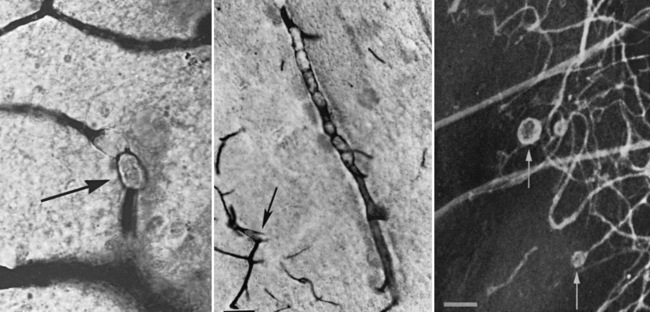
Fig. 101-4 Aneurysmal dilatations (arrows) in the cerebral microvasculature after cardiopulmonary bypass.
(From Moody D, Bell M, Challa VR, et al: Brain microemboli during cardiac surgery or aortography, Ann Neurol 28:477–486, 1990. Copyright © 1990 Wiley–Liss, Inc., a Wiley Company. Reproduced with permission of John Wiley & Sons, Inc.)
Mechanisms of Neurologic Injury with Extracorporeal Membrane Oxygenation
Extracorporeal oxygenation-circulation devices are used increasingly in children with critical heart disease as a “bridge” to recovery, surgery, or transplantation. Extracorporeal life-support devices were introduced into clinical practice by Kirklin and Lillehei in the mid-1950s for the CPB support of patients undergoing open-heart surgery [Lillehei, 1982; Moller et al., 2009]. On-going technological advances expanded the applications of these devices, and in the 1980s they emerged from the operating room to become established for the intensive care management of severe but potentially reversible cardiorespiratory failure. In the early years, the principal target population in children was the term newborn with respiratory failure due to meconium aspiration and persistent pulmonary hypertension, and subsequently congenital diaphragmatic hernia [Bartlett et al., 1977]. Since its inception, an estimated 20,000 infants have had extracorporeal membrane oxygenation (ECMO) for respiratory failure [Lequier et al., 2008]. The advent of nitric oxide pulmonary vasodilators obviated the need for ECMO in many cases of respiratory failure due to pulmonary hypertension [Christou et al., 2000; Clark et al., 2000]. Coinciding with its waning use for respiratory failure, ECMO became used increasingly for critical but potentially reversible circulatory failure. There are two broad indications for so-called cardiac ECMO in children. Most commonly, cardiac ECMO is used to support children with severe cardiovascular failure, for which spontaneous recovery or effective intervention is possible. Included in this group are newborn infants who fail to transition effectively from a fetal to an extrauterine circulation; children with intractable cardiac failure awaiting transplant; and children who fail to wean off CPB after cardiac surgery. Another indication for cardiac ECMO, employed in some centers, is cardiorespiratory arrest refractory to conventional resuscitative measures (cardiopulmonary resuscitation [CPR]-ECMO) [del Nido et al., 1992]. The use of CPR-ECMO has been recommended by the American Heart Association for in-hospital cardiac arrest that is potentially reversible or amenable to transplant.
ECMO may injure the brain through multiple mechanisms that promote either ischemic or hemorrhagic injury. Carotid ligation causes an immediate reduction in blood flow to the ipsilateral middle cerebral artery, which may be restored only partially by collateral flow through the circle of Willis and the external carotid artery. This reduced flow may cause direct ischemia and may interfere with cerebral autoregulation, rendering that hemisphere vulnerable to systemic hypotension. Jugular vein ligation can result in cerebral venous hypertension, which may predispose to venous infarction, and which further decreases the cerebral arteriovenous pressure gradient. Systemic heparinization increases the risk of intracranial hemorrhage, which may be either primary or due to hemorrhagic transformation of an ischemic lesion. Finally, ECMO causes elevated levels of inflammatory cytokines, which may contribute to the development of PVL [Fortenberry et al., 1996; Kinney and Back, 1998].
The Neurologic Outcome of Children Undergoing Extracorporeal Membrane Oxygenation
An important determinant of long-term neurologic outcome following ECMO is the underlying indication for its use. Given the significant clinical instability and illness severity preceding ECMO, and the difficulty obtaining definitive brain imaging acutely, it remains difficult to distinguish between brain injury associated with the ECMO technique itself and that due to the underlying critical illness. For a number of reasons, the outcome after cardiac ECMO is less favorable than for respiratory ECMO. This finding is perhaps not surprising since cerebral ischemia is known to be a more potent cause of brain injury than is cerebral hypoxemia, a notion supported by the fact that the severity of arterial metabolic acidosis prior to ECMO is a significant predictor of adverse neurologic outcome [Cengiz et al., 2005].
Intracranial hemorrhage and infarction are the primary neurologic complications of ECMO, with previous estimates ranging from 25 to 50 percent overall, and severe lesions in 10–20 percent of infants [Volpe, 2001]. The most common sites of intracranial hemorrhage are into the lateral ventricles and posterior fossa structures. Cerebral ischemia may be global or focal, and may manifest as PVL [Jarjour and Ahdab-Barmada, 1994; Kinney and Back, 1998]. Clinical seizures occur in approximately 10 percent of both cardiac and respiratory ECMO cases [Dalton, 2004]. However, given the overall morbidity and the use of sedating-paralyzing drugs, it is not surprising that electrographic seizures have been detected in up to 70 percent of cases. [Kim and Stolar, 2000]. Despite this high risk for acute seizures, the risk for subsequent epilepsy is low. Sensorineural hearing impairment, often reversible, is reported in 4–15 percent of neonates after ECMO [Desai et al., 1997; Hofkosh et al., 1991; Schumacher et al., 1991]. ECMO survivors are at significant risk for neurocognitive delays; between 10 and 29 percent have developmental quotients below 70, the greatest risk being in the smaller infants [Hamrick et al., 2003; Revenis et al., 1992].
The short-term benefit of respiratory (VV) ECMO was established early, with multicenter survival rates around 75–80 percent; of note, a major criterion for ECMO eligibility was a predicted mortality of greater than 80 percent [Dalton et al., 2005; Stolar et al., 1991]. However, reports of neurologic injury in infants undergoing ECMO soon emerged, and this occurrence remains a major complication among survivors of ECMO. The reported long-term neurologic morbidity has ranged from 15 to 30 percent [Hanekamp et al., 2006; McNally et al., 2006; Robertson et al., 1995].
There is a relative paucity of data for the long-term outcome of cardiac (VA) ECMO. Overall survival is lower than for respiratory ECMO, and ranges from 30 to 55 percent [Balasubramanian et al., 2007; Chen et al., 2008; Taylor et al., 2007], but varies with the specific underlying indication. Children with dilated cardiomyopathy or myocarditis requiring ECMO may have survival rates as high as 80–90 percent [Duncan et al., 2001; McMahon et al., 2003]. Neurologic deficits have been described in up to 60 percent of cardiac ECMO survivors [Chow et al., 2004; Hamrick et al., 2003; Ibrahim et al., 2000], with mental retardation in 30–50 percent of survivors, depending on the indication [Hamrick et al., 2003; Lequier et al., 2008].
Not surprisingly, survival is significantly higher when ECMO is started before cardiac arrest than after, CPR-ECMO having the highest mortality and morbidity risks. While conventional CPR lasting 30 minutes or more has a survival of 5 percent or less [de Mos et al., 2006; Morris et al., 2004], survival after CPR-ECMO in patients who had failed conventional CPR is around 30–40 percent [Alsoufi et al., 2007; Chan et al., 2008; Lequier et al., 2008; Thiagarajan et al., 2007]. Risk factors for death in CPR-ECMO cases are severe metabolic acidosis (pH ≤ 7.00) prior to ECMO, persistent metabolic acidosis despite CPR-ECMO, and the development of multisystem organ failure [Kolovos et al., 2003; Kulik et al., 1996; Thiagarajan et al., 2007]. In a recent study of outcome following cardiac ECMO, including CPR-ECMO, survival to discharge was 46 percent, but more than 50 percent of survivors had mental retardation at long-term follow-up, with time to normalization of circulating lactate and the degree of inotropic support needed being significant predictors [Lequier et al., 2008].
Early Postoperative Manifestations of Neurologic Dysfunction
Twenty years ago, as many as 25 percent of children undergoing open heart operations demonstrated evidence of early postoperative neurologic dysfunction [Ferry, 1987, 1990; Newburger et al., 1993]. On-going advances in management, including those based on the randomized clinical trials discussed above, appear to be associated with a decrease in the incidence of postoperative neurologic dysfunction [Menache et al., 2002]. These early postoperative neurologic complications are often transient, and initially were considered of little long-term consequence. However, subsequent follow-up studies suggested that these early complications may be associated with long-term adverse outcome.[Bellinger et al., 1995].
Postoperative neurologic manifestations may result from pre-, intra-, and postoperative injury to the nervous system at any or multiple levels of the neuroaxis (Figure 101-5). However, the bedside clinical diagnosis of neurologic dysfunction in these critically ill infants may be complicated by the subtle manifestations of acute cerebrovascular injury, and further obscured by the widespread use of sedating or paralyzing medications. These confounding factors have delayed the accurate diagnosis of perioperative brain dysfunction and limited the formulation of rational management strategies.
Delayed Recovery of Consciousness
Prolonged delay in the recovery of consciousness after cardiac surgery, anesthesia, and sedation is not uncommon. The standard diagnostic guidelines [Plum and Posner, 1985] for the evaluation of altered mental status should be applied to these patients, although the precise mechanism(s) remains unclear in most cases. Ultimately, some of these children demonstrate clinical and neuroimaging features suggestive of cerebral HI/R injury. However, because intraoperative events specific for HI/R often are lacking, other potentially reversible etiologies should be excluded, including postoperative hepatic or renal impairment with accumulation of toxic metabolites, or impaired metabolism or excretion of sedating drugs. Prolonged use of neuromuscular blocking agents for ventilatory management may be associated with delayed recovery of motor function [Gooch et al., 1991; Partridge et al., 1990; Waitling and Dasta, 1994], and, in severe cases, may mimic impaired consciousness. Neuromuscular blockade may be excluded with a bedside peripheral nerve stimulator or nerve conduction studies. A significant minority of infants develop postoperative seizures, which may recur serially and often are clinically silent [Newburger et al., 1993]. In the absence of other causes to explain persistent impairment of consciousness, occult seizures or a prolonged postictal state should be considered.
Postoperative Seizures
Seizures are among the most common manifestations of neurologic dysfunction after infant open-heart surgery. Past series have reported clinical seizures in up to 15 percent of infants in the early postoperative period [Ehyai et al., 1984; Miller et al., 1995; Newburger et al., 1993], whereas clinically silent, electrographic seizures were detected by continuous electroencephalographic (EEG) monitoring in up to 20 percent of infants [Helmers et al., 1997]. More recent studies from large pediatric cardiac surgery centers report widely disparate postoperative seizure rates ranging from 1.2 to 17.7 percent [Clancy et al., 2003; Menache et al., 2002]. Risk factors for postoperative seizures include the use of DHCA, prolonged DHCA times, the presence of a ventricular septal defect, and pre-existing genetic conditions [Clancy et al., 2003; Helmers et al., 1997]. The recent decline in seizure incidence observed in some centers also may be attributable to refinements in extracorporeal perfusion support, such as the minimization of DHCA.
Two forms of early postoperative seizures may be distinguished, i.e., seizures with a readily identifiable cause and those without (so-called “postpump seizures”). The usual etiologies of seizures should be excluded, particularly reversible causes such as hypoglycemia and electrolyte disturbances. Cyclosporine A toxicity is a common cause of seizures in heart transplant recipients [Menache et al., 2002].
Postpump seizures
Postpump seizures (i.e., those without an identifiable etiology) were the most common form of postoperative seizures in previous years, but their incidence appears to be decreasing. Postpump seizures tend to have certain characteristic clinical features and are confined almost exclusively to young infants. Conversely, postoperative seizures in older infants and children usually are associated with an identifiable cause. Postpump seizures often are assumed to reflect intraoperative HI/R injury; however, they differ from other post-HI/R (e.g., birth asphyxia) seizures in several ways. First, the onset of postpump seizures (including EEG seizures) is typically delayed until 24–48 hours postoperatively. This is significantly later than postasphyxial seizures, which often present within the first 12 hours after birth. Second, although not always benign, the long-term outcome of postpump seizures [Bellinger et al., 1995] is markedly better than the 50 percent incidence of adverse neurologic outcome seen after postasphyxial seizures [Andre et al., 1990; Bergman et al., 1983; Volpe, 2001].
The temporal predilection for postpump seizures is relatively circumscribed to a “window” of several days (Figure 101-6). During this period, seizures tend to recur and, not uncommonly, status epilepticus develops. After several days, the tendency for further seizures decreases rapidly. The clinical manifestations of postpump seizures may be subtle, especially in infants receiving sedating or paralyzing drugs. Behavioral changes may be confined to paroxysmal changes in autonomic function, such as tachycardia, hypertension, and pupillary dilatation. Convulsive activity, when evident, is often focal or multifocal. Bedside EEG helps distinguish true epileptic phenomena from other behavioral or autonomic changes [Mizrahi, 1987; Scher and Painter, 1990]. Furthermore, the ability of EEG to detect clinically occult seizure activity helps guide anticonvulsant drug therapy. Finally, highly focal EEG abnormalities may reflect an underlying structural lesion (e.g., stroke), indicating the need for neuroimaging.
The therapeutic approach to postpump seizures is dictated best by their typical clinical course. After reversible etiologies, such as hypoglycemia, hypomagnesemia, and hypocalcemia, [Lynch and Rust, 1994; Satur et al., 1993] have been excluded, anticonvulsant therapy should commence. In view of the tendency toward repeated seizures and status epilepticus, the initial goal is rapid achievement of therapeutic anticonvulsant levels by an intravenous route. The vast majority of postoperative seizures are controlled by standard anticonvulsant regimens used in infants. Careful cardiorespiratory monitoring is essential when using potentially cardiotoxic anticonvulsants during the postoperative period, when myocardial dysfunction or arrhythmias are prevalent. Specifically, in infants with established myocardial dysfunction, cautious administration of phenobarbital is advisable, whereas pre-existent conduction disturbances, particularly bradyarrhythmias, necessitate careful monitoring of phenytoin therapy [Cranford et al., 1978]. The apparently circumscribed window of susceptibility for these postpump seizures and the rarity of subsequent epilepsy allow successful early withdrawal of anticonvulsant agents, often before hospital discharge.
Prognosis of postoperative seizures
The prognosis of postoperative seizures depends on the underlying etiology. Postpump seizures without an obvious etiology previously were considered benign, transient events. Although a significant association between postoperative seizures and worse neurodevelopmental outcome and MRI abnormalities has been demonstrated in a large prospective study, [Bellinger et al., 1995; Rappaport et al., 1998], this has not been the case in other studies [Gaynor et al., 2006]. Epilepsy is a rare sequel to typical postpump seizures [Ehyai et al., 1984], although West’s syndrome has been described in occasional survivors of intractable postoperative seizures [du Plessis et al., 1994a]. The long-term outcome of infants with an identified cause for their postoperative seizures is related to the specific etiology. Seizures in infants with brain dysgenesis are associated with an almost universal poor long-term outcome, and epilepsy is a common complication. Stroke in young infants commonly presents with seizures. The overall risk for subsequent epilepsy in these infants ranges from 20 to 30 percent [Lanska et al., 1991; Yang et al., 1995], and relates to age at stroke and the post-stroke latency to first seizure. Specifically, the risk for later epilepsy is lowest when stroke occurs in the newborn infant [Levy et al., 1985] and when seizures are an early manifestation of stroke [Yang et al., 1995]. These features should be considered when planning maintenance antiepileptic drug therapy.
Stroke in the Early Postoperative Period
Vaso-occlusive stroke in children with CHD diagnosed in the early postoperative period likely has an etiologic profile that differs from stroke occurring prior to or remote from cardiac surgery. The latter category of stroke is discussed later in this chapter. Since strokes diagnosed in the early postoperative period are likely to be clinically silent during the operation and early postoperative recovery [Chen et al., 2009], their incidence is likely underestimated. A recent 10-year review described a 5.4 per 1000 incidence of arterial and venous occlusive brain injury within the first 3 days of cardiac surgery [Domi et al., 2008]. Another study using MRI in the first 14 days after surgery found a 10 percent incidence of stroke that included both vaso-occlusive and global hypoperfusion (“watershed”) injuries; vaso-occlusive lesions comprised less than half these “strokes” [Chen et al., 2009] (Figure 101-7). Of interest, the vast majority of these lesions were clinically silent in the acute phase.
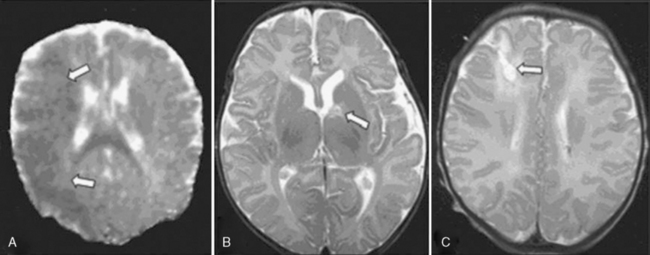
Fig. 101-7 Illustrative magnetic resonance imaging findings of infarcts (arrows).
(From Chen J, Zimmerman RA, Jarvik GP, et al: Perioperative stroke in infants undergoing open heart operations for congenital heart disease, Ann Thorac Surg 88:823, 2009, Figure 1. Reprinted with permission.)
Certain features of open-heart surgery and the immediate postoperative period may predispose to stroke and warrant brief review. During the intraoperative period, both embolic and thrombotic mechanisms may cause cerebral vaso-occlusive injury. Particulate and gaseous emboli originating from the CPB apparatus or surgical field bypass the normal pulmonary filtration system during CPB and thus enter the systemic circulation directly [Boyajian et al., 1993; Moody et al., 1990; Padayachee et al., 1987; Solis et al., 1975]. Improvements in bypass circuits, particularly the replacement of bubble oxygenators by membrane oxygenators, have decreased the incidence of macroembolic injury [Nussmeier and McDermott, 1988]. The impact of these advances on the incidence of microvascular injury [Fish, 1988] is unclear. The marked inflammatory response triggered by the extensive and prolonged exposure between bypass blood and artificial surfaces [Kirklin et al., 1983; Millar et al., 1993; Steinberg et al., 1993] is known to trigger complex cascades, including endothelial-leukocyte interactions [del Zoppo, 1994; Elliott and Finn, 1993; Feuerstein et al., 1994; Lucchesi, 1993]. The resulting microvascular dysfunction may predispose to intravascular thrombosis or thromboembolism, manifesting with vaso-occlusive brain injury.
In the early postoperative period, several factors predispose to cardiogenic stroke. First, vascular stasis may result from localized areas of low flow within the heart [du Plessis et al., 1995a; Rosenthal et al., 1995a, 1995b], or global ventricular dysfunction. Prolonged postoperative immobility predisposes to systemic venous stasis and thrombosis. Transient pulmonary hypertension is common after CPB, elevating venous pressure and decreasing the rate of blood flow through the right heart chambers and central veins. Injury to native tissue or the presence of prosthetic tissue alters vascular surfaces in contact with the circulation. A number of these stroke risk factors may be present after the Fontan procedure, the most common final operation for CHD with a single ventricle physiology [Day et al., 1995; Dobell et al., 1986; du Plessis et al., 1995a; Hutto et al., 1991; Mathews et al., 1986; Rosenthal et al., 1995a, 1995b], and is discussed in more detail below.
Movement Disorders After Cardiac Surgery
For many years, movement disorders have been considered an uncommon but dreaded complication of deep hypothermic cardiac surgery [Barrat-Boyes, 1990; Bergouignan et al., 1961; Bjork and Hultquist, 1962; Brunberg et al., 1974; Chaves and Scaltsas-Persson, 1988; Curless et al., 1994; DeLeon et al., 1990; Donaldson et al., 1990; Huntley et al., 1993; Medlock et al., 1993; Robinson et al., 1988; Wical and Tomasi, 1990; Wong et al., 1992]. Although less commonly described in recent years, these postoperative movement disorders are often dramatic, frequently intractable, and ultimately debilitating, with severe forms associated with substantial mortality.
Choreoathetosis is the most frequently reported dyskinesia after cardiac surgery, but a spectrum of movement disorders, including oculogyric crises [du Plessis et al., 1994b