Nervous System
1. Induction, including primary induction of the nervous system by the underlying notochord and secondary inductions driven by neural tissues themselves
2. Proliferation, first as a response of the neuroectodermal cells to primary induction and later to build up critical numbers of cells for virtually all aspects of morphogenesis of the nervous system
3. Pattern formation, in which cells respond to genetic or environmental cues in forming the fundamental subdivisions of the nervous system
4. Determination of the identity of specific types of neuronal or glial cells
5. Intercellular communication and the adhesion of like cells
6. Cell migration, of which a variety of distinct patterns is found in the nervous system
7. Cellular differentiation of neurons and glial cells
8. Formation of specific connections or synapses between cells
9. Stabilization or elimination of specific interneuronal connections, sometimes associated with massive episodes of cell death of unconnected neurons
10. Progressive development of integrated patterns of neuronal function, which results in coordinated reflex movements
Establishment of the Nervous System
As described in Chapter 5, primary induction of the nervous system results in the formation of a thickened ectodermal neural plate overlying the notochord. Much of the dorsal ectoderm in gastrulating embryos produces the signaling protein bone morphogenetic protein-4 (BMP-4), which inhibits the dorsal ectoderm from forming neural tissue. Instead of sending positive signals to the overlying ectoderm, the neural inducers, noggin and chordin, block the inhibitory influence of BMP-4 and allow the dorsal ectoderm to form neural tissue (the neural plate [see Fig. 5.8]).
Shortly after neural induction, further signals from the notochord and head organizing regions (prechordal plate and anterior visceral endoderm) result in the expression of the transcription factor Otx-2 in the forebrain-midbrain region and Gbx2 in the hindbrain region. The expression boundary between these two transcription factors forms the isthmic organizer. The signaling molecules fibroblast growth factor-8 (FGF-8) and Wnt-1 diffuse from this boundary and are instrumental in setting up the pattern for forming the midbrain and hindbrain. Then, under the influence of specific combinations of Hox genes and other transcription factors, the hindbrain undergoes a highly regular segmentation into rhombomeres, which presage the overall organization of the entire facial and cervical region (see Fig. 11.12).
The neural tube, which is the morphological manifestation of the earliest stages in establishing the nervous system, is a prominent structure. In a human, it dominates the cephalic end of the embryo (see Fig. 6.1). This chapter describes how the early neural tube develops into the major morphological and functional components of the mature nervous system.
Early Shaping of the Nervous System
Closure of the neural tube first occurs in the region where the earliest somites appear; closure spreads cranially and caudally (see Fig. 6.1). The unfused regions of the neural tube are known as the cranial and caudal neuropores. Even before the closure of the neuropores (24 days’ gestation for the cranial neuropore and 26 days’ gestation for the caudal neuropore), some fundamental subdivisions in the early nervous system have become manifest. The future spinal cord and brain are recognizable, and within the brain the forebrain (prosencephalon), midbrain (mesencephalon), and hindbrain (rhombencephalon) can be distinguished (Fig. 11.1A).
A prominent force in shaping the early nervous system is the overall bending of the cephalic end of the embryo into a “C” shape. Associated with this bending is the appearance at the end of the third week of a prominent cephalic flexure of the brain at the level of the mesencephalon (see Fig. 11.1A). Soon the brain almost doubles back on itself at the cephalic flexure. At the beginning of the fifth week, a cervical flexure appears at the boundary between the hindbrain and the spinal cord.
By the fifth week, the original three-part brain has become subdivided further into five parts (Fig. 11.2; see Fig. 11.1B). The prosencephalon gives rise to the telencephalon (endbrain), with prominent lateral outpocketings that ultimately form the cerebral hemispheres, and a more caudal diencephalon. The diencephalon is readily recognizable because of the prominent lateral optic vesicles that extend from its lateral walls. The mesencephalon, which is sharply bent by the cephalic flexure, remains undivided and tubular in its overall structure. The roof of the rhombencephalon becomes very thin, and there are early indications of the subdivision of the rhombencephalon into a metencephalon and a more caudal myelencephalon. These five subdivisions of the early brain represent a fundamental organization that persists through adulthood. Many further structural and functional components give added layers of complexity to the brain over the next several weeks of embryonic life.
Histogenesis Within the Central Nervous System
Proliferation Within the Neural Tube
Shortly after induction, the thickening neural plate and early neural tube become organized into a pseudostratified epithelium (Fig. 11.3). In this type of epithelium, the nuclei appear to be located in several separate layers within the elongated neuroepithelial cells. The nuclei undertake extensive shifts of position within the cytoplasm of the neuroepithelial cells.
The neuroepithelial cells are characterized by a high degree of mitotic activity, and the position of the nuclei within the neural tube and their stage in the mitotic cycle are closely correlated (Fig. 11.4). DNA synthesis occurs in nuclei located near the external limiting membrane (the basal lamina surrounding the neural tube). As these nuclei prepare to go into mitosis, they migrate within the cytoplasm toward the lumen of the neural tube, where they undergo mitotic division. The orientation of the mitotic spindle during this division predicts the fate of the daughter cells. If the metaphase plate (plane of cleavage) is perpendicular to the apical (inner) surface of the neural tube, the two daughter cells slowly migrate in tandem back toward the outer side of the neural tube, where they prepare for another round of DNA synthesis (see Fig. 11.4).
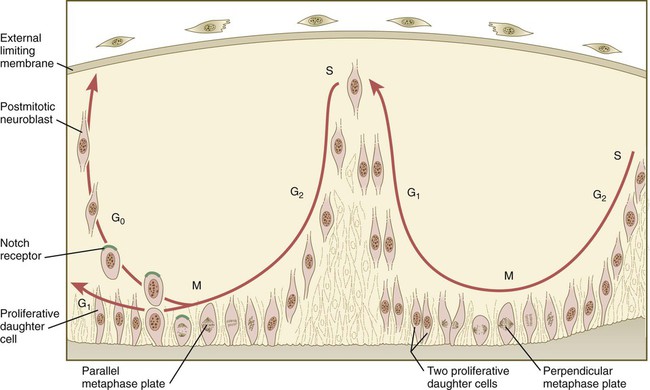
In the pseudostratified epithelial cells that constitute the early neural tube, nuclei that synthesize DNA (S phase) are located near the external limiting membrane. These nuclei move toward the inner margin of the neural tube, where mitosis (M) occurs. If the metaphase plate is perpendicular to the inner margin, the two daughter cells remain in a proliferative state and migrate back toward the external limiting membrane for another round of DNA synthesis. If in the next mitosis the metaphase plate is oriented parallel to the inner margin, one daughter cell remains in the proliferative state. The other daughter cell, which expresses Notch, leaves the mitotic cycle to become a neuroblast.
In contrast, if the plane of cleavage is parallel to the inner surface of the neural tube, the daughter cells undergo dramatically different fates. The daughter cell that is closer to the inner surface migrates away very slowly and remains a proliferative progenitor cell that is capable of mitosis. The daughter cell that is closer to the basal surface (external limiting membrane) inherits a high concentration of the Notch receptor on its surface and quickly moves away from the apical surface as a postmitotic neuroblast (see Fig. 11.4). The neuroblasts, cellular precursors of neurons, begin to produce cell processes that ultimately become axons and dendrites.
Cell Lineages in Histogenesis of the Central Nervous System
The origins of most cells found in the mature central nervous system can be traced to multipotential stem cells within the early neuroepithelium (Fig. 11.5). These cells undergo numerous mitotic divisions before maturing into bipotential progenitor cells, which give rise to either neuronal or glial progenitor cells. Activation of the proneural genes neurogenin 1 and neurogenin 2 promotes the differentiation of neurons from the bipolar progenitor cells. Glial cells differentiate under the influence of other stimuli. This developmental bifurcation is accompanied by a change in gene expression. Multipotential stem cells express an intermediate filament protein called nestin. Nestin is downregulated as descendants of bipolar progenitor cells separate into neuronal progenitor cells, which express neurofilament protein, and glial progenitor cells, which express glial fibrillary acidic protein.
The other major lineage stemming from the bipotential progenitor cells is the glial line. Glial progenitor cells continue to undergo mitosis, and their progeny split into several lines. One, the O-2A progenitor cell (see Fig. 11.5), is a precursor to two lines of glial cells that ultimately form the oligodendrocytes and type 2 astrocytes. Another glial lineage gives rise to type 1 astrocytes. Human oligodendrocytes arise from progenitor cells located in the ventral ventricular zone (see MN in Fig. 11.10) alongside the floor plate. From there, they spread throughout the brain and spinal cord and ultimately form the myelin coverings around neuronal processes in the white matter. The formation of oligodendrocyte precursors depends on an inductive signal from the notochord (sonic hedgehog [shh]). If the notochord is transplanted alongside dorsal neural tube, oligodendrocyte precursors differentiate there, thus showing that cells with the potential to form oligodendrocytes reside in that area, but normally do not develop because of the lack of an adequate inductive signal.
The third glial lineage has a more complex history. Radial progenitor cells give rise to radial glial cells, which act as guidewires in the brain for the migration of young neurons (see Fig. 11.23). When the neurons are migrating along the radial glial cells during midpregnancy, they inhibit the proliferation of the radial glial cells. After neuronal cell migration, the radial glial cells, now free from the inhibitory influence of the neurons, reenter the mitotic cycle. Their progeny can transform into several cell types. Some can seemingly cross lineage lines and differentiate into type 1 astrocytes (see Fig. 11.5). Other progeny differentiate into various specialized glial cell types, ependymal cells, and even adult neural stem cells. According to some authors, the remaining neuroepithelial cells represent another source of ependymal cells.
Fundamental Cross-Sectional Organization of the Developing Neural Tube
The developing spinal cord is a useful prototype for studying the overall structural and functional features of the central nervous system because it preserves its fundamental organization through much of development. With the beginning of cellular differentiation in the neural tube, the neuroepithelium thickens and appears layered. The layer of cells closest to the lumen (central canal) of the neural tube remains epithelial and is called the ventricular zone (the ependymal zone in older literature). This zone, which still contains mitotic cells, ultimately becomes the ependyma, a columnar epithelium that lines the ventricular system and central canal of the central nervous system (Fig. 11.6). Farther from the ventricular zone is the intermediate (formerly called mantle) zone, which contains the cell bodies of the differentiating postmitotic neuroblasts. As the neuroblasts continue to produce axonal and dendritic processes, the processes form a peripheral marginal zone that contains neuronal processes, but not neuronal cell bodies.
As the spinal cord matures, the intermediate zone becomes the gray matter, in which the cell bodies of the neurons are located. The marginal zone is called the white matter because of the color imparted by the numerous tracts of myelinated nerve fibers in that layer (see Fig. 11.6). During development, the proliferating progenitor cell populations in the ventricular zone become largely exhausted, but it is now known that a subpopulation persists into adulthood as neural stem cells. The remaining cells differentiate into the epithelium of the ependymal layer.
The basal plate represents the motor component of the spinal cord. Axons arising from neurons located in the ventral horn of the gray matter exit the spinal cord as ventral motor roots of the spinal nerves (see Fig. 11.15). The gray matter of the alar plate, called the dorsal horn, is associated with sensory functions. Sensory axons from the spinal ganglia (neural crest derivatives) enter the spinal cord as dorsal roots and synapse with neurons in the dorsal horn. A small projection of gray matter between the dorsal and ventral horns at spinal levels T1 to L2 contains cell bodies of autonomic neurons. This projection is called the lateral horn or sometimes the intermediolateral gray column (see Fig. 11.6).
The floor plate is far more than an anatomical connection between the right and left basal plates. Cells of the future floor plate are the first to differentiate in the neural plate after primary induction of the nervous system. Experimental work has shown a specific inductive influence of the notochord on the neuroepithelial cells that overly it. If an extra notochord is grafted along the lateral surface of the neural tube, the neuroepithelial cells closest to it acquire the properties of floor plate cells (Fig. 11.7). Conversely, if a segment of normal notochord is removed, the neuroepithelial cells overlying it do not acquire the properties of floor plate cells. Through its action on the floor plate, the notochord also exerts a profound effect on the organization of the dorsal and ventral roots that enter and leave the spinal cord. If the notochord is absent, the neural tube closes, but recognizable dorsal and ventral roots are absent. Numerous ectopic nerve fibers appear in their place (Fig. 11.8). If the future floor plate is split, the side of the neural tube on which the notochord is located develops normal dorsal and ventral roots, whereas the side lacking these structures gives off ectopic nerves (see Fig. 11.7C).
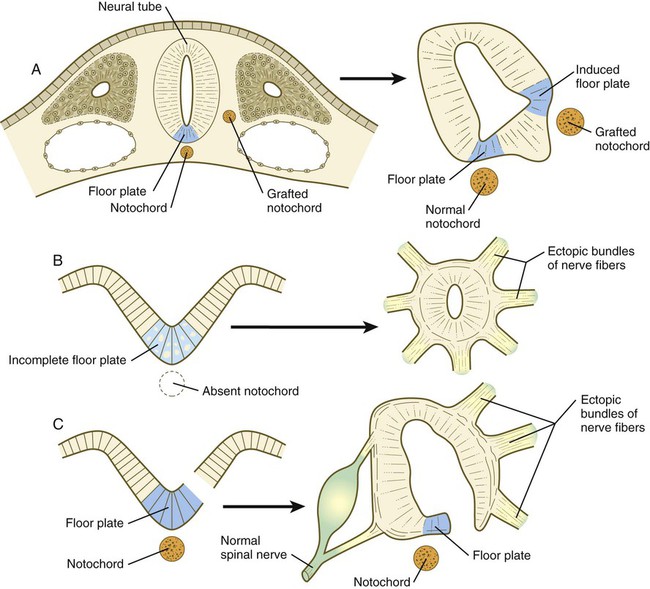
A, Grafting an extra notochord near the neural tube induces a secondary floor plate. B, In the absence of a notochord, a very incomplete floor plate forms, and nerve fibers exit from multiple sites around the spinal cord. C, Slitting the neural plate on one side of the floor plate removes the wall of the neural tube from the influence of the notochord, allowing the disorganized exit of nerve fibers from that part of the spinal cord. (Adapted from Hirano S, Fuse S, Sohal GS: Science 251:310-313, 1991.)
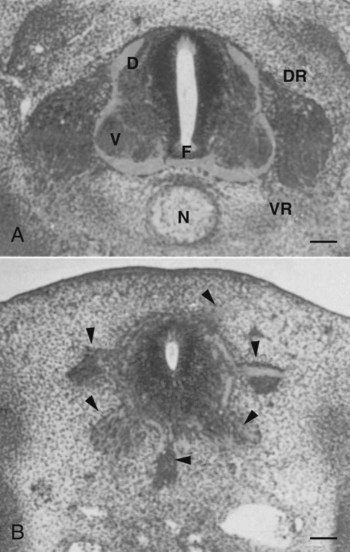
A molecular basis for cross-sectional pattern formation within the early neural plate and neural tube has been identified (Fig. 11.9). The homeobox-containing transcription factors, Pax-3, Pax-7, Msx-1, and Msx-2, are expressed throughout the early neural plate. Before the neural plate has folded over to become the neural tube, the notochord, which is adherent to the midline neural plate at this stage, releases shh. Local hedgehog signaling stimulates the neural plate cells directly above the notochord to transform into the floor plate. One of the first stages of this transformation is the repression of Pax-3 and Pax-7 expression, which allows the neuroectodermal cells near the midline of the neural plate to adopt a ventral fate (i.e., floor plate or basal plate). Cells of the floor plate itself then become sites of production of shh.
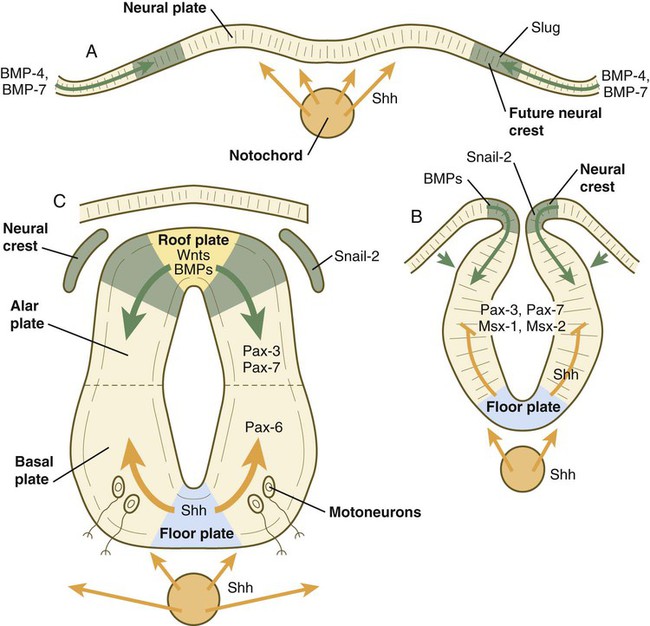
A, Signals from sonic hedgehog (Shh) (orange arrows) in the notochord induce the floor plate. B, In the dorsal part of the future neural tube, bone morphogenetic protein-4 (BMP-4) and BMP-7 (green arrows) from the ectoderm adjacent to the neural tube induce snail-2 in the future neural crest and maintain Pax-3 and Pax-7 expression dorsally. Ventrally, Shh, now produced by the floor plate, induces motoneurons. C, Shh, produced by the floor plate, suppresses the expression of dorsal Pax genes (Pax-3 and Pax-7) in the ventral half of the neural tube. Wnts and BMPs counter this effect by exerting a dorsalizing influence.
Development of the overall cross-sectional organization of the neural tube involves not only a ventralizing influence from the notochord, but also an opposing dorsalizing influence from the epidermal ectoderm adjacent to the developing neural tube. In the lateral regions of the neural plate (future dorsal region of the neural tube), BMP-4 and BMP-7, expressed by non-neural ectoderm at the ectodermal–lateral neural plate junction, exert a dorsalizing inductive effect on the neuroectodermal cells that results in the formation of the roof plate, which takes shape soon after the last neural crest cells have emigrated from the neural tube. BMP within the roof plate acts as a patterning signal and induces the further dorsalizing molecules Pax-3, Pax-7, Msx-1, and Msx-2 (see Fig. 11.9). Dorsal Wnt signaling promotes the proliferation of neural progenitor cells and also works with BMPs as an overall dorsalizing influence in the dorsoventral patterning of neurons. After closure of the neural tube, signals from the roof plate induce a series of six early and two late generated dorsal interneuronal types in a manner reminiscent of the better defined specification of ventral interneurons (see later).
While the broader regions of the cross section of the spinal cord are being set in place, a tightly controlled molecular grid forms the basis for specification of the major types of neurons found in the ventral part of the spinal cord. Within the basal plate is an array of five types of neurons—motoneurons and four types of interneurons—that are arranged in a well-defined dorsoventral pattern. These classes of neurons are specified by specific combinations of homeodomain transcription factors, whose pattern of expression is set by a gradient of shh emanating from the floor plate as modulated by the activating and repressive properties of the Gl-1 to Gl-3 proteins (Fig. 11.10). Some of these transcription factors (class I) are repressed at various dorsoventral levels by the shh gradient, whereas others (class II) are induced by shh (see Fig. 11.10). The net result is that a different combination of the transcription factors at each dorsoventral level specifies each of the five types of neurons, which are characterized by their own unique molecular signature. One in particular, islet-1, is characteristic of motoneurons. Shortly after the production of motoneurons has ceased, a shift in regulatory factors stimulates the production of glial progenitor cells from the ventral neuroepithelium. This leads to the formation of the oligodendrocytes that become closely associated with the neurons.
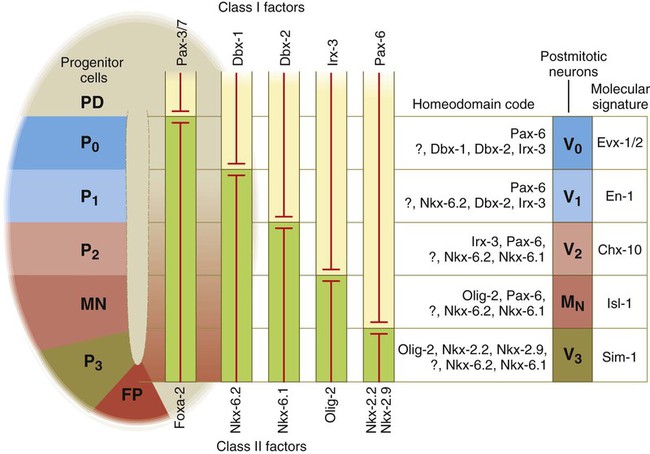
At the left, various classes of progenitor neurons are given labels beginning in P. At the right of the neural tube, a decreasing concentration gradient of sonic hedgehog is indicated by the fading red-brown background. The class I factors (upper bars) are repressed by sonic hedgehog, whereas the class II factors are induced by sonic hedgehog. To the right of the bars is the set of homeodomain codes that specify the various levels of precursor neurons, and at the far right is the molecular signature of the neurons. FP, floor plate; MN, motoneuron precursors.
Craniocaudal Pattern Formation and Segmentation
Through neural induction, the early central nervous system becomes organized into broad regions that develop cranial, middle, and caudal characteristics. This is soon followed by the appearance of the morphological subdivisions outlined in Figure 11.2. At an even finer level, segments called rhombomeres appear in the region of the hindbrain (see Fig. 6.3), and a less distinct series of subdivisions called prosomeres appears in the forebrain.
Patterning in the Hindbrain and Spinal Cord Regions
The rhombomeres (Fig. 11.11), introduced in Chapter 6, are the morphological reflection of a highly segmentally ordered pattern of expression of a variety of developmentally prominent transcription factors (Fig. 11.12). The establishment of the isthmic organizer and the pathways that set up this pattern are discussed in Chapter 6 (see pp. 95-96).
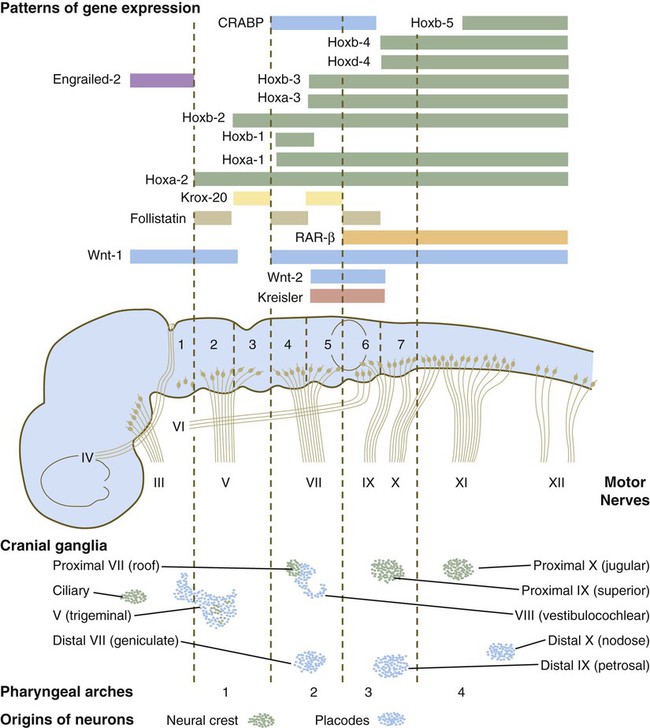
The bars refer to craniocaudal levels of expression of a given gene product. Cranial sensory nerves derived from the neural crest and placodal precursors are laid out in proper register. CRABP, cytoplasmic retinoic acid–binding protein; RAR, retinoic acid receptor. (Adapted from Noden DM: J Craniofac Genet Dev Biol 11:192-213, 1991.)
The correspondence between the rhombomeres of the developing hindbrain and other structures of the cranial and pharyngeal arch region is remarkable (see Chapter 14). The cranial nerves, which have a highly ordered pattern by which they supply structures derived from the pharyngeal arches and other structures in the head, have an equally highly ordered origin with respect to the rhombomeres (Fig. 11.13). Cranial nerve V innervates structures derived from the first pharyngeal arch. Cranial nerves VII and IX innervate the second and third arch structures. In embryos of birds, the species studied most extensively, cell bodies of the motor components of cranial nerves V, VII, and IX are initially found exclusively in rhombomeres 2, 4, and 6.
The cell bodies (within the central nervous system, the collection of cell bodies of a single cranial nerve is called a nucleus) of the cranial nerves that innervate the pharyngeal arches arise in register along the craniocaudal axis. The motor nuclei of other cranial nerves that innervate somatic structures (e.g., extraocular muscles or the tongue) arise in a different craniocaudal column along the hindbrain and do not occupy contiguous rhombomeres (see Fig. 11.13).
Patterning in the Midbrain Region
A fundamental patterning mechanism in the midbrain region is a molecular signaling center (isthmic organizer) located at the border between the mesencephalon and the metencephalon (see Fig. 6.4). The principal signaling molecule is FGF-8, which is expressed in a narrow ring at the anterior border of the first rhombomere, a subdivision of the metencephalon. Acting with Wnt-1, FGF-8 induces the expression of engrailed genes En-1 and En-2 and Pax-2 and Pax-5, which are expressed in decreasing concentrations at increasing distances from the FGF-8 signaling center (see Fig. 6.4). The main function of Wnt-1 seems to be the stimulation of local cell proliferation, whereas the overall organizing function belongs to FGF-8. The isthmic organizer induces and polarizes the dorsal midbrain region and the cerebellum. Grafts of the isthmic organizer or beads releasing FGF-8 alone into more cranial regions of the forebrain of the avian embryo induce a second tectum (dorsal mesencephalon or colliculi in mammals). Similarly, isthmic grafts into regions of the hindbrain can induce supernumerary cerebellar structures.
Cranially, the midbrain is separated from the forebrain (diencephalon) through a different set of molecular interactions. The alar plate of the diencephalon is characterized by the expression of Pax-6, a molecule that, among other things, acts as the master gene underlying eye formation (see Chapter 13). The mesencephalon is a domain of En-1 expression. Through the action of intermediate negative regulators, Pax-6 inhibits En-1 expression, whereas En-1 directly inhibits Pax-6 expression. The craniocaudal level at which each of these molecules inhibits the other becomes a sharp diencephalic-mesencephalic border.
Patterning in the Forebrain Region
Although much less apparent than in the hindbrain, the neuromeric organization of the early brain extends into the forebrain region as a set of three prosomeres, which extend from the midbrain-forebrain boundary through the thalamus (Fig. 11.14). Prosomeres 1 to 3 (p1 to p3), the most posterior prosomeres, become incorporated into the diencephalon, with p2 and p3 forming the dorsal and ventral thalamus, which serves as a major relay station for transmitting neural signals between the cerebral cortex and the body. An earlier representation suggested that an additional set of prosomeres (p4 to p6) formed the organizational basis for the rostral diencephalon (hypothalamus) and telencephalon. A more recent interpretation, however, places these structures in what has been called the secondary rhombencephalon, a developmental field that encompasses the entire prechordal portion of the neural tube. Within this domain, the basal plate develops into the major regions of the hypothalamus, the structure that integrates autonomic nervous functions and controls endocrine release from the pituitary. The alar plate in this domain is the precursor of the cerebral cortex, the basal ganglia (collectively the telencephalon), and the optic vesicles (diencephalic structures that take the lead in formation of the eyes). As development progresses, the secondary rhombencephalon becomes sharply folded beneath p2 to p3, and in humans the enormous outgrowth of the alar plates of the secondary rhombencephalon envelops the other prosomeres as the telencephalic vesicles (future cerebral cortex).
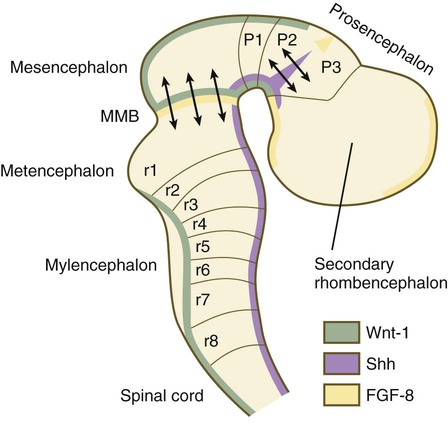
The midbrain/hindbrain signaling region is indicated by the arrows just rostral to the first rhombomere. The arrows between the second and third prosomeres represent the zona limitans interthalamica, a signaling region in the forebrain. MMB, mesencephalic-metencephalic border. (Adapted from Bally-Cuif L, Wassef M: Determination events in the nervous system of the vertebrate embryo, Curr Opin Genet Dev 5:450-458, 1995.)
Discrete patterns of gene expression also mirror the basic regional organization of the forebrain. Early in development, FGF-8, secreted by the anterior neural ridge (see Fig. 6.4B), induces the expression of Foxg-1, formerly called BF-1 (brain factor-1), which regulates development of the telencephalon and optic vesicles. Within the forebrain, a thin rim of expression of the transcription factor Nkx 2.2 marks the border between alar and basal plates. Along the dorsoventral axis, the alar plates are characterized by the expression of Emx1 and Emx2 and Pax6, important regulators of regional identity within the cerebral cortex. In an intermediate region of the prosencephalic alar plate, Emx expression drops out, leaving Pax-6 to function as the master gene controlling eye development (see Chapter 13). In the more ventral area that eventually forms the basal ganglia, Dlx (distalless) is a dominant expressed gene.
Similar to the spinal cord, the ventral forebrain is induced and organized by shh, secreted by midline axial structures. In the absence of shh signaling in this area, the tissues of the ventral forebrain are greatly reduced, leading sometimes to midline fusion of the optic vesicles and a general reduction of the growth of the midface region. This situation results in a type of anomaly called holoprosencephaly (see p. 309), which in extreme cases is accompanied by cyclopia.
At the border between the future dorsal (p2) and ventral (p3) thalamus is a narrow band of shh expression called the zona limitans interthalamica. Signals emanating from the zona limitans interthalamica specify aspects of cellular identity and behavior in the diencephalic regions on either side (see Fig. 6.4B). The mechanisms leading to the formation of the zona limitans interthalamica are poorly understood, but this structure arises directly above the anterior tip of the notochord. This finding suggests that the formation of this structure, similar to that of the isthmus, is a reflection of an earlier molecular boundary zone.
Peripheral Nervous System
Structural Organization of a Peripheral Nerve
The formation of a peripheral nerve begins with the outgrowth of axons from motor neuroblasts located in the basal plate (the future ventral horn of the gray matter) of the spinal cord (Fig. 11.15). Near the dorsal part of the spinal cord, thin processes also begin to grow from neural crest–derived neuroblasts that have aggregated to form the spinal ganglia. Dendrites, which conduct impulses toward the nerve cell body, grow from the sensory neurons toward the periphery. Axons, which conduct impulses away from the cell body, penetrate the dorsolateral aspect of the spinal cord and terminate in the dorsal horn (the gray matter of the alar plate). Within the gray matter, short interneurons connect the terminations of the sensory axons to the motoneurons. These three connected neurons (motor, sensory, and interneuron) constitute a simple reflex arc through which a sensory stimulus can be translated into a simple motor response. Autonomic nerve fibers are also associated with typical spinal nerves.
A long-standing question concerning the organization of the nervous system involves the interface between the central nervous system and the peripheral nervous system, in particular how their respective cellular components are kept separate. Such separation at the points of exit of the motor axons from the ventral neural tube and the entry into the dorsal neural tube of spinal axons from the dorsal roots is accomplished by localized boundary caps of neural crest cells (Fig. 11.16). The boundary caps act as selective filters, which allow the free passage of outgrowing and ingrowing axons between the neural tube and the periphery, but which serve as a barrier to keep cells in their appropriate compartment. In the absence of boundary caps, many cell bodies of motoneurons translocate away from the lateral motor column (their normal location) into the space outside the neural tube.
Within a peripheral nerve, the neuronal processes can be myelinated or unmyelinated. At the cellular level, myelin is a multilayered spiral sheath consisting largely of phospholipid material that is formed by individual Schwann cells (neural crest derivatives) that wrap themselves many times around a nerve process like a jelly roll (see Fig. 11.16). This wrapping serves as a form of insulation that largely determines the character of the electrical impulse (action potential) traveling along the neuronal process. Unmyelinated nerve fibers are also embedded in the cytoplasm of Schwann cells, but they lack the characteristic spiral profiles of myelinated processes (see Fig. 11.16).
Patterns and Mechanisms of Neurite Outgrowth
An actively elongating neurite is capped by a growth cone (Fig. 11.17). Growth cones are characterized by an expanded region of cytoplasm with numerous spikelike projections called filopodia. In vitro and in vivo studies of living nerves show that the morphology of an active growth cone is in a constant state of flux, with filopodia regularly extending and retracting as if testing the local environment. Growth cones contain numerous cytoplasmic organelles, but much of the form and function of the filopodia depends on the large quantities of actin microfilaments that fill these processes. In a growth cone, an equilibrium exists between the extension of actin microfilaments by terminal addition and resorption at the proximal end. Under conditions favorable for growth, the balance tips toward extension, whereas an unfavorable environment results in the resorption of the microfilaments and collapse of the growth cone.
Many molecules are involved in the guidance of neuronal outgrowth (Table 11.1). Some of these molecules can exert either attractive or repulsive actions, depending on the character of the neuron, the time in development, or some combination of intrinsic and extrinsic characteristics. To a greater or lesser extent, the nature of the reaction of the growth cone is determined locally, because in vitro studies have shown that such reactions can occur even when the neuronal process is cut off from the cell body.
Table 11.1
Receptor/Ligand Pairs Known to Influence Axonal Outgrowth* During Embryonic Development
Ligand | Receptor |
Slit | Robo-1, Robo-4 |
Ephrin | Eph |
Netrin | UNC-5, DCC |
Semaphorin | Plexin, Neuropilin |
VEGF | VEGFR, Neuropilin |
Draxin | Netrin receptors |
Outgrowing axons and dendrites differ in several important ways. In contrast to axons, dendrites contain microtubules with polarity running in both directions (Fig. 11.18). Another prominent difference is the absence of GAP-43 protein in growing dendrites. Among the first signs of polarity of a developing neuron are the concentration of GAP-43 in the outgrowing axon and its disappearance from the dendritic processes.
Neurite-Target Relations During Development of a Peripheral Nerve
In the case of motoneurons, evidence that very specific cues guide individual nerves and axons to their muscle targets is increasing. Tracing and transplantation studies have shown that outgrowing motor nerves to limbs supply the limb muscles in a well-defined order, and that after minor positional dislocations, they seek out the correct muscles (see Fig. 10.28). More recent evidence suggests that even at the level of neurons, “fast” axons are attracted to the precursors of fast muscle fibers, and “slow” axons are attracted to the precursors of slow muscle fibers. There are many similar examples of target specificity in dendrites in the peripheral nervous system and of dendrites and axons in the central nervous system. Even within a single developing muscle fiber, an aggregation of muscle-specific tyrosine kinase receptor molecules determines the exact site at which the outgrowing axonal terminal connects with the muscle fiber.
When a motor axon and a muscle fiber meet, a complex series of changes in the nerve and muscle fibers mark the formation of a functional synapse, in this case called a neuromuscular junction (Fig. 11.19). The early changes consist of (1) the cessation of outgrowth of the axon, (2) the preparation of the nerve terminal for the ultimate release of appropriate neurotransmitter molecules, and (3) modifications of the muscle fibers at the site of nerve contact so that the neural stimulus can be received and translated into a contractile stimulus. Both neural and muscular components of the neuromuscular junction are involved in stabilizing the morphological and functional properties of this highly specialized synapse.
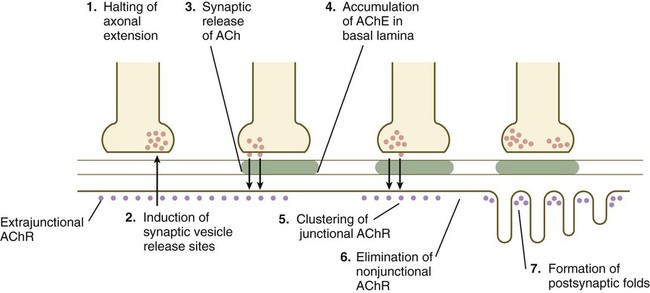
ACh, acetylcholine; AChE, acetylcholinesterase; AChR, acetylcholine receptors.
As the growth cone of a motoneuron reaches a muscle fiber, synaptic vesicles begin to accumulate in the growth cone, and an interaction with a nerve-specific form of laminin begins to stabilize the neuromuscular contact. Further stabilization is provided by a protein, agrin, which becomes concentrated at the neuromuscular junction. The synaptic vesicles store and ultimately release the neurotransmitter substance acetylcholine from the nerve terminal (see Fig. 11.19). Before the developing muscle fiber is contacted by the motoneuron, acetylcholine receptors (nonjunctional type) are scattered throughout the length of the muscle fiber. After initial nerve contact, myonuclei in the vicinity of the neuromuscular junction produce junction-specific acetylcholine receptors that reside on nerve-induced postjunctional folds of the muscle fiber membrane, and the scattered nonjunctional receptors disappear. Between the nerve terminal and the postsynaptic apparatus of the muscle fiber lies a basal lamina containing molecules that stabilize the acetylcholine receptors at the neuromuscular junction and acetylcholinesterase, an enzyme produced by the muscle fiber.
Factors Controlling Numbers and Kinds of Connections Between Neurites and End Organs in the Peripheral Nervous System
1. Some axons fail to reach their normal target, and cell death is a way of eliminating them.
2. Cell death could be a way of reducing the size of the neuronal pool to something appropriate to the size of the target.
3. Similarly, cell death could compensate for a presynaptic input that is too small to accommodate the neurons in question.
4. Neuronal cell death may also be a means of eliminating connection errors between the neurons and their specific end organs.
Autonomic Nervous System
The autonomic nervous system is the component of the peripheral nervous system that subserves many of the involuntary functions of the body, such as glandular activity and motility within the digestive system, heart rate, vascular tone, and sweat gland activity. It has two major divisions—the sympathetic and parasympathetic nervous system. Components of the sympathetic nervous system arise from the thoracolumbar levels (T1 to L2) of the spinal cord, whereas the parasympathetic nervous system has a widely separated dual origin from the cranial and sacral regions. Both components of the autonomic nervous system consist of two tiers of neurons: preganglionic and postganglionic. Postganglionic neurons are derivatives of the neural crest (see Chapter 12).
Sympathetic Nervous System
Preganglionic neurons of the sympathetic nervous system arise from the intermediate horn (visceroefferent column) of the gray matter in the spinal cord. At levels from T1 to L2, their myelinated axons grow from the cord through the ventral roots, thus paralleling the motor axons that supply the skeletal musculature (Fig. 11.20). Shortly after the dorsal and ventral roots of the spinal nerve join, the preganglionic sympathetic axons, which are derived from the neuroepithelium of the neural tube, leave the spinal nerve via a white communicating ramus. They soon enter one of a series of sympathetic ganglia to synapse with neural crest–derived postganglionic neurons.
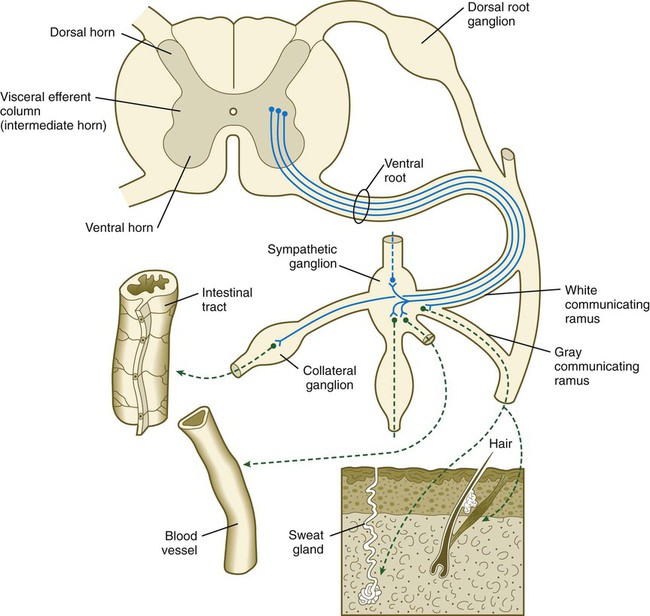
First-order sympathetic neurons are indicated by solid blue lines; second-order sympathetic neurons are indicated by dashed green lines.
The sympathetic ganglia, the bulk of which are organized as two chains running ventrolateral to the vertebral bodies, are laid down by neural crest cells that migrate from the closing neural tube along a special pathway (see Fig. 12.4). When the migrating sympathetic neuroblasts have reached the site at which the sympathetic chain ganglia form, they spread cranially and caudally until the extent of the chains approximates that seen in an adult. Some of the sympathetic neuroblasts migrate farther ventrally than the level of the chain ganglia to form a variety of other collateral ganglia (e.g., celiac and mesenteric ganglia), which occupy variable positions within the body cavity. The adrenal medulla can be broadly viewed as a highly modified sympathetic ganglion.
The outgrowing preganglionic sympathetic neurons either terminate within the chain ganglia or pass through on their way to more distant sympathetic ganglia to form synapses with the cell bodies of the second-order postganglionic sympathetic neuroblasts (see Fig. 11.20). Axons of some postganglionic neuroblasts, which are unmyelinated, leave the chain ganglia as a parallel group and reenter the nearest spinal nerve through the gray communicating ramus. When in the spinal nerve, these axons continue to grow until they reach appropriate peripheral targets, such as sweat glands, arrector pili muscles, and walls of blood vessels. Axons of other postganglionic sympathetic neurons leave their respective ganglia as tangled plexuses of nerve fibers and grow toward other visceral targets.
Parasympathetic Nervous System
The neural crest precursors of the postganglionic neurons often undertake extensive migrations (e.g., from the hindbrain to final locations in the walls of the intestines). The migratory properties of the neural crest precursors of parasympathetic neurons are impressive, but this population of cells also undergoes a tremendous expansion until the final number of enteric neurons approximates the number of neurons in the spinal cord. Evidence is increasing that factors in the gut wall stimulate the mitosis of the neural crest cells migrating there. A striking demonstration of the stimulatory powers of the gut is the ability of pieces of gut wall transplanted along the neural tube to cause great expansion of the region of the neural tube closest to the graft (Fig. 11.21).
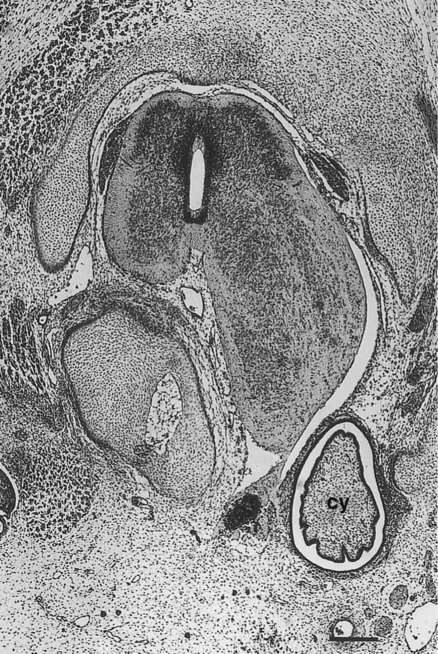
A graft of quail duodenum was placed between the neural tube and somites of a chick embryo host. The spinal cord on the side near the graft of gut has greatly enlarged, causing secondary distortion of the musculoskeletal structures near it. cy, cyst of donor endoderm. (From Rothman TP and others: Dev Biol 124:331-346, 1987.)
Differentiation of Autonomic Neurons
At late stages in their development, autonomic neurons still retain flexibility in their choice of neurotransmitter. Sympathetic neurons in newborn rats are normally adrenergic, and if grown in standard in vitro culture conditions, these neurons produce large amounts of norepinephrine and negligible amounts of acetylcholine. If the same neurons are cultured in a medium that has been conditioned by the presence of cardiac muscle cells, they undergo a functional conversion and instead produce large amounts of acetylcholine (Fig. 11.22).
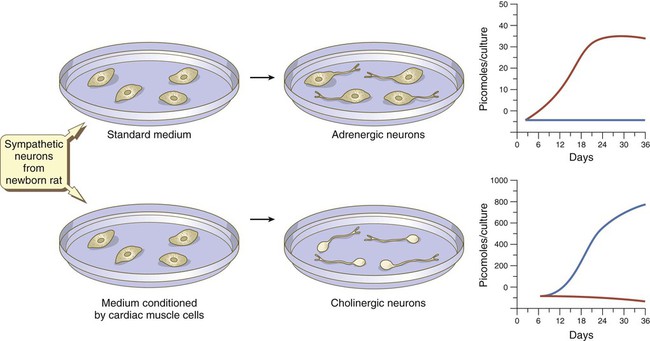
In standard medium, the neurons become adrenergic; in medium conditioned by cardiac muscles, they become cholinergic. Levels of norepinephrine are in red; levels of acetylcholine are in blue. (Based on Patterson PH and others: Sci Am 239:50-59, 1978.)
Congenital Aganglionic Megacolon (Hirschsprung’s Disease)
If a newborn exhibits symptoms of complete constipation in the absence of any demonstrable physical obstruction, the cause is usually an absence of parasympathetic ganglia from the lower (sigmoid) colon and rectum. This condition, commonly called aganglionic megacolon or Hirschsprung’s disease (see p. 350), is normally attributed to the absence of colonization of the wall of the lower colon by neural crest–derived parasympathetic neuronal precursors, probably of cranial origin. In rare cases, greater parts of the colon lack ganglia.
Later Structural Changes in the Central Nervous System*
Histogenesis Within the Central Nervous System
A major difference between the brain and the spinal cord is the organization of the gray and white matter. In the spinal cord, the gray matter is centrally located, with white matter surrounding it (see Fig. 11.6). In many parts of the brain, this arrangement is reversed, with a large core of white matter and layers of gray matter situated superficial to this core.
A fundamental process in histogenesis within the brain is cell migration. From their sites of origin close to the ventricles in the brain, neuroblasts migrate toward the periphery by following set patterns. These patterns often result in a multilayering of the gray substance of the brain tissue. Key players in the migratory phenomenon are radial glial cells, which extend long processes from cell bodies located close to the ventricular lumen toward the periphery of the developing cortex (Fig. 11.23). Young postmitotic neurons, which are usually simple bipolar cells, wrap themselves around the radial glial cells and use them as guides on their migrations from their sites of origin to the periphery.
Spinal Cord
The early spinal cord is divided into alar and basal plate regions, which are precursors for the sensory and motor regions of the spinal cord (see Fig. 11.6). The mature spinal cord has a similar organization, but these regions are subdivided further into somatic and visceral components. Within the brain, still another layer of input and output is added with “special” components. These components are summarized in Box 11.1.
A gross change of the spinal cord that is of clinical significance is the relative shortening of the spinal cord in relation to the vertebral column (Fig. 11.24). In the first trimester, the spinal cord extends the entire length of the body, and the spinal nerves pass through the intervertebral spaces directly opposite their site of origin. In later months, growth of the posterior part of the body outstrips that of the vertebral column and the spinal cord, but growth of the spinal cord lags significantly behind that of the vertebral column. This disparity is barely apparent in the cranial and thoracic regions, but at birth the spinal cord terminates at the level of L3. By adulthood, the spinal cord terminates at L2.
Myelencephalon
The myelencephalon, the most caudal subdivision of the rhombencephalon (see Figs. 11.1 and 11.2), develops into the medulla oblongata of the adult brain (Fig. 11.25). It is in many respects a transitional structure between the brain and spinal cord, and the parallels between its functional organization and that of the spinal cord are readily apparent (Fig. 11.26). Much of the medulla serves as a conduit for tracts that link the brain with input and output nodes in the spinal cord, but it also contains centers for the regulation of vital functions such as the heartbeat and respiration.
The fundamental arrangement of alar and basal plates with an intervening sulcus limitans is retained almost unchanged in the myelencephalon. The major topographical change from the spinal cord is a pronounced expansion of the roof plate to form the characteristic thin roof overlying the expanded central canal, which in the myelencephalon is called the fourth ventricle (see Fig. 11.37). (Details of the ventricles and the coverings of the brain and spinal cord are presented later in this chapter.)
Metencephalon
The metencephalon, the more cranial subdivision of the hindbrain, consists of two main parts: the pons, which is directly continuous with the medulla, and the cerebellum, a phylogenetically newer and ontogenetically later-appearing component of the brain (see Fig. 11.25). The formation of these structures depends on the inductive activity of FGF-8 emanating from the isthmic organizer (see Fig. 6.4).
As its name implies, the pons, derived from the basal plate, serves as a bridge that carries tracts of nerve fibers between higher brain centers and the spinal cord. Its fundamental organization remains similar to that of the myelencephalon, with three sets of afferent and efferent nuclei (Fig. 11.27). In addition to these nuclei, other special pontine nuclei, which originated from alar plate–derived neuroblasts, are present in the ventral white matter. The caudal part of the pons also has an expanded roof plate similar to that of the myelencephalon.
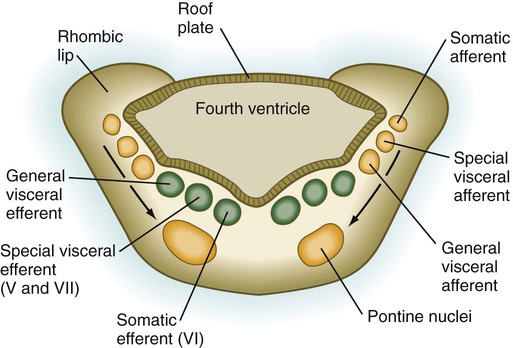
Motor tracts are shown in green; sensory tracts are orange. (Adapted from Sadler T: Langman’s medical embryology, ed 6, Baltimore, 1990, Williams & Wilkins.)
The future site of the cerebellum is first represented by the rhombic lips of the 5- to 6-week embryo. The rhombic lips represent the diamond (rhombus)–shaped border between the thin roof plate and the main body of the rhombencephalon (Fig. 11.28). The rhombic lips, situated from rhombomeres 1 to 8 (r1 to r8), are the product of an inductive interaction between the roof plate (through BMP signaling) and the neural tube within the original rhombencephalon. The cerebellum proper arises from the anterior (cerebellar) rhombic lips (r1), whereas the posterior rhombic lips (r2 to r8) give rise to the migratory precursors of a variety of ventrally situated nuclei (e.g., olivary and pontine nuclei; see Figs. 11.26 and 11.27) that are located in the metencephalon and rhombencephalon. Soon after the induction of the rhombic lips, precursors of granule cells migrate anteriorly along the dorsal region of r1 from the cerebellar rhombic lips to form a transient germinal epithelium called the external granular layer (Fig. 11.29). After terminal mitotic divisions, the postmitotic external granule cells undergo a ubiquitin-mediated second radial migration toward the interior of the future cerebellum. En route, these cells pass through a layer of precursors of the larger Purkinje cells, which are migrating radially in the opposite direction. Once past the Purkinje cells, the migrating granule cells settle in the inner granular layer, simply called the granular layer in the mature cerebellum (Fig. 11.30E; see Fig. 11.29). A strong interaction exists between the Purkinje cells and the granule cells, which become the most numerous cell type within the entire central nervous system. Purkinje cells secrete shh, which is a key mitogen inducing proliferation of granule precursor cells.
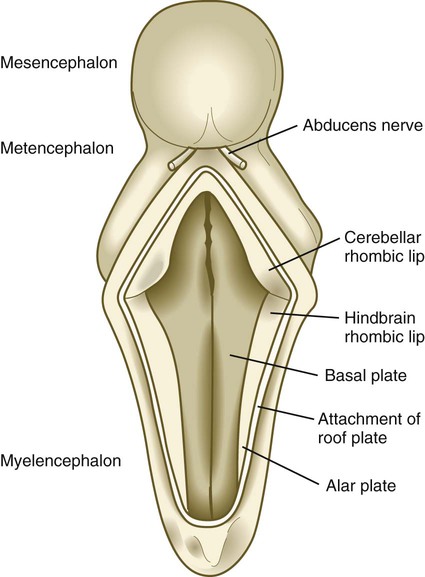
The roof of the fourth ventricle has been opened.
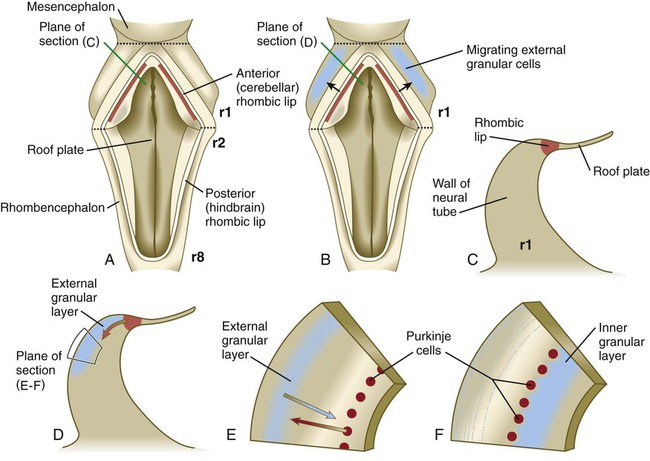
A, Dorsal view of the hindbrain. B, Dorsal view of the early migration of the external granular cells. C, Cross-sectional view of the premigratory stage, indicated by the red line in A. D, Cross-sectional view of the early migratory stage, indicated by the red line in B. E and F, Cross-sectional views of later stages of inward migration of the external granular cells and outward migration of the Purkinje cells.
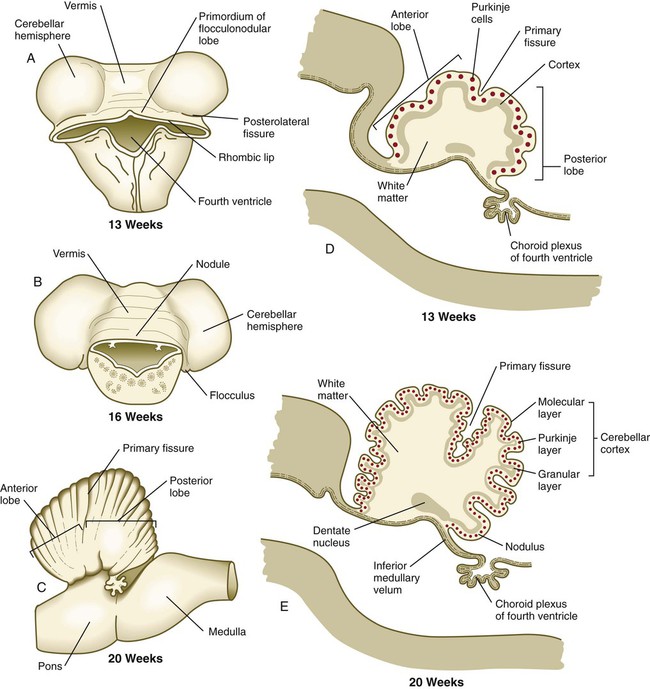
A and B, Dorsal views. C, Lateral view. D and E, Sagittal sections.
Until the end of the third month, the expansion of the cerebellar rhombic lips is mainly anterior and inward, but thereafter the rapid growth in volume of the cerebellum is directed outward (see Fig. 11.30). As the volume of the developing cerebellum expands, the two lateral rhombic lips join in the midline, thus giving the early cerebellar primordium a dumbbell appearance. The cerebellum then enters a period of rapid development and external expansion. As the complex process of cerebellar histogenesis proceeds, many fibers emanating from the vast number of neurons generated in the cerebellar cortex leave the cerebellum through a pair of massive superior cerebellar peduncles, which grow into the mesencephalon.
Mesencephalon
The mesencephalon, or midbrain, is structurally a relatively simple part of the brain in which the fundamental relationships between the basal and alar plates are preserved (Fig. 11.31). As in the spinal cord, dorsoventral organization within the midbrain is heavily based on shh signaling from the floor, which in addition to promoting neuronal development within the basal plate, suppresses the expression of molecules characteristic of the alar plate. A late function of Otx-2 in the region of the border between the alar and basal plates confines shh activity to the basal part of the midbrain.
The third major region of the mesencephalon is represented by prominent ventrolateral bulges of white matter called the cerebral peduncles (crus cerebri; see Fig. 11.31). Many of the major descending fiber tracts pass through these structures on their way from the cerebral hemispheres to the spinal cord.
Diencephalon
Development of the early diencephalon is characterized by the appearance of two pairs of prominent swellings on the lateral walls of the third ventricle. These swellings line the greatly expanded central canal in this region (see Fig. 11.25). The largest pair of masses represents the developing thalamus, in which neural tracts from higher brain centers synapse with the tracts of other regions of the brain and brainstem. Among the many thalamic nuclei are those that receive input from the auditory and visual systems and transmit them to the appropriate regions of the cerebral cortex. In later development, the thalamic swellings may thicken to the point where they meet and fuse in the midline across the third ventricle. This connection is called the massa intermedia.
In early embryos (specifically, embryos around 7 to 8 weeks’ gestational age), a pair of less prominent bulges dorsal to the thalamus marks the emergence of the epithalamus (see Fig. 11.25), a relatively poorly developed set of nuclei relating to masticatory and swallowing functions. The most caudal part of the diencephalic roof plate forms a small diverticulum that becomes the epiphysis (pineal body), a phylogenetically primitive gland that often serves as a light receptor. Under the influence of light-dark cycles, the pineal gland secretes (mainly at night) melatonin, a hormone that inhibits function of the pituitary-gonadal axis of hormonal control.
A ventral downgrowth from the floor of the diencephalon, known as the infundibular process, joins with a midline outpocketing from the stomodeum (Rathke’s pouch) to form the two components of the pituitary gland. The development of the pituitary gland is discussed in detail in Chapter 14.
The optic cups are major outpocketings of the diencephalic wall during early embryogenesis. Earlier in development, the ventral diencephalon constitutes a single optic field, characterized by the expression of Pax-6. Then the single optic field is separated into left and right optic primordia by anterior movements of ventral diencephalic cells, which depend on the expression of the gene cyclops. Further development of the optic cups and the optic nerves (cranial nerve II) is discussed in Chapter 13.
Telencephalon
Telencephalic development is the product of interactions among three patterning centers in the forebrain. The rostral patterning center, derived from the earlier anterior neural ridge (see Fig. 6.4B), secretes FGF-8, which directly affects the two other patterning centers—the dorsal patterning center (sometimes called the cortical hem), which produces BMPs and Wnts, and the ventral patterning center, which produces shh. Acting through molecules, such as Emx-2, FGF-8 plays a significant role in the overall growth of the telencephalon. FGF-8 mutants are characterized by reduced telencephalic size and a shift toward sensory versus frontal functions. Wnts, produced by the dorsal patterning center, promote the formation of caudal telencephalic structures, such as the hippocampus, and BMPs pattern the dorsal midline and induce choroid plexus formation. Working through the downstream molecule, Nkx-2.1, FGF-8 from the rostral patterning center may provide an early step in ventralizing the telencephalon through its effect on shh. After these early patterning events, telencephalic development is marked by tremendous growth.
Development of the telencephalon is dominated by the massive expansion of the bilateral telencephalic vesicles, which ultimately become the cerebral hemispheres (see Fig. 11.25). The walls of the telencephalic vesicles surround the expanded lateral ventricles, which are outpocketings from the midline third ventricle located in the diencephalon (see Fig. 11.37). Although the cerebral hemispheres first appear as lateral structures, the dynamics of their growth cause them to approach the midline over the roofs of the diencephalon and mesencephalon (Fig. 11.32). The two cerebral hemispheres never meet in the dorsal midline because they are separated by a thin septum of connective tissue (part of the dura mater) known as the falx cerebri. Below this septum, the two cerebral hemispheres are connected by the ependymal roof of the third ventricle.
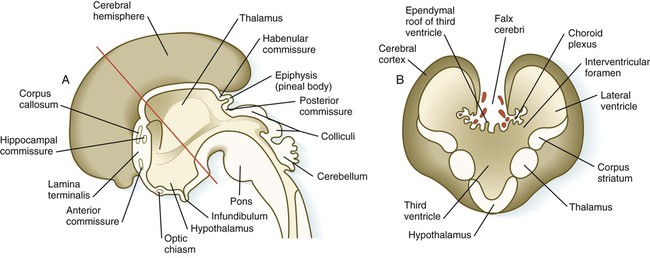
A, Sagittal section through the brain. B, Cross section through the level indicated by the red line in A. (Adapted from Moore K, Persaud T: The developing human, ed 5, Philadelphia, 1993, WB Saunders.)
Although the cerebral hemispheres expand greatly during the early months of pregnancy, their external surfaces remain smooth until the fourteenth week. With continued growth, the cerebral hemispheres undergo folding at several levels of organization. The most massive folding involves the large temporal lobes, which protrude laterally and rostrally from the caudal part of the cerebral hemispheres. From the fourth to the ninth month of pregnancy, the expanding temporal lobes and the frontal and parietal lobes completely cover areas of the cortex known as the insula (island) (Fig. 11.33). While these major changes in organization are occurring, other precursors of major surface landmarks of the definitive cerebral cortex are being sculpted. Several major sulci and fissures begin to appear as early as the sixth month. By the eighth month, the sulci (grooves) and gyri (convolutions) that characterize the mature brain take shape.
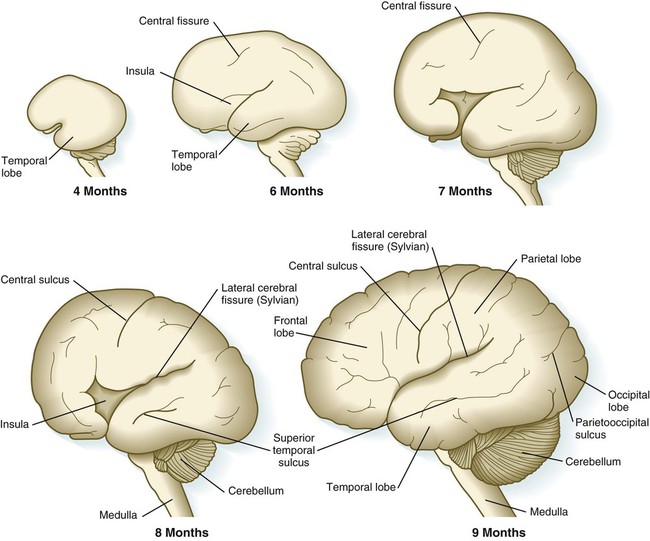
Internally, the base of each telencephalic vesicle thickens to form the comma-shaped corpus striatum (Fig. 11.34). Located dorsal to the thalamus, the corpus striatum becomes more C-shaped as development progresses. With histodifferentiation of the cerebral cortex, many fiber tracts converge on the area of the corpus striatum, which becomes subdivided into two major nuclei—the lentiform nucleus and the caudate nucleus. These structures, which are components of the complex aggregation of nuclei known as the basal ganglia, are involved in the unconscious control of muscle tone and complex body movements.
Aside from the telencephalic vesicles, the other major component of the early telencephalon is the lamina terminalis, which forms its median rostral wall (Fig. 11.35; see Fig. 11.37A). Initially, the two cerebral hemispheres develop separately, but toward the end of the first trimester of pregnancy, bundles of nerve fibers begin to cross from one cerebral hemisphere to the other. Many of these connections occur through the lamina terminalis.
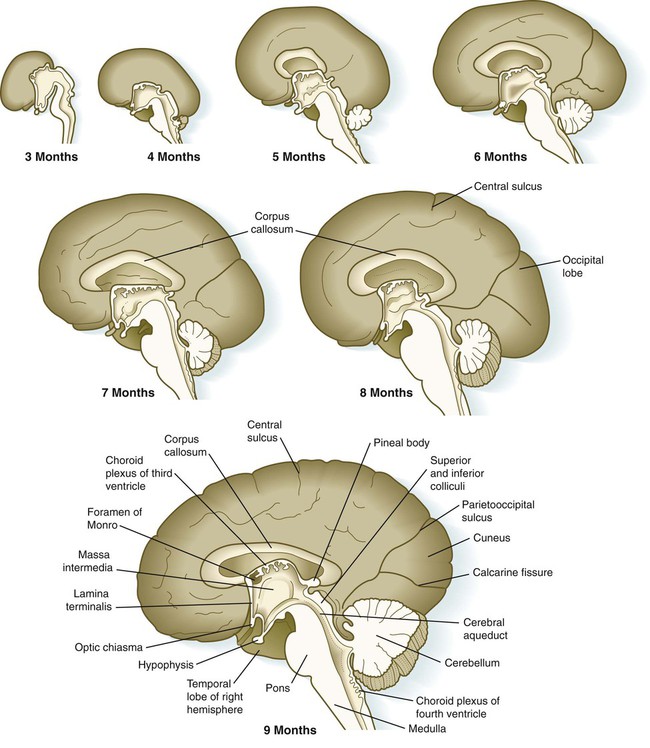
The first set of connections to appear in the lamina terminalis becomes the anterior commissure (see Fig. 11.25B), which connects olfactory areas from the two sides of the brain. The second connection is the hippocampal commissure (fornix). The third commissure to take shape in the lamina terminalis is the corpus callosum, the most important connection between the right and left halves of the brain. It initially forms (see Fig. 11.32A) at 74 days as a small bundle in the lamina terminalis, but it expands greatly to form a broad band connecting a large part of the base of the cerebral hemispheres (see Fig. 11.35). Formation of the corpus callosum is complete by 115 days. In mutations of the homeobox gene, EMX2, the corpus callosum fails to form, thus leading to an anomaly sometimes called schizencephaly (split brain). Other commissures not related to the lamina terminalis are the posterior and habenular commissures (see Fig. 11.32), which are located close to the base of the pineal gland, and the optic chiasma, the region in the diencephalon where parts of the optic nerve fibers cross to the other side of the brain.
Neuroanatomists subdivide the telencephalon into several functional components that are based on the phylogenetic development of this region. The oldest and most primitive component is called the rhinencephalon (also the archicortex and paleocortex). As the name implies, it is heavily involved in olfaction. The morphologically dominant cerebral hemispheres are called the neocortex. In early development, much of the telencephalon is occupied by rhinencephalic areas (Fig. 11.36), but with the expansion of the cerebral hemispheres, the neocortex takes over as the component occupying most of the mass of the brain.
Ventricles, Meninges, and Cerebrospinal Fluid Formation
The ventricular system of the brain represents an expansion of the central canal of the neural tube. As certain parts of the brain take shape, the central canal expands into well-defined ventricles, which are connected by thinner channels (Fig. 11.37). The ventricles are lined by ependymal epithelium and are filled with clear cerebrospinal fluid. Cerebrospinal fluid is formed in specialized areas called choroid plexuses, which are located in specific regions in the roof of the third, fourth, and lateral ventricles. Choroid plexuses are highly vascularized structures that project into the ventricles (see Fig. 11.32B) and secrete cerebrospinal fluid into the ventricular system.
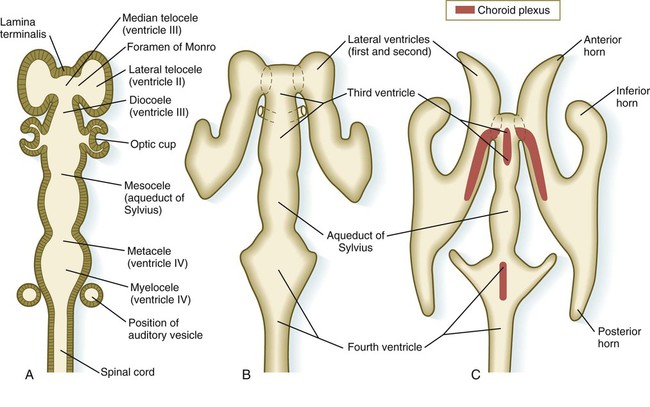
A, Section from an early embryo. B, Ventricular system during expansion of the cerebral hemispheres. C, Postnatal morphology of the ventricular system.
If an imbalance exists between the production and resorption of cerebrospinal fluid, or if its circulation is blocked, the fluid may accumulate within the ventricular system of the brain and, through increased mechanical pressure, result in massive enlargement of the ventricular system. This condition causes thinning of the walls of the brain and a pronounced increase in the diameter of the skull, a condition known as hydrocephalus (Fig. 11.38). The blockage of fluid can result from congenital stenosis (narrowing) of the narrow parts of the ventricular system, or it can be the result of certain fetal viral infections.
Cranial Nerves
Although based on the same fundamental plan as the spinal nerves, the cranial nerves (Fig. 11.39) have lost their regular segmental arrangement and have become highly specialized (Table 11.2). One major difference is the tendency of many cranial nerves to be either sensory (dorsal root based) or motor (ventral root based), rather than mixed, as is the case with the spinal nerves.
Table 11.2
Cranial Nerve | Associated Component of Central Nervous System | Functional Components | Distribution |
Olfactory (I) | Telencephalon/olfactory placode | Special sensory (olfaction) | Olfactory area of nose |
Optic (II) | Diencephalon (evagination) | Special sensory (vision) | Retina of eye |
Oculomotor (III) | Mesencephalon | Motor, autonomic (minor) | Intraocular and four extraocular muscles |
Trochlear (IV) | Mesencephalon (isthmus) | Motor | Superior oblique muscle of eye |
Trigeminal (V) | Metencephalon (r2, r3, [pharyngeal arch 1]) | Sensory, motor (some) | Derivatives of branchial arch I |
Abducens (VI) | Metencephalon (r5, r6) | Motor | Lateral rectus muscle of eye |
Facial (VII) | Metencephalic/myelencephalic junction (r4 [pharyngeal arch 2]) | Motor | Derivatives of branchial arch II |
Sensory (some) | |||
Autonomic (minor) | |||
Auditory (VIII) | Metencephalic/myelencephalic junction (r4-6, otic placode) | Special sensory (hearing, balance) | Inner ear |
Glossopharyngeal (IX) | Myelencephalon (r6, r7 [pharyngeal arch 3]) | Sensory, motor (some) | Derivatives of pharyngeal arch III |
Vagus (X) | Myelencephalon (r7, r8 [pharyngeal arch 4]) | Sensory, motor, autonomic (major) | Derivatives of pharyngeal arch IV |
Accessory (XI) | Myelencephalon (r7, r8 [pharyngeal arch 4]) | Motor | Gut, heart, visceral organs |
Spinal cord | Autonomic (minor) | Some neck muscles | |
Hypoglossal (XII) | Myelencephalon (r8 [arch 4]) | Motor | Tongue muscles |
The cranial nerves can be subdivided into several categories on the basis of their function and embryological origin. Cranial nerves I and II (olfactory and optic) are often regarded as extensions of brain tracts rather than true nerves. Cranial nerves III, IV, VI, and XII are pure motor nerves that seem to have evolved from primitive ventral roots. Nerves V, VII, IX, and X are mixed nerves with motor and sensory components, and each nerve supplies derivatives of a different pharyngeal arch (Fig. 11.40; see Table 11.2 and Fig. 14.34).
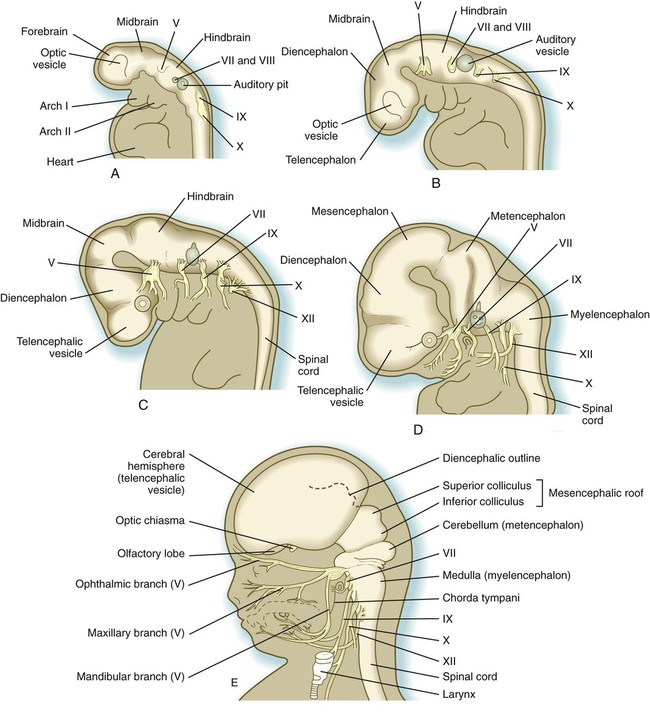
A, At


The sensory components of the nerves supplying the pharyngeal arches (V, VII, IX, and X) and the auditory nerve (VIII) have a multiple origin from the neural crest and the ectodermal placodes, which are located along the developing brain (see Fig. 13.1). These nerves have complex, often multiple sensory ganglia. Neurons in some parts of the ganglia are of neural crest origin, and neurons of other parts of ganglia arise from placodal ectoderm. (Ectodermal placodes are discussed on p. 269.)
Development of Neural Function
Spontaneous uncoordinated movements typically begin when the embryo is more than 7 weeks old. Later coordinated movements (see Fig. 18.7) are the result of the establishment of motor tracts and reflex arcs within the central nervous system. Behavioral development during the last trimester, which has been revealed by studying premature infants, is often subtle and reflects the structural and functional maturation of neuronal circuits.
The development of functional circuitry can be illustrated by the spinal cord. Several stages of structural and functional maturation can be identified (Fig. 11.41). The first is a prereflex stage, which is characterized by the initial differentiation (including axonal and dendritic growth) of the neurons according to a well-defined sequence, starting with motoneurons, followed by sensory neurons, and finally including the interneurons that connect the two (see Figs. 11.15 and 11.41A). The second stage consists of closure of the primary circuit, which allows the expression of local segmental reflexes. While the local circuit is being set up, other axons are growing down descending tracts in the spinal cord or are crossing from the other side of the spinal cord. When these axons make contact with the components of the simple reflex that was established in the second stage, the anatomical basis for intersegmental and cross-cord reflexes is set up. Later in the fetal period, these more complex circuits are completed, and the tracts are myelinated by oligodendrocytes.
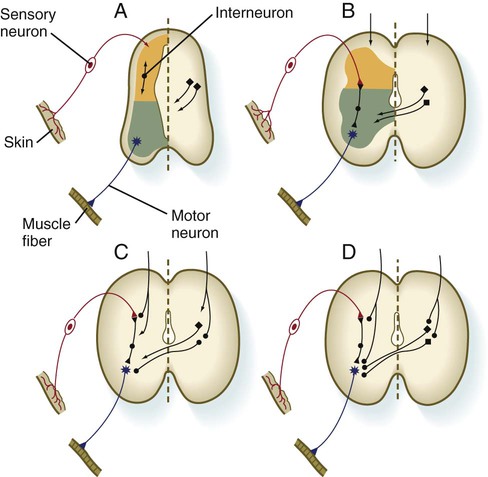
A, Presynaptic stage. B, Closure of the primary reflex circuit. C, Connections with longitudinal and lateral inputs. D, Completion of circuits and myelination. (Based on Bodian D: In Quartan GC, Melnechuk T, Adelman G, eds: The neurosciences: second study program, New York, 1970, Rockefeller University Press, pp 129-140.)
Clinical Correlation 11.1 presents congenital malformations of the nervous system.
Summary
While the neural tube is closing, its open ends are the cranial and caudal neuropores. The newly formed brain consists of three parts: the prosencephalon, the mesencephalon, and the rhombencephalon. The prosencephalon later subdivides into the telencephalon and the diencephalon, and the rhombencephalon forms the metencephalon and myelencephalon.
Within the neural tube, neuroepithelial cells undergo active mitotic proliferation. Their daughter cells form neuronal or glial progenitor cells. Among the glial cells, radial glial cells act as guidewires for the migration of neurons from their sites of origin to definite layers in the brain. Microglial cells arise from mesoderm.
The neural tube divides into ventricular, intermediate, and marginal zones. Neuroblasts in the intermediate zone (future gray matter) send out processes that collect principally in the marginal zone (future white matter). The neural tube is also divided into a dorsal alar plate and a ventral basal plate. The basal plate represents the motor component of the spinal cord, and the alar plate is largely sensory.
Through an induction by the notochord, mediated by shh, a floor plate develops in the neural tube. Further influences of shh, produced by the notochord and the floor plate, result in the induction of motoneurons in the basal plate.
Much of the early brain is a highly segmented structure. This structure is reflected in the rhombomeres and molecularly in the patterns of expression of homeobox-containing genes. Neurons and their processes developing within the rhombomeres follow specific rules of behavior with respect to rhombomere boundaries. Nerve processes growing from the spinal cord react to external cues provided by the environment of the somites. Neurons and neural crest cells can readily penetrate the anterior but not the posterior mesoderm of the somite.
The midbrain and metencephalic structures are specified by a signaling center, the isthmic organizer, at the midbrain-hindbrain border. FGF-8 is one of the major signaling molecules. The forebrain is divided into three segments called prosomeres. FGF-8, secreted by the anterior neural ridge, induces the expression of Foxg-1, which regulates development of the telencephalon and optic vesicles. Shh, secreted by midline axial structures, organizes the ventral forebrain.
A peripheral nerve forms by the outgrowth of motor axons from the ventral horn of the spinal cord. The outgrowing axons are capped by a growth cone. This growing tip continually samples its immediate environment for cues that guide the amount and direction of axonal growth. The motor component of a peripheral nerve is joined by the sensory part, which is based on neural crest–derived cell bodies in dorsal root ganglia along the spinal cord. Axons and dendrites from the sensory cell bodies penetrate the spinal cord and grow peripherally with the motor axons. Connections between the nerve and end organs are often mediated through trophic factors. Neurons that do not establish connections with peripheral end organs often die.
The autonomic nervous system consists of two components: the sympathetic nervous system and the parasympathetic nervous system. Both components contain preganglionic neurons, which arise from the central nervous system, and postganglionic components, which are of neural crest origin. Typically, sympathetic neurons are adrenergic, and parasympathetic neurons are cholinergic. The normal choice of transmitter can be overridden, however, by environmental factors so that a sympathetic neuron can secrete acetylcholine.
The spinal cord functions as a pathway for organized tracts of nerve processes and an integration center for local reflexes. During the fetal period, growth in the length of the spinal cord lags behind that of the vertebral column, thus pulling the nerve roots and leaving the end of the spinal cord as a cauda equina.
Within the brain, the myelencephalon retains an organizational similarity to the spinal cord with respect to the tracts passing through, but centers that control respiration and heart rate also form at the site. The metencephalon contains two parts: the pons (which functions principally as a conduit) and the cerebellum (which integrates and coordinates many motor movements and sensory reflexes). In the cerebellum, which forms from the rhombic lips, the gray matter forms on the outside. The ventral part of the mesencephalon is the region through which the major tracts of nerve processes that connect centers in the cerebral cortex with specific sites in the spinal cord pass. The dorsal part of the mesencephalon develops the superior and inferior colliculi, which are involved with the integration of visual and auditory signals.
The diencephalon and the telencephalon represent modified alar plate regions. Many important nuclei and integrating centers develop in the diencephalon, among them the thalamus, hypothalamus, neural hypophysis, and pineal body. The eyes also arise as outgrowths from the diencephalon. In humans, the telencephalon ultimately overgrows other parts of the brain. Similar to the cerebellum, the telencephalon is organized with the gray matter in layers outside the white matter. Neuroblasts migrate through the white matter to these layers by using radial glial cells as their guides.
Within the central nervous system, the central canal expands to form a series of four ventricles in the brain. Specialized vascular plexuses form cerebrospinal fluid, which circulates throughout the central nervous system. Around the brain and spinal cord, two layers of mesenchyme form the meninges.
The cranial nerves are organized on the same fundamental plan as the spinal nerves, but they have lost their regular segmental pattern and have become highly specialized. Some are purely motor, others are purely sensory, and others are mixed.
Many congenital malformations of the nervous system are based on incomplete closure of the neural tube or associated skeletal structures. In the spinal cord, the spectrum of defects ranges from a widely open neural tube (rachischisis) to relatively minor defects in the neural arch over the cord (spina bifida occulta). A similar spectrum of defects is seen in the brain.
Neural function appears in concert with the structural maturation of various components of the nervous system. The first reflex activity is seen in the sixth week. During successive weeks, the reflex movements become more complex, and spontaneous movements appear. Final functional maturation coincides with myelination of the tracts and is not completed until many years after birth.
*The later changes in the central nervous system are so extensive that an exhaustive treatment of even one aspect, such as morphology, is well beyond the scope of this book. This section instead stresses fundamental aspects of the organization of the central nervous system and summarizes the major changes in the organization of the brain and spinal cord.