Chapter 36 Nanomedicine in Ophthalmology
Nanotechnology provides an important new set of tools for the diagnosis and treatment of ocular diseases. Miniaturization of devices, chip-based technologies, and novel nanosized materials and chemical assemblies already provide novel tools that are contributing to improved healthcare in the 21st century and will impinge directly on ophthalmology.1–4 In this chapter, we review general principles of nanotechnology and nanomedicine as well as properties of nanomachines. We also consider specific and potential applications of nanotechnology to ophthalmology, including drug, peptide, and gene delivery; imaging; minimally invasive physiological monitoring; prosthetics; regenerative medicine; and surgical technology. Finally, we consider obstacles to incorporation of nanotechnology into ophthalmology. Each of these topics has been reviewed in detail previously.5–7
General principles of nanotechnology and nanomedicine
Nanotechnology
Regarding information storage, Richard Feynman, who conceived of the field of nanotechnology, calculated that it was possible to write the entire 24 volumes of the Encyclopaedia Britannica on the head of a pin.8 If one did not simply etch the letters on to the surface of the pin but also used the interior of the material also, he calculated that one could fit all the information that humans had accumulated up to December 1959 (estimated at 1015 bits) in a cube of material 1/200 inch wide, comparable to the size of a piece of dust.8 Today, through nanotechnology-based precision assembly of matter, storage densities of 1011 bits per cm2 have been demonstrated, which closely approximates Feynman’s vision.9 Efficient information storage is crucial for the complexity of biological systems, as each eukaryotic cell stores an enormous amount of information. A retinal pigment epithelial (RPE) cell has a diameter of approximately 4 × 10–4 inches (1 × 10–3 cm), and each cell stores the blueprint to create an entire human in DNA molecules (3 billion chemical basepairs, ~25 000 genes).
Regarding computation, Feynman also noted that biological systems do not simply store information, they create measurable outputs. The human brain has the capacity to make judgments, e.g., recognize a person’s face (even if shown at different distances, under different lighting conditions, at different angles), or play chess. Feynman reasoned that if computers could have as many computational elements as our brains, they could make judgments as well.8 Today, sophisticated facial recognition can be accomplished with a powerful laptop computer (versus poorly in 1959, with a much larger computer), due to the development of microprocessors and sophisticated software. Defeating a grand master at chess, however, requires a supercomputer. A Cray XT5 supercomputer uses ~40 kW power/cabinet, and each cabinet measures ~81 × 23 × 57 inches3 (larger than a refrigerator) and weighs ~1530 lb (694 kg) (http://www.cray.com/downloads/CrayXT5Blade.pdf). It is remarkable that the “computer” in our cranium does not require the amount of rare elements, generate the heat, or have the energy requirements of a supercomputer. Thus, the evolution of our cognitive capacities from infancy to adulthood (derived from the interaction between a DNA template-guided, manufactured neuronal network and the external environment) is one demonstration that it is possible to develop nanoscale mechanical systems that create complex, measurable outputs.
Nanomachines are highly efficient.10 When organized in massively parallel structures, for example, nanomotors can generate large forces (e.g., muscles that move massive animals such as whales) or large electrical currents (e.g., those generated by the Hunter’s organ of electric eels). Nanomotors also can direct delicate processes such as ion transport and chromosomal migration during mitosis. Nanomachines are not only highly efficient, they typically have long operational half-lives and are mass-produced easily.
Nanomedicine
The aim of nanomedicine is the comprehensive monitoring, control, construction, repair, defense, and improvement of human biological systems at the molecular level, using engineered nanodevices and nanostructures, operating massively in parallel at the single-cell level, performing “single-cell medicine,” ultimately to achieve medical benefit.11 Integration of nanoscale technologies with the practice of medicine will alter profoundly our approach to the diagnosis, treatment, and prevention of disease.12 We will begin to diagnose and treat diseases at the single-cell level, for example, rather than just at the organ level.
General principles of nanotechnology as applied to nanomedicine include:
1. Biomimicry: the approach that cells use to direct molecules within a cell and/or direct molecules/machines to the proper cells in the body
2. Size and location drive biocompatibility and biological efficacy
3. Engineer feedback control into therapeutic systems (e.g., therapeutic gene synthesis)3,4,13–15
4. Molecules as machines: engineer molecules to perform specific physical tasks, such as opening ion channels, to alter cell and organism behavior16–18
5. “Pseudointelligence” resulting from intelligent design, e.g., self-assembly of extracellular matrix (ECM) molecules15,19–23
6. Highly interdisciplinary undertaking: development of nanotechnologies typically involves expertise in biology, engineering, chemistry, and physics.18,24–26
The functional properties of living systems arise not only from their component parts, but also from how these parts are assembled, which dictates interactions between the parts, the nature and flow of information within the system, and the outputs that the system produces.6 Thus, one concept from biology that may be important for development of nanomachines in medicine is that spatial control of the distribution of nanomachines directly affects the efficiency of the macromolecular assembly and nature of this assembly’s work product.27 Spatial control can be achieved through the use of membranes and anchoring molecules that place enzymes and substrates in proximity (e.g., as occurs in the endoplasmic reticulum for synthesis of ECM proteins, in the mitochondrial membrane for electron transport, and on the cell surface for ECM ligand integrin-mediated changes in intracellular signaling).28 This concept is exploited in the area of neural prosthetics, as described later in this chapter. Conversely, this engineering approach also permits segregation of molecules (e.g., segregation of lysosomal enzymes from the cytoplasm). Microelectromechanical systems (MEMS) or nanoelectromechanical systems (NEMS)-based techniques can be used to create engineered scaffolds (see next paragraph) that achieve this spatial control. One might also use synthesized lipid bilayer membranes, as occurs in nature.
One can produce nanomachines by assembling naturally occurring ones.10,29–32 M/NEMS-based engineering, however, permits the construction of small devices using computer-aided design and by the repeated application of a number of procedures, including oxidation, photolithography, etching, diffusion, sputtering, chemical vapor deposition, ion implantation, and epitaxy, as illustrated by the devices described later in this chapter. Control of features down to the submicron level permits production of mechanical structures at length scales ranging from 100 nm or less to greater than 1 cm. The ability to create complex microfabricated biomaterial substrates using these techniques enables one to define surface microarchitecture, topography, and feature size. By engineering the microenvironment, one can control individual cell responses utilizing structures at the micro- and nanoscale to alter cellular attachment and motility, attenuate the foreign-body response, simulate tissue organization, and promote cell differentiation.33–40
Properties of nanomachines8
Physical properties
Feynman noted that, at the size scale of intracellular structures and molecules, materials acquire seemingly surprising properties that are predictable based on the principles of quantum physics.8 For instance, carbon becomes stronger than steel; gold melts at room temperature, and aluminum becomes highly explosive. Quantities such as weight and inertia are of relatively little importance.
Inhomogeneity of materials (e.g., metals versus plastics) might limit their utility.8 Magnetic properties on a very small scale are not the same as on a large scale. Lubrication is not needed if the machine is small enough because heat loss is rapid (due to the large surface area:volume ratio). Some of these properties produce unexpected results. For example, rapid heat loss might prevent gasoline from exploding, which would make a nanoscale internal combustion engine impossible.8
The influence of gravity on the function of true nanomachines probably is negligible because their mass is that of atoms (Fg = GmAmB/r2).8 On the other hand, the distance between the elements is in nanometers. Because of van der Waals forces, parts of nanomachines might adhere to each other, which might not be desirable. Electrical resistance may be very large with nanocircuits, a feature that might be useful in biological systems. Another problem with nanocircuits is the inverse relationship between the size of the device and the amount of noise generated (Hooge’s rule). The development of stacked graphene sheets may provide a solution to this problem and facilitate the development of circuits much smaller than those in conventional silicone-based computer chips.41 However, when working on the scale of atoms, circuits might not be needed, and quantized energy levels might be manipulated for energy transfer according to the laws of quantum mechanics. This property is exploited in some nanoparticle-based imaging technologies.
Vacancy-engineered mixed-valence state cerium oxide (CeO2) nanoparticles (nanoceria) illustrate the useful properties that materials can develop at the nanoscale. Alteration in the oxidation state of CeO2 creates defects in its lattice structure via loss of oxygen or its electrons. As their size decreases, nanoceria (3–5 nm diameter) demonstrate formation of more oxygen vacancies in the crystal structure.42,43 As described later in this chapter, vacancy-engineered nanoceria may function as a highly effective treatment for ocular conditions associated with oxidative damage.
Manufacture
At each step in the process of manufacturing smaller and smaller machines, one must improve the accuracy of the equipment.8 Feynman speculated that if devices are built on the scale of 5–10 atoms, then it should be possible to mass-produce them such that they are perfect copies of each other.8 One useful outcome of a “nanomanufacturing” process is that the material costs of billions of these machines would be minimal since each is so small that minimal material is used. Nanomachines can have the capacity to repair and build themselves. Indeed, most nanoparticle structures are self-assembling under the proper thermodynamic conditions, allowing for production of a large number of virtually identical nanostructures.
Some properties of nanomachines are illustrated by the design and manufacture of an axon surgery platform. Using microtechnology, electrokinetic axon manipulation (i.e., dielectrophoresis), and cell fusion (i.e., electrofusion), Sretavan et al.24 developed a paradigm of direct axon repair involving the substitution of damaged axon regions with healthy segments from donor axons. This multidisciplinary group developed a multifunctional axon surgery platform that is ~1 mm3 (Figs 36.1 and 36.2). The cutting device consists of a silicon nitride knife with an ultrasharp knife-edge mounted on to a silicon-based compliant knife suspension (Fig. 36.1). The knife edge’s radius of curvature (~20 nm) is similar to the diameter of a single microtubule. Because the knife is manufactured from a silicon nitride membrane, it is nearly transparent, which permits visualization of axons during the cutting procedure. The mechanical compliance of the suspension can be varied to deliver sufficient force for cutting different tissues (e.g., single axons) or for harvesting specific cell populations from histological tissue sections. The authors envision future improvements such as sensors as well as force-generating actuation mechanisms that automatically deliver a controlled cutting stroke, and they indicate that both piezoelectric and thermal expansion actuation mechanisms can deliver forces in the range needed for axon cutting. A femtosecond laser might also be used for axotomy.44 The goal is to develop a microcutting device with on-board sensing and actuation that can function as a semiautonomous instrument, requiring only initiating commands from the surgeon. Important limitations to the practical application of this invention remain. The authors estimate, for example, that ~20 seconds are required to repair a single axon using dielectrophoresis and electrofusion.24 Cutting and fusing multiple axons simultaneously might enable relatively rapid repair of several thousand axons.
Applications to ophthalmology
Nanomedicine will foster revolutionary advances in the diagnosis and treatment of disease. Nanomedicine is likely to have a major impact on biopharmaceuticals (e.g., drug delivery, drug discovery),45 implantable materials (e.g., tissue regeneration scaffolds, bioresorbable materials), implantable devices (e.g., IOP monitors,46 glaucoma drainage valves47), and diagnostic tools (e.g., infectious disease diagnosis, genetic testing, imaging, IOP monitoring). Nanotechnology will bring about the development of regenerative medicine (i.e., replacement and improvement of cells, tissues, and organs), ultrahigh resolution in vivo imaging, microsensors and feedback devices, and artificial vision. “Regenerative nanomedicine,” a new subfield of nanomedicine, uses nanoparticles containing gene transcription factors and other modulating molecules that allow reprogramming of cells in vivo.
Delivery of drugs, peptides, and genes
General considerations regarding nanoparticles
Nanoparticles are colloidal carrier systems that can improve the efficacy of drug delivery by overcoming diffusion barriers, permitting reduced dosing (through more efficient tissue targeting) as well as sustained delivery (Fig. 36.3). These features are attractive for drug treatment of chronic conditions such as glaucoma,48 uveitis,49 or retinal edema (due to venous occlusion or choroidal neovascularization (CNV)) as well as for treatment of intraocular tumors and other conditions associated with cell proliferation such as capsular fibrosis after cataract surgery, ocular neovascularization, and proliferative vitreoretinopathy. Nanoscale-engineered cell substrata (e.g., nanowires) and carbon nanotubes also can be used for gene and drug delivery.50–52
Strategies in the design of nanoparticles for therapeutic purposes have been reviewed thoroughly by Petros and DeSimone.53 Particle size, shape, and surface properties influence nanoparticle biodistribution. Particle size, for example, affects whether the particle is internalized via phagocytosis, macropinocytosis, caveolar-mediated endocytosis, or clathrin-mediated endocytosis, which in turn results in exposure of the nanoparticle to different intracellular environments.54 The cell surface receptor, nucleolin, transports compacted polylysine DNA nanoparticles into cells and directly to the nucleus.55
One can target nanoparticles to specific cells by attaching to the particle surface ligands/antibodies/peptides/aptamers for receptors/molecules that are abundant on the surface of the target cell/tissue.53 This approach can have complications. Receptor aggregation on the cell surface, for example, can induce unintended events, such as apoptosis.56 One can engineer the nanoparticle for a particular mode of intracellular entry depending on the choice of nanoparticle targeting molecules, e.g., cholesterol favors uptake via caveolin-mediated endocytosis, and trans-activating transcriptional activator peptide favors macropinocytosis.57,58 Nanoparticle surface chemistry also can be manipulated to trigger cargo release under specific circumstances. For example, when exposed to a reducing environment such as is present in the cytosol, reductively labile disulfide-based crosslinks between the carrier and cargo are broken.59,60 Approaches for targeting nanoparticles to particular subcellular organelles, e.g., mitochondria61 or the nucleus,62 also have been developed.
Liposomes and polymer–drug conjugates are among the most frequently used nanoparticles for therapeutic purposes. Liposomes, which carry hydrophobic or hydrophilic cargo, can be coated with ligands that direct them to specific cell surface receptors for cell targeting as well as with polymers that prolong their half-life in the circulatory system. Poly(ethylene glycol) (PEG) can be conjugated with different molecules to enhance their solubility and stability in plasma and to reduce immunogenicity.53 Opsonization by immunoglobulin and/or complement proteins can lead to recognition of the nanoparticle as foreign and induce a hypersensitivity reaction.63,64 Coating a nanoparticle with albumin and/or PEG can create a hydrophilic surface that temporarily resists protein adsorption, thus prolonging the particle’s bioavailability.53,65,66 This approach allows for much longer drug circulation and concomitant lowering of therapeutic-level drug doses, which in turn can reduce many unintended side-effects.
Dendrimers are synthetic, highly branched polymers that have precisely controllable nanoscale scaffolding and nanocontainer properties, which in some senses mimic the properties of macromolecules such as DNA and ribonucleic acid (RNA).67 The diameter of poly(amidoamine) dendrimer ranges from 1.5 to 14.5 nm.68 As generation (G) number increases, the number of active terminal groups doubles. G3 dendrimers, for example, contain 32 terminal groups, and G4 dendrimers contain 64 terminal groups. In poly(amidoamine) dendrimers, full generations (e.g., G3) have terminal amine or hydroxyl groups while half-generation dendrimers (e.g., G3.5) have carboxylic acid terminal groups. Dendrimers have been explored as vehicles for controlled drug delivery, including cancer therapy, pilocarpine, gatifloxacin, and for vascular endothelial growth factor (VEGF) inhibition.69–72 Marano et al.,70 for example, used a lipophilic amino acid dendrimer to deliver an anti-VEGF oligonucleotide into rats’ eyes with laser-induced CNV. The dendrimer–oligonucleotide conjugate inhibited CNV development for 4–6 months by up to 95%, whereas eyes injected with oligonucleotide alone showed no treatment benefit compared to vehicle-injected controls at these times. The dendrimer–oligonucleotide conjugate was well tolerated in vivo. Ideta et al.73 used dendrimer porphyrin encapsulated by a polymeric micelle to treat laser-induced CNV in rodents and found significant enhancement of photodynamic therapy efficacy with less light energy required for CNV occlusion.
Antibiotic therapy
Typically, only a small fraction (<5%) of topically administered medications is biologically available due to limited ocular penetration and rapid clearance from the aqueous humor. Because dendrimers contain surface functional groups as well as void spaces within and between their branches, they can serve as delivery vehicles for therapeutic modalities such as carboplatin.72 Dendrimeric polyguanidilyated translocators (DPT) are nano-sized dendrimers that translocate molecules across biological barriers efficiently. Durairaj et al.69 used a six-guanidine group-containing dendrimer to enhance gatifloxacin solubility (fourfold) and delivery to the anterior and posterior segment of rabbits. The DPT–gatifloxacin complexes (346 nm) enhanced tissue concentration in the conjunctiva (13-fold) and cornea (twofold). A single dose resulted in sustained aqueous humor levels (t1/2 = 9 hours), potentially allowing decreased frequency of administration (e.g., once-daily dosing). After multiple dosing, DPT–gatifloxacin achieved therapeutic levels in the vitreous humor for 12 hours (versus no drug levels detectable at 12 hours after topical gatifloxacin alone).
Antimetabolite therapy
Shaunak et al.74 used anionic, polyamidoamine, generation 3.5 dendrimers to make novel water-soluble conjugates of d(+)-glucosamine and d(+)-glucosamine 6-sulfate with immunomodulatory and antiangiogenic properties, respectively. Dendrimer glucosamine inhibited Toll-like receptor 4-mediated lipopolysaccharide-induced synthesis of proinflammatory chemokines (i.e., macrophage inflammatory protein (MIP)-1α, MIP-1β, interleukin (IL)-8) and proinflammatory cytokines (i.e., tumor necrosis factor-α, IL-1β, IL-6) primarily from immature human monocyte-derived dendritic cells and monocyte-derived macrophages, but allowed upregulation of the costimulatory molecules CD25, CD80, CD83, and CD86. Dendrimer glucosamine 6-sulfate blocked fibroblast growth factor-2-mediated human umbilical vein endothelial cell proliferation, but not VEGF-mediated proliferation, and neoangiogenesis in human Matrigel and placental angiogenesis assays. When dendrimer glucosamine and dendrimer glucosamine 6-sulfate were used together in a validated, clinically relevant rabbit model of scar tissue formation after glaucoma filtration surgery,75,76 they increased the long-term success of the surgery from 30% to 80% (P = 0.029). A clinical trial of this modality to reduce scarring after trabeculectomy, however, was not successful (P Khaw, R Ritch, personal communication).
Neurotrophic factor therapy
Nanoparticles can deliver growth and neurotrophic factors to cells.77 Intravitreal nanoparticle-based basic fibroblast growth factor (bFGF) delivery, for example, provides sustained retinal rescue in Royal College of Surgeons (RCS) rats.78 In RCS rats, the RPE cells have a mutation that prevents proper outer-segment phagocytosis, with secondary rod and cone photoreceptor degeneration.79 Some patients with retinitis pigmentosa (RP) have this same mutation.80–82 Sakai et al.78 prepared bFGF nanoparticles using gelatin isolated from bovine bone collagen and human recombinant bFGF. The nanoparticle diameter, assessed using dynamic light scattering, was ~585 nm.
Glaucoma, a leading cause of blindness worldwide, is associated with progressive RGC death and optic nerve atrophy.83 Intravitreal glial cell line-derived neurotrophic factor (GDNF)-loaded biodegradable (poly)lactic-co-glycolic acid (PLGA) microspheres provide sustained RGC protection in a rodent model of glaucoma.84 Microspheres (~8 µm diameter) containing GDNF were fabricated using a modification of a spontaneous emulsion technique.85 Since adeno-associated virus (AAV)-mediated GDNF secretion from glia delays retinal degeneration in a rat model of RP,86 it is possible that nanoparticle-mediated GDNF delivery can be applied to treating RP-like diseases.
Antioxidant therapy
Age-related macular degeneration (AMD), RP, diabetic retinopathy, and retinopathy of prematurity are characterized, in part, by the presence of oxidative damage.87–92 As noted above, alteration in the oxidation state of CeO2 nanoparticles creates defects in its lattice structure via loss of oxygen or its electrons. Chen et al.93 posited that engineered nanoceria can scavenge reactive oxygen intermediates because the large surface area-to-volume ratio at 5 nm diameter enables CeO2 to regenerate its activity and thereby act catalytically. (Unlike nanoceria, most free-radical scavengers require repetitive dosing.) Chen et al.93 showed that intravitreal injection of nanoceria prevents light-induced photoreceptor damage in rodents, even if injected after the initiation of light exposure. Vacancy-engineered nanoceria also inhibit the development of and promote regression of pathological retinal neovascularization in the Vldlr knockout mouse, which carries a loss-of-function mutation in the very low-density lipoprotein receptor gene and whose phenotype resembles a clinical entity known as retinal angiomatous proliferation (Figs 36.4 and 36.5).94,95 This regression occurs even if nanoceria are injected intravitreally after the mutant retinal phenotypes are established (Fig. 36.6). Because nanoceria are a catalytic and regenerative antioxidant, a single injection has a prolonged effect (measured in weeks). Nanoceria inhibition of increased VEGF levels in this model94 may mean that CeO2 nanoparticles will be effective in treating macular edema in diabetic eyes and CNV-induced retinal edema in AMD eyes.96–98
C-60 fullerenes are cage-like structures (truncated icosahedron) of carbon atoms with antioxidant properties.99 Malonic acid C-60 derivatives (carboxyfullerenes) can eliminate superoxide anion and H2O2, and inhibit lipid peroxidation.8 Systemic administration of the C-3 carboxyfullerene isomer delayed motor deterioration and death in a mouse model of familial amyotrophic lateral sclerosis.89,100 It might be useful in the treatment of retinal diseases associated with oxidative damage.
Iron is an essential element for enzymes involved in the phototransduction cascade, in outer-segment disc membrane synthesis, and in the conversion of all-trans-retinyl ester to 11-cis-retinol in the RPE.101–103 Free Fe2+ catalyzes the conversion of hydrogen peroxide to hydroxyl radical, which is a highly reactive species that causes oxidative damage (e.g., lipid peroxidation, DNA strand breaks).104 Increased intracellular iron causes oxidative photoreceptor damage.105 Polymeric nanoparticles can be used to chelate metals. Liu et al.106 showed that a chelator-nanoparticle system complexed with iron, when incubated with human plasma, preferentially adsorbs apolipoprotein E and apolipoprotein A-I, which should facilitate transport into and out of the brain via mechanisms used for transporting low-density lipoprotein. Iron accumulation in the RPE and Bruch’s membrane is greater in AMD eyes than in controls, including cases with early AMD and late stages of the disease (i.e., geographic atrophy, CNV).107 Some of this iron is chelatable.107 Although it is not proven that iron overload is a cause of AMD,108–111 iron chelation might have a therapeutic effect. Thus, the technology developed by Liu et al.106 might have utility for treating AMD eyes.
Immune-suppressive therapy
Cell-based therapy might be sight-restoring for patients with degenerative retinal diseases such as RP and AMD. An immune response to transplanted cells may depend upon the cell types included in the cellular therapy.112 Nanotechnology provides immune-suppressive therapy (local or systemic) in selected cases where its need is anticipated.112–114 In preclinical models, for example, nanoparticles are helpful in managing corneal allograft rejection. Yuan et al.115 manufactured 300-nm-diameter rapamycin-loaded chitosan/polylactic acid nanoparticles and demonstrated that they extended median allograft survival by 17% in rabbits compared with aqueous rapamycin eye drops. Topical chitosan particles were well tolerated in this study, but intraocular chitosan nanoparticles may not be well tolerated.116
Studies of experimental autoimmune uveoretinitis (EAU) demonstrate that nanoparticles can be used to modulate the inflammatory response in the retina and choroid. EAU is a T-cell-mediated autoimmune disease that targets the retina and related tissues and serves as a model for human autoimmune ocular diseases.117 Nanosuspensions of relatively insoluble glucocorticoids (developed using a high-pressure homogenization method) enhance the rate and extent of drug absorption as well as the intensity and duration of drug action, compared with conventional solutions and microcrystalline suspensions.49 Rats with EAU clear poly(lactic acid) (PLA) nanoparticles rapidly from the systemic circulation.118 As noted above, PEG can be used to modify the surface of the nanoparticles, which reduces opsonization and interactions with the mononuclear phagocyte system.119 Sakai et al.120 prepared polymeric nanoparticles with encapsulated betamethasone phosphate. These nanosteroid particles (~120 nm diameter) were composed of PLA homopolymer and a block copolymer of PEG.121 In vivo imaging of inflamed eyes of rats with EAU demonstrated greater nanoparticle accumulation and higher betamethasone concentration in eyes of PLA-PEG nanoparticle-treated rats versus PLA nanoparticle-treated rats. PLA-PEG nanosteroid-treated EAU rats also had lower clinical and histopathological scores for ocular inflammation. The stronger therapeutic effect of PLA-PEG nanosteroids versus PLA nanosteroids may be due to prolonged blood circulation and sustained release in situ as well as due to targeting to inflamed eyes (the latter effect resulting from the small diameter of the nanoparticles).121 EAU also responds very well to intravitreal liposomal tacrolimus (mean diameter = 200 nm) with no side-effects on retinal function or systemic cellular immunity.122
Gene therapy
Nonviral vectors
Viral vectors deliver genes efficiently but can be associated with risks such as immunogenicity and insertional mutagenesis. Nonviral vectors (e.g., polymers, lipids) and other methods (e.g., electroporation, nucleofection) have high gene-carrying capacity, low risk of immunogenicity, relatively low cost, and, possibly, greater ease of production.123 Nanoparticles can deliver genes efficiently to stem cells124 and have been explored as a means for gene delivery in the diagnosis and treatment of ocular disease.3,125–127 As viruses do, nanoparticles can use transactivating sequences that allow them to deploy the host cell machinery to manufacture therapeutic molecules in situ. Because these sequences can contain an upstream biomolecular control sensor, therapeutic molecules can be manufactured in situ under tight feedback control.3,4
Electrostatic interaction of cationic polymers with negatively charged DNA/RNA molecules results in condensation of the material into particles ranging from 8 to 500 nm in diameter, protection of the genes from enzymes, and mediation of cellular entry.26,128 Complexes of cationic polymers and plasmid DNA, termed polyplexes, can have transfection efficiency comparable to adenoviral vectors.129 In addition to nanometer size, polyplexes have large vector capacity, are stable in nuclease-rich environments, and can have relatively high transfectivity for both dividing and nondividing cells.127,129 For example, nanoparticles compacted with a lysine 30-mer linked to 10 kDa PEG-containing cytomegalovirus-cystic fibrosis transmembrane conductance regulator (CMV-CFTR) cDNA were used successfully in a phase I/II clinical trial for the treatment of cystic fibrosis.130 Some particles, however, have low transfection efficiency, and the duration of gene expression can be short. When it occurs, toxicity is related to nanoparticle chemistry.26
To some degree, compacted DNA nanoparticles can be targeted to different tissues in the eye through selection of an appropriate injection site (e.g., intravitreal injection can target the cornea, trabecular meshwork, lens, and inner retina; subretinal injection can target the outer retina and RPE).127 Nanoparticle size and charge influence migration through the vitreous cavity.131 Farjo et al.127 demonstrated that after subretinal injection of compacted lysine 30-mer DNA nanoparticles, gene expression is observed throughout the retina and not just at the site of the injection. By choosing cell-specific promoters, one can achieve additional specificity in the locus of gene expression. The rhodopsin promoter, for example, drives expression in rod photoreceptors, and the human red opsin promoter drives expression in cone photoreceptors.132–134 Interphotoreceptor retinoid-binding protein drives expression in both rods and cones.135 The vitelliform macular dystrophy promoter drives expression in RPE cells.136
Cai et al.132,137 used a specific formulation of DNA nanoparticles consisting of single molecules of DNA compacted with 10 kDa PEG-substituted lysine 30-mer peptides containing the wild-type retinal degeneration slow (Rds) gene, peripherin/rds, to induce cone photoreceptor rescue in an animal model (rds+/–) of RP. After injection into the subretinal space, these particles did not induce a detectable immune response, cytotoxicity, or disruption of retinal function. These compacted plasmid DNA nanoparticles are small (8–20 nm), have rod or ellipsoid shape (depending on the counterion used), and have a large carrying capacity (at least up to 20 kilobases).127,137 PLGA nanoparticles can deliver genes to RPE cells in vitro and in vivo relatively efficiently and safely,138 and PLGA DNA nanoparticles can be associated with long-term gene expression.139 PLGA DNA nanoparticles tend to be larger than polylysine DNA nanoparticles,140,141 which may affect cellular uptake mechanism and delivery to the nucleus. PLGA DNA nanoparticles might be used to deliver therapeutic genes for conditions associated with RPE gene mutations, e.g., Best disease142 and a form of Leber congenital amaurosis.143–145
Albumin has a highly charged amino acid content, which facilitates its action as a carrier for charged drugs and oligonucleotides. Albumin-derived nanoparticles that deliver plasmids containing genes for the Flt receptor (VEGFR1) which binds free VEGF penetrate keratocyte cytoplasm, and provide sustained inhibition of injury-induced corneal neovascularization.146
Despite these promising results, concerns involving nanoparticle use remain. Although the immune response to polylysine-based nanoparticles seems to be less than that for capsid proteins, for example, the efficiency of gene transfer is not as high since most are degraded in the endosomal complexes.147 As a result, one may generate an immune response because one must use large numbers of nanoparticles to achieve therapeutic useful transfection. Also, the apparent low immunogenicity observed in murine models of RP may not be observed in human patients because the immune response to both nanoparticles and viruses varies from one species to another.147
Viral vectors
Critical issues for successful gene therapy include: (1) vector uptake, transport, and uncoating; (2) vector genome persistence; (3) sustained transcriptional expression; (4) the host immune response; and (5) insertional mutagenesis and cancer.147–149 Virus-based gene therapy can induce immune responses, including innate, humoral, and cell-mediated, that are directed against the vector and/or the transgene product.147,150,151 Primary humoral responses directed against the vector can limit its capacity to deliver genes to the target cells as well as the ability to readminister the virus to the patient (e.g., when treating the fellow eye with a second surgical procedure).147 An immediate innate immune response and a secondary antigen-dependent response to intravenous administration of recombinant adenoviral vectors, for example, caused death in a patient with ornithine transcarbamylase deficiency.152,153 A humoral response against the transgene product may neutralize the therapeutic protein.147 A cell-mediated immune response against the vector or transgene product can eliminate the transduced cells.147 Two patients with hemophilia B, for example, developed vector dose-dependent transaminitis that limited hepatocyte-derived factor IX expression to less than 2 months due to CD8+ memory T cells that recognized AAV serotype 2 (AAV2) capsids and eliminated AAV2-transduced hepatocytes.154,155 The innate immune response can cause local and/or systemic toxicity and amplify a secondary antigen-dependent immune response.147 The likelihood of an immune response is influenced by the dose of viral particles,156 which in turn is influenced by the efficiency of vector uptake and gene expression, as well as by the specificity of targeting. If dendritic cells or antigen-presenting cells take up the vector, for example, an immune response is more likely.
Nanoengineering of the viral capsid and transgene may provide a means to solve some of these problems. Recombinant AAVs (rAAVs) have been used successfully to treat preclinical models of human ocular disease and also have been used to treat humans with Leber congenital amaurosis.143,144,157 Modifications of the virus to improve clinical effectiveness illustrate some of the nanoengineering strategies that have been employed in this area. AAVs are small (4.7 kilobase carrying capacity), nonpathogenic, single-stranded DNA parvoviruses that can transduce dividing and nondividing cells.158 The capsid is critical for extracellular events related to the recognition of specific receptors, which influences cell tropism, as well as intracellular processes involving AAV trafficking and uncoating. In turn, the latter processes influence transduction kinetics and transgene expression efficiency.159,160 Due to previous exposure to various AAV serotypes, a significant proportion of the population harbors neutralizing antibodies that can block gene delivery.151,161,162 Because administration of low doses of viral vector might mitigate the severity of this problem, two nanoengineering techniques have been used to improve vector cellular tropism, transduction efficiency, and immunogenicity: directed evolution and site-directed mutagenesis. These are discussed below. Other nanoengineering devices (e.g., DNA transposons,163 bacteriophage recombinases164) may provide clinically useful means to achieve stable, safe DNA integration in the host genome and sustained transgene expression in the future.
Directed evolution of AAV capsids has generated vectors that are highly resistant to neutralizing antibodies.165,166 Maheshri et al.166 used error-prone polymerase chain reaction and a staggered extension process167 to generate an AAV2 library (>106 independent clones) with randomly distributed capsid mutations and then used high-throughput approaches (i.e., exposure of mutants to heparin affinity chromatography (wild-type AAV2 binds to heparan sulfate) or repeated amplification of AAV2 mutants that retain infectivity in the presence of serum containing neutralizing antibodies) to identify mutant AAV2 capsids with altered receptor-binding properties and the capacity to bind with very low affinity to neutralizing antibodies. This approach can be quite powerful. One mutagenesis and three selection steps generated mutant capsids, for example, with a threefold improved neutralizing antibody titer (versus wild-type capsid) and a ~7.5% infectivity at serum levels that completely neutralized wild-type infectivity.166 Directed evolution has been used to generate AAV variants that transduce Müller cells after intravitreal injection,168,169 which may provide a means to deliver growth factors to photoreceptors and RPE cells. These growth factors retard the progression of retinal degeneration in preclinical models of RP86,170,171 and possibly in human patients also.172
Zhong et al.173 demonstrated that site-directed mutagenesis174 of surface-exposed tyrosine residues increases vector transduction efficiency 30-fold in vivo at one log lower vector dose compared to wild-type AAV2. The increased transduction efficiency is due to impaired capsid ubiquitination and improved intracellular trafficking to the nucleus. (Epidermal growth factor receptor protein tyrosine kinase (EGFR-PTK) signaling impairs AAV2 vector transduction by impairing nuclear transport of the vectors175; EGFR-PTK can phosphorylate AAV2 capsids at tyrosine residues,175,176 and tyrosine-phosphorylated AAV2 vectors enter cells efficiently but do not transduce well, in part because the AAV capsids are ubiquitinated and then degraded by the proteasome.175,177) Thus, the T-cell response to AAV2 capsids seems to be manageable by using surface-exposed tyrosine mutant vectors. Another rate-limiting step in transduction efficiency, the conversion of single-stranded viral genome to double-stranded AAV DNA, has been overcome by deleting the terminal resolution site from one rAAV inverted terminal repeat, which prevents replication initiation at the mutated end, to generate self-complementary AAV (scAAV) vectors.178,179 (AAV has a tendency to package DNA dimers when the replicating genome is half the length of the wild type.)
Ocular applications
Due to their relatively low immunogenicity, ability to target many nondividing cells, and capacity for sustained efficient therapeutic gene expression after a single treatment,159 rAAV vectors have been used to treat preclinical models of human retinal disease.180,181 Site-directed mutagenesis technology has been used to improve the treatment of degenerative retinal disease in these preclinical models. Vectors containing point mutations in surface-exposed capsid tyrosine residues in AAV serotypes 2, 8, and 9 display strong and widespread transgene expression in retinal cells after intravitreal or subretinal delivery.182 Petrs-Silva et al.182 demonstrated that tyrosine-to-phenylalanine capsid scAAV2 mutants showed much greater transduction efficiency (10–20-fold higher transgene expression) of the entire retina (including photoreceptors) after intravitreal injection compared to scAAV with wild-type capsids (Fig. 36.7). Mutants of scAAV2, scAAV8, and scAAV9 also enhanced transduction of RGCs compared to wild-type AAV2 (e.g., 106-fold reduction in the number of virus particles needed for RGC transfection with mutant scAAV2 compared to wild-type AAV2). Intravitreal delivery may offer an important clinical advantage over subretinal delivery. Subretinal virus delivery, which has been used in clinical studies,143–145 requires pars plana vitrectomy in the operating room and has a higher likelihood of complications (e.g., retinal tear) than intravitreal delivery, which can be done in an office under topical anesthesia. On the other hand, the subretinal space is a relatively immune-privileged site,183 which may reduce the likelihood of an immune response after repeat virus treatment. Li et al.184 demonstrated that a humoral immune response against AAV2 capsid proteins occurs after intravitreal but not after subretinal vector delivery. Subretinal injection of one of the mutant scAAVs also transduced Müller cells. These studies demonstrate two strategies for reducing the immune response to viral vectors via site-directed mutagenesis: increasing transduction efficiency, which permits lower doses of vector, and creation of multiple effective serotypes, which can be used sequentially for subsequent therapy.
Imaging
The use of nanomaterials for biomedical imaging has been reviewed.26,185,186 In addition to drug delivery, polyamidoamine dendrimer prototypes may be used as targeted diagnostic magnetic resonance imaging (MRI) contrast agents. Gold nanoparticles have been used to enhance tumor identification with computed tomography as well as for colorimetric biosensing. Colorimetric biosensing is based on the fact that the plasmon resonance frequency is a function of the average distance between the gold particles as well as their size, shape, and the dielectric properties of their environment. A plasmon is a quantum of plasma oscillation and can be viewed as the quantization of the oscillation of free electron density against the fixed positive charges in a metal. Gold nanoparticles (5 nm), for example, are orange-red but turn blue-purple when aggregated to larger nanoparticles.186 Colloids of gold nanoparticles impart vibrant colors to the stained-glass windows of some Gothic churches. If a gold nanoparticle–receptor complex is bound to a crosslinking molecule, nanoparticle clustering can occur with an associated colorimetric change.187 Superparamagnetic iron oxide (SPIO) nanoparticles have been approved for use by the US Food and Drug Administration as MRI contrast agents.188 Typically, an SPIO nanoparticle is composed of an iron oxide core coated with dextran, which renders it water-soluble.189 For in vivo long-term imaging, the label is internalized by endocytosis or phagocytosis190 with or without an excipient. These particles can have diameters of 60–150 nm and can be visualized using MRI. SPIO-labeled stem cells have been visualized in patients with brain trauma.191 Limitations of this approach include the dilution of signal associated with cell replication and/or migration.192 Also, no information is provided regarding the state of differentiation of the transplanted cells. Cytotoxicity (e.g., via iron-catalyzed generation of reactive oxygen species) might be a limitation of this approach.26 Quantum dots (Qdots) are light-emitting nanocrystals (2–10 nm) composed of atoms from groups II–VI (e.g., CdSe, ZnSe) or III–V (e.g., InP) of the periodic table.26 In contrast to SPIO nanoparticles, Qdots can be visualized with optical imaging (versus more complex MRI), including optical coherence tomography. In contrast to most organic dyes and fluorescent proteins, Qdots have durable fluorescence intensity, which helps one to distinguish the signal from background autofluorescence, and a broad excitation/narrow emission spectrum, which permits analysis of multiple cell targets with a single excitation wavelength.193 Qdots can be used to monitor survival, distribution, and differentiation of stem cells in vivo.194,195 Limitations can include signal dilution (due to cell proliferation and/or migration) as well as cytotoxicity.26 In some cases, toxicity might arise from oxidative degradation of Qdots with subsequent Cd release and mitochondrial damage.196,197 Coupling SPIO nanoparticles or Qdots to antibodies that recognize molecules such as components of the complement system or molecular constituents associated with AMD-induced changes in Bruch’s membrane198 might provide a means to image the biochemical and/or structural abnormalities associated with AMD.5,199 This capacity may help one assess the effectiveness of AMD treatments that target early molecular changes of the disease.
Nanowires are structures with a diameter of less than 100 nm and indefinite length. Semiconductor nanowires have unique electronic properties and sizes comparable with biological structures involved in cellular communication, thus making them promising nanostructures for establishing active interfaces with biological systems.200 Cells can be grown on nanowire arrays, which can measure electrical functions in different parts of the same cell. Ophthalmic applications might include monitoring RGC survival/physiology in glaucoma patients or photoreceptor/RPE survival/physiology in transplant recipients.5
Minimally invasive physiological monitoring
We have reviewed this issue extensively6 and recapitulate these observations here. Continuous measurement of critical biophysical properties can give insight into disease pathogenesis and the efficacy of a given treatment modality. An assembly of such monitors could be useful for telemedicine and remote patient monitoring, particularly for patients with chronic diseases such as glaucoma (e.g., detect elevated IOP, progressive optic nerve atrophy), diabetic retinopathy (e.g., detect macular edema, retinal neovascularization), and AMD (e.g., detect subretinal fluid/retinal edema associated with CNVs).6 Ideally, the technology would permit repeated (if not continuous) noninvasive monitoring of preselected biomarkers. These platforms should function with minimal power (e.g., rather than incorporating a battery, utilize continuously available power sources) and provide accurate, precise information over an extended period of time (e.g., years). Recall that IOP is a dynamic quantity that fluctuates from moment to moment and diurnally (higher while asleep). It may be that nocturnal IOP (and blood pressure) measurement is more critical to glaucoma management rather than the daytime measurement performed in an outpatient setting.201 When IOP is measured over 24 hours, the results often lead to a change in glaucoma management.201–203 Thus, continuous, minimally invasive IOP measurement could be an important innovation in glaucoma management. Finally, intraocular monitoring devices must be small because there is little unutilized space within the eye.
IOP measurement typically is done with a Goldmann tonometer. This device does not measure IOP directly but instead measures the force required to applanate a corneal surface whose circular area is 7.35 mm2 ([3.06/2]2 π). As a result, changes in corneal thickness as well as changes in corneal tension at different IOPs (Laplace’s law for a thin-walled sphere: T = (P × R)/2 h, where T = tension in the wall, P = pressure difference across the sphere wall, R = sphere radius of curvature, and h = thickness of the wall) can affect the accuracy of IOP measurements done with the Goldmann tonometer.204
A number of nano-based IOP monitoring systems have been developed, but since this chapter is focused on retinal disease, they will only be briefly described. One noninvasive approach to IOP monitoring involves the use of a wireless contact lens.205 Leonardi and coworkers developed a disposable silicone soft contact lens with an embedded sensor.206
An alternative approach that may be better suited for more invasive monitoring relies on capacitive pressure sensors (versus strain gauges).207–209
Chen et al.210 developed a passive, biocompatible, micro-machined pressure sensor, based on the concept of a Bourdon tube (a thin-walled tube with an elliptical cross-sectional shape that can measure pressure quite accurately).
Challenges to the approaches mentioned above include short range (due to low signal-to-noise ratio), limited stability (e.g., due to variable mechanical contact with the cornea), high profile (despite use of MEMS technology), and high manufacturing costs. Rizq et al.211 developed a piezoresistive IOP sensor that is implanted into the suprachoroidal space. The device is micro-machined and is fabricated with associated electronics (interface circuit, radiofrequency powering, and reverse telemetry) with full onboard electronics to provide active readout and superior signal-to-noise ratio, range, and accuracy. This device measured IOP accurately in human cadaver eyes. Dresher and Irazoqui46 designed a compact, ultra-low-power operational amplifier that can be used to record IOP. This CMOS operational amplifier can be incorporated with a wireless IOP monitoring system. It has a power consumption of 736 nW, chip area of 0.023 mm2, and output impedance of 69 Ω to drive low-impedance loads. The authors envision implantation of the device into the suprachoroidal space and have designed and fabricated a high-frequency transmitter integrated circuit that has sufficient signal-to-noise ratio margin for a high data rate transmission wirelessly.212 There is a possibility for choroid/retinal damage with suprachoroidal insertion and a likely need for a method to secure the device (e.g., sutures, glue) to ensure reproducible measurements. In addition it is not clear what the maximum distance between the sensor and the receiver antenna will be, which might place logistical constraints on the use of the device for continuous IOP monitoring.
Coupling diagnostics and therapeutics
Theranostics
Theranostics refers to a process in which diagnosis of a disease state, individualized for a particular patient (even to particular cells within a patient), is coupled with therapy that is targeted precisely in its amount, nature, and location.6 Prow et al.,3 for example, developed a biosensor DNA tethered to a magnetic nanoparticle. The biosensor uses an enhanced green fluorescent protein (EGFP) reporter gene driven by an antioxidant response element (ARE). The ARE is activated by oxidative stress and enhances the expression of genes downstream to it. Exposure of the cells to hyperoxia drives the expression of EFGP. This engineered nanoparticle penetrates endothelial cells (preferentially dividing cells), and after subretinal injection, these biosensor nanoparticles report the activation of the ARE in diabetic rat RPE.213 The antioxidant biosensor could provide a means for clinicians to identify patients likely to need therapy (e.g., babies with retinopathy of prematurity who will need laser photocoagulation or other treatment) at a time before clinical manifestations of severe disease are evident. By coupling a therapeutic gene (e.g., catalase, peroxidase, superoxide dismutase) to the ARE (in addition to a reporter gene such as EGFP), one creates a combined diagnostic–therapeutic device that enables endothelial cells (or any cells that take up the nanoparticle) to “treat themselves” in the setting of oxidative damage.6 Therapeutic possibilities are limited primarily by one’s knowledge of the pathways involved in disease pathogenesis. One could couple genes to manage angiogenesis, for example pigment epithelial-derived factor,214 and to serve as a neurotrophin in eyes with AMD-associated CNV and geographic atrophy. Attractive features of this approach to gene therapy are: (1) one uses the cell’s endogenous metabolic machinery to drive the expression of exogenous genes that will enhance the cell’s capacity to manage environmental stress; and (2) individual cells titer their own therapeutic enzyme/drug concentration in relation to their “toxic” exposure and endogenous capacity to respond to environmental stress. The concept and some experimental results are shown in Fig. 36.8.
Prosthetics: molecules as machines (e.g., light-sensitive ion channels), abiotic–biotic interfaces
Induced photosensitivity
The use of molecules as machines will revolutionize neural prosthetics. The application of this concept to induced photosensitivity has been reviewed in detail elsewhere.6,7 Here we recapitulate this analysis. Although rewiring of inner retinal circuits and inner retinal neuronal degeneration occur in association with photoreceptor degeneration in RP,215,216 it is possible to create visually useful percepts by stimulating RGCs electrically.217–220 Use of light-sensitive ion channels, rather than electrodes, to stimulate RGCs provides an alternative approach to retinal cell stimulation.16–18,221 Induced light sensitivity has the potential for noninvasive neuronal stimulation with high spatial resolution.
Channelopsin-2 is a light-gated ion channel derived from green algae and is sensitive to blue light. When its attached chromophore, all-trans retinaldehyde, undergoes reversible photoisomerization, channelopsin-2 undergoes a conformational change that alters its permeability to mono- and divalent cations.222 In contrast to mammalian rhodopsin, which loses its chromophore after 11-cis retinal-all-trans retinal isomerization, channelopsin-2 remains attached to its chromophore after all-trans to 11-cis retinal isomerization. Thus, there seems to be no need to provide chromophore to the transduced cells. The complex of channelopsin-2 and all-trans retinal is termed channelrhodopsin-2 (ChR2). Using a rAAV delivery system (rAAV serotype-2) in rd1 mice, which have a null mutation in a cyclic GMP phosphodiesterase (PDE6b), and in RCS rats, which have a mutation in the tyrosine kinase, Mertk, ChR2 expression can be achieved in inner retinal neurons (primarily ON and OFF RGCs). Each of the latter mutations causes some form of RP in humans. ChR2 converts these neurons into cells that respond to light with membrane depolarization.223–227 In addition, intraocular injection of rAAV2-ChR2 can restore the ability of the animals to encode light signals in the retina and transmit them to the visual cortex.
Recombinant AAV2 vectors have been used to deliver channelopsin-2 to retinal cells (primarily ganglion and amacrine cells) in wild-type adult mice stably for up to 18 months. Up to 20% of ganglion cells were infected (with normal morphology), and a sufficient number of functional ChR2 channels were maintained to drive robust ganglion cell membrane depolarization and spike firing in response to light.228 These investigators estimated that, at high viral concentrations, approximately 40% of all A-II amacrine cells were labeled. In mammals, rod signals are related through rod bipolar cells to A-II amacrine cells. These signals are coupled on to ON and OFF cone pathways by gap junctions and glycinergic synapses, respectively. Thus, the ability to target A-II amacrine cells with this vector may enable recovery of both ON and OFF light responses in RP retinas.228 Restoration of both ON and OFF pathways probably will be important for achieving good contrast sensitivity and proper spatial and temporal signal processing.229,230 Recombinant AAV2-mediated transfection of retinal neurons in nonhuman primates (marmoset) via intravitreal injection results in functional expression of ChR2 in all retinal neurons, but preferentially ganglion cells (all major types).231 Regional variations in transfection efficiency seemed to correlate with the thickness of the inner limiting membrane. This potential barrier for rAAV2-mediated intravitreal gene delivery could, in principle, be overcome by internal limiting membrane peeling, a standard technique in vitreoretinal surgery, or by enzymatic digestion of the inner limiting membrane.232
Zhang et al.233 showed that expression of halorhodopsin (HaloR), a yellow light-activated chloride ion pump from halobacteria, in inner retinal neurons converts them into OFF cells. In these experiments, HaloR was ~20-fold less sensitive to light than ChR2. HaloR and ChR2 coexpressing cells can produce ON, OFF, and ON-OFF responses, depending on the illumination wavelength.233 Experiments in these preclinical models indicate that kinetics of ChR2- and HaloR-mediated light responses are compatible with temporal processing requirements of visual information in the retina. A current limitation of this approach is that ChR2 and HaloR both exhibit low light sensitivity, with threshold activation light intensities ~5–6 log units higher than those of cones.223,233 Furthermore, the light intensity operating range of microbial rhodopsins is 2–3 log units, compared to normal retinal dynamic range of 10 log units. Doroudchi et al.234 achieved stable, specific expression of ChR2 in ON bipolar cells using a rAAV vector packaged in a tyrosine-mutated capsid. Light levels that elicited visually guided behaviors were within the physiological range of cone photoreceptors. There was no evidence of induced inflammation or toxicity. As indicated above, signal convergence from bipolar cells on to RGCs may mean that targeting ChR2 to rod bipolar cells will provide increased light sensitivity as well as higher spatial resolution, but this approach may be compromised by the alterations in synaptic circuitry that accompany photoreceptor degeneration.215,216,235–237
Greenberg et al.238 reconstructed an excitatory center and antagonistic surround by targeting humanized ChR2 to the somata and enhanced HaloR to the dendrites of RGCs (Figs 36.9 and 36.10). This approach to the deployment of optical neuromodulators retains crucial information processing (edge detection) while being independent of the state of inner retinal circuit remodeling during degeneration. Fusion of the humanized ChR2 to ankyrinG polypeptide localized this opsin to the soma and proximal dendrites because ankryins couple sodium channels to the spectrin-actin network. Fusion of enhanced HaloR to PSD-95 protein targeted this opsin to the dendritic regions in RGCs. As a result, Greenberg and coworkers nanoengineered RGCs with differential spatial and spectral photosensitivity. Depending on which opsin is fused to ankyrinG and which to PDS-95, both ON and OFF-center ganglion cells could be created. Because this approach generated nonphysiological center surround dimensions, Greenberg et al.238 preprocessed the visual image with gaussian blurring, such that when convolved with the dimensions of the soma and dendrites, the gaussians approximated the relative dimensions of the ganglion cells’ center and surround receptive fields. Thus, imaging processing enabled extraction of edge information. These data and the above considerations indicate that ChR2/HaloR-based RGC prosthetics will require image preprocessing to perform light amplification, dynamic range compression, and local gain control operations.238
In typical RP, the rod photoreceptors degenerate first, and cone degeneration follows.216 Even after their outer segments are lost, cone cell bodies remain for a time. Busskamp et al.239 demonstrated that enhanced HaloR expression in light-insensitive cones (via AAV transduction) can restore light sensitivity in mouse models of RP (i.e., the rd1 mouse, which models fast forms of retinal degeneration, and Cnga3–/–; Rho–/– double-knockout, which models a slow form of retinal degeneration). Targeted expression of enhanced HaloR in photoreceptors was achieved using human rhodopsin, human red opsin, and mouse cone arrestin promoters. In rd1 mice, the resensitized cones activate all retinal cone pathways, drive directional selectivity, and activate cortical circuits. In rd1 mice and, to a lesser degree, in Cnga3–/–; Rho–/– mice, the resensitized cones mediate visually guided behaviors. Despite the synaptic reorganization of the inner retina that accompanies RP progression, when stimulated by light, HaloR-transfected photoreceptors seemed to convey information through bipolar cells to RGCs, including both ON and OFF pathways. These effects were obtained even when only ~25% of the cone cell bodies remained.
Allosteric photoswitches provide an alternative approach to using light-sensitive ion channels to stimulate RGCs.16–18,221 To create the photoswitch, ion channels were re-engineered using a light-sensitive azobenzene linker. Short-wavelength light (380 nm) drives the azobenzene moiety into a cis configuration, and longer-wavelength light (500 nm) as well as darkness (azobenzenes thermally isomerize to the lower-energy trans state) drive it into a ~0.7 nm more extended trans configuration. The active moiety can interact with the ion channel in only one of the isomeric states, which leads to a change in ion movement across the cell membrane. Coupling the azobenzene (AZO) moiety to maleimide (MAL) enables the photoswitch to be tethered to the Shaker potassium channel. Coupling the azobenzene moiety to a quaternary ammonium (QA) group enables the MAL-AZO-QA molecule to block the potassium channel when in the trans configuration. To enable modulation of the ionotropic glutamate receptor subtype 6, a cysteine was introduced into the ligand-binding domain of the receptor, enabling the maleimide moiety to tether the photoswitch to the receptor. The quaternary ammonium group was replaced with a glutamate agonist. When in the trans configuration, the agonist is positioned outside the ligand-binding pocket, and when in the cis configuration, the agonist occupies the binding pocket and activates the channel (Fig. 36.11). Acrylamide azobenzene quaternary ammonium, a variant of the MAL-AZO-QA molecule, permits one to affinity label endogenous potassium channels without receptor mutagenesis or genetic manipulation of the target cells (e.g., RGCs).240 A genetically and chemically engineered light-gated mammalian ion channel, the light-activated glutamate receptor (LiGluR), has been expressed selectively in RGCs of the rd1 mouse.241 In this preclinical model of RP, the LiGluR restores light sensitivity to the RGCs, reinstates light responsiveness to the primary visual cortex, and restores both the pupillary reflex and a natural light avoidance behavior. Transducing photoreceptors with ChR2 and HaloR to induce light sensitivity is an example of using molecules to re-engineer cells and their behavior. Here, one is using bionanotechnology to re-engineer proteins first and to re-engineer cell behavior second.7
Rather than modifying the genetic material of host cells to express light-sensitive membrane proteins, Greenbaum and coworkers have used proteoliposomes as vehicles to deliver photoactive transmembrane structures (specifically, the photosystem I (PSI) reaction center, a pigment–protein complex present in the photosynthetic membranes of bacteria, algae, and plants) to mammalian cells.242,243 By placing PSI reaction centers close to voltage-gated ion channels, the light-induced charge separation potential generated by the PSI can trigger a change in membrane potential if the PSI can be packed sufficiently close to the ion channel.244 Preliminary results show that illumination of cells treated with PSI liposomes made with PSI purified from plant leaves results in depolarization (unpublished results, Rohan, Citron, Humayun, and Chow), as well as cytoplasmic calcium elevation. In vivo experiments in blind rats show that, after subretinal injections of PSI liposomes, 33% of rats recovered an optokinetic response to light stimulation (unpublished results, Humayun, Chow, and Greenbaum).
Bionic retina
Subretinal implants that provide precisely patterned electrical stimuli aim to restore vision in patients suffering from retinal degenerative disease. These devices convert a real-time video image of the world into electrical signals that are transmitted to the retina via a microelectrode array. Nanotechology has played an important role in the progress in this field,245–268 and this technology is reviewed in detail in Chapter 126, Artificial vision.
Regenerative medicine: nanostructured scaffolds to control cell phenotype
Regenerative medicine deals with repairing or replacing tissues and organs by using advanced materials and methodologies. Transplantation of cultured autologous limbal stem cells, for example, has permitted recovery of corneal integrity and visual function following chemical corneal injury.269 Regenerative nanomedicine can involve use of nanoparticles containing gene transcription factors and other modulating molecules that direct reprogramming of cells in vivo. Some examples of applications of these techniques have been described earlier, e.g., use of polyplexes to treat RP, restoring light sensitivity by transfecting cones with rAAV-HaloR, and the induction of light-sensitivity in neurons (i.e., ganglion cells) without viral transfection via acrylamide azobenzene quaternary ammonium. A further advance in regenerative ophthalmic medicine would be to replace damaged or dead retinal neurons in patients with chronic retinal detachment, RP, AMD, and allied diseases. Conditions such as retinal detachment, AMD, and RP cause blindness primarily through photoreceptor death. Reactive changes in the synaptic circuitry of second- and third-order neurons also occur,215,270 but we suspect these changes will not decisively limit visual recovery, particularly if photoreceptor replacement can be achieved before atrophy is extensive. Cell-based therapy may be sight-preserving and/or sight-restoring for these patients.271–279 Fetal retina sheet transplants, for example, have been effective in preclinical models.280 Also, retinal progenitor cells and even adult photoreceptors can integrate into host retina and improve some aspects of visual function.281,282 There is evidence, however, that cell isolation and bolus injection are associated with significant cell death.282,283
Strategies for neuronal replacement are nascent. Regeneration of damaged retina occurs readily in fish (from Müller cells) and amphibians (from RPE cells) via endogenous mechanisms.284 Thus, one approach is to identify the genes needed to reprogram remaining neurons in the damaged adult to a more primitive state that enables reconstitution of intact neural tissue. Although mammals may possess a central marginal zone from which retinal progenitor cells can be harvested, this region does not readily support retinal regeneration. Müller cells, however, may be an endogenous source of retinal progenitor cells. Transplantation of cells derived from human embryonic stem cells may provide an alternative means by which to provide sight-restoring therapy to patients with blindness arising from retinal degenerative disease. Retinal cells derived from human embryonic stem cells or induced pluripotent stem cells can be transplanted into mouse retina, differentiate into rod photoreceptors, integrate with the host retina, and, in some cases, mediate visual behavior.271–279 Genes mediating the transformation of retinal progenitors to mature neurons are being identified.284
The ECM may be crucial for successful retinal regeneration. The ECM can alter cell behavior by modulating the expression of genes that promote retinal regeneration. Keratocyte shape, alignment, and migration, for example, are guided by nanotopography.285 These features might be altered to improve biointegration of prosthetic devices such as artificial corneas.285 In addition, RPE survival on submacular Bruch’s membrane from AMD eyes is improved substantially when a provisional, “healthy” ECM is present.286 Stem cells are prevented from exiting the mitotic cycle by environments called niches,287 which comprise cellular and noncellular elements (i.e., ECM components). Physical features of the ECM that influence cell behavior and phenotype include the size, lateral spacing, surface chemistry, and geometry of ECM ligands.288–290
Engineering scaffolds to support cell transplants
Microscale topographical cues can influence retinal291 and neural292 progenitor cell attachment and differentiation independently of biochemical cues. The size, lateral spacing, surface chemistry, and geometry of ECM ligands are physical features of the ECM that influence cell behavior and phenotype.288–290 Scaffolds with the proper nanoscale features might improve transplant efficacy by preventing anoikis (apoptosis due to absence of cell adhesion to the ECM substrate), promoting maintenance of a differentiated phenotype, providing proper three-dimensional organization of the cell–ECM assembly, and promoting a supportive host response to the transplant.293 Nanofiber scaffolds might be used to create niches for stem cell self-renewal or as substrates supporting delivery of sheets of cells.26 Nanofiber scaffolds have a high surface area-to-volume ratio and can present a high density of epitopes to cells, thus promoting neural progenitor cell differentiation.294 These scaffolds can serve not only as a cell delivery platform; they can serve as a temporary ECM that maintains cell survival and differentiation while the transplanted cells elaborate their own ECM and degrade the scaffold. Ellis-Behnke et al.295 reported that a designed self-assembling peptide nanofiber scaffold promoted axonal regeneration through the severed optic tract of hamsters. The regenerated axons reconnected to target tissues and promoted visual recovery.
Scaffolds for cell transplantation to the subretinal space
Hynes and Lavik296 have reviewed the materials, fabrication methods, and results of scaffold-assisted RPE and retinal cell transplantation in detail. Scaffolds can maintain proper three-dimensional organization of tissue (structural support), aid in cell delivery, influence cell behavior (e.g., differentiation), and deliver drugs or trophic molecules.296 Naturally occurring materials (e.g., Descemet’s membrane, lens capsule, Bruch’s membrane, amniotic membrane) can serve as scaffolds, but, as Hynes and Lavik296 point out, variations in material quality, availability, and infectious disease concerns probably supersede their attractive features, including biocompatibility and ease of handling. Naturally occurring polymers, such as collagen and fibrin, have the positive features of naturally occurring membranes and have been used as cell scaffolds, but product consistency, allergic response, and infection risk remain as problems. Use of synthetic polymers enables one to regulate the biological properties (e.g., biodegradability, biocompatibility), mechanical properties (e.g., thickness, deformability), three-dimensional structure (e.g., porosity), and distribution of bioactive molecules (e.g., laminin, GDNF) precisely. Unfortunately, synthetic scaffolds may have undesirable features. For example, although poly(l-lactic acid)/poly(lactic-co-glycolide acid) (PLLA/PLGA) scaffolds improve cell delivery 10-fold, their use can be complicated by inflammation and fibrosis.283,297 While thin (6 µm) and capable of promoting retinal progenitor cell differentiation,25 spin-cast poly(methyl methacrylate) (PMMA) scaffolds are not biodegradable. PMMA scaffolds also require surface modification with either laminin or a combination of laminin and poly-l-lysine for retinal progenitor cells to attach. Poly(ε-caprolactone) (PCL), in contrast, is biodegradable, biocompatible, can be spin-cast to a thin film (5 µm) with controlled microtopography (that favors cell adherence), and promotes the differentiation of retinal progenitor cells.291,298
Most synthetic scaffolds for cell transplantation have been manufactured using techniques adapted from microchip fabrication methods, such as photolithography and soft lithography.296 Microfabrication permits construction of scaffolds with precise architecture25,299,300 (e.g., pore size, to improve cell retention; groove width, to improve cell morphology; and distribution of bioactive molecules,25,291,298 to improve cell attachment and/or differentiation) (Figs 36.12 and 36.13). Tao et al.25 compared adhesion of retinal progenitor cells to polymer, as well as migration and differentiation in the host retina for PMMA laminin-coated scaffolds (6 µm thickness) with either smooth or porous (11 µm diameter) surface topography after transplantation into the subretinal space of C57BL/6 mice. Transplantation using porous scaffolds demonstrated enhanced retinal progenitor cell adherence during transplantation and allowed for greater process outgrowth and cell migration into the host retinal layers whereas transplantation with nonporous scaffolds showed limited retinal progenitor cell retention. A related strategy involves implantation of composite grafts. Redenti et al.298 studied the survival, differentiation, and migration into the retina of mouse retinal progenitor cells cultured on laminin-coated nanowire PCL scaffolds in C57bl/6 and rhodospsin–/– mouse retinal explants and transplant recipients. Retinal progenitor cells were cultured on smooth PCL and both short (2.5 µm) and long (27 µm) nanowire PCL scaffolds. Scaffolds with adherent mouse retinal progenitor cells were then either cocultured with, or transplanted to, wild-type and rhodopsin–/– mouse retina. Robust retinal progenitor cell proliferation on each type of PCL scaffold was observed. Retinal progenitor cells cultured on nanowire scaffolds demonstrated increased expression of mature bipolar and photoreceptor markers. PCL-anchored retinal progenitor cells migrated into the retina of both wild-type and rhodopsin knockout mice. Using microfabrication processes, Sodha et al.299 have manufactured a biodegradable thin-film cell encapsulation scaffold in PCL as a possible cell transplantation vehicle. Utilizing a modified spin-assisted solvent casting and melt templating technique, individual thin-film 2–2.5-D PCL layers (<10 µm) were structured with varying micro- and nanogeometries (protrusions, cavities, pores, particles). Thin-film layers were aligned and thermally bonded to form the three-dimensional cell encapsulation scaffold (<30 µm). These scaffolds promoted retinal progenitor cell retention and were appropriately permeable.
Surgical technology
Albert Hibbs suggested the notion of doing surgery by “swallowing the surgeon.”8 He proposed that this nanosurgeon could travel in blood vessels, identify areas of damaged tissue, and remove or repair them. The nanosurgical ophthalmic operating theater is in its infancy.301–306 The axon surgery platform24 was described earlier in this chapter. While peripheral nerve repair may be its first clinical application, the availability of a microsurgical operating platform may render its introduction into ophthalmology relatively easy. Kim and Lieber307 developed nanotweezers for the manipulation of nanostructures. Electrically conducting, mechanically robust carbon nanotubes were attached to electrodes fabricated on fine-glass micropipettes. The free ends of the nanotubes came into apposition as increasing voltage was applied across the electrodes (Fig. 36.14). Use of these nanotweezers for intraocular surgery would be difficult since the electric current that closes the tweezers might cause tissue coagulation, and the presence of a polar fluid environment might alter the properties of the tweezers. Alternatively, one can use MEMS technology to synthesize micromechanical forceps.308 Robotic surgical manipulators would be needed to perform fine movements with true nanotweezers (humans have minimally a 50-µm tremor amplitude), and imaging systems providing very high magnification would be needed to enable the surgeon to visualize the target tissue and the instruments themselves.5 Nanosurgical devices will enable performance of procedures such as internal recanalization of retinal artery and vein occlusions, dissection of complex epiretinal membranes, repair of retinal breaks (through tissue regeneration), and reanastomosis of severed optic nerves.5
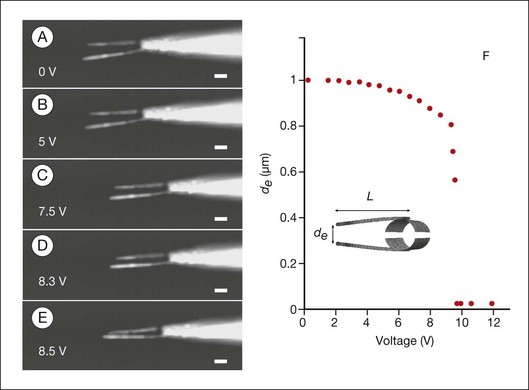
Fig. 36.14 Use of nanotechnology to create nanoinstruments for surgery. The electromechanical response of the nanotube nanotweezers is illustrated. The arms are made of carbon nanotubes, which have great tensile strength and also conduct electricity.307 (A–E) Dark-field optical micrographs of the nanotube arms at potentials ranging from 0 to 8.5 V. Scale bars represent 1 µm. The arms are 50 nm wide and 4 µm long. Increasing deflection of the nanotweezers with increasing voltage is illustrated. (F) Calculated voltage response of the carbon nanotweezers. Separation of the ends of the nanotube arms, de, is plotted as a function of the applied voltage.
(Reproduced from Kim P, Lieber CM. Nanotube nanotweezers. Science 1999;286:2148–21, with permission from the American Association for the Advancement of Science, and from Zarbin MA, Montemagno C, Leary JF, et al. Nanomedicine in ophthalmology: the new frontier. Am J Ophthalmol 2010;150:144–62.)
Obstacles to incorporation of nanotechnology into ophthalmology
Persistence of nanoparticles despite immune surveillance
The biodistribution of nanoparticles and their persistence in tissues and organs are still not well known.309 Locating and visualizing suboptical nanoparticles in large areas of tissues is an extremely difficult task. The ultimate confirmation of nanoparticle presence in organs and tissues is transmission electron microscopy, but it is impossible to scan large areas to find out where to look at this suboptical level.
Unintended biological consequences
A major advantage of nanomedical approaches is that one can minimize unintended biological consequences by using highly targeted nano drug delivery systems. That targeting plus the fact that one to two orders of magnitude smaller amounts of drugs are delivered in vivo greatly reduce the possible unintended consequences and adverse side-effects. Nonetheless, it is difficult to predict the biological response to a given nanomaterial in many cases. Test protocols to assess nanomaterial safety exist,310 but hazards are identified on a case-by-case basis at this time. In general, the toxicity of nanoparticles reflects their chemistry.26 Carbon nanotube toxicity, for example, depends on size, shape, surface coating, and concentration,311 and the nanotubes can be genotoxic.312 A variety of approaches can be taken to address these limitations (summarized by Cai et al.126).
Conclusion
Nanotechnology involves the creation and use of materials and devices at the size scale of intracellular structures and molecules and involves systems and constructs on the order of <100 nm. Nanomedicine involves the comprehensive monitoring, control, construction, repair, defense, and improvement of human biological systems at the molecular level, using engineered nanodevices and nanostructures, operating massively in parallel at the single-cell level, ultimately to achieve medical benefit.11 Individual atoms and molecules can be manipulated to form microscopic tubes, spheres, wires, and films for specific tasks, such as generating electricity or transporting drugs in the body.
The incorporation of nanotechnology in clinical medicine is a translational research endeavor. The earliest impact of nanomedicine is likely to involve the areas of biopharmaceuticals (e.g., drug delivery, drug discovery),45 implantable materials (e.g., tissue regeneration scaffolds, bioresorbable materials), implantable devices (e.g., IOP monitors,46 glaucoma drainage valves47), and diagnostic tools (e.g., genetic testing, imaging, IOP monitoring). Some applications of nanotechnology to ophthalmology are summarized in Table 36.1. Nanotechnology will bring about the development of regenerative medicine (i.e., replacement and improvement of cells, tissues, and organs), ultrahigh resolution in in vivo imaging, microsensors and feedback devices, and artificial vision. As illustrated in this chapter, nanotechnology will play an important role in both early- and late-stage intervention in the management of blinding diseases. “Regenerative nanomedicine,” a new subfield of nanomedicine, uses nanoparticles containing gene transcription factors and other modulating molecules that allow for the reprogramming of cells in vivo. Nanotechnology already has been applied to the measurement and treatment of different disease states in ophthalmology, and many additional innovations will occur during the next century. These discoveries, as they are operationalized from preclinical models to clinical practice, are likely to have a major impact on the development of sight-preserving and sight-restoring treatments for conditions that currently lead to irreversible blindness.
Nanotechnology element | Application | Target disease+/condition |
---|---|---|
Nanoparticles (carbon nanotube, dendrimer, fullerene, nanoceria, liposome, micelle, polypex) |
AMD, age-related macular degeneration; MRI, magnetic resonance imaging; CT, computed tomography; OCT, optical coherence tomography; ROP, retinopathy of prematurity.
(Reproduced with permission from Zarbin MA, Montemagno C, Leary JF, et al. Nanomedicine in ophthalmology: the new frontier. Am J Ophthalmol 2010;150:144–62.)
1 Haglund EM, Seale-Goldsmith M-M, Leary JF. Design of multifunctional nanomedical systems. Ann Biomed Eng. 2009;37:2048–2063.
2 Seale M-M, Leary JF. Nanobiosystems. WIREs Nanomed Nanobiotechnol. 2009;1:553–567.
3 Prow T, Grebe R, Merges C, et al. Nanoparticle tethered antioxidant response element as a biosensor for oxygen induced toxicity in retinal endothelial cells. Mol Vis. 2006;12:616–625.
4 Prow T, Smith JN, Grebe R, et al. Construction, gene delivery, and expression of DNA tethered nanoparticles. Mol Vis. 2006;12:606–615.
5 Zarbin MA, Montemagno C, Leary JF, et al. Nanomedicine in ophthalmology: the new frontier. Am J Ophthalmol. 2010;150:144–162.
6 Zarbin MA, Montemagno C, Leary JF, et al. Nanotechnology in ophthalmology. Can J Ophthalmol. 2010;45:457–476.
7 Zarbin MA, Montemagno C, Leary JF, et al. Regenerative nanomedicine and the treatment of degenerative retinal diseases. Wiley Interdiscip Rev Nanomed Nanobiotechnol. 2012;4:113–137.
8 Feynman R. There’s plenty of room at the bottom. Eng Sci. 1960;23:22–36.
9 Ball P. High-density memory: a switch in time. Nature. 2007;445:362–363.
10 Soong RK, Bachand GD, Neves HP, et al. Powering an inorganic nanodevice with a biomolecular motor. Science. 2000;290:1555–1558.
11 Freitas RA, Jr. What is nanomedicine? Nanomedicine. 2005;1:2–9.
12 Morrow KJ, Jr., Bawa R, Wei C. Recent advances in basic and clinical nanomedicine. Medical Clin North Am. 2007;91:805–843.
13 Prow T, Salazar JH, Rose WA, et al. Nanomedicine – nanoparticles, molecular biosensors and targeted gene/drug delivery for combined single-cell diagnostics and therapeutics. Proc SPIE. 2004;5318:1–11.
14 Prow T, Rose WA, Wang NA, et al. Biosensor-controlled gene therapy/drug delivery with nanoparticles for nanomedicine. Proc SPIE. 2005;5692:199–208.
15 Seale M, Haglund E, Cooper CL, et al. Design of programmable multilayered nanoparticles with in situ manufacture of therapeutic genes for nanomedicine. Proc SPIE. 6430, 2007. 643003–1–7
16 Banghart M, Borges K, Isacoff E, et al. Light-activated ion channels for remote control of neuronal firing. Nat Neurosci. 2004;7:1381–1386.
17 Volgraf M, Gorostiza P, Numano R, et al. Allosteric control of an ionotropic glutamate receptor with an optical switch. Nature Chem Biol. 2006;2:47–52.
18 Szobota S, Gorostiza P, Del Bene F, et al. Remote control of neuronal activity with a light-gated glutamate receptor. Neuron. 2007;54:535–545.
19 Barnes CP, Sell SA, Boland ED, et al. Nanofiber technology: designing the next generation of tissue engineering scaffolds. Adv Drug Deliv Rev. 2007;59:1413–1433.
20 Causa F, Netti PA, Ambrosio L. A multi-functional scaffold for tissue regeneration: The need to engineer a tissue analogue. Biomaterials. 2007;28:5093–5099.
21 Goldberg M, Langer R, Jia X. Nanostructured materials for applications in drug delivery and tissue engineering. J Biomat Sci. 2007;18:241–268.
22 Quondamatteo F. Assembly, stability and integrity of basement membranes in vivo. Histochem J. 2002;34:369–381.
23 Weigel T, Schinkel G, Lendlein A. Design and preparation of polymeric scaffolds for tissue engineering. Exp Rev Med Devices. 2006;3:835–851.
24 Sretavan DW, Chang W, Hawkes E, et al. Microscale surgery on single axons. Neurosurgery. 2005;57:635–646. discussion 646
25 Tao S, Young C, Redenti S, et al. Survival, migration and differentiation of retinal progenitor cells transplanted on micro-machined poly(methyl methacrylate) scaffolds to the subretinal space. Lab on a chip. 2007;7:695–701.
26 Ferreira L, Karp JM, Nobre L, et al. New opportunities: the use of nanotechnologies to manipulate and track stem cells. Cell Stem Cell. 2008;3:136–146.
27 Montemagno CD. Integrative technology for the twenty-first century. Ann N Y Acad Sci. 2004;1013:38–49.
28 Wendell DW, Patti J, Montemagno CD. Using biological inspiration to engineer functional nanostructured materials. Small. 2006;2:1324–1329.
29 Wendell D, Todd J, Montemagno C. Artificial photosynthesis in ranaspumin-2 based foam. Nano Lett. 2010;10:3231–3236.
30 Liu H, Schmidt JJ, Bachand GD, et al. Control of a biomolecular motor-powered nanodevice with an engineered chemical switch. Nat Mater. 2002;1:173–177.
31 Xi J, Schmidt JJ, Montemagno CD. Self-assembled microdevices driven by muscle. Nat Mater. 2005;4:180–184.
32 Bachand GD, Montemagno CD. Constructing organic/inorganic NEMS devices powered by biomolecular motors. Biomed Microdevices. 2000;3:179–184. 2
33 Ainslie KM, Tao SL, Popat KC, et al. In vitro immunogenicity of silicon-based micro- and nanostructured surfaces. ACS Nano. 2008;2:1076–1084.
34 Curtis AS, Gadegaard N, Dalby MJ, et al. Cells react to nanoscale order and symmetry in their surroundings. IEEE Trans Nanobiosci. 2004;3:61–65.
35 Dalby MJ, Riehle MO, Sutherland DS, et al. Changes in fibroblast morphology in response to nano-columns produced by colloidal lithography. Biomaterials. 2004;25:5415–5422.
36 Gallagher JO, McGhee KF, Wilkinson CD, et al. Interaction of animal cells with ordered nanotopography. IEEE Trans Nanobiosci. 2002;1:24–28.
37 Huang NF, Patel S, Thakar RG, et al. Myotube assembly on nanofibrous and micropatterned polymers. Nano Lett. 2006;6:537–542.
38 Kim DHK, Suh KY, Choi SK, et al. Modulation of adhesion and growth of cardiac myocytes by surface nanotopography. 27th Annual International Conference of the Engineering in Medicine and Biology Society. 2005. p. 4091–4
39 Popat KC, Leoni L, Grimes CA, et al. Influence of engineered titania nanotubular surfaces on bone cells. Biomaterials. 2007;28:3188–3197.
40 Yim EK, Reano RM, Pang SW, et al. Nanopattern-induced changes in morphology and motility of smooth muscle cells. Biomaterials. 2005;26:5405–5413.
41 Lin YM, Avouris P. Strong suppression of electrical noise in bilayer graphene nanodevices. Nano Lett. 2008;8:2119–2125.
42 Deshpande S, Patil S, Kuchibhatla SV, et al. Size dependency variation in lattice parameter and valency states in nanocrystalline cerium oxide. Appl Phys Lett. 2005;87:133113.
43 Tsunekawa S, Sahara R, Kawazoe Y, et al. Lattice relaxation of monosize CeO2-x nanocrystalline particles. Appl Surface Sci. 1999;152:53–56.
44 Yanik MF, Cinar H, Cinar HN, et al. Neurosurgery: functional regeneration after laser axotomy. Nature. 2004;432:822.
45 Wei C, Wei W, Morris M, et al. Nanomedicine and drug delivery. Exp Rev Med Devices. 2007;91:863–870.
46 Dresher RP, Irazoqui PP. A compact nanopower low output impedance CMOS operational amplifier for wireless intraocular pressure recordings. Conf Proc IEEE Eng Med Biol Soc. 2007;2007:6056–6059.
47 Pan T, Brown JD, Ziaie B. An artificial nano-drainage implant (ANDI) for glaucoma treatment. Conf Proc IEEE Eng Med Biol Soc. 2006;1:3174–3177.
48 Chu TC, He Q, Potter DE. Biodegradable calcium phosphate nanoparticles as a new vehicle for delivery of a potential ocular hypotensive agent. J Ocul Pharmacol Ther. 2002;18:507–514.
49 Kassem MA, Abdel Rahman AA, Ghorab MM, et al. Nanosuspension as an ophthalmic delivery system for certain glucocorticoid drugs. Int J Pharm. 2007;340:126–133.
50 Kim W, Ng JK, Kunitake ME, et al. Interfacing silicon nanowires with mammalian cells. J Am Chem Soc. 2007;129:7228–7229.
51 Kostarelos K, Lacerda L, Pastorin G, et al. Cellular uptake of functionalized carbon nanotubes is independent of functional group and cell type. Nature nanotechnol. 2007;2:108–113.
52 Bianco A, Kostarelos K, Prato M. Applications of carbon nanotubes in drug delivery. Curr opin chem biol. 2005;9:674–679.
53 Petros RA, DeSimone JM. Strategies in the design of nanoparticles for therapeutic applications. Nature rev. 2010;9:615–627.
54 Rejman J, Oberle V, Zuhorn IS, et al. Size-dependent internalization of particles via the pathways of clathrin- and caveolae-mediated endocytosis. Biochem J. 2004;377:159–169.
55 Chen X, Kube DM, Cooper MJ, et al. Cell surface nucleolin serves as receptor for DNA nanoparticles composed of pegylated polylysine and DNA. Mol Ther. 2008;16:333–342.
56 Renschler MF, Bhatt RR, Dower WJ, et al. Synthetic peptide ligands of the antigen binding receptor induce programmed cell death in a human B-cell lymphoma. Proc Natl Acad Sci U S A. 1994;91:3623–3627.
57 Bareford LM, Swaan PW. Endocytic mechanisms for targeted drug delivery. Adv Drug Deliv Rev. 2007;59:748–758.
58 Torchilin VP. Cell penetrating peptide-modified pharmaceutical nanocarriers for intracellular drug and gene delivery. Biopolymers. 2008;90:604–610.
59 Kirpotin D, Hong K, Mullah N, et al. Liposomes with detachable polymer coating: destabilization and fusion of dioleoylphosphatidylethanolamine vesicles triggered by cleavage of surface-grafted poly(ethylene glycol). FEBS Lett. 1996;388:115–118.
60 Saito G, Swanson JA, Lee KD. Drug delivery strategy utilizing conjugation via reversible disulfide linkages: role and site of cellular reducing activities. Adv Drug Deliv Rev. 2003;55:199–215.
61 Boddapati SV, D’Souza GG, Erdogan S, et al. Organelle-targeted nanocarriers: specific delivery of liposomal ceramide to mitochondria enhances its cytotoxicity in vitro and in vivo. Nano Lett. 2008;8:2559–2563.
62 Wagstaff KM, Jans DA. Importins and beyond: non-conventional nuclear transport mechanisms. Traffic (Copenhagen, Denmark). 2009;10:1188–1198.
63 Moghimi SM, Hamad I, Andresen TL, et al. Methylation of the phosphate oxygen moiety of phospholipid-methoxy(polyethylene glycol) conjugate prevents PEGylated liposome-mediated complement activation and anaphylatoxin production. FASEB J. 2006;20:2591–2593.
64 Hamad I, Christy Hunter A, Rutt KJ, et al. Complement activation by PEGylated single-walled carbon nanotubes is independent of C1q and alternative pathway turnover. Mol Immunol. 2008;45:3797–3803.
65 Yokoe J, Sakuragi S, Yamamoto K, et al. Albumin-conjugated PEG liposome enhances tumor distribution of liposomal doxorubicin in rats. Int J Pharm. 2008;353:28–34.
66 Furumoto K, Yokoe J, Ogawara K, et al. Effect of coupling of albumin on to surface of PEG liposome on its in vivo disposition. Int J Pharm. 2007;329:110–116.
67 Tomalia DA, Reyna LA, Svenson S. Dendrimers as multi-purpose nanodevices for oncology drug delivery and diagnostic imaging. Biochem Soc Trans. 2007;35:61–67.
68 Hahn U, Gorka M, Vogtle F, et al. Light-harvesting dendrimers: efficient intra- and intermolecular energy-transfer processes in a species containing 65 chromophoric groups of four different types. Angew Chem Int Ed Engl. 2002;41:3595–3598. 14
69 Durairaj C, Kadam RS, Chandler JW, et al. Nanosized dendritic polyguanidilyated translocators for enhanced solubility, permeability, and delivery of gatifloxacin. Invest Ophthalmol Vis Sci. 2010;51:5804–5816.
70 Marano RJ, Toth I, Wimmer N, et al. Dendrimer delivery of an anti-VEGF oligonucleotide into the eye: a long-term study into inhibition of laser-induced CNV, distribution, uptake and toxicity. Gene Ther. 2005;12:1544–1550.
71 Vandamme TF, Brobeck L. Poly(amidoamine) dendrimers as ophthalmic vehicles for ocular delivery of pilocarpine nitrate and tropicamide. J Control Release. 2005;102:23–38.
72 Kang SJ, Durairaj C, Kompella UB, et al. Subconjunctival nanoparticle carboplatin in the treatment of murine retinoblastoma. Arch Ophthalmol. 2009;127:1043–1047.
73 Ideta R, Tasaka F, Jang WD, et al. Nanotechnology-based photodynamic therapy for neovascular disease using a supramolecular nanocarrier loaded with a dendritic photosensitizer. Nano Lett. 2005;5:2426–2431.
74 Shaunak S, Thomas S, Gianasi E, et al. Polyvalent dendrimer glucosamine conjugates prevent scar tissue formation. Nat Biotechnol. 2004;22:977–984.
75 Mead AL, Wong TT, Cordeiro MF, et al. Evaluation of anti-TGF-beta2 antibody as a new postoperative anti-scarring agent in glaucoma surgery. Invest Ophthalmol Vis Sci. 2003;44:3394–3401.
76 Siriwardena D, Khaw PT, King AJ, et al. Human antitransforming growth factor beta(2) monoclonal antibody – a new modulator of wound healing in trabeculectomy: a randomized placebo controlled clinical study. Ophthalmology. 2002;109:427–431.
77 Ferreira L, Park H, Choe H, et al. Human embryoid bodies containing nano- and micro-particulate delivery vehicles. Adv Mat. 2008;20:2285–2291.
78 Sakai T, Kuno N, Takamatsu F, et al. Prolonged protective effect of basic fibroblast growth factor-impregnated nanoparticles in Royal College of Surgeons rats. Invest Ophthalmol Vis Sci. 2007;48:3381–3387.
79 Vollrath D, Feng W, Duncan JL, et al. Correction of the retinal dystrophy phenotype of the RCS rat by viral gene transfer of Mertk. Proc Natl Acad Sci U S A. 2001;98:12584–12589.
80 Gal A, Li Y, Thompson DA, et al. Mutations in MERTK, the human orthologue of the RCS rat retinal dystrophy gene, cause retinitis pigmentosa. Nat Genet. 2000;26:270–271.
81 Charbel Issa P, Bolz HJ, Ebermann I, et al. Characterisation of severe rod-cone dystrophy in a consanguineous family with a splice site mutation in the MERTK gene. Br J Ophthalmol. 2009;93:920–925.
82 Mackay DS, Henderson RH, Sergouniotis PI, et al. Novel mutations in MERTK associated with childhood onset rod-cone dystrophy. Mol Vis. 2010;16:369–377.
83 Quigley HA, Broman AT. The number of people with glaucoma worldwide in 2010 and 2020. Br J Ophthalmol. 2006;90:262–267.
84 Jiang C, Moore MJ, Zhang X, et al. Intravitreal injections of GDNF-loaded biodegradable microspheres are neuroprotective in a rat model of glaucoma. Mol Vis. 2007;13:1783–1792.
85 Fu K, Harrell R, Zinski K, et al. A potential approach for decreasing the burst effect of protein from PLGA microspheres. J Pharm Sci. 2003;92:1582–1591.
86 Dalkara D, Kolstad KD, Guerin KI, et al. AAV mediated GDNF secretion from retinal glia slows down retinal degeneration in a rat model of retinitis pigmentosa. Mol Ther. 2011;19:1602–1608.
87 Brownlee M. A radical explanation for glucose-induced beta cell dysfunction. J Clin Invest. 2003;112:1788–1790.
88 Yorek MA. The role of oxidative stress in diabetic vascular and neural disease. Free Radic Res. 2003;37:471–480.
89 Dugan LL, Lovett EG, Quick KL, et al. Fullerene-based antioxidants and neurodegenerative disorders. Parkinsonism Relat Disord. 2001;7:243–246.
90 Shen JK, Dong A, Hackett SF, et al. Oxidative damage in age-related macular degeneration. Histol Histopathol. 2007;22:1301–1308.
91 Komeima K, Rogers BS, Campochiaro PA. Antioxidants slow photoreceptor cell death in mouse models of retinitis pigmentosa. J Cell Physiol. 2007;213:809–815.
92 Papp A, Nemeth I, Karg E, et al. Glutathione status in retinopathy of prematurity. Free Radic Biol Med. 1999;27:738–743.
93 Chen J, Patil S, Seal S, et al. Rare earth nanoparticles prevent retinal degeneration induced by intracellular peroxides. Nat Nanotechnol. 2006;1:142–150.
94 Zhou X, Wong LL, Karakoti AS, et al. Nanoceria inhibit the development and promote the regression of pathologic retinal neovascularization in the Vldlr knockout mouse. PLoS ONE. 2011;6:e16733.
95 Truong SN, Alam S, Zawadzki RJ, et al. High resolution Fourier-domain optical coherence tomography of retinal angiomatous proliferation. Retina. 2007;27:915–925.
96 Elman MJ, Aiello LP, Beck RW, et al. Randomized trial evaluating ranibizumab plus prompt or deferred laser or triamcinolone plus prompt laser for diabetic macular edema. Ophthalmology. 2010;117:1064–1077. e35
97 Rosenfeld PJ, Brown DM, Heier JS, et al. Ranibizumab for neovascular age-related macular degeneration. N Engl J Med. 2006;355:1419–1431.
98 Brown DM, Kaiser PK, Michels M, et al. Ranibizumab versus verteporfin for neovascular age-related macular degeneration. N Engl J Med. 2006;355:1432–1444.
99 Kroto HW, Heath JR, O’Brien SC, et al. C60: Buckminsterfullerene. Nature. 1985;318:162–163.
100 Dugan LL, Turetsky DM, Du C, et al. Carboxyfullerenes as neuroprotective agents. Proc Natl Acad Sci U S A. 1997;94:9434–9439.
101 Shichi H. Microsomal electron transfer system of bovine retinal pigment epithelium. Exp Eye Res. 1969;8:60–68.
102 Yau KW, Baylor DA. Cyclic GMP-activated conductance of retinal photoreceptor cells. Annu Rev Neurosci. 1989;12:289–327.
103 Moiseyev G, Chen Y, Takahashi Y, et al. RPE65 is the isomerohydrolase in the retinoid visual cycle. Proc Natl Acad Sci U S A. 2005;102:12413–12418.
104 Halliwell B, Gutteridge JM. Oxygen toxicity, oxygen radicals, transition metals and disease. Biochem J. 1984;219:1–14.
105 Rogers BS, Symons RC, Komeima K, et al. Differential sensitivity of cones to iron-mediated oxidative damage. Invest Ophthalmol Vis Sci. 2007;48:438–445.
106 Liu G, Men P, Harris PL, et al. Nanoparticle iron chelators: a new therapeutic approach in Alzheimer disease and other neurologic disorders associated with trace metal imbalance. Neurosci Lett. 2006;406:189–193.
107 Hahn P, Milam AH, Dunaief JL. Maculas affected by age-related macular degeneration contain increased chelatable iron in the retinal pigment epithelium and Bruch’s membrane. Arch Ophthalmol. 2003;121:1099–1105.
108 Hahn P, Qian Y, Dentchev T, et al. Disruption of ceruloplasmin and hephaestin in mice causes retinal iron overload and retinal degeneration with features of age-related macular degeneration. Proc Natl Acad Sci U S A. 2004;101:13850–13855.
109 Dunaief JL, Richa C, Franks EP, et al. Macular degeneration in a patient with aceruloplasminemia, a disease associated with retinal iron overload. Ophthalmology. 2005;112:1062–1065.
110 Chowers I, Wong R, Dentchev T, et al. The iron carrier transferrin is upregulated in retinas from patients with age-related macular degeneration. Invest Ophthalmol Vis Sci. 2006;47:2135–2140.
111 He X, Hahn P, Iacovelli J, et al. Iron homeostasis and toxicity in retinal degeneration. Prog Retin Eye Res. 2007;26:649–673.
112 Gullapalli VKKM, Wang H, Sugino IK, et al. Retinal pigment epithelium and photoreceptor transplantation frontiers. In: Ryan SJ, ed. Retina. 4th ed. Philadelphia, PA: Mosby; 2006:2597–2613.
113 Zarbin MA. Functionalizing cell-based therapy for age-related macular degeneration. Am J Ophthalmol. 2007;143:681–682.
114 Zarbin MA. Retinal pigment epithelium-retina transplantation for retinal degenerative disease. Am J Ophthalmol. 2008;146:151–153.
115 Yuan XB, Yuan YB, Jiang W, et al. Preparation of rapamycin-loaded chitosan/PLA nanoparticles for immunosuppression in corneal transplantation. Int J Pharm. 2008;349:241–248.
116 Prow TW, Bhutto I, Kim SY, et al. Ocular nanoparticle toxicity and transfection of the retina and retinal pigment epithelium. Nanomedicine. 2008;4:340–349.
117 Caspi RR. Experimental autoimmune uveoretinitis in the rat and mouse. Curr Protoc Immunol. 2003. Chapter 15:Unit 15 6
118 Sakai T, Kohno H, Ishihara T, et al. Treatment of experimental autoimmune uveoretinitis with poly(lactic acid) nanoparticles encapsulating betamethasone phosphate. Exp Eye Res. 2006;82:657–663.
119 Bazile D, Prud’homme C, Bassoullet MT, et al. Stealth Me.PEG-PLA nanoparticles avoid uptake by the mononuclear phagocytes system. J Pharm Sci. 1995;84:493–498.
120 Ishihara T, Kubota T, Choi T, et al. Polymeric nanoparticles encapsulating betamethasone phosphate with different release profiles and stealthiness. Int J Pharm. 2009;375:148–154.
121 Sakai T, Ishihara T, Higaki M, et al. Therapeutic effect of stealth-type polymeric nanoparticles with encapsulated betamethasone phosphate on experimental autoimmune uveoretinitis. Invest Ophthalmol Vis Sci. 2011;52:1516–1521.
122 Zhang R, He R, Qian J, et al. Treatment of experimental autoimmune uveoretinitis with intravitreal injection of tacrolimus (FK506) encapsulated in liposomes. Invest Ophthalmol Vis Sci. 2010;51:3575–3582.
123 Glover DJ, Lipps HJ, Jans DA. Towards safe, non-viral therapeutic gene expression in humans. Nat Rev Genet. 2005;6:299–310.
124 Kutsuzawa K, Chowdhury EH, Nagaoka M, et al. Surface functionalization of inorganic nano-crystals with fibronectin and E-cadherin chimera synergistically accelerates trans-gene delivery into embryonic stem cells. Biochem Biophys Res Commun. 2006;350:514–520.
125 Mo Y, Barnett ME, Takemoto D, et al. Human serum albumin nanoparticles for efficient delivery of Cu, Zn superoxide dismutase gene. Mol Vis. 2007;13:746–757.
126 Cai X, Conley S, Naash M. Nanoparticle applications in ocular gene therapy. Vision Res. 2008;48:319–324.
127 Farjo R, Skaggs J, Quiambao AB, et al. Efficient non-viral ocular gene transfer with compacted DNA nanoparticles. PLoS ONE. 2006;1:e38.
128 Pack DW, Hoffman AS, Pun S, et al. Design and development of polymers for gene delivery. Nat Rev. 2005;4:581–593.
129 Incani V, Tunis E, Clements BA, et al. Palmitic acid substitution on cationic polymers for effective delivery of plasmid DNA to bone marrow stromal cells. J Biomed Mater Res A. 2007;81:493–504.
130 Konstan MW, Davis PB, Wagener JS, et al. Compacted DNA nanoparticles administered to the nasal mucosa of cystic fibrosis subjects are safe and demonstrate partial to complete cystic fibrosis transmembrane regulator reconstitution. Hum Gene Ther. 2004;15:1255–1269.
131 Pitkanen L, Ruponen M, Nieminen J, et al. Vitreous is a barrier in nonviral gene transfer by cationic lipids and polymers. Pharm Res. 2003;20:576–583.
132 Cai X, Conley SM, Nash Z, et al. Gene delivery to mitotic and postmitotic photoreceptors via compacted DNA nanoparticles results in improved phenotype in a mouse model of retinitis pigmentosa. FASEB J. 2010;24:1178–1191.
133 Flannery JGZS, Vaquero MI, LaVail MM, et al. Efficient photoreceptor-targeted gene expression in vivo by recombinant adeno-associated virus. Proc Natl Acad Sci U S A. 1997;94:6916–6921.
134 Li Q, Timmers AM, Guy J, et al. Cone-specific expression using a human red opsin promoter in recombinant AAV. Vision Res. 2008;48:332–338.
135 Porrello K, Bhat SP, Bok D. Detection of interphotoreceptor retinoid binding protein (IRBP) mRNA in human and cone-dominant squirrel retinas by in situ hybridization. J Histochem Cytochem. 1991;39:171–176.
136 Esumi N, Oshima Y, Li Y, et al. Analysis of the VMD2 promoter and implication of E-box binding factors in its regulation. J Biol Chem. 2004;279:19064–19073.
137 Cai X, Nash Z, Conley SM, et al. A partial structural and functional rescue of a retinitis pigmentosa model with compacted DNA nanoparticles. PLoS ONE. 2009;4:e5290.
138 Bejjani RA, BenEzra D, Cohen H, et al. Nanoparticles for gene delivery to retinal pigment epithelial cells. Mol Vis. 2005;11:124–132.
139 Conley SM, Cai X, Naash MI. Nonviral ocular gene therapy: assessment and future directions. Curr Opin Mol Ther. 2008;10:456–463.
140 Conley SM, Naash MI. Nanoparticles for retinal gene therapy. Prog Retin Eye Res. 2010;29:376–397.
141 Ravi Kumar MN, Bakowsky U, Lehr CM. Preparation and characterization of cationic PLGA nanospheres as DNA carriers. Biomaterials. 2004;25:1771–1777.
142 Marmorstein AD, Marmorstein LY, Rayborn M, et al. Bestrophin, the product of the Best vitelliform macular dystrophy gene (VMD2), localizes to the basolateral plasma membrane of the retinal pigment epithelium. Proc Natl Acad Sci U S A. 2000;97:12758–12763.
143 Maguire AM, Simonelli F, Pierce EA, et al. Safety and efficacy of gene transfer for Leber’s congenital amaurosis. N Engl J Med. 2008;358:2240–2248.
144 Bainbridge JW, Smith AJ, Barker SS, et al. Effect of gene therapy on visual function in Leber’s congenital amaurosis. N Engl J Med. 2008;358:2231–2239.
145 Cideciyan AV, Hauswirth WW, Aleman TS, et al. Human RPE65 gene therapy for Leber congenital amaurosis: persistence of early visual improvements and safety at 1 year. Hum Gene Ther. 2009;20:999–1004.
146 Jani PD, Singh N, Jenkins C, et al. Nanoparticles sustain expression of Flt intraceptors in the cornea and inhibit injury-induced corneal angiogenesis. Invest Ophthalmol Vis Sci. 2007;48:2030–2036.
147 Kay MA. State-of-the-art gene-based therapies: the road ahead. Nat Rev Genet. 2011;12:316–328.
148 Donsante A, Miller DG, Li Y, et al. AAV vector integration sites in mouse hepatocellular carcinoma. Science. 2007;317:477.
149 Hacein-Bey-Abina S, Von Kalle C, Schmidt M, et al. LMO2-associated clonal T cell proliferation in two patients after gene therapy for SCID-X1. Science. 2003;302:415–419.
150 Boutin S, Monteilhet V, Veron P, et al. Prevalence of serum IgG and neutralizing factors against adeno-associated virus (AAV) types 1, 2, 5, 6, 8, and 9 in the healthy population: implications for gene therapy using AAV vectors. Hum Gene Ther. 2010;21:704–712.
151 Erles K, Sebokova P, Schlehofer JR. Update on the prevalence of serum antibodies (IgG and IgM) to adeno-associated virus (AAV). J Med Virol. 1999;59:406–411.
152 Raper SE, Chirmule N, Lee FS, et al. Fatal systemic inflammatory response syndrome in a ornithine transcarbamylase deficient patient following adenoviral gene transfer. Mol Genet Metab. 2003;80:148–158.
153 Zaiss AK, Machado HB, Herschman HR. The influence of innate and pre-existing immunity on adenovirus therapy. J Cell Biochem. 2009;108:778–790.
154 Manno CS, Pierce GF, Arruda VR, et al. Successful transduction of liver in hemophilia by AAV-factor IX and limitations imposed by the host immune response. Nat Med. 2006;12:342–347.
155 Mingozzi F, Maus MV, Hui DJ, et al. CD8(+) T-cell responses to adeno-associated virus capsid in humans. Nat Med. 2007;13:419–422.
156 Petry H, Brooks A, Orme A, et al. Effect of viral dose on neutralizing antibody response and transgene expression after AAV1 vector re-administration in mice. Gene Ther. 2008;15:54–60.
157 Hauswirth WW, Aleman TS, Kaushal S, et al. Treatment of Leber congenital amaurosis due to RPE65 mutations by ocular subretinal injection of adeno-associated virus gene vector: short-term results of a phase I trial. Hum Gene Ther. 2008;19:979–990.
158 Goncalves MA. Adeno-associated virus: from defective virus to effective vector. Virol J. 2005;2:43.
159 Surace EM, Auricchio A. Versatility of AAV vectors for retinal gene transfer. Vision Res. 2008;48:353–359.
160 Yang GS, Schmidt M, Yan Z, et al. Virus-mediated transduction of murine retina with adeno-associated virus: effects of viral capsid and genome size. J Virol. 2002;76:7651–7660.
161 Zaiss AK, Muruve DA. Immunity to adeno-associated virus vectors in animals and humans: a continued challenge. Gene Ther. 2008;15:808–816.
162 Sun JY, Anand-Jawa V, Chatterjee S, et al. Immune responses to adeno-associated virus and its recombinant vectors. Gene Ther. 2003;10:964–976.
163 Ivics Z, Izsvak Z. The expanding universe of transposon technologies for gene and cell engineering. Mob DNA. 2010;1:25.
164 Calos MP. The phiC31 integrase system for gene therapy. Curr Gene Ther. 2006;6:633–645.
165 Kwon I, Schaffer DV. Designer gene delivery vectors: molecular engineering and evolution of adeno-associated viral vectors for enhanced gene transfer. Pharm Res. 2008;25:489–499.
166 Maheshri N, Koerber JT, Kaspar BK, et al. Directed evolution of adeno-associated virus yields enhanced gene delivery vectors. Nat Biotechnol. 2006;24:198–204.
167 Zhao H, Giver L, Shao Z, et al. Molecular evolution by staggered extension process (StEP) in vitro recombination. Nat Biotechnol. 1998;16:258–261.
168 Koerber JT, Klimczak R, Jang JH, et al. Molecular evolution of adeno-associated virus for enhanced glial gene delivery. Mol Ther. 2009;17:2088–2095.
169 Klimczak RR, Koerber JT, Dalkara D, et al. A novel adeno-associated viral variant for efficient and selective intravitreal transduction of rat Müller cells. PLoS ONE. 2009;4:e7467.
170 LaVail MM, Yasumura D, Matthes MT, et al. Protection of mouse photoreceptors by survival factors in retinal degenerations. Invest Ophthalmol Vis Sci. 1998;39:592–602.
171 Chaum E. Retinal neuroprotection by growth factors: a mechanistic perspective. J Cell Biochem. 2003;88:57–75.
172 Sieving PA, Caruso RC, Tao W, et al. Ciliary neurotrophic factor (CNTF) for human retinal degeneration: phase I trial of CNTF delivered by encapsulated cell intraocular implants. Proc Natl Acad Sci U S A. 2006;103:3896–3901.
173 Zhong L, Li B, Mah CS, et al. Next generation of adeno-associated virus 2 vectors: point mutations in tyrosines lead to high-efficiency transduction at lower doses. Proc Natl Acad Sci U S A. 2008;105:7827–7832.
174 Wang W, Malcolm BA. Two-stage PCR protocol allowing introduction of multiple mutations, deletions and insertions using QuikChange site-directed mutagenesis. Biotechniques. 1999;26:680–682.
175 Zhong L, Zhao W, Wu J, et al. A dual role of EGFR protein tyrosine kinase signaling in ubiquitination of AAV2 capsids and viral second-strand DNA synthesis. Mol Ther. 2007;15:1323–1330.
176 Mah C, Qing K, Khuntirat B, et al. Adeno-associated virus type 2-mediated gene transfer: role of epidermal growth factor receptor protein tyrosine kinase in transgene expression. J Virol. 1998;72:9835–9843.
177 Zhong L, Zhou X, Li Y, et al. Single-polarity recombinant adeno-associated virus 2 vector-mediated transgene expression in vitro and in vivo: mechanism of transduction. Mol Ther. 2008;16:290–295.
178 McCarty DM, Fu H, Monahan PE, et al. Adeno-associated virus terminal repeat (TR) mutant generates self-complementary vectors to overcome the rate-limiting step to transduction in vivo. Gene Ther. 2003;10:2112–2118.
179 Yokoi K, Kachi S, Zhang HS, et al. Ocular gene transfer with self-complementary AAV vectors. Invest Ophthalmol Vis Sci. 2007;48:3324–3328.
180 Acland GM, Aguirre GD, Bennett J, et al. Long-term restoration of rod and cone vision by single dose rAAV-mediated gene transfer to the retina in a canine model of childhood blindness. Mol Ther. 2005;12:1072–1082.
181 Min SH, Molday LL, Seeliger MW, et al. Prolonged recovery of retinal structure/function after gene therapy in an Rs1h-deficient mouse model of X-linked juvenile retinoschisis. Mol Ther. 2005;12:644–651.
182 Petrs-Silva H, Dinculescu A, Li Q, et al. High-efficiency transduction of the mouse retina by tyrosine-mutant AAV serotype vectors. Mol Ther. 2009;17:463–471.
183 Streilein JW. Limitations in the study of immune privilege in the subretinal space of the rodent. Invest Ophthalmol Vis Sci. 1999;40:3069.
184 Li Q, Miller R, Han PY, et al. Intraocular route of AAV2 vector administration defines humoral immune response and therapeutic potential. Mol Vis. 2008;14:1760–1769.
185 Nune SK, Gunda P, Thallapally PK, et al. Nanoparticles for biomedical imaging. Exp Opin Drug Deliv. 2009;6:1175–1194.
186 Boisselier E, Astruc D. Gold nanoparticles in nanomedicine: preparations, imaging, diagnostics, therapies and toxicity. Chem Soc Rev. 2009;38:1759–1782.
187 Elghanian R, Storhoff JJ, Mucic RC, et al. Selective colorimetric detection of polynucleotides based on the distance-dependent optical properties of gold nanoparticles. Science. 1997;277:1078–1081.
188 Reimer P, Balzer T. Ferucarbotran (Resovist): a new clinically approved RES-specific contrast agent for contrast-enhanced MRI of the liver: properties, clinical development, and applications. Eur Radiol. 2003;13:1266–1276.
189 Wang YX, Hussain SM, Krestin GP. Superparamagnetic iron oxide contrast agents: physicochemical characteristics and applications in MR imaging. Eur Radiol. 2001;11:2319–2331.
190 Hsiao JK, Tai MF, Chu HH, et al. Magnetic nanoparticle labeling of mesenchymal stem cells without transfection agent: cellular behavior and capability of detection with clinical 1.5 T magnetic resonance at the single cell level. Magn Reson Med. 2007;58:717–724.
191 Zhu J, Zhou L, XingWu F. Tracking neural stem cells in patients with brain trauma. N Engl J Med. 2006;355:2376–2378.
192 Guzman R, Uchida N, Bliss TM, et al. Long-term monitoring of transplanted human neural stem cells in developmental and pathological contexts with MRI. Proc Natl Acad Sci U S A. 2007;104:10211–10216.
193 Michalet X, Pinaud FF, Bentolila LA, et al. Quantum dots for live cells, in vivo imaging, and diagnostics. Science. 2005;307:538–544.
194 Lei Y, Tang H, Yao L, et al. Applications of mesenchymal stem cells labeled with Tat peptide conjugated quantum dots to cell tracking in mouse body. Bioconjugate Chem. 2008;19:421–427.
195 Slotkin JR, Chakrabarti L, Dai HN, et al. In vivo quantum dot labeling of mammalian stem and progenitor cells. Dev Dyn. 2007;236:3393–3401.
196 Derfus AM, Chan WCW, Bhatia SN. Probing the cytotoxicity of semiconductor quantum dots. Adv Mat. 2004;4:11–18.
197 Lovric J, Cho SJ, Winnik FM, et al. Unmodified cadmium telluride quantum dots induce reactive oxygen species formation leading to multiple organelle damage and cell death. Chem Biol. 2005;12:1227–1234.
198 Zarbin MA. Current concepts in the pathogenesis of age-related macular degeneration. Arch Ophthalmol. 2004;122:598–614.
199 Takeda A, Baffi JZ, Kleinman ME, et al. CCR3 is a target for age-related macular degeneration diagnosis and therapy. Nature. 2009;460:225–230.
200 Cohen-Karni T, Timko BP, Weiss LE, et al. Flexible electrical recording from cells using nanowire transistor arrays. Proc Natl Acad Sci U S A. 2009;106:7309–7313.
201 Graham SL, Drance SM. Nocturnal hypotension: role in glaucoma progression. Surv Ophthalmol. 1999;43(Suppl 1):S10–S16.
202 Barkana Y, Anis S, Liebmann J, et al. Clinical utility of intraocular pressure monitoring outside of normal office hours in patients with glaucoma. Arch Ophthalmol. 2006;124:793–797.
203 Wax MB, Camras CB, Fiscella RG, et al. Emerging perspectives in glaucoma: optimizing 24-hour control of intraocular pressure. Am J Ophthalmol. 2002;133 Suppl:S1–10.
204 Lee GA, Khaw PT, Ficker LA, et al. The corneal thickness and intraocular pressure story: where are we now? Clin Exp Ophthalmol. 2002;30:334–337.
205 Leonardi M, Pitchon EM, Bertsch A, et al. Wireless contact lens sensor for intraocular pressure monitoring: assessment on enucleated pig eyes. Acta Ophthalmol. 2009;87:433–437.
206 Leonardi M, Leuenberger P, Bertrand D, et al. First steps toward noninvasive intraocular pressure monitoring with a sensing contact lens. Invest Ophthalmol Vis Sci. 2004;45:3113–3117.
207 Kakaday T, Hewitt AW, Voelcker NH, et al. Advances in telemetric continuous intraocular pressure assessment. Br J Ophthalmol. 2009;93:992–996.
208 Stangel K, Kolnsberg S, Hammerschmidt D, et al. A programmable intraocular CMOS pressure sensor system implant. IEEE J Solid-State Circuits. 2001;36:1094–1100.
209 Walter P, Schnakenberg U, vom Bogel G, et al. Development of a completely encapsulated intraocular pressure sensor. Ophthalmic Res. 2000;32:278–284.
210 Chen P-J, Rodger DC, Humayan MS, et al. Unpowered sprial-tube parylene pressure sensor for intraocular pressure sensing. Sensors Actuators A. 2006;127:276–282.
211 Rizq RN, Choi WH, Eilers D, et al. Intraocular pressure measurement at the choroid surface: a feasibility study with implications for implantable microsystems. Br J Ophthalmol. 2001;85:868–871.
212 Chow EY, Yang CL, Chlebowski A, et al. Implantable wireless telemetry boards for in vivo transocular transmission. IEEE Trans Microwave Theory Techniques. 2008;56:3200–3208.
213 Prow TW, Bhutto I, Grebe R, et al. Nanoparticle-delivered biosensor for reactive oxygen species in diabetes. Vision Res. 2008;48:478–485.
214 Becerra SP. Focus on Molecules: Pigment epithelium-derived factor (PEDF). Exp Eye Res. 2006;82:739–740.
215 Jones BW, Watt CB, Frederick JM, et al. Retinal remodeling triggered by photoreceptor degenerations. J Comp Neurol. 2003;464:1–16.
216 Milam AH, Li ZY, Fariss RN. Histopathology of the human retina in retinitis pigmentosa. Prog Retin Eye Res. 1998;17:175–205.
217 Lakhanpal RR, Yanai D, Weiland JD, et al. Advances in the development of visual prostheses. Curr Opin Ophthalmol. 2003;14:122–127.
218 Chen SJ, Mahadevappa M, Roizenblatt R, et al. Neural responses elicited by electrical stimulation of the retina. Trans Am Ophthalmol Soc. 2006;104:252–259.
219 Zrenner E. Will retinal implants restore vision? Science. 2002;295:1022–1025.
220 Weiland JD, Liu W, Humayun MS. Retinal prosthesis. Annu Rev Biomed Eng. 2005;7:361–401.
221 Choi SY, Sheng Z, Kramer RH. Imaging light-modulated release of synaptic vesicles in the intact retina: retinal physiology at the dawn of the post-electrode era. Vision Res. 2005;45:3487–3495.
222 Nagel G, Szellas T, Huhn W, et al. Channelrhodopsin-2, a directly light-gated cation-selective membrane channel. Proc Natl Acad Sci U S A. 2003;100:13940–13945.
223 Bi A, Cui J, Ma YP, et al. Ectopic expression of a microbial-type rhodopsin restores visual responses in mice with photoreceptor degeneration. Neuron. 2006;50:23–33.
224 Lagali PS, Balya D, Awatramani GB, et al. Light-activated channels targeted to ON bipolar cells restore visual function in retinal degeneration. Nat Neurosci. 2008;11:667–675.
225 Tomita H, Sugano E, Yawo H, et al. Restoration of visual response in aged dystrophic RCS rats using AAV-mediated channelopsin-2 gene transfer. Invest Ophthalmol Vis Sci. 2007;48:3821–3826.
226 Bowes C, Li T, Danciger M, et al. Retinal degeneration in the rd mouse is caused by a defect in the beta subunit of rod cGMP-phosphodiesterase. Nature. 1990;347:677–680.
227 D’Cruz PM, Yasumura D, Weir J, et al. Mutation of the receptor tyrosine kinase gene Mertk in the retinal dystrophic RCS rat. Hum Mol Genet. 2000;9:645–651.
228 Ivanova E, Pan ZH. Evaluation of the adeno-associated virus mediated long-term expression of channelrhodopsin-2 in the mouse retina. Mol Vis. 2009;15:1680–1689.
229 Wassle H. Parallel processing in the mammalian retina. Nat Rev Neurosci. 2004;5:747–757.
230 Jacobs AL, Werblin FS. Spatiotemporal patterns at the retinal output. J Neurophysiol. 1998;80:447–451.
231 Ivanova E, Hwang GS, Pan ZH, Troilo D. Evaluation of AAV-mediated expression of Chop2-GFP in the marmoset retina. Invest Ophthalmol Vis Sci. 2010;51:5288–5296.
232 Dalkara D, Kolstad KD, Caporale N, et al. Inner limiting membrane barriers to AAV-mediated retinal transduction from the vitreous. Mol Ther. 2009;17:2096–2102.
233 Zhang Y, Ivanova E, Bi A, et al. Ectopic expression of multiple microbial rhodopsins restores ON and OFF light responses in retinas with photoreceptor degeneration. J Neurosci. 2009;29:9186–9196.
234 Doroudchi MM, Greenberg KP, Liu J, et al. Virally delivered channelrhodopsin-2 safely and effectively restores visual function in multiple mouse models of blindness. Mol Ther. 2011.
235 Marc RE, Jones BW, Watt CB, et al. Extreme retinal remodeling triggered by light damage: implications for age related macular degeneration. Mol Vis. 2008;14:782–806.
236 Strettoi E, Pignatelli V, Rossi C, et al. Remodeling of second-order neurons in the retina of rd/rd mutant mice. Vision Res. 2003;43:867–877.
237 Gargini C, Terzibasi E, Mazzoni F, et al. Retinal organization in the retinal degeneration 10 (rd10) mutant mouse: a morphological and ERG study. J Comp Neurol. 2007;500:222–238.
238 Greenberg KP, Pham A, Werblin FS. Differential targeting of optical neuromodulators to ganglion cell soma and dendrites allows dynamic control of center-surround antagonism. Neuron. 2011;69:713–720.
239 Busskamp V, Duebel J, Balya D, et al. Genetic reactivation of cone photoreceptors restores visual responses in retinitis pigmentosa. Science. 2010;329:413–417.
240 Fortin DL, Banghart MR, Dunn TW, et al. Photochemical control of endogenous ion channels and cellular excitability. Nat Method. 2008;5:331–338.
241 Caporale N, Kolstad KD, Lee T, et al. LiGluR restores visual responses in rodent models of inherited blindness. Mol Ther. 2011;19:1212–1219.
242 Pennisi CPJP, Zachar V, Greenbaum E, et al. Incorporation of photosynthetic reaction centers in the membrane of human cells: Toward a new tool for optical control of cell activity. Cell Mol Bioeng. 2009;2:156–165.
243 Kuritz T, Lee I, Owens ET, et al. Molecular photovoltaics and the photoactivation of mammalian cells. IEEE Trans Nanobiosci. 2005;4:196–200.
244 Pennisi CP, Greenbaum E, Yoshida K. Spatial distribution of the electric potential from photosystem I reaction centers in lipid vesicles. IEEE Trans Nanobiosci. 2008;7:164–171.
245 Ahuja AK, Dorn JD, Caspi A, et al. Blind subjects implanted with the Argus II retinal prosthesis are able to improve performance in a spatial-motor task. Br J Ophthalmol. 2011;95:539–543.
246 Winter JO, Han N, Jensen R, et al. Adhesion molecules promote chronic neural interfaces following neurotrophin withdrawal. Conf Proc IEEE Eng Med Biol Soc. 2009;2009:7151–7154.
247 Kelly SK, Shire DB, Chen J, et al. Realization of a 15-channel, hermetically-encased wireless subretinal prosthesis for the blind. Conf Proc IEEE Eng Med Biol Soc. 2009;2009:200–203.
248 Zrenner E, Bartz-Schmidt KU, Benav H, et al. Subretinal electronic chips allow blind patients to read letters and combine them to words. Proc Biol Sci. 2011;278:1489–1497.
249 Julien S, Peters T, Ziemssen F, et al. Implantation of ultrathin, biofunctionalized polyimide membranes into the subretinal space of rats. Biomaterials. 2011;32:3890–3898.
250 Fujikado T, Kamei M, Sakaguchi H, et al. Testing of semichronically implanted retinal prosthesis by suprachoroidal-transretinal stimulation in patients with retinitis pigmentosa. Invest Ophthalmol Vis Sci. 2011;52:4726–4733.
251 Finlayson PG, Iezzi R. Glutamate stimulation of retinal ganglion cells in normal and s334ter-4 rat retinas: a candidate for a neurotransmitter-based retinal prosthesis. Invest Ophthalmol Vis Sci. 2010;51:3619–3628.
252 Kotov NA, Winter JO, Jan E, et al. Nanomaterials for neural interfaces. Adv Mater. 2009;21:3970–4004.
253 Lovric J, Bazzi HS, Cuie Y, et al. Differences in subcellular distribution and toxicity of green and red emitting CdTe quantum dots. J Mol Med. 2005;83:377–385.
254 Chan WH, Shiao NH, Lu PZ. CdSe quantum dots induce apoptosis in human neuroblastoma cells via mitochondrial-dependent pathways and inhibition of survival signals. Toxicol Lett. 2006;167:191–200.
255 Choi AO, Cho SJ, Desbarats J, et al. Quantum dot-induced cell death involves Fas upregulation and lipid peroxidation in human neuroblastoma cells. J Nanobiotechnol. 2007;5:1.
256 Maysinger D, Behrendt M, Lalancette-Hebert M, et al. Real-time imaging of astrocyte response to quantum dots: in vivo screening model system for biocompatibility of nanoparticles. Nano Lett. 2007;7:2513–2520.
257 Oberdorster E. Manufactured nanomaterials (fullerenes, C60) induce oxidative stress in the brain of juvenile largemouth bass. Environ Health Perspect. 2004;112:1058–1062.
258 Kim DH, Martin DC. Sustained release of dexamethasone from hydrophilic matrices using PLGA nanoparticles for neural drug delivery. Biomaterials. 2006;27:3031–3037.
259 Bharali DJ, Klejbor I, Stachowiak EK, et al. Organically modified silica nanoparticles: a nonviral vector for in vivo gene delivery and expression in the brain. Proc Natl Acad Sci U S A. 2005;102:11539–11544.
260 Zou LL, Huang L, Hayes RL, et al. Liposome-mediated NGF gene transfection following neuronal injury: potential therapeutic applications. Gene Ther. 1999;6:994–1005.
261 Green RA, Lovell NH, Wallace GG, et al. Conducting polymers for neural interfaces: challenges in developing an effective long-term implant. Biomaterials. 2008;29:3393–3399.
262 Abidian MR, Kim D-H, Martin DC. Conducting-polymer nanotubes for controlled drug release. Adv Mater. 2006;18:405–409.
263 Kim D-H, Richardson-Burns SM, Hendricks JL, et al. Effect of immobilized nerve growth factor on conductive polymers: electrical properties and cellular responses. Adv Functional Mater. 2007;17:79–86.
264 Cui X, Lee VA, Raphael Y, et al. Surface modification of neural recording electrodes with conducting polymer/biomolecule blends. J Biomed Mater Res. 2001;56:261–272.
265 Decher G. Fuzzy nanoassemblies: toward layered polymeric multicomposites. Science. 1997;277:1232–1237.
266 Gheith MK, Pappas TC, Lioppo AV, et al. Stimulation of neural cells by lateral currents in conductive layer-by-layer films of single-walled carbon nanotubes. Adv Mater. 2006;18:2975–2979.
267 Sinani VA, Koktysh DS, Yun B-G, et al. Collagen coating promotes biocompatibility of semiconductor nanoparticles in stratified LBL films. Nano Lett. 2003;3:1177–1183.
268 Pappas TC, Wickramanyake WM, Jan E, et al. Nanoscale engineering of a cellular interface with semiconductor nanoparticle films for photoelectric stimulation of neurons. Nano Lett. 2007;7:513–519.
269 Rama P, Matuska S, Paganoni G, et al. Limbal stem-cell therapy and long-term corneal regeneration. N Engl J Med. 2010;363:147–155.
270 Lewis GP, Linberg KA, Fisher SK. Neurite outgrowth from bipolar and horizontal cells after experimental retinal detachment. Invest Ophthalmol Vis Sci. 1998;39:424–434.
271 Lamba DA, Gust J, Reh TA. Transplantation of human embryonic stem cell-derived photoreceptors restores some visual function in Crx-deficient mice. Cell Stem Cell. 2009;4:73–79.
272 Lamba DA, Karl MO, Reh TA. Strategies for retinal repair: cell replacement and regeneration. Prog Brain Res. 2009;175:23–31.
273 Lamba DA, Karl MO, Ware CB, et al. Efficient generation of retinal progenitor cells from human embryonic stem cells. Proc Natl Acad Sci U S A. 2006;103:12769–12774.
274 Lamba DA, McUsic A, Hirata RK, et al. Generation, purification and transplantation of photoreceptors derived from human induced pluripotent stem cells. PLoS ONE. 2010;5:e8763.
275 Ikeda H, Osakada F, Watanabe K, et al. Generation of Rx+/Pax6+ neural retinal precursors from embryonic stem cells. Proc Natl Acad Sci U S A. 2005;102:11331–11336.
276 Osakada F, Ikeda H, Mandai M, et al. Toward the generation of rod and cone photoreceptors from mouse, monkey and human embryonic stem cells. Nat Biotechnol. 2008;26:215–224.
277 Hirami Y, Osakada F, Takahashi K, et al. Generation of retinal cells from mouse and human induced pluripotent stem cells. Neurosci Lett. 2009;458:126–131.
278 Osakada F, Jin ZB, Hirami Y, et al. In vitro differentiation of retinal cells from human pluripotent stem cells by small-molecule induction. J Cell Sci. 2009;122:3169–3179.
279 Meyer JS, Katz ML, Maruniak JA, et al. Embryonic stem cell-derived neural progenitors incorporate into degenerating retina and enhance survival of host photoreceptors. Stem Cells. 2006;24:274–283.
280 Seiler MJ, Aramant RB, Thomas BB, et al. Visual restoration and transplant connectivity in degenerate rats implanted with retinal progenitor sheets. Eur J Neurosci. 2010;31:508–520.
281 MacLaren RE, Pearson RA, MacNeil A, et al. Retinal repair by transplantation of photoreceptor precursors. Nature. 2006;444:203–207.
282 Gust J, Reh TA. Adult donor rod photoreceptors integrate into the mature mouse retina. Invest Ophthalmol Vis Sci.. 2011.
283 Tomita M, Lavik E, Klassen H, et al. Biodegradable polymer composite grafts promote the survival and differentiation of retinal progenitor cells. Stem Cells. 2005;23:1579–1588.
284 Karl MO, Reh TA. Regenerative medicine for retinal diseases: activating endogenous repair mechanisms. Trends Mol Med. 2010;16:193–202.
285 Pot SA, Liliensiek SJ, Myrna KE, et al. Nanoscale topography-induced modulation of fundamental cell behaviors of rabbit corneal keratocytes, fibroblasts, and myofibroblasts. Invest Ophthalmol Vis Sci. 2010;51:1373–1381.
286 Sugino IK, Gullapalli VK, Sun Q, et al. Cell-deposited matrix improves retinal pigment epithelium survival on aged submacular human Bruch’s membrane. Invest Ophthalmol Vis Sci. 2011;52:1345–1358.
287 Scadden DT. The stem-cell niche as an entity of action. Nature. 2006;441:1075–1079.
288 Park J, Bauer S, von der Mark K, et al. Nanosize and vitality: TiO2 nanotube diameter directs cell fate. Nano Lett. 2007;7:1686–1691.
289 Chua KN, Chai C, Lee PC, et al. Surface-aminated electrospun nanofibers enhance adhesion and expansion of human umbilical cord blood hematopoietic stem/progenitor cells. Biomaterials. 2006;27:6043–6051.
290 Dalby MJ, McCloy D, Robertson M, et al. Osteoprogenitor response to defined topographies with nanoscale depths. Biomaterials. 2006;27:1306–1315.
291 Steedman MR, Tao SL, Klassen H, et al. Enhanced differentiation of retinal progenitor cells using microfabricated topographical cues. Biomed Microdevices. 2010;12:363–369.
292 Recknor JB, Sakaguchi DS, Mallapragada SK. Directed growth and selective differentiation of neural progenitor cells on micropatterned polymer substrates. Biomaterials. 2006;27:4098–4108.
293 Mooney DJ, Vandenburgh H. Cell delivery mechanisms for tissue repair. Cell Stem Cell. 2008;2:205–213.
294 Silva GA, Czeisler C, Niece KL, et al. Selective differentiation of neural progenitor cells by high-epitope density nanofibers. Science. 2004;303:1352–1355.
295 Ellis-Behnke RG, Liang YX, You SW, et al. Nano neuro knitting: peptide nanofiber scaffold for brain repair and axon regeneration with functional return of vision. Proc Natl Acad Sci U S A. 2006;103:5054–5059.
296 Hynes SR, Lavik EB. A tissue-engineered approach towards retinal repair: scaffolds for cell transplantation to the subretinal space. Graefes Arch Clin Exp Ophthalmol. 2010;248:763–778.
297 Lavik EB, Klassen H, Warfvinge K, et al. Fabrication of degradable polymer scaffolds to direct the integration and differentiation of retinal progenitors. Biomaterials. 2005;26:3187–3196.
298 Redenti S, Tao S, Yang J, et al. Retinal tissue engineering using mouse retinal progenitor cells and a novel biodegradable, thin-film poly(e-caprolactone) nanowire scaffold. J Ocul Biol Dis Infor. 2008;1:19–29.
299 Sodha S, Wall K, Redenti S, et al. Microfabrication of a three-dimensional polycaprolactone thin-film scaffold for retinal progenitor cell encapsulation. J Biomat Sci. 2011;22:443–456.
300 Tao SL, Desai TA. Micromachined devices: the impact of controlled geometry from cell-targeting to bioavailability. J Controlled Release. 2005;109:127–138.
301 Sacconi L, O’Connor RP, Jasaitis A, et al. In vivo multiphoton nanosurgery on cortical neurons. J Biomed Optics. 2007;12:050502.
302 Ellis-Behnke RG, Teather LA, Schneider GE, et al. Using nanotechnology to design potential therapies for CNS regeneration. Curr Pharm Design. 2007;13:2519–2528.
303 Wang BG, Halbhuber KJ. Corneal multiphoton microscopy and intratissue optical nanosurgery by nanojoule femtosecond near-infrared pulsed lasers. Ann Anat. 2006;188:395–409.
304 Leary SP, Liu CY, Apuzzo ML. Toward the emergence of nanoneurosurgery: part III – nanomedicine: targeted nanotherapy, nanosurgery, and progress toward the realization of nanoneurosurgery. Neurosurgery. 2006;58:1009–1026. discussion-26
305 Fankhauser F, Niederer PF, Kwasniewska S, et al. Supernormal vision, high-resolution retinal imaging, multiphoton imaging and nanosurgery of the cornea – a review. Technol Health Care. 2004;12:443–453.
306 Konig K. Robert Feulgen Prize Lecture. Laser tweezers and multiphoton microscopes in life sciences. Histochem Cell Biol. 2000;114:79–92.
307 Kim P, Lieber CM. Nanotube nanotweezers. Science. 1999;286:2148–2150.
308 Bhisitkul RB, Keller CG. Development of microelectromechanical systems (MEMS) forceps for intraocular surgery. Br J Ophthalmol. 2005;89:1586–1588.
309 Zhang LW, Monteiro-Riviere NA. Mechanisms of quantum dot nanoparticle cellular uptake. Toxicol Sci. 2009;110:138–155.
310 Hoet P, Legiest B, Geys J, et al. Do nanomedicines require novel safety assessments to ensure their safety for long-term human use? Drug Saf. 2009;32:625–636.
311 Magrez A, Kasas S, Salicio V, et al. Cellular toxicity of carbon-based nanomaterials. Nano Lett. 2006;6:1121–1125.
312 Zhu L, Chang DW, Dai L, et al. DNA damage induced by multiwalled carbon nanotubes in mouse embryonic stem cells. Nano Lett. 2007;7:3592–3597.