Chapter 48 Mechanical Ventilation
I Introduction
Esteban and colleagues reviewed the use of mechanical ventilation in intensive care units (ICUs) in North America, South America, Spain, and Portugal. Among the indications for mechanical ventilation, acute respiratory failure was the most common (66% of patients), followed by coma (15%), exacerbation of chronic obstructive pulmonary disease (COPD, 13%), and neuromuscular weakness (5%). The principal causes of acute respiratory failure across all centers were pneumonia (16%), sepsis (16%), postoperative infection (15%), heart failure (12%), acute respiratory distress syndrome (ARDS, 12%), trauma (12%), unspecified causes (13%), and aspiration (3%). Endotracheal tubes were used three times more often than tracheostomies to provide artificial airways. There was some variability in the modes of ventilation used in the different countries participating in the study. Assist-control ventilation (ACV) was the most common worldwide, followed by synchronized intermittent mandatory ventilation (SIMV) with pressure support and by pressure-support ventilation (PSV) alone. However, in North American ICUs, ACV and SIMV were used equally.1
Common reasons for insertion of an artificial airway are to maintain airway patency, to prevent aspiration, to facilitate clearance of secretions, and to allow mechanical ventilatory support.2 There are several indications for mechanical ventilation:
II Initiation of Mechanical Ventilation
Mechanical ventilation can be delivered to the patient by invasive or noninvasive methods. Noninvasive positive-pressure ventilation (NIPPV) is delivered by an external nasal or a naso-oral interface such as a face mask. The decision to use invasive or noninvasive mechanical ventilation depends on the severity and rapid anticipated reversibility of the underlying condition and the mental status of the patient. NIPPV is useful in cases of hypercapnic respiratory failure, especially associated with COPD; obstructive sleep apnea; cardiogenic pulmonary edema; and hypercapnic respiratory insufficiency in persons with adequate mental status to remain communicative.3–5 Application of NIPPV requires frequent assessments to ensure that the desired goal of oxygenation or ventilation is being achieved.
A Tidal Volume
Tidal volume is the volume of air delivered to the lungs with each breath by the mechanical ventilator. Historically, initial tidal volumes were set at 10 to 15 mL/kg of actual body weight for patients with neuromuscular diseases. Over the past 2 decades, VILI has been associated with excessive tidal volume leading to alveolar distention.6,7 The mechanism of lung injury includes regional overinflation,8 stress of repeated opening and closing of lung units,9,10 and sheer stress between adjacent structures with differing mechanical properties.11
The low-tidal-volume strategy, which uses 6 mL/kg of predicted body weight, has become the standard of care for patients with ARDS, following the Acute Respiratory Distress Syndrome Network (ARDS Network) publication in 2000.12 The ARDS Network prospectively studied intubated patients with acute lung injury (ALI) or ARDS to determine whether a low-tidal-volume strategy, compared with a traditional-tidal-volume strategy, could improve mortality and decrease the total number of ventilator days. The final analysis showed a 23% reduction in all-cause mortality and a 9% absolute decrease in mortality with the use of a tidal volume of 6 mL/kg of predicted body weight and plateau pressures of 30 cm H2O or less, compared with the usual practice of 12 mL/kg of predicted body weight and plateau pressures of 50 cm H2O or less. Low tidal volume or so-called lung protective ventilation is recommended for all patients with ARDS. In patients without ARDS, a retrospective review demonstrated the relationship between ALI and the use of tidal volumes greater than 10 mL/kg of predicted body weight.13 Considering the current evidence, tidal volumes greater than 10 mL/kg of predicted body weight should not be routinely used in the care of the mechanically ventilated patient.12,13
C Positive End-Expiratory Pressure
PEEP is the alveolar pressure above the atmospheric pressure at end-expiration. Applied PEEP (i.e., extrinsic PEEP) through mechanical ventilation allows delivery of positive pressure at the end of expiration to keep the unstable lung units from collapse.14 PEEP increases the peak inspiratory pressure, which directly overcomes the opening pressure of the unstable lung units. Low levels of PEEP (3 to 5 cm H2O) are routinely used in patients on mechanical ventilation. It can decrease alveolar collapse at end-expiration and may reduce the incidence of ventilator-associated pneumonia.15 Higher levels of PEEP are employed to improve oxygenation in patients with hypoxic respiratory failure. Goals in managing ARDS are to optimize alveolar recruitment and decrease cycles of recruitment and derecruitment of alveolar lung units. Several strategies are used to determine optimal PEEP, but there are limited data to support their routine use. Determining the lower inflection point of the pressure-volume curve (Pflex), which reflects the transition from low to higher compliance, and applying PEEP of 2 cm H2O greater than this point may be used to estimate the appropriate level of applied PEEP (Fig. 48-1).16
Because it is often impractical to routinely obtain pressure-volume curves, algorithms have been developed (e.g., in ARDS Network trials), with PaO2/FIO2 ratios to set the recommended PEEP (Table 48-1).12 Measuring esophageal pressures to estimate transpulmonary pressures has been studied as a method to determine the appropriate applied PEEP in patients with ARDS, and this approach has demonstrated improvement in oxygenation and compliance.17 Trials of increasing or decreasing PEEP can also be used.18,19 Higher levels of PEEP in postoperative patients have had no benefit.20
Lung injury in patients with hypoxic respiratory failure is heterogeneous. Since the collapse and repeated opening and closing of unstable lung units leads to further injury, its prevention would be the optimal ventilator strategy. High PEEP has been used mitigate alveolar collapse and cyclic alveolar stress. Several trials demonstrated that high PEEP increased oxygenation but did not improve mortality rates.21,22 However, a meta-analysis of high PEEP trials indicated a mortality benefit for patients with a PaO2/FIO2 ratio of less than 200.23 The optimal method of applying adequate PEEP has not been established.14 Trials in ARDS patients demonstrate PEEP requirements are usually between 12 and 20 cm H2O.
D Fraction of Inspired Oxygen
On initiation of mechanical ventilation, the FIO2 usually is set at 1.0. The goal is to rapidly reduce the FIO2 to the target PaO2 and SpO2 to limit the consequences of supplemental oxygen. In most patients, a target PaO2 of 60 mm Hg and SpO2 of 90% meets oxygenation requirements. However, some patients may have higher PaO2 targets based on their underlying cardiopulmonary status (e.g., myocardial ischemia, pulmonary hypertension). In patients with ARDS, targeting a PaO2 as low as 50 mm Hg may be appropriate to limit alveolar injury.24 A prolonged high level of FIO2 has been associated with airway and parenchymal injury, atelectasis from nitrogen washout, and increased risk of diffuse alveolar damage, which is even higher in patients receiving bleomycin therapy.25,26 If the need for supplemental FIO2 remains greater than 0.6, FIO2 should be reduced with strategies such as applied PEEP and alternative ventilator modes.
E Peak Pressure
Peak airway pressure is a measurement of the maximum pressure felt by the airways on inspiration. In the passive patient, peak airway pressure, or peak pressure, depends on the respiratory rate, tidal volume, and inspiratory flow rate in volume-targeted modes of mechanical ventilation. When awake and active, the patient’s effort contributes to the peak pressure. In pressure-targeted ventilator modes, the peak pressure is directly related to the inspiratory pressure that is set and the inspiratory flow rate.27 Studies have not consistently shown barotrauma to be an adverse consequence of increased peak pressures.28,29 The peak airway pressure typically is higher than the plateau pressure, and the difference indicates airway resistance.
F Plateau Pressure
Plateau pressure is the pressure that is applied by the mechanical ventilator to the small airways and alveoli. The plateau pressure is measured at end-inspiration with an inspiratory hold maneuver on the mechanical ventilator that is 0.5 to 1 second. Meta-analysis demonstrated a significant correlation between plateau pressures greater than 35 cm H2O and the risk of barotrauma.30 In the ARDS Network trial, lower tidal volume ventilation with plateau pressures less than 30 mm Hg was associated with a lower mortality rate than that found for conventional tidal volume using plateau pressures less than 50 mm Hg.12
H Flow Rate
Flow rate, or peak inspiratory flow rate, is the maximum flow at which a set tidal volume breath is delivered by the ventilator. Most modern ventilators can deliver flow rates between 60 and 120 L/min. Flow rates should be titrated to meet the patient’s inspiratory demands.31 If the peak flow rate is too low for the patient, dyspnea, patient-ventilator asynchrony, and increased work of breathing may result. High peak flow rates increase peak airway pressures and lower mean airway pressures, which may decrease oxygenation.27
In most patients, peak flow rates of 60 L/min are adequate. Higher flow rates are required in patients with higher ventilator demands.31 Higher peak flow rates may also be necessary in patients with obstructive lung disease to decrease inspiratory time, thereby increasing the expiratory time and reducing the risk of developing auto-PEEP.32,33
I Flow Pattern
Modern mechanical ventilators can deliver various inspiratory flow patterns. The constant or square waveform is a description of the inspiratory flow that is delivered by the mechanical ventilator. It correlates with volume-cycled breaths, in which the inspiratory flow remains constant until the desired tidal volume is delivered and then remains at that level until expiration. In this pattern of inspiratory flow, the airway pressure varies and depends on the patient’s effort and compliance of the lung. The sinusoidal wave flow gradually increases and decreases throughout the respiratory cycle. In the decelerating ramp wave (i.e., saw-tooth wave), the flow rate begins maximally and decreases until the end of inspiration. It parallels normal inspiratory pattern most closely. The ramp wave yields the most homogenous distribution of ventilation in most conditions, decreases peak airway pressures, and improves carbon dioxide (CO2) elimination.34 For patients who are triggering the ventilator, this strategy of ventilation is recommended (Fig. 48-2).
III Common Modes of Mechanical Ventilation
Choice of the type of mechanical ventilation is most often determined by whether resting of respiratory muscles is indicated. Patients who are hemodynamically compromised, patients with severe oxygenation or ventilation derangements, and those undergoing general endotracheal anesthesia qualify for a rest of respiratory muscles. In these cases, it is prudent to choose a mode of ventilation that accomplishes ventilation without the need for spontaneous respirations; ACV is most often used. However, if use of muscles of respiration is desired, SIMV or PSV should be considered. Patients in whom the use of respiratory muscles is desired are usually those being weaned from mechanical ventilation or undergoing assessment of muscle strength and adequacy of spontaneous work of breathing. PSV is the only mode of the three that entirely relies on the patient spontaneously breathing. Table 48-2 shows the set and variable parameters in each common mode of ventilation. Control mode of ventilation (CMV) is the original mode of ventilation. In CMV, the patient receives a positive-pressure breath at a set rate without the ability to influence how it is delivered.
A Assist-Control Ventilation
VACV is a combination mode of ventilation in which the preset tidal volume is delivered in response to the inspiratory effort or if no patient effort occurs within a set period of time. The period is determined by the backup respiratory rate set on the ventilator. A patient-triggered breath is sensed by a change in airway flow or pressure. When the change reaches the trigger threshold, the ventilator delivers the predetermined tidal volume. In ACV, the limit variable that increases to the set threshold before inspiration ends is volume or flow, or both. The cycle variable that ends inspiration is volume or time. Peak inspiratory airway pressure and plateau pressure are variable in this setting. In patients with deep sedation or neuromuscular blockade, the ACV mode functions like CMV. The advantage of ACV is that it substantially decreases the work of breath and decreases myocardial oxygen demand. The disadvantages of ACV in the active patient are that it is less comfortable than spontaneous breathing and that it can induce respiratory alkalosis and breath-stacking (Table 48-3).
TABLE 48-3 Advantages and Disadvantages of Conventional Modes
Ventilation Mode | Advantages | Disadvantages |
---|---|---|
ACV |
ACV, Assist-control ventilation; PEEP, positive end-expiratory pressure; PSV, pressure-support ventilation; SIMV, synchronized intermittent mandatory ventilation.
Similar to volume-targeted ACV, the pressure-targeted ACV mode requires the user to input the frequency (i.e., desired respiratory rate), PEEP, and FIO2, but instead of a desired tidal volume, the user sets the upper limit of the inspiratory pressure that is allowable. As the ventilator delivers a breath, the inspiratory flow continues until the maximum pressure or allotted time is reached, and the flow then ceases. In pressure-targeted ACV, the tidal volume varies, and consistency is sacrificed to prevent barotrauma by high pressures (Table 48-4).35 Figure 48-3 depicts the differences in VACV and pressure assist-control ventilation (PACV) in graphs of pressure versus time and airflow versus time.
TABLE 48-4 Comparison of Volume-Targeted and Pressure-Targeted Assist-Control Ventilation
Parameter | Volume-Targeted Ventilation | Pressure-Targeted Ventilation |
---|---|---|
Frequency (rate) | Set | Set |
Tidal volume | Set | Variable |
Inspiratory flow | Set | Set |
Peak inspiratory pressure | Variable | Set |
PEEP | Set | Set |
FIO2 | Set | Set |
PEEP, Positive end-expiratory pressure; FIO2, fraction of inspired oxygen.
ARDS is commonly seen in medical and surgical patients and presents dilemmas in treatment.36 According to the 1994 American-European Consensus Conference definition, ARDS is recognized as a spectrum, which includes ALI, as defined by a ratio of the partial pressure of arterial oxygen to the fraction of inspired oxygen (PaO2/FIO2) of 300 or less, and ARDS, which is defined as a PaO2/FIO2 ratio of 200 or less. Other characteristics of ARDS are the acute onset of bilateral pulmonary infiltrates and a pulmonary capillary wedge pressure of less than 18 mm Hg (or no evidence of elevated left atrial pressure). ARDS is synonymous with noncardiogenic pulmonary edema.37 ALI has many direct and indirect causes. Examples of direct injury are pneumonia, orogastric fluid aspiration, and inhalation injury; indirect causes of injury include severe sepsis, shock, pancreatitis, blood product transfusion, and narcotic overdose.38
Ventilatory strategies for the management of ARDS rest on the results of the ARDS Network studies, which demonstrated that patients given tidal volumes of 6 mL/kg of predicted body weight had improved mortality rates compared with patients with tidal volumes of 12 mL/kg of predicted body weight. Another finding was that plateau pressures less than 30 cm H2O protect the lung (Fig. 48-4 and Table 48-5).12 In 1998, Amato and colleagues demonstrated the mortality benefit of lower tidal volumes and a lower rate of barotraumas (Fig. 48-5).16 Two meta-analyses demonstrated decreased mortality rates with the use of low tidal volume ventilation (i.e., lung-protective ventilation).39,40 In ARDS management, plateau pressures should be less than or equal to 30 cm H2O or the lowest possible level. A high-PEEP strategy decreased the mortality rate in a meta-analysis of 2299 ARDS patients.23 Randomized trials of ventilation in ARDS patients are summarized in Table 48-6.
TABLE 48-5 Main Outcome Variables in the Acute Respiratory Distress Syndrome (ARDS) Network Trial of Low Tidal Volumes versus Traditional Tidal Volumes in Patients with ARDS
B Synchronized Intermittent Mandatory Ventilation
SIMV is a frequently used mode of ventilation in hospital medical and surgical units. SIMV has some features that incorporate characteristics of ACV and PSV. SIMV uses the same settings as ACV: frequency, tidal volume, PEEP, and FIO2, but it also has a setting for a prescribed pressure support for a spontaneous breath. The purpose of the two types of breaths (i.e., mandatory and spontaneous) is to allow increased diaphragmatic activity and increased work of breathing by the patient when triggering spontaneous breaths. SIMV is sometimes used as a weaning mode, but it can prolong mechanical ventilation and is therefore not routinely recommended for weaning.41
When a patient is deeply sedated or paralyzed, SIMV functions the same as ACV. The patient receives the set number of mandatory breaths as determined by the set frequency at the prescribed tidal volume with the set PEEP and FIO2. However, in patients who are more awake, SIMV can assist the patient when he or she triggers a mandatory breath and can support the patient with the prescribed pressure support and PEEP in spontaneous breaths that are above the number of mandatory breaths set. The advantage of this mode is that it allows the patient to get the set number of mandatory breaths by controlling the breaths (if the patient is not initiating inspiration) or assisting breaths (if the patient is triggering the start of inspiration), and the patient is allowed to make an effort at spontaneous breaths. The use of SIMV also may reduce sedation requirements (see Table 48-3).42
C Pressure-Support Ventilation
PSV is used for patients who are awake enough to accomplish spontaneous breathing. PSV was initially developed to reduce work of breathing in SIMV but evolved into a stand-alone mode of ventilation. PSV augments the patient’s spontaneous inspiratory efforts with the selected level of positive airway pressure. The inspiratory pressure is delivered until the flow decreases to a predetermined level (usually 25% of peak flow). PSV allows the user to control the desired pressure support, PEEP, and FIO2. This mode relies entirely on spontaneous breaths by the patient, who must have an intact ventilatory drive. The work of breathing of PSV is inversely proportional to the level of pressure support and the flow rate.43 Because no tidal volume is guaranteed by this mode of ventilation, pressure support must be titrated to help the patient achieve an adequate tidal volume. However, any change in lung compliance or airway impedance results in a change in tidal volume. A certain level of pressure support is needed to overcome the resistance of the ventilator circuit and endotracheal tube. This typically is less than 10 cm H2O, but it can be higher with narrower endotracheal tubes.44 Pressure support above that needed to overcome resistance supplements the achieved tidal volumes. Typical pressure support settings are 5 to 25 mm H2O. When full ventilator support is needed for the patient, PSV may not be the ideal mode because it requires a higher work of breathing and minute ventilation is not guaranteed (see Table 48-3).
D Pressure-Regulated Volume Control
Pressure-regulated volume control (PRVC) can be employed with ACV or SIMV. With PRVC, the ventilator monitors the patient’s effort and varies the peak inspiratory pressure that is allowed with the inspiratory flow to achieve the set tidal volume. The ventilator provides a breath at a low pressure and then calculates the peak pressure necessary to deliver the set tidal volume. That pressure level is delivered during the next breath. If the target is not attained, the peak pressure is adjusted by 1 to 3 cm H2O for the next breath. This allows breath-to-breath correction and enables increased airway pressure control. Because the inspiratory time and flow are autoregulated, it results in a smaller increase in plateau airway pressures with a given tidal volume. Because the ventilator responds to the patient’s effort in PRVC, as the patient’s ventilator demand increases, the inspiratory pressure decreases, thereby increasing the patient’s work of breathing.45
IV Uncommon Modes of Ventilation
A Inverse-Ratio Ventilation
Inverse-ratio ventilation (IRV) is positive-pressure ventilation with an inspiratory-expiratory (I : E) ratio of greater than 1. It has been used in the management of severe ARDS to improve oxygenation when PEEP has been optimized.46 I : E ratios usually range from 1.2 to 1.5, whereas in IRV, they may be 1 : 1, 2 : 1, or higher. Increasing the inspiratory time increases mean airway pressure without increasing the inspiratory plateau pressure, which may improve oxygenation.47,48 This application is most commonly used with time-cycled pressure-control ventilation (PCV), but it can also be used with volume-cycled ventilation. The improvements in oxygenation are modest, and carbon dioxide elimination is preserved or enhanced48,49; however, not all studies have shown benefit.50 Development of auto-PEEP is common in IRV, and it may be responsible for some of the improvements in oxygenation, but it also increases the risk of barotrauma. Because the benefits of IRV are controversial, it should be limited to use in patients with severe ARDS with refractory hypoxemia.51
B Airway Pressure–Release Ventilation
Airway pressure–release ventilation (APRV) is similar to a blend of inverse-ratio PCV and SIMV. APRV offers two levels of continuous positive airway pressure (CPAP) ventilation, in which it uses high and low pressures to aid in recruitment of atelectatic lung units and allows spontaneous breathing.52,53 The continuous high positive pressure (Phigh) is delivered by the ventilator for a prolonged duration (Thigh) and then drops to the lower pressure (Plow) for a short duration (Tlow). Spontaneous breathing can occur during high and low pressures. Overall, the lower pressure is set to manage hypoxemia and the upper level to promote CO2 elimination. When the patient cannot initiate breaths, the mode is identical to inverse-ratio PCV.54 One study of 24 patients with ARDS showed that when APRV was compared with PSV, APRV improved oxygenation and cardiac parameters, along with improvements in ventilation-perfusion matching in the lung.55
APRV can decrease peak airway pressures, improve oxygenation, improve alveolar recruitment, and improve cardiac output, but the findings have been inconsistent, and there has been no evidence of mortality benefit.56–59 During periods of transition between low and high pressures, patient ventilator dyssynchrony can occur. APRV is not recommended for patients with obstructive lung disease or high levels of minute ventilation (Figs. 48-6 and 48-7).
Bi-level ventilation is similar to APRV but has additional features.60 The transitions from low and high pressures are coordinated with the patient’s effort to reduce dyssynchrony. Tlow usually is longer in bi-level ventilation, which allows for more spontaneous breaths to occur at this pressure level. As in APRV, bi-level ventilation has been used primarily in patients with ALI or ARDS, and it should be avoided in patients with obstructive lung disease because of the risk of auto-PEEP due to shortened expiratory times.
C High-Frequency Ventilation
High-frequency oscillatory ventilation (HFOV), sometimes called the oscillator, is a means of improving oxygenation by providing an oxygen-rich and CO2-poor gas at high respiratory rates that rapidly mixes with sinusoidal flow at stroke volumes that approximate anatomic dead space. HFOV is the most commonly used high-frequency ventilator in adults. HFOV uses a pump to generate a respiratory frequency of 3 to 6 Hz or 180 to 360 breaths/min. In a 1984 publication, Chang described five mechanisms of oxygen delivery by high-frequency oscillation: direct alveolar ventilation of proximal airways; bulk convective gas mixing in conductive airways by recirculation of air among neighboring airways in different cycles of opening and closing of the alveolus; convective transport of gases; longitudinal dispersion by airway turbulence; and molecular diffusion.61 Additional work is needed to delineate the exact mechanisms involved in gas exchange in high-frequency ventilation.
Selection guidelines for HFOV do not exist, but patients with ARDS who develop refractory hypoxemia on conventional ventilation are sometimes considered.62–64 In patients with ALI that progresses to ARDS, high PEEP and FIO2 values may be required to sustain oxygenation. HFOV uses high airway pressures during very short time intervals to help recruit and oxygenate atelectatic lung. The mean airway pressure is set by manipulation of the inspiratory flow rate and an expiratory back-pressure valve. A multicenter trial of 148 patients with ARDS compared conventional ventilation with high-frequency oscillatory ventilation. Patients were randomized to receive conventional mechanical ventilation or high-frequency oscillatory ventilation and were followed to compare 30-day ventilator-free survival. Results demonstrated improved 30-day mortality rates in the HFOV group, but it did not rise to statistical significance, nor did various secondary end points, including rates for 6-month mortality and duration of mechanical ventilation.63 HFOV in patients with obstructive lung disease (e.g., COPD, asthma) may lead to significant auto-PEEP.
The oscillator requires vigilance on the part of the physician and respiratory therapist because patients are usually hypoxemic at the initiation of HFOV. Because the oscillator is uncomfortable for patients, they usually require increased sedation and often need pharmacologic paralysis. Careful titration of inspiratory pressure and frequency are needed to obtain optimal settings. During HFOV, ventilation is a passive process, and the patient must have the endotracheal tube cuff deflated to allow for passive exhalation of CO2. Overall, HFOV appears to be equivalent to conventional ventilation in ARDS patients and useful in the management of refractory hypoxemia and severe air leaks (Fig. 48-8).65
In high-frequency jet ventilation (HFJV), a pressurized gas is introduced by inserting a cannula from the HFJV device into the endotracheal tube. An initial pressure of 35 pounds per square inch is set with a rate of 100 to 150 breaths/min and an inspiratory fraction of 30%.66 HFJV is more commonly employed in pediatric patients, but it has some use in laryngeal surgery. The drawback of conventional jet ventilators is there is not an adequate measure of intrapulmonary pressure in the circuit, and the patient may be at risk for volutrauma from overdistention of distal airways.
High-frequency pressure ventilation (HFPV) is a time-cycled, pressure-limited mode of ventilation that delivers subphysiologic tidal volumes at rates as high as 500 breaths/min.67 HFPV has been used in burn units, specifically for patients with inhalation lung injury, and for salvage therapy in patients with severe ARDS.68 The basic tenets are the same as for HFOV, but it oscillates at two different pressure levels. A Phasitron valve at the end of the endotracheal tube delivers small tidal volumes at frequencies of 200 to 900 breaths/min superimposed on PCV. Whereas the HFOV uses rapid oscillations of small volumes at high frequencies that transiently reach high airway pressures, the HFPV prolongs the application of high airway pressure at high frequencies to assist in clearance of mucus and in sloughing airway secretions. A single-center, prospective, randomized trial comparing HFPV with low tidal volume ventilation in burn patients with ALI demonstrated no difference in mortality rates or ventilator-free days.69
V Noninvasive Ventilation
NIPPV provides ventilatory support through an external interface such as a nasal or oronasal mask that is firmly secured to the face. The physiologic effect of NIPPV rests in its ability to provide positive pressure into the nasopharynx or oropharynx that splints open the airway and is then transmitted downstream to the lungs, where it increases lung volume. Several cardiovascular effects are seen with NIPPV in this setting. Decreased venous return occurs, and the increased intrathoracic pressure can decrease afterload and thereby increase cardiac output. Figure 48-9 details the interaction of the effects of NIPPV on the cardiopulmonary system.
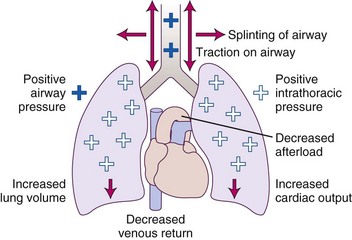
Figure 48-9 Physiologic effects of positive airway pressure.
(Adapted from Antonescu-Turcu A, Parthasarathy S: CPAP and Bi-level PAP therapy: New and established roles. Respir Care 55:1216–1228, 2010.)
Patient selection is paramount in the decision to initiate NIPPV. NIPPV is not recommended for patients with upper airway obstruction, cardiac arrest, hemodynamic instability, respiratory arrest, injury of the face, massive gastrointestinal bleeding, a high risk of aspiration, significantly depressed mentation, or an inability to clear secretions (Table 48-7).70–72
TABLE 48-7 Factors for Success or Failure of Noninvasive Positive-Pressure Ventilation
Success | Failure |
---|---|
A-a, Alveolar to arterial; APACHE, Acute Physiology and Chronic Health Evaluation; PaCO2, arterial partial pressure of carbon dioxide; NIPPV, noninvasive positive-pressure ventilation.
NIPPV has been useful in many situations, including exacerbations of COPD with hypercapnia,73–75 cardiogenic pulmonary edema,76–79 hypercapnic respiratory failure (i.e., Glasgow Coma Scale score greater than 10), and hypoxic respiratory failure.
NIPPV can be delivered by a standard ventilator through a face mask, nasal mask, or nasal plugs. Heated humidification increases the patient’s comfort.80 Common modes of ventilation used to deliver NIPPV are CPAP, bi-level positive airway pressure (BPAP), PSV, proportional-assist ventilation (PAV), and ACV. Modes of NIPPV may be selected on the basis of patient characteristics. For example, patients who require greater support in reducing the work of breathing should be placed on ACV mode, or the patient’s comfort may be increased with PSV.81 There was no difference in mortality rates between these modalities for various disease states.82–85 The patient should be monitored very closely after initiation of NIPPV. If prompt improvement is not evident within 1 or 2 hours, the physician should proceed to intubation.86 In a prospective, multicenter, cohort study, NIPPV failed in 30% of patients. The highest intubation rates occurred in patients with ARDS (51%) or community-acquired pneumonia (50%), and the lowest rates were for those with pulmonary contusion (18%) or cardiogenic pulmonary edema (10%) (Fig 48-10).87
NIPPV improves mortality rates and length of stay for patients with severe COPD exacerbations.4 A meta-analysis of patients with severe COPD exacerbations and mild COPD exacerbations demonstrated no mortality benefit for the patients with milder COPD exacerbations.74 In cases of cardiogenic pulmonary edema, NIPPV decreases the rate of intubation, but the mortality benefit is uncertain because of conflicting study results.3,88 Patients with hypoxic respiratory failure and asthma exacerbations may benefit from NIPPV. NIPPV use in post-extubation failure has been studied.89,90 If NIPPV is used immediately on extubation in patients with hypercapnia during the spontaneous breathing trial, it may prevent reintubation and is associated with a reduction in mortality rates.91 NIPPV use in patients after the development of post-extubation failure did not reduce reintubation rates and increased mortality rates, with a longer median time from failure to reintubation in the NIPPV group.92
VI Weaning From Mechanical Ventilation
In 2001, a collective task force from the American College of Chest Physicians, the American Association of Respiratory Care, and the American College of Critical Care Medicine examined the issue of discontinuation of mechanical ventilation and defined patients who required prolonged mechanical ventilation and strategies to liberate them from the mechanical ventilator. They found that patients who are mechanically ventilated spend approximately 42% of their ventilator time undergoing the weaning process. The task force offered 12 recommendations to standardize practice for discontinuing mechanical ventilation, including searching for causes of respiratory failure; early discontinuation of sedation of postoperative patients; ensuring daily spontaneous breathing trials for patients who meet the criteria for hemodynamic, pulmonary, and mental stability; outlining criteria for evaluation of patients on a spontaneous breathing trial; and strategies for prolonged weaning and daily spontaneous breathing trials assisted by nonphysician practitioners within the health care organization.93 The clinical criteria outlined by the task force includes clinically improving cause of respiratory failure, adequate oxygenation (defined as PaO2/FIO2 greater than 150 mm Hg or oxyhemoglobin saturation greater than 90%, while receiving FIO2 less than or equal to 0.4 and a PEEP less than or equal to 5 cm H2O); hemodynamic stability (absent or low-dose vasopressors and no signs of myocardial ischemia); arterial pH greater than 7.25; and a patient that is able to initiate a spontaneous inspiratory effort.93
A study of 300 patients published in 1996 demonstrated the value of daily screening of patients by trials of spontaneous breathing. In the study, patients were assessed daily by the nurse and respiratory therapist, and if preset guidelines were met, patients underwent a 2-hour spontaneous breathing trial. If the patient passed the trial, the physician was notified, and the patient was extubated. The study demonstrated a decrease in ventilator days; a decrease in the number of complications, including reintubation; and lower hospital cost.94 Another study showed a decrease in the number of ventilator days and ICU days with daily weaning of sedation.95
The task force also addressed the use of noninvasive ventilation and prolonged ventilator dependence. Successful weaning was defined as 48 hours free of the ventilator; weaning as early as possible, using spontaneous breathing trials; avoidance of SIMV as a weaning mode; and use of PSV or AC after a failed weaning attempt. NIPPV should not routinely be used after failed extubation; it should be used only in patients with hypercapnia, although CPAP may be useful in preventing hypoxemia in postoperative patients (Table 48-8).96
In 1995, the Spanish Lung Failure Collaborative Group prospectively compared the use of intermittent mandatory ventilation (IMV), PSV, intermittent trials of spontaneous breathing, and once-daily spontaneous breathing trials. The study showed that spontaneous breathing trials in either form were superior to IMV or PSV in terms of time from initiation of weaning to successful extubation and probability of successful weaning (Fig 48-11).41
To illustrate the interaction of spontaneous breathing trials with daily interruption of sedation, the Awakening and Breathing Trial in 2008 showed that after randomizing 336 patients to the standard protocol or to daily discontinuation of sedation and daily spontaneous breathing trials, the intervention group had 3 fewer days on the mechanical ventilator, fewer ICU days, shorter hospital days, and improved survival rates compared with the standard protocol (Figs. 48-12 and 48-13).97 Numerous weaning parameters have been studied to hasten liberation from mechanical ventilation, but none have been shown to be superior to using clinical criteria combined with an algorithmic approach to discontinuation of sedation and ventilation.
VII Complications of Mechanical Ventilation
A Mechanical Complications
Mechanical ventilation may produce complications from use of an orotracheal or nasotracheal tube and complications of using positive-pressure ventilation. Common complications of artificial airways are laryngeal edema and irritation, tracheal stenosis, sinusitis, vocal cord damage, and paralysis. Complications of positive-pressure ventilation include barotrauma, which may lead to alveolar rupture and a continuum of pneumothorax, pneumomediastinum, and subcutaneous emphysema. The lungs are susceptible to alveolar distention due to high tidal volumes delivered by the ventilator to alveoli as a result of preset volumes. Due to the heterogenous nature of the lung, even lower volumes may be disproportionally delivered to open alveoli. Atelectotrauma, or cyclic atelectasis, is sheer-force trauma resulting from repeated opening and collapsing of the alveolus in response to positive-pressure ventilation (Fig. 48-14).
Volutrauma and atelectotrauma are within the spectrum of ill-defined entities of ventilator-associated lung injury (VALI), which appears to be more common in lungs of patients with ARDS or ALI. Although causation has not been determined, VALI has been observed in patients who have undergone mechanical ventilation. VILI is an entity well described in animal models. It is a syndrome of diffuse alveolar damage that is morphologically identical to ARDS and that is caused by mechanical ventilation.98 Alveolar injury results in increased permeability, loss of functional surfactant, release of cytokines, and alveolar collapse (Fig. 48-15). Other factors that may be associated with an increased risk for VALI include immunosuppression,99 high ventilator rates,100 supine body position, and hyperthermia.101
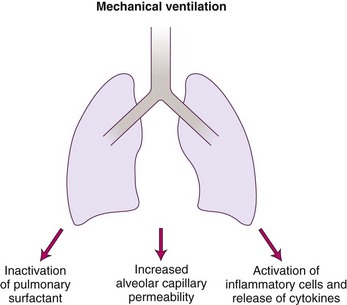
Figure 48-15 Downstream effects of lung injury from mechanical ventilation.
(From Papadokos PJ, Lachmann B: The open lung concept of mechanical ventilation: The role of recruitment and stabilization. Crit Care Clin 23:241–250, 2007.)
1 Auto-PEEP
Intrinsic PEEP or auto-PEEP results from incomplete alveolar emptying before the initiation of the next breath.102 The alveolar pressures remain positive relative to atmospheric pressures at end-expiration. High minute ventilation from high tidal volumes or respiratory rates is a common cause. When high respiratory rates exist, expiratory time may be decreased to a point at which the full tidal volume is not exhaled before the next breath. High tidal volumes are less likely to be exhaled entirely before the next breath. Patients with obstructive lung diseases such as COPD and asthma often have auto-PEEP while on mechanical ventilation due to disease-related limited expiratory flow.103 Expiratory resistance from any obstruction of the endotracheal tube (e.g., kinks, secretions) or patient-ventilator asynchrony can lead to auto-PEEP. Auto-PEEP can result from high tidal volumes, a high respiratory rate, or a decreased expiratory time relative to inhalation time in any disease state (Table 48-9).
Causes of Auto-PEEP | Factors |
---|---|
High minute ventilation | High tidal volume |
High respiratory rate | |
Expiratory flow limitation | Airway narrowing from bronchospasm, collapse, inflammation, or remodeling |
Expiratory resistance | Patient-ventilator asynchrony |
Narrow or obstructed endotracheal tube | |
Secretions |
PEEP, Positive end-expiratory pressure.
2 Consequences of Auto-PEEP
Auto-PEEP can decrease venous return, reduce ventricular compliance, and induce hypotension.104 Hypovolemic patients are at increased risk for PEEP-related hypotension. Alveolar distention from auto-PEEP can lead to barotrauma, worsening oxygenation from ventilation-perfusion () mismatch, and VALI. Auto-PEEP increases the work of breathing by raising the pressure the patient must generate to trigger a ventilator breath. If the breath is triggered at −2 cm H2O and the auto-PEEP is 6 cm H2O, the patient needs to overcome both (−8 cm H2O of negative pressure) to initiate a breath. Auto-PEEP increases peak and plateau pressures in volume-controlled modes and decreases tidal volume in pressure-cycled ventilation.24 Auto-PEEP also increases peak and plateau pressures, which can lead to an overestimation of thoracic compliance. Increased plateau pressures can be transmitted to the intrathoracic vessels and can lead to an overestimation of the central venous pressures and the pulmonary artery occlusion pressure (Table 48-10).
Affected System | Consequences |
---|---|
Cardiac |
CVP, Central venous pressure; PCWP, pulmonary capillary wedge pressure; PEEP, positive end-expiratory pressure.
Auto-PEEP can be monitored in several ways but sometimes can be difficult to detect. The graphs of flow versus time demonstrate initiation of a new breath before the expiration reaches zero flow. End expiratory alveolar pressure, measured by introducing an end-expiratory breath-hold and subtracting applied PEEP, can quantitate auto-PEEP. The breath-hold allows the pressure in the proximal airways to equilibrate with alveolar pressure. Auscultation for airflow at the end of expiration is also useful.105
Management of auto-PEEP is targeted at promoting alveolar emptying and increasing expiratory time. Reducing minute ventilation by targeting tidal volume or the respiratory rate, or both, and by increasing inspiratory flow can be effective measures in reducing auto-PEEP. Management of the underlying condition is important, especially for patients with obstructive lung diseases treated with bronchodilators, steroids, and antimicrobial therapies. In patients with COPD or asthma, the limited expiratory flow can be counterbalanced by application of extrinsic PEEP.106 Small amounts of extrinsic PEEP can decrease intrinsic PEEP by keeping the small airways open at end-expiration. However, the applied PEEP should be less than intrinsic PEEP to prevent an increase in alveolar pressures.107
B Infectious Complications
Ventilator-associated pneumonia (VAP), a type of hospital-acquired pneumonia (HAP), is defined as a diagnosis of pneumonia 48 to 72 hours after endotracheal intubation. Studies of management with noninvasive ventilation show a decreased incidence of pneumonia when endotracheal intubation is avoided.91 The earlier pneumonia is diagnosed after onset of mechanical ventilation, the better the prognosis. Pneumonia diagnosed later in the course of mechanical ventilation is more likely to be caused by a drug-resistant organism and carries a worse prognosis. Several practices can help to minimize the occurrence of pneumonia on the ventilator, including elevating the head of the bed to the semirecumbent position of 30 to 45 degrees, aggressive weaning of sedation, use of orotracheal and orogastric tubes to decrease the potential for sinusitis, daily assessments to liberate from mechanical ventilation, avoiding gastric overdistention, avoiding unplanned extubations, oral care with antiseptic solution, and limiting contamination of ventilator tubing.108,109 Once a VAP occurs, there is no difference in mechanical ventilation–free days, the length of ICU days, the number of organ failure–free days and mortality rates on day 60 between the groups receiving between 8 days and 15 days of treatment with appropriate antibiotics.110
IX Clinical Pearls
• The indications for mechanical ventilation include hypoxemic and hypercapnic respiratory failure, altered mentation with patient inability to protect the airway, hemodynamic instability, and to maintain adequate oxygenation and ventilation during deep sedation, anesthesia, or neuromuscular blockade.
• Positive end-expiratory pressure (PEEP) improves oxygenation in patients with hypoxic respiratory failure, optimizes alveolar recruitment and decreases cycles of recruitment and derecruitment of alveolar lung units.
• Assist-control ventilation (ACV) can be volume targeted, upon initiation of mechanical ventilation, or pressure targeted when increased oxygenation is required, but there is concern about increasing airway pressures.
• A lung-protective strategy should be employed in all patients with acute lung injury (ALI) or acute respiratory distress syndrome (ARDS). Using low tidal volumes of 6 mL/kg of predicted body weight with plateau pressures of 30 cm H2O or less has become the standard of care for patients with ARDS.
• Synchronized intermittent mandatory ventilation (SIMV) incorporates some characteristics of ACV and pressure-support ventilation (PSV). The purpose of the two types of breaths (mandatory and spontaneous) is to allow increased diaphragmatic activity and increased work of breathing when triggering spontaneous breaths.
• Airway pressure–release ventilation (APRV) uses high and low pressures to aid in recruitment of atelectatic lung units and allows spontaneous breathing. It can decrease airway pressures and improve oxygenation, alveolar recruitment, and cardiac output.
• High-frequency ventilation, sometimes called the oscillator, improves oxygenation by providing oxygen-rich and CO2-poor gas that rapidly mixes with sinusoidal flow at stroke volumes that approximate anatomic dead space. Each type of high-frequency ventilation—high-frequency jet ventilation, high-frequency oscillatory ventilation, and high-frequency percussive ventilation—has its subtleties and specific indications.
• Noninvasive positive-pressure ventilation (NIPPV) is useful in many situations, including exacerbations of COPD, cardiogenic pulmonary edema, and hypercapnic respiratory failure in patients with a Glasgow Coma Scale score of more than 10.
• During weaning from mechanical ventilation, daily interruption of sedation (unless contraindicated by severe hypoxemia or the need for neuromuscular blockade) and daily trials of spontaneous breathing should be employed for all patients.
All references can be found online at expertconsult.com.
12 Acute Respiratory Distress Syndrome Network. Ventilation with lower tidal volumes as compared with traditional tidal volumes for acute lung injury and the acute respiratory distress syndrome. N Engl J Med. 2000;342:1301–1308.
13 Gajic O, Dara SI, Mendez JL, et al. Ventilator associated lung injury in patients without acute lung injury at the onset of mechanical ventilation. Crit Care Med. 2004;32:1817–1824.
16 Amato MB, Barbas CS, Medeiros DM, et al. Effect of protective-ventilation strategy on mortality in the acute respiratory distress syndrome. N Engl J Med. 1998;338:347–354.
21 Brower RG, Lanken PN, MacIntyre N, et al. National heart, lung, and blood institute ARDS clinical trials network. Higher versus lower positive end-expiratory pressures in patients with the acute respiratory distress syndrome. N Engl J Med. 2004;351:327–336.
23 Briel M, Meade M, Mercat A, et al. Higher vs lower positive end-expiratory pressure in patients with acute lung injury and acute respiratory distress syndrome: Systematic review and meta-analysis. JAMA. 2010;303:865–873.
41 Esteban A, Frutos F, Tobin MJ, et al. A comparison of four methods of weaning patients from mechanical ventilation. Spanish Lung Failure Collaborative Group. N Engl J Med. 1995;332:345–350.
63 Derdak S, Mehta S, Stewart TE, et al. Multicenter oscillatory ventilation for acute respiratory distress syndrome trial (MOAT) study investigators. Am J Respir Crit Care Med. 2002;166:801–808.
87 Antonelli M, Conti G, Moro ML, et al. Predictors of failure of noninvasive positive pressure ventilation in patients with acute hypoxemic respiratory failure: A multi-center study. Intensive Care Med. 2001;27:1718–1728.
97 Girard TD, Kress JP, Fuchs BD, et al. Efficacy and safety of a paired sedation and ventilator weaning protocol for mechanically ventilated patients in intensive care (Awakening and Breathing Controlled trial): A randomised controlled trial. Lancet. 2008;371:126–134.
108 American Thoracic Society/Infectious Disease Society of America. Guidelines for the management of adults with hospital-acquired, ventilator-associated, and healthcare-associated pneumonia. Am J Respir Crit Care Med. 2005;171:388–416.
1 Esteban A, Anzueto A, Alia I, et al. How is mechanical ventilation employed in the intensive care unit? An international utilization review. Am J Respir Crit Care Med. 2000;161:1450–1458.
2 Plummer AL, Gracey DR. Consensus conference on artificial airways in patients receiving mechanical ventilation. Chest. 1989;96:178–180.
3 Gray A, Goodacre S, Newby DE, et al. Noninvasive ventilation in acute cardiogenic pulmonary edema. for the 3CPO Trialists. N Engl J Med. 2008;359:142–151.
4 Ram FS, Wellington S, Rowe B, et al. Non-invasive positive pressure ventilation for treatment of respiratory failure due to sever acute exacerbations of chronic obstructive pulmonary disease. Cochrane Data-base Syst Rev. 3, 2005. CD004360
5 Winck JC, Azevedo LF, Costa-Pereira A, et al. Efficacy and safety of non-invasive ventilation in the treatment of acute cardiogenic pulmonary edema—A systematic review and meta-analysis. Crit Care. 2006;10:R69–R87.
6 Dreyfuss D, Saumon G. Ventilator induced lung injury: Lessons from experimental studies. Am J Respir Crit Care Med. 1998;157:294–323.
7 International Consensus Conferences in Intensive Care Medicine. Ventilator-associated lung injury in ARDS. Am J Respir Crit Care Med. 1999;160:2118–2124.
8 Nieszkowska A, Lu Q, Vieira S, et al. Incidence and regional distribution of lung overinflation during mechanical ventilation with positive end-expiratory pressure. Crit Care Med. 2004;32:1496–1503.
9 Bilek AM, Dee KC, Gaver DP, 3rd. Mechanisms of surface-tension-induced epithelial cell damage in a model of pulmonary airway reopening. J Appl Physiol. 2003;94:770–783.
10 Muscedere JG, Mullen JB, Gan K, et al. Tidal ventilation at low airway pressures can augment lung injury. Am J Respir Crit Care Med. 1994;149:1327–1334.
11 Mead J, Takishima T, Leith D. Stress distribution in lungs: A model of pulmonary elasticity. J Appl Physiol. 1970;28:596–608.
12 Acute Respiratory Distress Syndrome Network. Ventilation with lower tidal volumes as compared with traditional tidal volumes for acute lung injury and the acute respiratory distress syndrome. N Engl J Med. 2000;342:1301–1308.
13 Gajic O, Dara SI, Mendez JL, et al. Ventilator associated lung injury in patients without acute lung injury at the onset of mechanical ventilation. Crit Care Med. 2004;32:1817–1824.
14 Mercat A, Richard JC, Vielle B, et al. Expiratory pressure (express) study group: Positive end-expiratory pressure setting in adults with acute lung injury and acute respiratory distress syndrome: A randomized controlled trial. JAMA. 2008;299:646–655.
15 Manzano F, Fernández-Mondéjar E, Colmenero M, et al. Positive-end expiratory pressure reduces incidence of ventilator-associated pneumonia in nonhypoxemic patients. Crit Care Med. 2008;36:2225–2231.
16 Amato MB, Barbas CS, Medeiros DM, et al. Effect of protective-ventilation strategy on mortality in the acute respiratory distress syndrome. N Engl J Med. 1998;338:347–354.
17 Talmor D, Sarge T, Malhotra A, et al. Mechanical ventilation guided by esophageal pressure in acute lung injury. N Engl J Med. 2008;359:2095.
18 Downs JB, Klein EF, Modell JH. The effect of incremental PEEP on PaO2 in patients with respiratory failure. Anesth Analg. 1973;52:210–215.
19 Tugrul S, Akinci O, Ozcan PE, et al. Effects of sustained inflation and post-inflation positive end expiratory pressure in acute respiratory distress syndrome: Focusing on pulmonary and extrapulmonary forms. Crit Care Med. 2003;31:738–744.
20 Good JT, Jr., Wolz JF, Anderson JT, et al. The routine use of positive end-expiratory pressure after open heart surgery. Chest. 1979;76:397–400.
21 Brower RG, Lanken PN, MacIntyre N, et al. National heart, lung, and blood institute ARDS clinical trials network. Higher versus lower positive end-expiratory pressures in patients with the acute respiratory distress syndrome. N Engl J Med. 2004;351:327–336.
22 Meade MO, Cook DJ, Guyatt GH, et al. Lung open ventilation study investigators: Ventilation strategy using low tidal volumes, recruitment maneuvers, and high positive end-expiratory pressure for acute lung injury and acute respiratory distress syndrome: A randomized controlled trial. JAMA. 2008;299:637–645.
23 Briel M, Meade M, Mercat A, et al. Higher vs lower positive end-expiratory pressure in patients with acute lung injury and acute respiratory distress syndrome: Systematic review and meta-analysis. JAMA. 2010;303:865–873.
24 Hess D, Kacmarek RM. Essentials of mechanical ventilation, ed 2. New York: McGraw-Hill; 2002.
25 Sackner MA, Landa J, Hirsch J, Zapata A. Pulmonary effects of oxygen breathing. A 6-hr study in normal men. Ann Intern Med. 1975;82:40–43.
26 Tryka AF, Skornik WA, Godleski JJ, et al. Potentiation of bleomycin-induced lung injury by exposure to 70% oxygen. Morphologic assessment. Am Rev Respir Dis. 1982;126:1074–1079.
27 Slutsky AS. Mechanical ventilation. American College of Chest Physicians’ Consenseus Conference. Chest. 1993;104:1833–1859.
28 Petersen GW, Baier H. Incidence of pulmonary barotrauma in a medical ICU. Crit Care Med. 1983;11:67–69.
29 Weg JG, Anzueto A, Balk RA, et al. The relation of pneumothorax and other air leaks to mortality in the acute respiratory distress syndrome. N Engl J Med. 1998;338:341–346.
30 Boussarsar M, Thierry G, Jaber S, et al. Relationship between ventilatory settings and barotrauma in the acute respiratory distress syndrome. Intensive Care Med. 2002;28:406–413.
31 Marini JJ, Rodrigues RM, Lamb V. The inspiratory workload of patient-initiated mechanical ventilation. Am Rev Respir Dis. 1986;134:902–909.
32 Connors AF, Jr., McCaffree DR, Gray BA. Effect of inspiratory flow rate on gas exchange during mechanical ventilation. Am Rev Respir Dis. 1981;124:537–543.
33 Pepe PE, Marini JJ. Occult positive end expiratory pressure in mechanically ventilated patients with airflow obstruction. Am Rev Respir Dis. 1982;126:166–179.
34 Al-Saady N, Bennett ED. Decelerating inspiratory flow waveform improves lung mechanics and gas exchange in patients on intermittent positive-pressure ventilation. Intensive Care Med. 1985;11:68–75.
35 Marini JJ. Point: Is pressure assist-control preferred over volume assist-control mode for lung protective ventilation in patients with ARDS? Yes. Chest. 2011;140:286–290.
36 Fan E, Needham DM, Stewart TE. Ventilatory management of acute lung injury and acute respiratory distress syndrome. JAMA. 2005;294:2889–2896.
37 Ware LB, Matthay MA. The acute respiratory distress syndrome. N Engl J Med. 2000;342:1334–1349.
38 Wheeler AP, Bernard GR. Acute lung injury and the acute respiratory distress syndrome: A clinical review. Lancet. 2007;369:1553–1565.
39 Petrucci N, Iacovelli W. Ventilation with lower tidal volumes versus traditional tidal volumes in adults for acute lung injury and acute respiratory distress syndrome. Cochrane Database Syst Rev. 2, 2004. CD003844
40 Putensen C, Theuerkauf N, Zinserling J, et al. Meta-analysis: Ventilation strategies and outcomes of the acute respiratory distress syndrome and acute lung injury. Ann Intern Med. 2009;151:566–576.
41 Esteban A, Frutos F, Tobin MJ, et al. A comparison of four methods of weaning patients from mechanical ventilation. Spanish Lung Failure Collaborative Group. N Engl J Med. 1995;332:345–350.
42 Groeger JS, Levinson MR, Carlon GC. Assist control versus synchronized intermittent mandatory ventilation during acute respiratory failure. Crit Care Med. 1989;17:607–612.
43 MacIntyre NR. Respiratory function during pressure support ventilation. Chest. 1986;89:677–683.
44 Banner MJ, Kirby RR, Blanch PB, et al. Decreasing imposed work of the breathing apparatus to zero using pressure-support ventilation. Crit Care Med. 1993;21:1333–1338.
45 Chiumello D, Pelosi P, Calvi E, et al. Different modes of assisted ventilation in patients with acute respiratory failure. Eur Respir J. 2002;20:925–933.
46 Abraham E, Yoshihara G. Cardiorespiratory effects of pressure controlled inverse ratio ventilation in severe respiratory failure. Chest. 1989;96:1356–1359.
47 Gurevitch MJ, Van Dyke J, Young ES, et al. Improved oxygenation and lower peak airway pressure in severe adult respiratory distress syndrome. Treatment with inverse ratio ventilation. Chest. 1986;89:211–213.
48 Tharratt RS, Allen RP, Albertson TE. Pressure controlled inverse ratio ventilation in severe adult respiratory failure. Chest. 1988;94:755–762.
49 Lain DC, DiBenedetto R, Morris SL, et al. Pressure control inverse ratio ventilation as a method to reduce peak inspiratory pressure and provide adequate ventilation and oxygenation. Chest. 1989;95:1081–1088.
50 Mercat A, Diehl JL, Michard F, et al. Extending inspiratory time in acute respiratory distress syndrome. Crit Care Med. 2001;29:40–44.
51 Shanholtz C, Brower R. Should inverse ratio ventilation be used in adult respiratory distress syndrome? Am J Respir Crit Care Med. 1994;149:1354–1358.
52 Downs JB, Stock MC. Airway pressure release ventilation: A new concept in ventilatory support. Crit Care Med. 1987;15:459–461.
53 Stock MC, Downs JB, Frolicher DA. Airway pressure release ventilation. Crit Care Med. 1987;15:462–466.
54 Myers TR, MacIntyre NR. Does airway pressure release ventilation offer important new advantages in mechanical ventilator support? Respir Care. 2007;50:452–458.
55 Putensen C, Mutz NJ, Putensen-Himmer G, et al. Spontaneous breathing during ventilator support improves ventilation-perfusion distributions in patients with acute respiratory distress syndrome. Am J Respir Crit Care Med. 1999;159:1241–1248.
56 Cane RD, Peruzzi WT, Shapiro BA. Airwary pressure release ventilation in severe acute respiratory failure. Chest. 1991;100:460–463.
57 Neumann P, Golisch W, Strohmeyer A, et al. Influence of different release times on spontaneous breathing pattern during airway pressure release ventilation. Intensive Care Med. 2002;28:1742–1749.
58 Räsänen J, Cane RD, Downs JB, et al. Airway pressure release ventilation during acute lung injury: A prospective multicenter trial. Crit Care Med. 1991;19:1234–1241.
59 Varpula T, Valta P, Niemi R, et al. Airway pressure release ventilation as a primary ventilatory mode in acute respiratory distress syndrome. Acta Anaesthesiol Scand. 2004;48:722–731.
60 Rose L, Hawkins M. Airway pressure release ventilation and biphasic positive airway pressure: A systematic review of definitional criteria. Intensive Care Med. 2008;34:1766–1773.
61 Chang HK. Mechanisms of gas transport during ventilation by high-frequency oscillation. J Appl Physiol. 1984;56:553–563.
62 Bollen CW, van Well GT, Sherry T, et al. High frequency oscillatory ventilation compared with conventional mechanical ventilation in adult respiratory distress syndrome: A randomized controlled trial. Crit Care. 2005;9:R430R439.
63 Derdak S, Mehta S, Stewart TE, et al. High-frequency oscillatory ventilation for acute respiratory distress syndrome in adults: A randomized, controlled trial. Multicenter Oscillatory Ventilation for Acute Respiratory Distress Syndrome Trial (MOAT) Study Investigators. Am J Respir Crit Care Med. 2002;166:801–808.
64 Mentzelopoulos SD, Roussos C, Koutsoukou A, et al. Acute effects of combined high-frequency oscillation and tracheal gas insufflation in severe acute respiratory distress syndrome. Crit Care Med. 2007;35:1500–1508.
65 Galvin I, Krishnamoorthy R, Saad RSG. Management of advanced ARDS complicated by bilateral pneumothoraces with high frequency oscillatory ventilation in an adult. Br J Anaesth. 2004;93:454–456.
66 Standiford TJ, Morganroth ML. High-frequency ventilation. Chest. 1989;96:1380–1389.
67 Salim A, Martin M. High-frequency percussive ventilation. Crit Care Med. 2005;33(Suppl):S241–S245.
68 Reper P, Wibaux O, Van Laeke P, et al. High frequency percussive ventilation and conventional ventilation after smoke inhalation: A randomised study. Burns. 2002;28:503–508.
69 Chung KK, Wolf SE, Renz EM, et al. High-frequency percussive ventilation and low tidal volume ventilation in burns: A randomized controlled trial. Crit Care Med. 2010;38:1970–1977.
70 American Thoracic Society, The European Respiratory Society, The European Society of Intensive Care Medicine, and The Societe De Reanimation De Langue Francaise Intensive Care Medicine. : Noninvasive positive pressure ventilation in acute respiratory failure. Am J Respir Crit Care Med. 2001;163:283–291.
71 British Thoracic Society Standards of Care Committee. Non-invasive ventilation in acute respiratory failure. Thorax. 2002;57:192–211.
72 Keenan SP, Sinuff T, Burns KE, et al. Clinical practice guidelines for the use of noninvasive positive-pressure ventilation and noninvasive continuous positive airway pressure in the acute care setting. CMAJ. 2011;183:E195–E214.
73 Brochard L, Mancebo J, Wysocki M, et al. Noninvasive ventilation for acute exacerbations of chronic obstructive pulmonary disease. N Engl J Med. 1995;333:817–822.
74 Keenan SP, Sinuff T, Cook DJ, et al. Which patients with acute exacerbation of chronic obstructive pulmonary disease benefit from noninvasive positive-pressure ventilation? A systematic review of the literature. Ann Intern Med. 2003;138:861–870.
75 Lightowler J, Wedzicha J, Elliott M, et al. Non-invasive positive pressure ventilation to treat respiratory failure resulting from exacerbations of chronic obstructive pulmonary disease: Cochrane systematic review and meta-analysis. BMJ. 2003;326:185–190.
76 Bersten AD, Holt AW, Vedig AE, et al. Treatment of severe cardiogenic pulmonary edema with continuous positive airway pressure delivered by face mask. N Engl J Med. 1991;325:1825–1830.
77 Mehta S, Al-Hashim AH, Keenan SP. Noninvasive ventilation in patients with acute cardiogenic pulmonary edema. Respir Care. 2009;54:186–195.
78 Nava S, Carbone G, DiBattista N, et al. Noninvasive ventilation in cardiogenic pulmonary edema. A multicenter randomized trial Am J Respir Crit Care Med. 2003;168:1432–1437.
79 Vital FM, Saconato H, Ladeira MT, et al. Non-invasive positive pressure ventilation (CPAP or bilevel NPPV) for cardiogenic pulmonary edema. Cochrane Database Syst Rev. 3, 2008. CD005351
80 Holland AE, Denehy L, Buchan CA, et al. Efficacy of a heated passover humidifier during noninvasive ventilation: A bench study. Respir Care. 2007;52:38–44.
81 Girault C, Richard JC, Chevron V, et al. Comparative physiologic effects of noninvasive assist-control and pressure support ventilation in acute hypercapnic respiratory failure. Chest. 1997;111:1639–1649.
82 Fernández-Vivas M, Caturla-Such J, González de la Rosa J, et al. Noninvasive pressure support versus proportional assist ventilation in acute respiratory failure. Intensive Care Med. 2003;29:1126–1133.
83 Nouira S, Boukef R, Bouida W, et al. Non-invasive pressure support ventilation and CPAP in cardiogenic pulmonary edema: A multicenter randomized study in the emergency department. Intensive Care Med. 2011;37:249–256.
84 Peter JV, Moran JL, Phillips-Hughes J, et al. Effect of non-invasive positive pressure ventilation (NIPPV) on mortality in patients with acute cardiogenic pulmonary oedema: A meta-analysis. Lancet. 2006;367:1155–1163.
85 Wysocki M, Richard JC, Meshaka P. Noninvasive proportional assist ventilation compared with noninvasive pressure support ventilation in hypercapnic acute respiratory failure. Crit Care Med. 2002;30:323–329.
86 Antón A, Güell R, Gómez J, et al. Predicting the result of noninvasive ventilation in severe acute exacerbations of patients with chronic airflow limitation. Chest. 2000;117:828–833.
87 Antonelli M, Conti G, Moro ML, et al. Predictors of failure of noninvasive positive pressure ventilation in patients with acute hypoxemic respiratory failure: A multi-center study. Intensive Care Med. 2001;27:1718–1728.
88 Masip J, Roque M, Sánchez B, et al. Noninvasive ventilation in acute cardiogenic pulmonary edema: systematic review and meta-analysis. JAMA. 2005;294:3124–3130.
89 Fernández MM, Villagrá A, Blanch L, et al. Non-invasive mechanical ventilation in status asthmaticus. Intensive Care Med. 2001;27:486–492.
90 Meduri GU, Cook TR, Turner RE, et al. Noninvasive positive pressure ventilation in status asthmaticus. Chest. 1996;110:767–774.
91 Nava S, Ambrosino N, Clini E, et al. Noninvasive mechanical ventilation in the weaning of patients with respiratory failure due to chronic obstructive pulmonary disease. Ann Intern Med. 1998;128:721–728.
92 Ferrer M, Valencia M, Nicolas JM, et al. Early noninvasive ventilation averts extubation failure in patients at risk: A randomized trial. Am J Respir Crit Care Med. 2006;173:164–170.
93 A Collective Task Force Facilitated by the American College of Chest Physicians, the American Association of Respiratory Care; and the American College of Critical Care Medicine. Evidence-based guidelines for weaning and discontinuing ventilatory support. Chest. 2001;120:375S–395S.
94 Ely EW, Baker AM, Dunagan DP, et al. Effect on the duration of mechanical ventilation of identifying patients capable of breathing spontaneously. N Engl J Med. 1996;335:1864–1869.
95 Kress JP, Pohlman AS, O’Connor MF, et al. Daily interruption of sedative infusions in critically ill patients undergoing mechanical ventilation. N Engl J Med. 2000;342:1471–1477.
96 Boles J-M, Bion J, Connors A, et al. Statement of the Sixth International Consensus Conference on Intensive Care Medicine. Weaning from mechanical ventilation. Eur Respir J. 2007;29:1033–1056.
97 Girard TD, Kress JP, Fuchs BD, et al. Efficacy and safety of a paired sedation and ventilator weaning protocol for mechanically ventilated patients in intensive care (Awakening and Breathing Controlled trial): A randomised controlled trial. Lancet. 2008;371:126–134.
98 Dos Santos CC, Slutsky SS. Mechanisms of ventilator-induced lung injury: A perspective. J Appl Physiology. 2000;89:1645–1655.
99 Kawano T, Mori S, Cybulsky M, et al. Effect of granulocyte depletion in a ventilated surfactant-depleted lung. J Appl Physiol. 1987;62:27–33.
100 Hotchkiss JR, Blanch LL, Murias G, et al. Effects of decreased respiratory frequency on ventilator induced lung injury. Am J Respir Crit Care Med. 2000;161:463–468.
101 Suzuki S, Hochkiss JR, Takahashi T, et al. Effect of core body temperature on ventilator-induced lung injury. Crit Care Med. 2004;32:144–149.
102 Armaganidis A, Stavrakaki-Kallergi K, Koutsoukou A, et al. Intrinsic positive end-expiratory pressure in mechanically ventilated patients with and without tidal expiratory flow limitation. Crit Care Med. 2000;28:3837.
103 Tuxen DV. Detrimental effects of positive end-expiratory pressure during controlled mechanical ventilation of patients with severe airflow obstruction. Am Rev Respir Dis. 1989;140:5–9.
104 Takata M, Robotham JL. Ventricular external constraint by the lung and pericardium during positive end-expiratory pressure. Am Rev Respir Dis. 1991;143:872–875.
105 Kress JP, O’Connor MF, Schmidt GA. Clinical examination reliably detects intrinsic positive end-expiratory pressure in critically ill, mechanically ventilated patients. Am J Respir Crit Care Med. 1999;159:290–294.
106 Smith TC, Marini JJ. Impact of PEEP on lung mechanics and work of breathing in severe airflow obstruction. J Appl Physiol. 1988;65:1488–1499.
107 Ranieri VM, Giuliani R, Cinnella G, et al. Physiologic effects of positive end-expiratory pressure in patients with chronic obstructive pulmonary disease during acute ventilatory failure and controlled mechanical ventilation. Am Rev Respir Dis. 1993;147:5–13.
108 American Thoracic Society/Infectious Disease Society of America. Guidelines for the management of adults with hospital-acquired, ventilator-associated, and healthcare-associated pneumonia. Am J Respir Crit Care Med. 2005;171:388–416.
109 Coffin SE, Klompas M, Classen D, et al. Strategies to prevent ventilator-associated pneumonia in acute care hospitals. Infect Control Hosp Epidemiol. 2008;29(Suppl 1):S31–S40.
110 Chastre J, Wolff M, Fagon J-Y, et al. Comparison of 8 vs 15 days of antibiotic therapy for ventilator-associated pneumonia in adults. JAMA. 2003;290:2588–2598.
111 Brochard L, Roudot-Thoraval E, Roupie E, et al. Tidal volume reduction for prevention of ventilator-induced lung injury in the acute respiratory distress syndrome. The Multicenter Trial Group on tidal volume reduction in ARDS. Am J Respir Crit Care Med. 1998;158:1831–1838.
112 Brower R, Shanholtz C, Shade D, et al. Prospective randomized, controlled trial comparing traditional versus reduced tidal volume ventilation in acute respiratory distress syndrome patients. Crit Care Med. 1999;27:1492–1498.
113 Stewart TE, Meade MO, et al. Evaluation of a ventilation strategy to prevent barotrauma in patients with high risk for acute respiratory distress syndrome. Pressure- and Volume-Limited Ventilation Strategy Group. N Engl J Med. 1998;338:355–361.