Kidney Anatomy and Physiology
Macroscopic Anatomy
The kidneys are paired organs located retroperitoneally, one on each side of the vertebral column between T12 and L3.1 The right kidney is slightly lower than the left because of the position of the liver. Each kidney is approximately 12 centimeters (cm) long, 6 cm wide, and 2.5 cm thick in the adult. Kidney size and weight varies between men and women; 125 to 170 grams in men, and 115 to 155 grams in women.1 The kidneys are protected anteriorly and posteriorly by the rib cage and by a tough fibrous capsule that encloses each kidney. Additional protection is provided by a cushion of perirenal fat and the support of the kidney fascia.
Internally, the kidneys are made up of two distinct areas: the cortex and the medulla. The kidney cortex is the outer layer and contains the glomeruli, proximal tubules, cortical portions of the loops of Henle, distal tubules, and cortical collecting ducts. The kidney cortex is about 1 cm in thickness. The kidney medulla is the inner kidney layer, made up of the pyramids, which contain the medullary portions of the loops of Henle, the vasa recta, and the medullary portions of the collecting ducts. Numerous pyramids taper and join to form a minor calyx; several minor calyces join to form a major calyx. The major calyces then join and enter the funnel-shaped kidney pelvis, a 5- to 10-mL conduit that directs urine into the ureter (Fig. 25-1).
The kidney system also includes the urinary drainage system—the ureters, bladder, and urethra (Fig. 25-2). The ureters are fibromuscular tubes that exit the central part of the kidney pelvis. The ureters are 28 to 34 cm in length and enter the urinary bladder at an oblique angle. As urine is formed by the kidneys, the urine flows through the ureters by peristalsis. The peristaltic action of the ureters and the angle at which the ureters enter the bladder help prevent reflux of urine from the bladder back up into the kidneys. The bladder is a muscular sac within the pelvis and has a capacity of 280 to 500 mL. Urine leaves the bladder through the urethral orifice and is excreted from the body through the urethra. The male urethra is about 20 cm long; the female urethra is 3 to 5 cm long.
Vascular Anatomy
The kidneys are highly vascular and receive up to 20% of the cardiac output—about 1 liter to 1.2 L/min of blood flow.2 Blood enters the kidneys through the renal arteries, which branch bilaterally from the abdominal aorta. The renal artery divides into arterial branches that become progressively smaller vessels, eventually ending with the afferent arterioles. A single afferent arteriole supplies blood to each glomerulus, a tuft of capillaries that is the first structure of the nephron. The nephron is often described as the functional unit of the kidneys.1
Blood exits the glomerulus by the efferent arteriole, which connects with the peritubular capillary network, also known as the vasa recta (straight vessels), that parallel the long loops of Henle. The intricate capillary network maintains the intracapillary pressure that allows water and solutes to move between the tubules and the capillaries for urine formation and the concentration and dilution of urine. The capillaries then rejoin and form gradually enlarging venous vessels, until the blood leaves each kidney through the renal vein and returns to the general circulation by the inferior vena cava.2
Microscopic Structure and Function
Each kidney is made up of about one million nephrons, the functional units of the kidneys. Because of the vast number of nephrons, the kidneys can continue to function even when several thousand nephrons are damaged or destroyed by disease or injury. Each nephron has the ability to perform all of the individual functions of the kidneys. The nephron is made up of several distinct structures: the glomerulus, the Bowman capsule, the proximal tubule, the loop of Henle, the distal tubule, and the collecting duct (Fig. 25-3).
Glomerulus
The first structure of each nephron is the glomerulus, a high-pressure capillary bed that serves as the filtering point for the blood. Positive filtration pressure in the glomerulus is achieved as a result of the high arterial pressure as the blood enters the afferent arteriole and the resistance created by the smaller efferent arteriole as the blood exits the glomerulus. As a result of the positive-pressure gradient, fluid and solutes are filtered through the glomerular capillary walls. The glomerulus has three layers: the endothelium, the basement membrane, and the epithelium. The inner endothelial layer lines the glomerulus and contains numerous pores that allow filtration of fluid and small solutes from the blood. The middle basement membrane layer also controls filtration according to the size, electrical charge, protein-binding capability, and shape of the molecules. This complex is also described as the glomerular filtration barrier (GFB). It is freely permeable to water and small or midsized molecules but large molecules such as albumin and red blood cells are prevented from entering the filtrate.3 The presence of large molecules in the urine is a signal that the glomerular membrane is damaged. The outer epithelium layer contains pores that allow the filtered blood, or filtrate, into the Bowman space.
The Bowman Capsule
A filtrate of plasma, usually called the ultrafiltrate, enters the Bowman space, which is surrounded by the Bowman capsule, a tough, membranous layer of epithelial cells that completely surrounds the glomerular capillary bed. The Bowman space is located between the capillary walls of the glomerulus and the inner layer of the Bowman capsule and contains the initial filtrate from the blood. Fluid, solutes, and other substances filtered by the glomerulus collect in the space. The Bowman space is a continuous structure that joins the first portion of the nephron’s tubular system—the proximal tubule.1
Proximal Tubule
The proximal tubule is located in the cortex of the kidney and has a large surface area available for solute and fluid transportation. The proximal tubule resorbs (takes back) most of the filtered water and sodium and many of the solutes the body does not routinely excrete in the urine. Solutes that are usually resorbed include all of the glucose, some of the water-soluble vitamins, most phosphate and bicarbonate, and much of the potassium, chloride, and calcium that is filtered by the glomerulus. Proteins are resorbed in the proximal tubule by two specialized receptors known as megalin and cubilin that bind albumin and vitamin-binding proteins.1 Creatinine is minimally resorbed and is excreted in the urine.
In addition to its major role in resorbing water and solutes from the filtrate, the proximal tubule secretes organic anions and cations into the tubular lumen. Ammonia is produced from the metabolism of glutamine in the mitochondria of the proximal tubule cells, where ammonia (NH3) combines with hydrogen (H+) to form ammonium (NH4+), which is secreted into the proximal tubule lumen.1
Collecting Duct
Several distal tubules join to form a collecting duct that begins in the cortex and extends through the medulla to empty into the papilla. The final composition of the urine occurs in the collecting duct, primarily because of the transport of potassium, sodium, and water. Water permeability is determined by the absence or presence of ADH. In the absence of, or with small amounts of ADH, the urine becomes dilute, whereas larger amounts of ADH result in concentrated urine. The filtrate usually is more concentrated when it leaves the collecting duct than it was when it entered. Acidification of the urine is accomplished by the transport of bicarbonate and hydrogen in the collecting duct. Several collecting ducts then combine to form the pyramids. After the urine leaves the collecting ducts, no change in the composition of the filtrate occurs. Box 25-1 summarizes tubular resorption and secretion in the various structures of the nephron.
Urine Formation
Glomerular Filtration
The first process in urine formation is glomerular filtration, which depends on glomerular blood flow, pressure in the Bowman space, and plasma oncotic pressure.2 Glomerular blood flow is the most important of these three factors and is maintained through an autoregulatory mechanism within the kidneys.2 The autoregulatory mechanism maintains consistent kidney blood flow and perfusion at a constant level as long as the mean arterial pressure (MAP) remains between 80 and 180 mm Hg. The afferent and efferent arterioles of the glomeruli have the ability to increase or decrease the glomerular blood flow rate through selective dilation and constriction. When the mean arterial blood pressure is decreased, the afferent arteriole dilates, and the efferent arteriole constricts to maintain a higher pressure in the glomerular capillary bed and maintain the GFR at 125 mL/min. The ability of the kidneys to autoregulate blood flow begins to fail when the mean arterial blood pressure is less than 80 mm Hg or greater than 180 mm Hg.
Tubular Resorption
Active Transport
The threshold concentration of a substance is important in active transport. The threshold of a substance is the plasma level of a substance at which none of the substance appears in the urine.2 When the threshold of a substance in the plasma is exceeded, progressively larger amounts of the substance appear in the urine because the large amounts cannot be resorbed. For example, the serum threshold concentration for glucose is about 180 mg/dL. At or below a plasma glucose concentration of 180 mg/dL, all glucose is actively resorbed from the kidney tubules back into the circulation, and none is excreted in the urine. When the plasma glucose concentration is above 180 mg/dL, the threshold concentration is exceeded, and some of the glucose cannot be resorbed from the tubules and is excreted in the urine.
Functions of the Kidneys
Blood Pressure Regulation
The kidneys regulate arterial blood pressure by maintaining the circulating blood volume by means of fluid balance and by altering peripheral vascular resistance through the renin-angiotensin-aldosterone system (RAAS). Regulation by the RAAS occurs in the juxtaglomerular apparatus (JGA), a group of specialized cells located around the afferent arteriole where the distal tubule and afferent arteriole make contact4,5 (see Fig. 25-3). Another group of specialized cells is located near the distal tubule; known as the macula densa, these cells control a feedback mechanism from the distal tubule to the afferent arteriole to control blood flow through the afferent arteriole.4
The JGA synthesizes, stores, and releases renin.5 Renin is released in response to reduced pressure in the glomerulus, sympathetic stimulation of the kidneys, and a decrease in the amount of sodium in the distal tubule.5 Renin enters the lumen of the afferent arteriole and is released into the general circulation. Renin is then converted to angiotensin I, which is further converted to angiotensin II as the blood circulates through the lungs. Angiotensin II is an active compound that causes afferent and efferent arteriole vasoconstriction, resulting in an increased vascular resistance, and it therefore maintains hydrostatic pressure within the kidneys. A powerful vasoconstrictor, angiotensin II also causes increased systemic vascular resistance and therefore increased arterial blood pressure. Intracellular actions of angiotensin II constitute an emerging area of research.6,7 Angiotensin II also stimulates the release of aldosterone by the adrenal cortex.
Aldosterone acts on the distal tubule to facilitate sodium and water resorption, resulting in an expanded circulating blood volume and increased blood pressure. When the arterial blood pressure increases, the JGA reduces the release of renin, and the RAAS is less active. Figure 25-4 summarizes the major aspects of the renin-angiotensin-aldosterone mechanism.
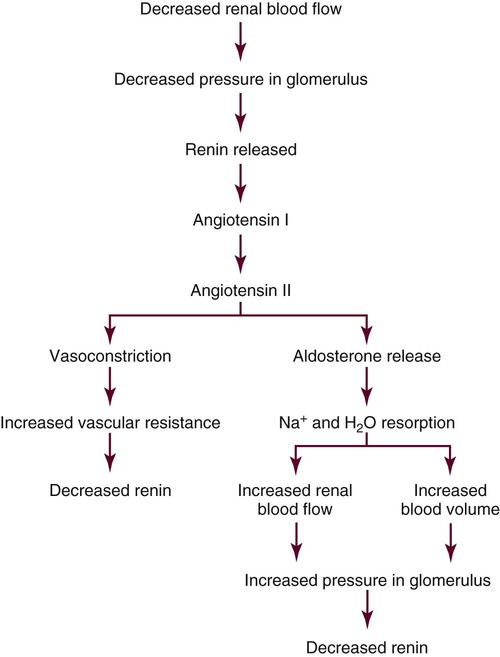
Erythrocyte Production
The kidneys secrete erythropoietin, the hormone that controls erythrocyte (red blood cell) production in the bone marrow. Erythropoietin is released in response to a decrease in the amount of oxygen delivered to the kidneys, such as in anemia or prolonged hypoxia.8 The hormone remains active for about 24 hours after release and stimulates the bone marrow to increase the production of erythrocytes. The absence of erythropoietin, which occurs in individuals with kidney failure, results in a profound anemia that is treated by administering synthetic erythropoietin or by blood transfusion therapy.8,9
Vitamin D Activation
The kidneys convert vitamin D from food sources into an active form for use by the body. Active vitamin D stimulates the absorption of calcium by the intestine and resorption of calcium by the tubules so that calcium is available for bone and tooth metabolism and blood clotting functions. When the kidneys fail, the body is unable to convert dietary vitamin D to its active form, calcium is poorly absorbed, and bone disease and other immunologic deficiencies result.10 Lack of vitamin D may also be responsible for changes in the immune system that increase the risk of infection in patients with chronic kidney disease (CKD).11
Prostaglandin Synthesis
Prostaglandins are vasoactive substances that dilate or constrict the arteries. The kidney produces two vasodilatory prostaglandins (PGs): E and I; they are typically abbreviated PGE1 and PGI2.12 The prostaglandins produced by the kidneys have only local blood flow effects with minimal or no systemic effects. The primary prostaglandins produced by the kidneys are the vasodilators PGE1 and PGI2, which act on the afferent arteriole to maintain blood flow and glomerular perfusion and filtration. The vasodilating effects of the prostaglandins also counteract the effects of angiotensin II and the sympathetic nervous system on the kidneys and maintain blood flow to the kidney despite systemic vasoconstriction. Another prostaglandin that may affect kidney function is PGF2, which contributes to vasoconstriction in times of volume depletion. Box 25-2 lists the effects of prostaglandins.
Fluid Balance
Fluid Compartments
Between 45% and 60% of body weight is made up of water.13 The body has two main fluid compartments: intracellular and extracellular. The intracellular compartment is the fluid inside each of the body’s cells, and it accounts for 40% of the total body water content.13 The remaining fluid is outside the body’s cells and makes up the extracellular compartment. The extracellular compartment is composed of two distinct subcompartments: intravascular and interstitial. The intravascular compartment, meaning the fluid within the blood vessels, accounts for 5% of the body water. The interstitial compartment corresponds to the fluid in the tissue spaces outside of the body cells and the blood vessels and accounts for 15% of body water. Approximate amounts of fluid contained in each compartment are shown in Figure 25-5A.
Electrolytes
Electrolytes are elements or compounds that, when dissolved in water, dissociate into ions, electrically charged atomic particles. Ions in solution in the fluid allow the fluid to conduct an electrical current. A balance exists between cations (positively charged ions), anions (negatively charged ions), and other substances in the fluid compartments. Maintaining this balance is important to the normal function of all body systems. Electrolytes exist in differing amounts in each of the fluid compartments. The primary electrolytes and other substances of importance in fluid and electrolyte balance are shown by fluid compartment in Figure 25-5B.
Fluid Physiology
Tonicity
The terms isotonic, hypotonic, and hypertonic all refer to tonicity, or the osmolality of body fluids. Osmolality is a measure of the number of particles (solute) in a solution, and the value is stated in milliosmoles per kilogram (mOsm/kg) of water. The normal osmolality of body fluids is 275 to 295 mOsm/kg of body weight. Different hospital laboratories may use slightly different numbers, such as 280 to 300 mOsm/kg.13
Figure 25-6 shows the effects of the tonicity (osmolality) of fluid in the body. An isotonic solution has roughly the same concentration of particles as the blood plasma; cells within an isotonic solution maintain consistency and do not lose or gain fluid to their surroundings. A hypertonic solution contains a greater concentration of particles than that inside the cell and causes fluid to be drawn out of the cells. Used inappropriately, too much fluid may be withdrawn, causing a withering of the cell (crenation). A hypotonic solution contains a lesser concentration of particles than that inside the cell and causes fluid to be drawn into the cells. If used incorrectly, a hypotonic solution can cause too much fluid to enter the cell, causing the cells to swell and burst (hemolysis).
Movement of Water
The combined effects generated by ventricular contraction, colloid osmotic pressure in the intravascular space, solute content of the extracellular fluid (ECF), and solutes in the intracellular fluid (ICF), cause constant movement between the ICF and ECF compartments.13 Ultimately, a state of equilibrium is established, with a balance of fluid throughout the fluid compartments. An increase in plasma volume results in increased capillary hydrostatic pressure, forcing fluid into the interstitial space and creating edema. A decrease in plasma volume causes the movement of fluid from the interstitium into the vascular space because the interstitial hydrostatic pressure is greater than the capillary hydrostatic pressure.
Factors Controlling Fluid Balance
Antidiuretic Hormone and Aquaporins
ADH, also known as vasopressin, is secreted by the posterior pituitary gland and functions as the primary controller of ECF volume. Feedback messages for release of ADH are sent by osmoreceptors (water receptors) located in the hypothalamus. As serum osmolality rises above 285 mOsm/kg (normal range, 275 to 295 mOsm/kg), ADH is released and carried through the circulation to the nephrons. The kidney distal tubules, connecting tubules, and the collecting ducts alter their permeability to water by the action of three aquaporins. These are aquaporin-2 (AQP2), aquaporin-3 (AQP3), and aquaporin-4 (AQP4).14 ADH acts via the aquaporin-2 receptor (AQP2) on the distal tubule and collecting ducts to resorb water.14 ADH action in the kidney is predominantly mediated through the aquaporins.
The normal range of urinary osmolality is from 500 to 800 mOsm/kg. Box 25-3 identifies several additional mechanisms that stimulate the release of ADH. In addition to the usual stimuli, the presence of severe emotional or physical stress can initiate ADH release through the limbic system that surrounds the hypothalamus.
Aldosterone
Box 25-4 shows several factors that stimulate the release of aldosterone. The relationship between sodium and water plays an important role in the influence of the RAAS on body water regulation (see Fig. 25-4). A reduction in vascular volume stimulates the release of renin. Renin is converted to angiotensin I, which is converted to the powerful vasoconstrictor angiotensin II. Angiotensin II stimulates the adrenal glands to secrete aldosterone, which acts on the distal tubules to resorb sodium from the tubular lumen into the circulation. When sodium is retained, so is water. Angiotensin II also constricts the renal vasculature, reducing kidney blood flow and available glomerular filtrate, sending a signal to the posterior pituitary to release ADH. The two systems intertwine to maintain fluid and electrolyte balance.
Atrial Natriuretic Peptide
An additional influence on fluid and electrolyte regulation comes from the synthesis of atrial natriuretic peptide (ANP). This hormone is secreted from cells in the atria of the heart in response to hypernatremia, stimulation of stretch receptors as a result of increased volume, and increased pressure in the heart (Box 25-5). ANP affects sodium and water balance by blocking aldosterone and ADH production, initiating vasodilation, and stimulating increased sodium and water excretion by the collecting ducts of the kidneys. The physiologic effects of ANP include a reduction in fluid overload through diuresis, decreased cardiac workload, and reduction in cardiac preload and afterload.
Electrolyte Balance
Potassium
Potassium is the primary intracellular electrolyte and is responsible for numerous physiologic functions (Box 25-6). As with many solutes, diffusion and active transport across the cell membrane maintain potassium balance. Potassium leaves the cell by diffusion, moving toward the area of lesser concentration outside the cell, but it must be actively transported back into the cell to maintain cellular stability.13 One of the most important potassium functions in the body—that of aiding nervous impulse conduction and muscle contraction—is accomplished with the movement of potassium across the cell membrane. The gastrointestinal tract and skin excrete small amounts of potassium, but the major controllers of potassium stores are the kidneys.13 Potassium is resorbed by the proximal tubules and secreted into the distal tubules as needed to maintain balance. Resorption and secretion of potassium are influenced by many factors (Box 25-7). Of the estimated 60 to 100 mEq/day ingested by an individual, 90% of the potassium is resorbed before arriving at the distal tubule, where the remainder is usually excreted.
Sodium
Sodium is the most abundant extracellular electrolyte in the body and is associated with fluid balance and the amount of water retained or excreted by the kidneys.13 Along the length of the nephron sodium is resorbed from the filtrate. This is an active metabolic process that is regulated by site-specific sodium transporters.15 When diuretics are administered, sodium absorption is inhibited and consequently sodium is eliminated in the urine.15 Sodium plays an essential role in the transmission of nerve impulses through the sodium pump, or active transport mechanism, at the cellular level. Sodium is key to a number of physiologic functions (Box 25-8).
Calcium
Calcium is the electrolyte of greatest quantity in the body, with stores estimated at 1200 g. Of the total body calcium, 99% is contained in the bones.16 The remaining 1% is contained primarily in the ECF in the vascular space. The calcium contained within bone is in an inactive form that maintains bone strength and is a ready storehouse for mobilization of calcium to the serum in cases of depletion.16 In addition to bone metabolism, calcium is responsible for numerous other important functions, including myocardial contractility, coagulation, and neuromuscular activity (Box 25-9).
Phosphorus
As with calcium and magnesium, the serum values of phosphorus represent a minute portion of the actual body stores. Approximately 80% of the phosphorus is found in the bones.16 Most of the remaining phosphorus is intracellular, with only a small amount in the ECF. The primary function of phosphorus is the formation of ATP, which provides intracellular energy for active transport mechanisms across the cell membrane. Additional functions of phosphorus include cell membrane structure, acid–base balance, oxygen delivery to the tissues, cellular immunity, and bone strength (Box 25-10).
Phosphorus abnormalities are evident early in the course of kidney failure.17 The Third National Health and Nutrition Examination Survey (NHANES III, 1988-1994, which included 14,722 adults) revealed that people with mild or moderate kidney dysfunction—defined as a urinary creatinine clearance rate (CrCl) between 50 and 60 mL/min—already have elevations of serum phosphorus and potassium levels.17,18 In contrast, serum ionized calcium remains relatively unchanged until the creatinine clearance rate is extremely low (CrCl less than 20 mL/min) and kidney failure is advanced.
Magnesium
Magnesium is the second most important and abundant intracellular electrolyte; about 60% of it is located in the bone.13 The ECF contains only about 1% of the body’s magnesium, and the remaining amount resides in the ICF. The levels of other intracellular electrolytes, such as calcium and potassium, are affected by the level of magnesium. For example, calcium and magnesium compete for absorption in the gastrointestinal tract. If the dietary intake of calcium is higher than that of magnesium, calcium is preferentially resorbed and vice versa. The most important functions of magnesium are ensuring the transport of sodium and potassium across the cell membrane and as a cofactor in many intracellular enzyme reactions. Depletion of magnesium liberates potassium to the ECF, which causes an increase in the excretion of potassium by the kidney and hypokalemia. Magnesium also plays a role in maintaining neuromuscular activity, protein synthesis, and intracellular energy production (Box 25-11).
Chloride
Chloride is predominantly found in the ECF. Changes in serum chloride levels usually indicate changes in the other electrolytes or in acid–base balance. Chloride plays a major role in maintaining serum osmolality, water balance, and acid–base balance. Additional functions of chloride are listed in Box 25-12.
Bicarbonate
Bicarbonate () is an anion in the ECF, and it performs the essential function of maintaining the acid–base balance.13 Although bicarbonate is not solely responsible for the acid–base balance, it is the major ECF buffer. Bicarbonate levels in the body are in balance with carbonic acid (H2CO3) levels. The ratio between the two must remain proportional at 1 mEq of carbonic acid to 20 mEq of bicarbonate; otherwise, acid–base disturbances will result. When the carbonic acid level is elevated, acidosis results. When the bicarbonate level is high, alkalosis results.
Effects of Aging
Kidney function declines gradually with age, but this usually does not affect homeostasis in the healthy older adult unless proteinuria is present.19,20 Proteinuria is associated with complication in both the kidney and cardiovascular systems.19 With aging, the GFR declines by about 0.75 mL/min/year.19 However, despite the gradual decrease in the GFR and the associated reduction in clearance of creatinine, serum creatinine levels may not rise. This occurs because the reduced muscle mass associated with aging produces less creatinine to be excreted by the kidneys, essentially masking the overall effects of aging on the kidneys. As a result, relatively low levels of serum creatinine in older adults may be associated with reductions in the GFR and creatinine clearance. The gradual decline in kidney function that occurs with aging usually is not a threat to homeostasis because the remaining GFR is adequate. The Cockcroft-Gault formula is used to estimate GFR and includes age in the formula19 (see Chapter 26). However, when older adults become ill, the decline in kidney function can be accelerated, making older adults especially susceptible to acute and chronic kidney dysfunction.
Summary
Anatomy
• The kidneys are paired organs located retroperitoneally, one on each side of the vertebral column between T12 and L3.
• The nephron is the functional unit of the kidney; comprised of the glomerulus, proximal tubule, loop of Henle, distal tubule, and the collecting ducts.
Physiology
• The kidneys play a major role in maintaining homeostasis.
• Important functions of the kidneys include waste product removal, fluid electrolyte balance, and acid–base balance.
• Hormone functions of the kidneys support red blood cell production, blood pressure regulation, and bone metabolism.
• Kidney function declines gradually with age, making older adults more susceptible to kidney dysfunction during illness.