Chapter 29 Invasive Respiratory Support
Invasive ventilation is positive pressure ventilation applied via an endotracheal or tracheotomy tube. Most patients undergoing cardiac surgery do not have pulmonary disease and require invasive ventilation only until they have recovered from the surgery enough to be awakened. The ventilatory management of these patients is discussed in Chapter 17. In this chapter, the focus is on the care of patients with severe respiratory or cardiac disease who require prolonged and often complex forms of ventilation.
In the first part of the chapter the pathophysiologic effects of positive pressure ventilation are discussed. In the second part the principles of ventilator function are described. In the third part, ventilatory strategies in specific clinical situations, weaning ventilation, and tracheotomies are discussed. Because cardiothoracic intensive care units (ICUs) are often centers for mechanical cardiorespiratory support (see Chapter 22), a discussion of advanced ventilatory therapies, such as prone positioning and highfrequency oscillation, is also included. The indications for invasive ventilation are outlined in Chapter 27.
PATHOPHYSIOLOGY OF POSITIVE PRESSURE VENTILATION
In normal breathing, as the chest cavity expands during inspiration a negative pressure is generated within the alveoli, which draws air into the lungs. With invasive (or noninvasive) ventilation, positive pressure is applied to the airway and gas is blown into the lungs. Invasive ventilation is therefore nonphysiologic and has important respiratory and cardiac effects.1
Respiratory Effects
Gas Exchange
In patients with normal lungs, anesthesia and positive pressure ventilation increase ventilation-perfusion (V/Q) mismatch, which slightly impairs gas exchange. This is caused mainly by changes in the distribution of ventilation. In normal breathing, both ventilation and perfusion are greatest in the dependent regions of the lung, but in positive pressure breathing, ventilation is distributed more evenly throughout the lung. Thus, there is relatively more perfusion to dependent regions, which increases intrapulmonary shunt (a low V/Q ratio), and more ventilation to nondependent regions, which increases alveolar dead space (a high V/Q ratio). Intrapulmonary shunting reduces arterial oxygen tension (Pao2) whereas alveolar dead space increases arterial carbon dioxide tension (Paco2; see Chapter 1). Intrapulmonary shunting also occurs if functional residual capacity (FRC) is less than closing capacity, as occurs during anesthesia (see Chapter 1). Intrapulmonary shunting is partly ameliorated by positive end-expiratory pressure (PEEP).2
Barotrauma
Barotrauma is the rupture of alveolar units, respiratory bronchioles, or bullae, causing extrapulmonary air to accumulate; it may be seen as pulmonary interstitial emphysema, pneumomediastinum, or pneumothorax. Pneumothorax in patients receiving positive pressure ventilation is often under tension and can have significant effects on hemodynamics. Barotrauma is more likely in patients ventilated with high airway pressures. However, it is the transalveolar pressure that determines the risk for barotrauma, not the airway pressure. As can be seen in Figure 29-1, transalveolar pressure is higher for the same airway pressure with reduced lung compliance than it is with reduced chest wall compliance or increased airway resistance.
Ventilator-Induced Lung Injury
In certain circumstances, positive pressure ventilation can cause or contribute to acute lung injury.3 Patients most at risk for ventilator-induced lung injury are those with severe systemic (e.g., sepsis) or pulmonary (e.g., pneumonia) disease who are ventilated with large tidal volumes, high airway pressures, inadequate levels of PEEP, and high inspired oxygen concentrations.4 The mechanisms of ventilator-induced lung injury are complex but involve an exacerbation of systemic inflammation5 and physical trauma to the alveoli due to overdistension and the repeated opening and closing of lung units.6 Crude mortality rate in critically ill patients ventilated with high tidal volumes (12 ml/kg) is 9% higher than the rate in those ventilated with low tidal volumes (6 ml/kg).7 This observation has led to the concept of lung-protective ventilation, which is described subsequently.
Cardiac Effects
The main direct effects of positive ventilation on the cardiovascular system are reduced systemic venous return,8 altered right ventricular afterload,9 and reduced left ventricular afterload.10 The impact of these effects is determined by the particular patient’s underlying cardiac function and intravascular volume status. Positive pressure ventilation performed properly eliminates or substantially reduces the work of breathing, which also has an indirect positive effect on cardiovascular function.
Right Ventricular Afterload
The effect of invasive ventilation on right ventricular afterload is variable and depends on lung volume. With low lung volumes (e.g., due to atelectasis) positive pressure ventilation and PEEP optimize lung volume and improve gas exchange, both of which reduce right ventricular afterload. With high lung volumes (due to excessive ventilatory pressures or high levels of PEEP) the pulmonary capillaries become compressed, which increases afterload.11 Changes in right ventricular afterload have a substantial impact on cardiac output in patients who are hypovolemic or who have right ventricular dysfunction.
Left Ventricular Afterload
Positive pressure ventilation increases intrathoracic pressure, which reduces the transmural pressure gradient across the left ventricle. This reduces systolic wall stress and therefore afterload (see Chapter 1; see Equation 1-3). Positive pressure ventilation can lead to a substantial improvement in cardiac output in patients with left ventricular dysfunction and is an important adjuvant for treating acute heart failure.
VENTILATOR MODES AND CONTROLS
Ventilators have become very complex and have a bewildering array of modes and controls,13 despite a lack of evidence of improved outcomes.14 Further confusion is created because manufacturers call similar ventilatory modes different names.
At its most simple, invasive ventilation involves mandatory machine-delivered breaths of a given volume (or pressure), in which controls for fractional inspired oxygen (FIo2), breath rate, breath volume (or pressure), and the ratio of inspiratory time to expiratory time (the I:E ratio) are set.15 For this type of mandatory ventilation to succeed, the patient’s respiratory system must be entirely passive. Much of the complexity of modern ventilators comes from the desire to allow patients to take spontaneous breaths that are assisted by and synchronized with the ventilator.
Inspiratory Flow Generation: Volume Control or Pressure Control
Volume control ventilation provides a constant inspiratory flow to achieve a predefined tidal volume (Fig. 29-2). Airway pressure varies with inspiratory time and changes in lung compliance and airway resistance. When setting the ventilator in volume control mode, a tidal volume of 6 to 8 ml/kg should be chosen.
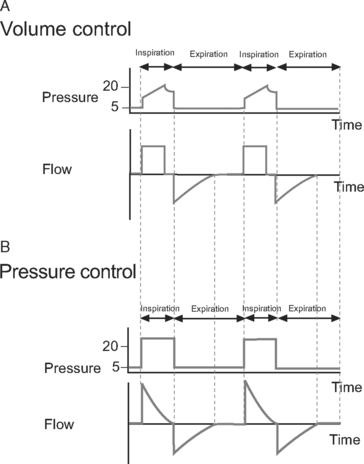
Figure 29.2 Pressure-time and flow-time curves for volume control and pressure control ventilation. A, Two volume control breaths are illustrated. The I:E ratio is approximately 1:2 and there is a short inspiratory pause at the end of inspiration. Plateau pressure is a slightly lower than peak pressure. Inspiratory flow is constant, whereas flow decreases during expiration. B, Two pressure control breaths are illustrated. The I:E ratio is approximately 1:2. No end-inspiratory pause is set. Inspiratory flow is decelerating and has fallen to zero by end-inspiration. In both A and B, expiratory flow has fallen to zero before the end-expiration. For the sake of clarity, the inspiratory rise time (see Fig. 28-4) has been set to zero; that is, peak inspiratory flow occurs immediately at the beginning of inspiration.
Pressure control ventilation provides a constant inspiratory pressure, which generates a decelerating inspiratory flow pattern (see Fig. 29-2). Tidal volume varies with inspiratory time and changes in lung compliance and airway resistance. The decelerating flow pattern distributes ventilation more evenly throughout the lung; in patients with V/Q mismatch, this improves oxygenation. It also allows some compensation for air leaks and limits inspiratory pressures, which helps to prevent ventilator-induced lung injury and barotrauma. The inspiratory pressure is chosen to achieve the desired tidal volume, usually 6 to 8 ml/kg, which typically requires a pressure of 15 to 30 cm H2O.16
Cycling
Time
In patients with increased airway resistance, a reduced respiratory rate and prolonged expiratory time is required to avoid dynamic lung hyperinflation (see subsequent material).17 For instance, a breath rate of 6 to 8 per minute with an inspiratory time of 1.3 seconds provides an expiratory time of 6.2 to 8.7 seconds and an I:E ratio of 1:4.7 to 1:6.7, respectively.
In patients with severe hypoxemia, a prolonged inspiratory time may enhance oxygenation by improving the ventilation of lung units with long time constants (see Chapter 1). In some circumstances, inverse ratio ventilation (e.g., an I:E ratio of 2:1 or 3:1) may be used (see Ventilatory Strategies in subsequent material).18
Flow
For some assisted modes of ventilation, such as pressure support, flow cycling is used to terminate inspiration and commence expiration. Termination of inspiration occurs when flow drops to a percentage of peak inspiratory flow (typically 0 to 25%). Flow cycling may improve patient-ventilator synchrony.19
Triggering
Triggering is the mechanism by which a patient’s spontaneous inspiratory effort is sensed and the ventilator is triggered to deliver inspiratory assistance. Thus, triggering is a method of cycling from expiration to inspiration that is not time dependent. Triggering is accomplished when the ventilator senses a change in flow or pressure within the ventilator circuit. Flow triggering is generally more sensitive than pressure triggering and results in improved patient-ventilator synchrony.15
Positive End-Expiratory Pressure
PEEP is positive pressure within the alveoli at endexpiration. It is applied by placing a pressure-limiting valve on the expiratory limb of the ventilator circuit. PEEP reduces extravascular lung water, prevents the opening and closing of small airways, and helps to recruit collapsed alveoli. This improves oxygenation, increases lung compliance, and reduces the risk of developing ventilator-induced lung injury.20,21 A low level of PEEP (5 cm H2O) should be applied to all patients who are invasively ventilated. High levels of PEEP (15 to 20 cm H2O) may be required in patients with acute respiratory distress syndrome (ARDS).22 Patients with increased airways resistance whose ventilators are set to an inadequate expiratory time may trap gas and generate auto-PEEP. By applying external PEEP at a level similar to that of the generated auto-PEEP, the inspiratory threshold load (see Fig. 27-4), and therefore the work of breathing, is reduced.
Ventilator Pressures and Graphics
Measured Pressures
Peak Pressure and Plateau Pressure.
Peak pressure is the maximum inspiratory pressure within the breathing circuit. It is affected by both respiratory compliance and airway resistance. Plateau pressure is the pressure within the breathing circuit following an end-inspiratory pause, which allows equalization of any pressure difference between the alveoli and the circuit. Thus, plateau pressure is indicative of alveolar pressure at end inspiration and is influenced by respiratory compliance alone. Plateau pressure may be measured (the patient must be heavily sedated, paralyzed, or both) using the inspiratory hold function on the ventilator or, in volume control mode, estimating it from the pressure-time curve (see Fig. 29-3) when an inspiratory pause or hold is used. Plateau pressure (PP) may be calculated from the respiratory system compliance (C) and the tidal volume (Vt), given:
Ventilator-induced lung injury is minimized by limiting plateau pressure to less than 32 cm H2O (and peak airway pressure to less than 35 cm H2O).4 However, when chest wall compliance is reduced (e.g., in cases of obesity or abdominal compartment syndrome), a higher plateau pressure may be tolerated (see Fig. 29-1).
With volume control ventilation, high peak and plateau pressures may be due to reduced lung or chest wall compliance (see Figs. 29-1 and 29-3), whereas high peak but normal plateau pressure is indicative of increased airway resistance (see Figs. 29-1 and 29-3).
End-Expiratory Pause Pressure and Auto-PEEP.
End-expiratory pause pressure is the pressure within the circuit following an end-expiratory pause, and it should equal the level of applied PEEP. If end-expiratory pause pressure is greater than the applied PEEP, auto-PEEP exists (Fig. 29-4).23 Auto-PEEP is caused by continued (delayed) emptying of alveoli at end-expiration and indicates increased airway resistance. The presence of auto-PEEP is suggested by continued gas flow at end-expiration on the flow-time curve (see Fig. 29-4).
Graphic Displays
Most ventilators can display real-time plots of derived respiratory indexes, which may be of assistance in adjusting ventilator settings.24 The most widely used are pressure-time and flow-time curves, dynamic pressure-volume loops, and flow-volume loops.
Pressure-Time and Flow-Time Curves.
Pressure-time and flow-time curves (see Figs. 29-2 to 29-4) are referred to in the earlier sections on flow generation, end-inspiratory pressure, and end-expiratory pressure. They are helpful in diagnosing abnormalities in compliance, resistance, and the presence of auto-PEEP.
The flow-time curve identifies whether flow has fallen to zero at end-expiration and end-inspiration. Continued flow at end-expiration indicates the presence of auto-PEEP (see Fig. 29-4). This may be remedied by increasing the expiratory time, which is usually achieved by reducing the breath rate. Continued flow at end-inspiration with pressure-control ventilation (see Fig. 29-3) may indicate increased airway resistance. It may be remedied by increasing the inspiratory time. However, if auto-PEEP exists as well, this may not be possible, and higher airway pressures may be required.
Dynamic Pressure-Volume Loops.
Dynamic pressure-volume loops (Fig. 29-5) provide a graphic representation of airway pressure against volume in real time for each breath. A dynamic pressure-volume loop differs from a static compliance curve because, in addition to the effect of respiratory system compliance, the effects of airway resistance, circuit resistance, and airflow are included.25
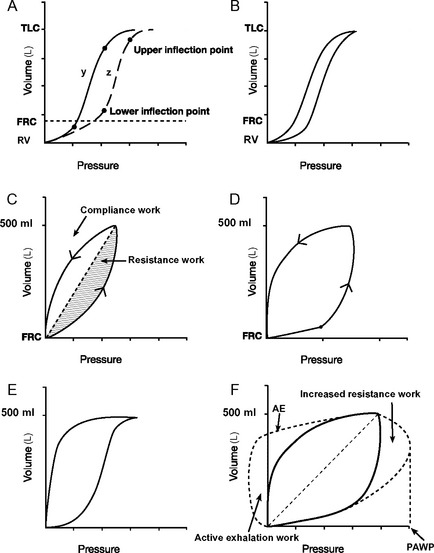
Figure 29.5 Pressure-volume loops. A, Two static pressure-volume curves (y and z; see also Fig. 1-15). Lower and upper inflexion points are shown on both curves. Above and below these inflexion points, compliance (ΔV/ΔP, the slope of the curve) is reduced. The lower inflexion point represents the lung volume at which some alveoli’s airways close (closing capacity); the upper inflexion point represents the start of overdistension. In curve y, the lower inflexion point lies below the functional residual capacity (FRC) and no airway closure occurs. In curve z, the inflexion point lies above the FRC, and airway closure will occur unless the FRC is increased by the application of PEEP. B, A dynamic pressure-volume curve is obtained by ventilating the lungs from residual volume (RV) to total lung capacity (TLC) when airways resistance is trivial. Hysteresis, in which lung volumes are different for a given pressure during inspiration and expiration is shown. Hysteresis is partly (but not solely) due to airway resistance. C, A dynamic pressure-volume curve is obtained when lungs are ventilated with a normal tidal volume from the FRC and airway resistance is normal. No inflexion points are seen, indicating no airway closure or overdistension. The shaded areas represent work to overcome respiratory system compliance (vertical hatch) and airway resistance (horizontal hatch). D, A dynamic pressure-volume curve with a lower inflexion point is indicative of inadequate PEEP. If a static pressure-volume curve were obtained, the FRC would be below the lower inflection point (curve z, A). E, A dynamic pressure-volume curve with an upper inflexion point is indicative of overinflation. This is termed “beaking.” F, The effect on the dynamic pressure-volume curve of increased airway resistance. Peak airway pressure (PAWP) and work of breathing (hatched areas) are increased because of the increased airway resistance. If expiration is active, the expiratory part of the curve will resemble the line labeled AE. Work done during active expiration is represented diagrammatically by the area labeled active exhalation work.
On a static respiratory system compliance curve (see Figs. 1-15 and 29-5A), upper and lower inflexion points can be identified. The upper inflexion point identifies overdistension of alveoli; the lower inflexion point identifies closing capacity. Ideally, the lungs should be ventilated between these two inflexion points, on the steep part of their compliance curves; that is, FRC should be above the lower inflexion point (as shown in Fig. 29-5A, curve y). The construction of a static compliance curve is impractical for routine care, and dynamic compliance curves are used instead (see Fig. 29-5B and C). Some ventilators can create an approximation of a static compliance curve by using the dynamic compliance curves generated by breaths of differing tidal volumes.
Inflexion points may be visible on dynamic-pressure volume curves but often they are not obvious. The presence of a lower inflexion point (see Fig. 29-5D) may indicate insufficient PEEP, whereas the presence of an upper inflexion point (see Fig. 29-5E) usually indicates overdistension due to excessive tidal volume. In patients ventilated appropriately with adequate PEEP and normal tidal volumes (6 to 8 ml/kg), ideally no inflexion points should be seen (see Fig. 29-5C). Changes in the dynamic pressure-volume loop that occur with increased airway resistance are shown in Figure 29-5F.
Managing Impaired Gas Exchange
Ventilator Management
Increasing FIo2.
Increasing the FIo2 is only partially effective in treating hypoxemia due to V/Q mismatch. Prolonged periods of ventilation at an FIo2 above 0.6 may exacerbate lung injury and should be avoided if possible.26 In patients with severe lung injury this may mean accepting a lower Sao2.
Altering the Tidal Volume.
To a certain extent, increasing tidal volume improves gas exchange, particularly carbon dioxide elimination. However, large tidal volumes (>10 ml/kg) overdistend open lung units and do not effectively recruit closed lung units, increasing the risk for ventilator-induced lung injury. Tidal volumes should be no higher than 10 ml/kg and preferably 6 to 8 ml/kg of ideal body weight.7
Decelerating Inspiratory Flow.
Changing from a constant inspiratory flow (volume control mode) to decelerating inspiratory flow (pressure control or hybrid volume control mode) may improve oxygenation and enhance carbon dioxide removal. However, there is no strong evidence to support the use of pressure control ventilation over volume control ventilation in patients with ARDS.27
Altering Respiratory Rate.
With increased airflow resistance, decreasing the respiratory rate to 6 to 8 breaths per minute reduces the risk of air trapping and dynamic lung hyperinflation.28 In restrictive lung disease (atelectasis, pulmonary edema, pneumonia, etc.), increasing the respiratory rate to 15 to 20 breaths per minute may improve gas exchange.
VENTILATORY STRATEGIES
Simple Adjuvant Therapies
Humidification
Humidification (see Chapter 28) of respiratory gases is essential to maintain mucociliary function and to prevent mucus plugging of large airways. Heat and moisture exchangers attached to the end of the endotracheal tube are suitable for use in patients who are mechanically ventilated for up to 48 hours.29 For patients who are ventilated for longer than 48 hours, a heated water-bath humidifier should be used.
Tracheal Suctioning
Intermittent tracheal suctioning prevents mucus plugging and lobar collapse but should be performed only when clinically indicated by coarse or noisy breath sounds, increased airway pressures, or deteriorating gas exchange. Closed (sterile) suctioning systems do not confer a benefit in terms of reduced incidence of ventilatorassociated pneumonia.30 Instillation of 0.9% saline (5 to 10 ml) and hand ventilation using a manual resuscitator is a useful maneuver if there is acute ventilatory compromise due to mucus plugging, but it should be performed only if normal suctioning is ineffective because it can have detrimental effects on oxygenation in the short term.31
Advanced Adjuvant Therapies
Recruitment Maneuvers
Recruitment maneuvers may be helpful in reopening collapsed alveoli.32 A recruitment maneuver involves the application of 30 to 40 cm H2O PEEP for 30 to 40 seconds. During the maneuver, patients should be heavily sedated or paralyzed so as to avoid respiratory effort. The maneuver may need to be terminated early if the patient develops severe hypotension or hypoxemia. Following the maneuver, PEEP is set to a very high level (20 to 25 cm H2O) and reduced in 2.5-cm-H2O increments every 10 minutes while observing the effect on gas exchange. The level of PEEP at which oxygenation deteriorates is the point at which airways closure is occurring. The recruitment maneuver should then be repeated and PEEP applied at a level just above that at which airway closure occurs. If no benefit is observed as a result of the intervention, no further recruitment maneuvers should be performed.33
Prone Positioning
Atelectasis is most likely to affect dependent pulmonary segments (see Fig. 27-2) which, because of the effects of gravity, are also the regions that receive preferential blood flow. With prone positioning, atelectatic regions are predominantly nondependent, and dependent regions are well ventilated and well perfused, which reduces intrapulmonary shunting. Prone positioning for 6 hours has been shown to improve oxygenation in 70% of patients with ARDS or acute lung injury (ALI),34 but a survival benefit has not been clearly demonstrated.35 Patients with ARDS of nonpulmonary origin (e.g., sepsis) obtain the greatest benefit. Prone positioning for 6- to 12-hour periods, alternating with 6- to 12-hour periods of supine positioning, may be considered in patients who require an FIo2 above 60% and PEEP above 10 cm H2O for a sustained period. If benefits are obtained, it should be continued until the FIo2 is less than 40% to 50% and PEEP less than 10 cm H2O.
Tracheal Gas Insufflation
A small-bore catheter may be placed through or beside the tracheal tube, with the end in the lower portion of the trachea and a low flow of oxygen administered (e.g., 2 l/min) to increase FIo2, augment PEEP, and wash out carbon dioxide.36
Inhaled Nitric Oxide
Inhaled nitric oxide is a potent pulmonary vasodilator that is used to treat severe pulmonary hypertension and right ventricular failure (see Chapter 24).37 Because inhaled nitric oxide is delivered only to ventilated regions of the lung, it also improves V/Q matching within the lung. Doses of 5 to 40 parts per million improve oxygenation in some patients with ARDS. However, the benefit is sustained for only 24 to 48 hours, and no improvement in patient outcome has been demonstrated.38 Inhaled nitric oxide may be considered in patients with severely impaired gas exchange that is resistant to other ventilatory measures. Sudden cessation of nitric oxide can cause marked hypoxemia and increased pulmonary vascular resistance, which can precipitate right ventricular failure.
Corticosteroids
With the exception of bronchospasm, corticosteroids have no role in the management of acute respiratory failure. There are conflicting data concerning the role of corticosteroids in persistent ARDS. One small, randomized trial involving only 24 patients demonstrated a marked survival benefit in patients with severe ARDS who had failed to improve by the seventh day of respiratory failure.39 However, a recent randomized trial involving 180 patients did not demonstrate any difference in survival rates in patients with ARDS of at least 7 days’ duration between those who were treated with methylprednisolone (2 mg/kg then 0.5 mg/kg every 6 hours for 14 days with subsequent tapering of the dosage) and those who were treated with placebo.40 Also, mortality rates were higher in the treatment group when methylprednisolone was started 2 or more weeks after the onset of ARDS. Some secondary outcomes, such as measures of gas exchange and number of ventilator days, were, however, better in steroid-treated patients. Routine use of corticosteroids for ARDS is not recommended.41
Specific Clinical Scenarios
Hypoxemia in the Early Postoperative Period
Occasionally, patients arrive from the operating room in a state of hypoxemia or hypoxemia rapidly develops in the ICU. Initial management is to rule out a life-threatening or easily correctable cause. The patient (along with the ventilator and breathing circuit) should be examined, and the postoperative chest radiograph should be reviewed. The common causes of hypoxemia in this situation are listed in Table 27-1.
Sometimes no cause for the hypoxemia is identified: the lungs are easy to ventilate, the chest radiograph is unremarkable, and Paco2 and pH are normal. This situation usually represents a combination of the adverse effects of positive pressure ventilation on V/Q matching, microatelectasis, impaired hypoxic pulmonary vasoconstriction, increased extravascular lung water, and low Svo2. It may be useful to suction the endotracheal tube and provide a gentle recruitment maneuver. Depending on the severity of the hypoxemia, no specific treatment may be required and the problem usually resolves over a few hours. If intervention is required, the following should be considered: (1) increasing PEEP; (2) stopping drugs that impair hypoxic pulmonary vasoconstriction; (3) using a decelerating inspiratory flow pattern; (4) increasing inspiratory time to more than 1.5 seconds; (5) deep sedation and paralysis. Superior vena cava oxygen saturation (SSVCo2) should be measured. Pulmonary artery catheterization, an echocardiogram, or both should be performed if there is hemodynamic instability or if SSVCo2 is low (see Chapter 20).
Patient-Ventilator Dysynchrony
Patient-ventilator dysynchrony is common when patients are awakened after cardiac surgery. Disorientation, biting the endotracheal tube, breathholding, shivering, and inappropriate ventilator settings all contribute to dysynchrony. It can present with impaired gas exchange, pulsus paradoxus, hypotension, and elevated central venous pressure. The hemodynamic picture may be identical to that of cardiac tamponade. The patient usually appears distressed, but that is not always the case.
Obesity
Patients who are morbidly obese are more likely to develop hypoxemia and require high airway pressures when mechanically ventilated. With obesity, FRC is reduced and closing capacity is elevated, resulting in increased V/Q mismatch (see Chapter 1). Chest wall compliance is reduced because of the weight of the thorax, abdomen, and breasts.
Patients should be nursed in a 30- to 45-degree head-up position. A strategy of small tidal volumes (6 ml/kg of ideal body weight) and a relatively rapid breath rate (14 to 18 breaths per minute) using pressure control ventilation helps to keep airway pressures to a minimum. Modest hypercapnia (pH >7.25) is acceptable. Despite this, increased peak and plateau airway pressures are common. However, high airway pressures are not especially dangerous because usually the transalveolar pressure is not increased (see Fig. 29-1).
Bronchospasm and Obstructive Lung Disease
The two major risks involved in invasive ventilation in patients with severe bronchospasm are (1) cardiovascular collapse secondary to dynamic lung hyperinflation and (2) tension pneumothorax. Dynamic lung hyperinflation may be avoided by ventilating with low breath rates and long expiratory times. Ventilation with a low minute volume (accepting a high Paco2) and maintenance of a normal inspiratory time minimize peak airway pressures. High peak airway pressures in this setting are not indicative of high transalveolar pressures (see Fig. 29-1); peak airway pressures above 35 cm H2O are preferable to shortening the expiratory time because the risk for barotrauma arises mainly from dynamic pulmonary hyperinflation or rupture of a bulla.
The following initial ventilator settings are appropriate for severe bronchospasm:
Bronchodilators may be administered into the ventilator circuit by nebulization or metered dose inhaler (see Chapter 28). In cases of hemodynamic collapse in which dynamic lung hyperinflation is suspected, the patient should be disconnected from the ventilator for 30 to 40 seconds to allow full expiration to occur (the “Lazarus maneuver”).
Cardiac Failure
Positive pressure ventilation reduces left ventricular afterload (see Chapter 1) and decreases extravascular lung water (pulmonary edema), which is beneficial in patients with left ventricular dysfunction. In the first instance, patients may respond to noninvasive ventilation (see Chapter 28). If invasive ventilation is required, no specific mode is recommended but high PEEP (>10 cm H2O) is rarely required. With pulmonary edema, disconnections from the ventilator to allow tracheal suctioning should be kept to an absolute minimum. Not only is suctioning ineffective in controlling pulmonary edema, but disconnecting the ventilator results in the loss of PEEP, which allows further extravasation of edema fluid.
ALI/ARDS and Lung-Protective Ventilation
Severe ARDS is relatively rare in cardiac surgery patients, but it may be encountered more frequently if the ICU functions as a referral center for extracorporeal membrane oxygenation (ECMO). ARDS is characterized by reduced lung compliance, impaired gas exchange, and a high risk for barotrauma (see Chapter 27). The goals in ventilating patients with ARDS are to sustain life while lung function recovers and to avoid ventilator-induced lung injury. The following ventilator settings and physiologic goals are appropriate:
The adjuvant ventilatory and nonventilatory strategies outlined should be considered, particularly recruitment maneuvers, prone positioning, inhaled nitric oxide, and reduction of metabolic rate. The strategy of small tidal volume ventilation with permissive hypercarbia is known as lung-protective ventilation, and it improves survival rates in patients with ARDS.7 For patients with life-threatening respiratory failure despite the treatment outlined, high-frequency oscillation or venovenous ECMO should be considered.
High-Frequency Oscillation
Patients with potentially reversible acute respiratory failure are occasionally unable to be kept alive with conventional ventilation. This most commonly occurs following lung transplantation and in patients with severe pneumonia complicated by ARDS. Such patients may be considered for either high-frequency oscillation or venovenous ECMO (see Chapter 22). High-frequency oscillation should be considered prior to ECMO in patients who fulfill ECMO criteria (see Chapter 22) but do not have severe hemodynamic instability.
A high-frequency oscillator contains a piston that moves back and forth rapidly as fresh gas (the bias gas flow) passes in front of it. The ventilator generates a constant positive airway pressure onto which small tidal breaths (<2 ml/kg) at very high rates (300 to 900/min) are superimposed.43 The constant positive airway pressure helps to recruit closed alveoli and therefore improves oxygenation, and the rapid, small breaths (oscillations) remove carbon dioxide.44
Ventilator Alarms
The causes and treatment of commonly employed alarms are listed in Table 29-1.
Alarm | Possible Causes | Interventions |
---|---|---|
High airway pressure with VC or low tidal volume with PC | Mucus plugging | Suction ETT |
Consider saline lavage | ||
Blocked circuit or HME | Ventilate with manual resuscitator, change HME and circuit | |
Endobronchial intubation | Review CXR and withdraw ETT | |
Obesity | Have patient sit up | |
Accept higher airway pressure | ||
Patient-ventilator dysynchrony | Reassure patient | |
Adjust ventilator settings (see text) | ||
Sedate and/or paralyze | ||
Increased airway resistance ± dynamic lung hyperinflation | Examine patient and ventilator graphics to confirm increased resistance ± auto-PEEP | |
Reduce breath rate and ensure adequate It and Et | ||
Treat bronchospasm if present | ||
Treat bronchospasm if present | ||
Suction if mucus plugging exists | ||
Decrease set PEEP | ||
In emergency: disconnect patient from ventilator for 30 seconds | ||
New lung disease causing reduced compliance (e.g., pulmonary edema, hemothorax, pneumothorax) | Examine patient and check ventilator graphics (see text) | |
Review CXR | ||
Treat specific pathology (see text) | ||
Blocked expiratory valve | Disconnect patient from ventilator | |
Hand ventilate with manual resuscitator | ||
Low expired minute volume | Spontaneous mode: apnea or hypoventilation | Change to mandatory ventilation mode |
Mandatory mode: cuff leak, circuit disconnection, large air leak from lung | Examine patient, circuit, and ventilator for leak | |
Check chest drains for bubbling; if present, consider reducing suction/lung isolation/surgery | ||
High expired minute volume | Hyperventilation, autotriggering, excessive pressure support or mandatory ventilation | Examine patient |
Ensure patient is not developing metabolic acidosis | ||
Check that ventilator settings are appropriate | ||
Treat respiratory alkalosis as appropriate (see text for details) | ||
High respiratory rate | Autotriggering: Water in the ventilator circuit, patient movement, hyperdynamic cardiac impulse, large cuff leak | Remove water from circuit |
Sedate patient | ||
Inflate cuff | ||
Inadequate respiratory support from ventilator | Decrease trigger sensitivity | |
Increase trigger sensitivity | ||
Increase ventilatory support | ||
Inadequate sedation | Optimize sedation |
CXR, chest radiograph; Et, expiratory time; ETT, endotracheal tube; HME, heat and moisture exchanger; It, inspiratory time; PC, pressure control; VC, volume control; PEEP, positive end expiratory pressure.
Weaning From Ventilation
For routine cardiac surgery patients, weaning from invasive ventilation is not required. Rather, sedation is stopped and, once patients begin making respiratory efforts, they are changed to a spontaneous breathing mode and extubated when awake (see Chapter 17).
Prolonged invasive ventilation for serious respiratory or cardiac disease involves two phases: (1) rest and repair; (2) recovery and retraining. During rest and repair, the aim is to reduce the work of breathing, provide adequate gas exchange, and avoid ventilator-induced lung injury. Patients are typically sedated but routine neuromuscular paralysis is not indicated because it prolongs the duration of invasive ventilation and increases the risk for critical illness polymyoneuropathy. Assist/control and SIMV with pressure support are commonly used modes during the rest and repair phase. Weaning (the recovery and retraining phase) is commenced once certain criteria have been met (Table 29-2).45
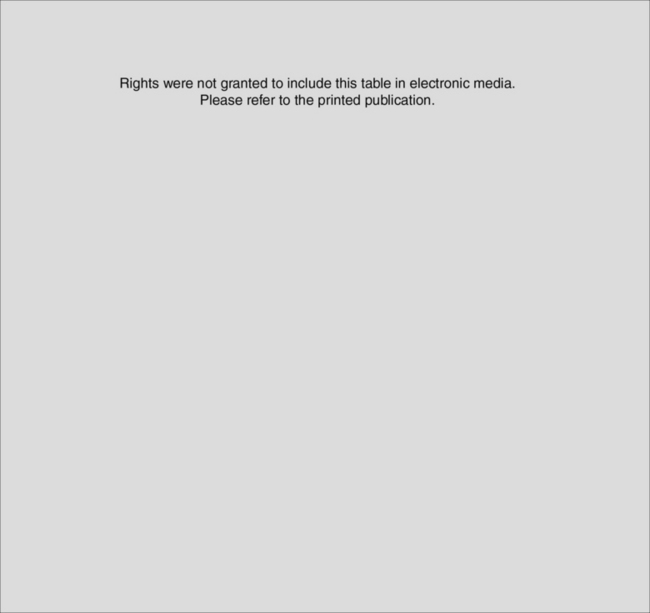
Table 29-2 Criteria for Commencing Weaning of Ventilatory Support
Rights were not granted to include this table in electronic media. Please refer to the printed book.
From MacIntyre NR, Cook DJ, Ely EW Jr, et al: Evidence-based guidelines for weaning and discontinuing ventilatory support: a collective task force facilitatd by the American College of Chest Physicians, the American Association for Respiratory Care, and the American College of Critical Care Medicine. Chest 120:375S-395S, 2001. PEEP, positive end-expiratory pressure.
Methods of Weaning
Multiple ventilatory techniques have been used to facilitate weaning. They include: (1) assist/control mode; (2) SIMV with a gradual reduction in the breath rate; (3) pressure support mode with a gradual reduction in the level of support; (4) frequent trials of spontaneous breathing. Trials of spontaneous breathing or a gradual reduction in the level of pressure support result in shorter periods of ventilation than does an SIMV-based weaning strategy.46 In one study, trials of spontaneous breathing were superior to gradual reductions in pressure support.47
Regardless of the weaning technique, the following should be borne in mind. First, the use of a protocol shortens weaning time.48 Second, the time required for weaning varies considerably among patients, from a few hours to longer than a month. Thus, clinicians must be prepared to modify weaning plans according to individual patients’ responses. Third, patients should never be allowed to exhaust themselves because it causes a loss of muscle strength that may require a further period of rest and recovery before weaning can be attempted again.
Failure to Wean
Cardiac dysfunction is a common cause of failure to wean.49 A patients who is difficult to wean should undergo an echocardiogram. If left ventricular systolic function is impaired, treatment with angiotensin-converting enzyme inhibitors, digoxin, furosemide, and spironolactone may facilitate weaning. Significant pericardial collections should be drained.
Excessive fluid administration can increase extravascular lung water and impair respiratory function. Also, patients receiving positive pressure ventilation tend to retain sodium and water. Daily water requirements are low in ventilated patients—about 20 ml/kg/day (see Chapter 32). The daily fluid balance should be closely scrutinized, and treatment with furosemide may be warranted even if cardiac function is normal.
Optimal nutrition is vital during weaning. Excess calories,50 particularly in the form of carbohydrates,51 increase carbon dioxide production and increase respiratory work. Too few calories prolong weakness and contribute to ventilator dependence.
Critical illness polymyoneuropathy (see Chapter 37) is a common cause of prolonged weaning. Treatment is supportive, and exacerbating factors, such as the use of corticosteroids and neuromuscular blockers, should be kept to a minimum.
Extubation
Numerous indexes have been proposed for predicting successful extubation (Table 29-3), but none have high positive predictive values.52 Prior to extubation, patients should be breathing comfortably and have satisfactory respiratory rates, tidal volumes, oxygenation (Pao2/FIo2 >20 κPa or 150 mmHg), and cough. They should be able to obey simple commands.
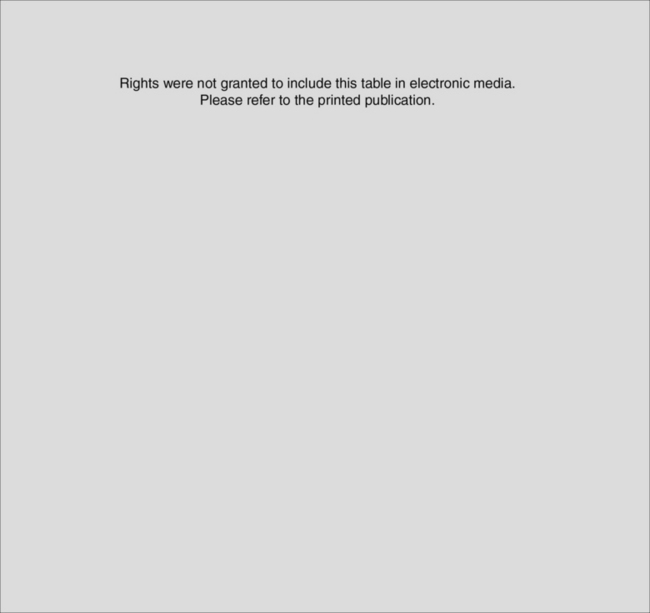
Table 29-3 Predictors of Successful Extubation
Rights were not granted to include this table in electronic media. Please refer to the printed book.
From MacIntyre NR, Cook DJ, Ely EW Jr, et al: Evidence-based guidelines for weaning and discontinuing ventilatory support: a collective task force facilitated by the American College of Chest Physicians; the American Association for Respiratory Care; and the American College of Critical Care Medicine. Chest 120:375S-395S, 2001.
Tracheotomy
Every patient who is ventilated for longer than 7 to 10 days should be considered for a tracheotomy, ideally at the beginning of the recovery and retraining phase.53 Early tracheotomy (within the first week) may reduce the total number of days on the ventilator.54 A tracheotomy provides greatly increased tolerance of ventilation, more effective clearance of secretions, the ability to remove and reinstitute invasive ventilation easily, and the potential to speak and eat.45 The main disadvantages of tracheotomies are procedure related. Tracheotomies do not increase the incidence of sternal wound infection or mediastinitis in cardiac surgery patients.55–57 Percutaneous insertion is at least as safe as open surgical placement58 and has the advantage of being able to be performed at the bedside. The technique of percutaneous tracheotomy is described in Chapter 40.
Management of Tracheotomies
A large-sized tracheotomy tube—typically 9 mm internal diameter for males and 8 mm internal diameter for females—should be used so as to minimize airway resistance. Initially, a nonfenestrated tube with a high-volume, low-pressure cuff should be used. The cuff should be inflated during positive pressure ventilation but may be deflated during periods of T-tube breathing. An inner tube may be used; it has the advantage of being able to be removed and cleaned. Adequate humidification of inspired gas should be provided by a heated water-bath (see Chapter 28); heat and moisture exchangers are suitable only for short-term use, and they impose increased circuit resistance.
In the case of a correctly placed tube of appropriate size, no airway leak should occur with a cuff pressure of less than 20 cm H2O and a volume of less than 10 ml. Cuff pressure should be checked regularly with a manometer. The presence of an airway leak despite appropriate cuff volume and pressure suggests that the tracheotomy tube has become partially dislodged (see Chapter 40).
Speaking.
When a patient is breathing spontaneously on a T-tube with the cuff down, a one-way (inhalation only) valve may be attached to the tracheotomy tube to allow the patient to exhale around the tube and through the vocal cords. In this way, speech is possible. Speech may be facilitated by the insertion of a fenestrated tracheotomy tube (Fig. 29-7A and B), which can be used either without an inner tube or with a fenestrated inner tube is a patient with an established tracheotomy. However, this requires changing the tracheotomy tube, which should be performed no sooner than 1 week after percutaneous insertion, when the tracheotomy track is well formed. Although positive pressure breathing is technically possible with a fenestrated tracheotomy combined with a nonfenestrated inner tube (Fig 29-7 D), it may be problematic due to airway leak, and a fenestrated tube should be inserted only once the patient has been weaned, for the most part, from positive pressure ventilation.
Swallowing and Eating.
Between 30% and 50% of patients with tracheotomies are observed to aspirate when swallowing is examined by video fluoroscopy, irrespective of whether or not the cuff is inflated. In 80% of cases, aspiration is clinically silent.59,60 During swallowing, a tracheotomy tube prevents normal laryngeal elevation, which is one of the mechanisms of protecting the airway from soiling by food particles. The inflated tracheotomy tube cuff also alters upper esophageal function and predisposes to aspiration. Patients with tracheotomies are often weak, with poor cough and impaired vocal cord function. Thus, patients should not routinely be fed orally; rather, nutrition should be provided via a fine-bore nasogastric or nasojejunal tube. However, during prolonged weaning, eating may significantly enhance a patient’s mood and oral comfort and the desire to get better. If a decision is made to offer a trial of feeding, the patient should be helped into a sitting position, the cuff deflated, and a small volume of soft food offered; soft food is less likely to be aspirated than is a liquid.
1 Fessler HE. Heart-lung interactions: applications in the critically ill. Eur Respir J. 1997;10:226-237.
2 Auler JOJr, Carmona MJ, Barbas CV, et al. The effects of positive end-expiratory pressure on respiratory system mechanics and hemodynamics in postoperative cardiac surgery patients. Braz J Med Biol Res. 2000;33:31-42.
3 Gattinoni L, Carlesso E, Cadringher P, et al. Physical and biological triggers of ventilator-induced lung injury and its prevention. Eur Respir J. 2003;47(suppl):S15-S25.
4 Adams AB, Simonson DA, Dries DJ. Ventilator-induced lung injury. Respir Care Clin North Am. 2003;9:343-362.
5 Ranieri VM, Suter PM, Tortorella C, et al. Effect of mechanical ventilation on inflammatory mediators in patients with acute respiratory distress syndrome: a randomized controlled trial. JAMA. 1999;282:54-61.
6 Pinhu L, Whitehead T, Evans T, et al. Ventilator-associated lung injury. Lancet. 2003;361:332-340.
7 The Acute Respiratory Distress Syndrome Network. Ventilation with lower tidal volumes as compared with traditional tidal volumes for acute lung injury and the acute respiratory distress syndrome. The Acute Respiratory Distress Syndrome Network. N Engl J Med. 2000;342:1301-1308.
8 van den Berg PC, Grimbergen CA, Spaan JA, et al. Positive pressure inspiration differentially affects right and left ventricular outputs in postoperative cardiac surgery patients. J Crit Care. 1997;12:56-65.
9 Vieillard-Baron A, Loubieres Y, Schmitt JM, et al. Cyclic changes in right ventricular output impedance during mechanical ventilation. J Appl Physiol. 1999;87:1644-1650.
10 Steingrub JS, Tidswell M, Higgins TL. Hemodynamic consequences of heart-lung interactions. J Intens Care Med. 2003;18:92-99.
11 Koganov Y, Weiss YG, Oppenheim A, et al. Positive end-expiratory pressure increases pulmonary venous vascular resistance in patients after coronary artery surgery. Crit Care Med. 1997;25:767-772.
12 Pacher R, Frass M, Hartter E, et al. The role of alpha-atrial natriuretic peptide in fluid retention during mechanical ventilation with positive end-expiratory pressure. Klin Wochenschrift. 1986;64(suppl 6):64-67.
13 Kapadia F. Mechanical ventilation: simplifying the terminology. Postgrad Med J. 1998;74:330-335.
14 Branson RD, Johannigman JA. What is the evidence base for the newer ventilation modes ? Respir Care. 2004;49:742-760.
15 Tobin MJ. Advances in mechanical ventilation. N Engl J Med. 2001;344:1986-1996.
16 Rappaport SH, Shpiner R, Yoshihara G, et al. Randomized, prospective trial of pressure-limited versus volume-controlled ventilation in severe respiratory failure. Crit Care Med. 1994;22:22-32.
17 Blanch L, Bernabe F, Lucangelo U. Measurement of air trapping, intrinsic positive end-expiratory pressure, and dynamic hyperinflation in mechanically ventilated patients. Respir Care. 2005;50:110-123.
18 Malarkkan N, Snook NJ, Lumb AB. New aspects of ventilation in acute lung injury. Anaesthesia. 2003;58:647-667.
19 Branson R. Understanding and implementing advances in ventilator capabilities. Curr Opin Crit Care. 2004;10:23-32.
20 Colmenero-Ruiz M, Fernandez-Mondejar E, Fernandez-Sacristan MA, et al. PEEP and low tidal volume ventilation reduce lung water in porcine pulmonary edema. Am J Respir Crit Care Med. 1997;155:964-970.
21 Fernandez Mondejar E, Vazquez Mata G, Cardenas A, et al. Ventilation with positive end-expiratory pressure reduces extravascular lung water and increases lymphatic flow in hydrostatic pulmonary edema. Crit Care Med. 1996;24:1562-1567.
22 Suh GY, Kwon OJ, Yoon JW, et al. A practical protocol for titrating “optimal” PEEP in acute lung injury: recruitment maneuver and PEEP decrement. J Korean Med Sci. 2003;18:349-354.
23 Brochard L. Intrinsic (or auto-) positive end-expiratory pressure during spontaneous or assisted ventilation. Intens Care Med. 2002;28:1552-1554.
24 Burns SM. Working with respiratory waveforms: how to use bedside graphics. AACN Clin Issues. 2003;14:133-144.
25 Lu Q, Rouby JJ. Measurement of pressure-volume curves in patients on mechanical ventilation: methods and significance. Crit Care. 2000;4:91-100.
26 Jenkinson SG. Oxygen toxicity. New Horiz. 1993;1:504-511.
27 Campbell RS, Davis BR. Pressure-controlled versus volume-controlled ventilation: does it matter ? Respir Care. 2002;47:416-424.
28 Conrad SA, Zhang S, Arnold TC, et al. Protective effects of low respiratory frequency in experimental ventilator-associated lung injury. Crit Care Med. 2005;33:835-840.
29 Nakagawa NK, Macchione M, Petrolino HM, et al. Effects of a heat and moisture exchanger and a heated humidifier on respiratory mucus in patients undergoing mechanical ventilation. Crit Care Med. 2000;28:312-317.
30 Vonberg RP, Eckmanns T, Welte T, et al. Impact of the suctioning system (open vs. closed) on the incidence of ventilation-associated pneumonia: meta-analysis of randomized controlled trials. Intens Care Med. 2006;32:1329-1335.
31 Raymond SJ. Normal saline instillation before suctioning: helpful or harmful ? A review of the literature. Am J Crit Care. 1995;4:267-271.
32 Hess DR, Bigatello LM. Lung recruitment: the role of recruitment maneuvers. Respir Care. 2002;47:308-317.
33 Marini JJ. Recruitment maneuvers to achieve an ‘open lung’ —whether and how ? Crit Care Med. 2001;29:1647-1648.
34 Gattinoni L, Tognoni G, Brazzi L, et al. Ventilation in the prone position. The Prone-Supine Study Collaborative Group. Lancet. 1997;350:815.
35 Gattinoni L, Tognoni G, Pesenti A, et al. Effect of prone positioning on the survival of patients with acute respiratory failure. N Engl J Med. 2001;345:568-573.
36 Nahum A. Tracheal gas insufflation as an adjunct to mechanical ventilation. Respir Care Clin North Am. 2002;8:171-185.
37 Bender KA, Alexander JA, Enos JM, et al. Effects of inhaled nitric oxide in patients with hypoxemia and pulmonary hypertension after cardiac surgery. Am J Crit Care. 1997;6:127-131.
38 Taylor RW, Zimmerman JL, Dellinger RP, et al. Low-dose inhaled nitric oxide in patients with acute lung injury: a randomized controlled trial. JAMA. 2004;291:1603-1609.
39 Meduri GU, Headley AS, Golden E, et al. Effect of prolonged methylprednisolone therapy in unresolving acute respiratory distress syndrome: a randomized controlled trial. JAMA. 1998;280:159-165.
40 Steinberg KP, Hudson LD, Goodman RB, et al. Efficacy and safety of corticosteroids for persistent acute respiratory distress syndrome. N Engl J Med. 2006;354:1671-1684.
41 Suter PM. Lung inflammation in ARDS—friend or foe ? N Engl J Med. 2006;354:1739-1742.
42 Ni Chonghaile M, Higgins B, Laffey JG. Permissive hypercapnia: role in protective lung ventilatory strategies. Curr Opin Crit Care. 2005;11:56-62.
43 Chan KP, Stewart TE. Clinical use of high-frequency oscillatory ventilation in adult patients with acute respiratory distress syndrome. Crit Care Med. 2005;33:S170-S174.
44 Pillow JJ. High-frequency oscillatory ventilation: mechanisms of gas exchange and lung mechanics. Crit Care Med. 2005;33:S135-S141.
45 MacIntyre NR, Cook DJ, Ely EWJr, et al. Evidence-based guidelines for weaning and discontinuing ventilatory support: a collective task force facilitated by the American College of Chest Physicians; the American Association for Respiratory Care; and the American College of Critical Care Medicine. Chest. 2001;120:375S-395S.
46 Meade M, Guyatt G, Sinuff T, et al. Trials comparing alternative weaning modes and discontinuation assessments. Chest. 2001;120:425S-437S.
47 Esteban A, Frutos F, Tobin MJ, et al. A comparison of four methods of weaning patients from mechanical ventilation. Spanish Lung Failure Collaborative Group. N Engl J Med. 1995;332:345-350.
48 Meade MO, Guyatt GH, Cook DJ. Weaning from mechanical ventilation: the evidence from clinical research. Respir Care. 2001;46:1408-1415.
49 Richard C, Teboul JL. Weaning failure from cardiovascular origin. Intens Care Med. 2005;31:1605-1607.
50 van den Berg B, Stam H. Metabolic and respiratory effects of enteral nutrition in patients during mechanical ventilation. Intens Care Med. 1888;14:206-211.
51 Cook D, Meade M, Guyatt G, et al. Trials of miscellaneous interventions to wean from mechanical ventilation. Chest. 2001;120:438S-444S.
52 Conti G, Montini L, Pennisi MA, et al. A prospective, blinded evaluation of indexes proposed to predict weaning from mechanical ventilation. Intens Care Med. 2004;30:830-836.
53 Heffner JE. The role of tracheotomy in weaning. Chest. 2001;120:477S-481S.
54 Griffiths J, Barber VS, Morgan L, et al. Systematic review and meta-analysis of studies of the timing of tracheostomy in adult patients undergoing artificial ventilation. BMJ. 2005;330:47-1243.
55 Borger MA, Rao V, Weisel RD, et al. Deep sternal wound infection: risk factors and outcomes. Ann Thorac Surg. 1998;65:1050-1056.
56 Byhahn C, Rinne T, Halbig S, et al. Early percutaneous tracheostomy after median sternotomy. J Thorac Cardiovasc Surg. 2000;120:329-334.
57 Gummert JF, Barten MJ, Hans C, et al. Mediastinitis and cardiac surgery —an updated risk factor analysis in 10,373 consecutive adult patients. Thorac Cardiovasc Surg. 2002;50:87-91.
58 Freeman BD, Isabella K, Lin N, et al. A meta-analysis of prospective trials comparing percutaneous and surgical tracheostomy in critically ill patients. Chest. 2000;118:1412-1418.
59 Elpern EH, Scott MG, Petro L, ET AL. Pulmonary aspiration in mechanically ventilated patients with tracheostomies. Chest. 1994;105:563-566.
60 Leder SB. Incidence and type of aspiration in acute care patients requiring mechanical ventilation via a new tracheotomy. Chest. 2002;122:1721-1726.