CHAPTER 1 Introduction to Cells
Biology is based on the fundamental laws of nature embodied in chemistry and physics, but the origin and evolution of life on earth were historical events. This makes biology more like astronomy than like chemistry and physics. Neither the organization of the universe nor life as we know it had to evolve as it did. Chance played a central role. Throughout history and continuing today, the genes of some organisms sustain chemical changes that are inherited by their progeny. Many of the changes reduce the fitness of the organism, but some changes improve fitness. Over the long term, competition between sister organisms with random differences in their genes determines which organisms survive in various environments. Although these genetic differences ensure survival, they do not necessarily optimize each chemical life process. The variants that survive merely have a selective advantage over the alternatives. Thus, the molecular strategy of life processes works well but is often illogical. Readers would likely be able to suggest simpler or more elegant mechanisms for many cellular processes described in this book.
In spite of obvious differences in size, design, and behavior, all forms of life share many molecular mechanisms because they all descended from a common ancestor that lived 3 or 4 billion years ago (Fig. 1-1). This founding organism no longer exists, but it must have utilized biochemical processes similar to the biological processes that sustain contemporary cells.
Over several billion years, living organisms diverged from each other into three great divisions: Bacteria, Archaea, and Eucarya (Fig. 1-1). Archaea and Bacteria were considered to be one kingdom until the 1970s; then ribosomal RNA sequences revealed that they were different divisions of the tree of life, having branched from each other early in evolution. The origin of eukaryotes is still uncertain, but they inherited genes from both Archaea and Bacteria. One possibility is that eukaryotes originated when an Archaea fused with a Bacterium. Note that multicellular eukaryotes (green, blue, and red in Fig. 1-1) evolved relatively recently, hundreds of millions of years after earlier, single-celled eukaryotes first appeared. Also note that algae and plants branched off before fungi, our nearest relatives on the tree of life.
This book focuses on the underlying molecular mechanisms of biological function at the cellular level. Chapter 1 starts with a brief description of the main features that set eukaryotes apart from prokaryotes and then covers the general principles that apply equally to eukaryotes and prokaryotes. It closes with a preview of the major components of eukaryotic cells. Chapter 3 covers the macromolecules that form cells, while Chapters 4 and 5 introduce the chemical and physical principles required to understand how these molecules assemble and function. Armed with this introductory material, the reader will be prepared to circle back to Chapter 2 to learn what is known of the origins of life and the evolution of the forms of life that currently inhabit the earth.
Features That Distinguish Eukaryotic and Prokaryotic Cells
Although sharing a common origin and basic biochemistry, cells vary considerably in their structure and organization (Fig. 1-2). Although diverse in terms of morphology and reliance on particular energy sources, Bacteria and Archaea have much in common, including basic metabolic pathways, gene expression, lack of organelles, and motility powered by rotary flagella. All eukaryotes (protists, algae, plants, fungi, and animals) differ from the two extensive groups of prokaryotes (Bacteria and Archaea) in having a compartmentalized cytoplasm with membrane-bounded organelles including a nucleus.
A plasma membrane surrounds all cells, and additional intracellular membranes divide eukaryotes into compartments, each with a characteristic structure, biochemical composition, and function (Fig. 1-2). The basic features of eukaryotic organelles were refined more than 1.5 billion years ago, before the major groups of eukaryotes diverged. The nuclear envelope separates the two major compartments: nucleoplasm and cytoplasm. The chromosomes carrying the cell’s genes and the machinery to express these genes reside inside the nucleus; they are in the cytoplasm of prokaryotes. Most eukaryotic cells have endoplasmic reticulum (the site of protein and phospholipid synthesis), a Golgi apparatus (an organelle that adds sugars to membrane proteins, lysosomal proteins, and secretory proteins), lysosomes (a compartment for digestive enzymes), peroxisomes (containers for enzymes involved in oxidative reactions), and mitochondria (structures that convert energy stored in the chemical bonds of nutrients into ATP in addition to other functions). Cilia (and flagella) are ancient eukaryotic specializations used by many cells for motility or sensing the environment. Table 1-1 lists the major cellular components and some of their functions.
Cellular Component | Description |
---|---|
Plasma membrane | A lipid bilayer, 7 nm thick, with integral and peripheral proteins; the membrane surrounds cells and contains channels, carriers and pumps for ions and nutrients, receptors for growth factors, hormones and (in nerves and muscles) neurotransmitters, plus the molecular machinery to transduce these stimuli into intracellular signals |
Adherens junction | A punctate or beltlike link between cells with actin filaments attached on the cytoplasmic surface |
Desmosome | A punctate link between cells associated with intermediate filaments on the cytoplasmic surface |
Gap junction | A localized region where the plasma membranes of two adjacent cells join to form minute intercellular channels for small molecules to move from the cytoplasm of one cell to the other |
Tight junction | An annular junction sealing the gap between epithelial cells |
Actin filament | “Microfilaments,” 8 nm in diameter; form a viscoelastic network in the cytoplasm and act as tracks for movements powered by myosin motor proteins |
Intermediate filament | Filaments, 10 nm in diameter, composed of keratin-like proteins that act as inextensible “tendons” in the cytoplasm |
Microtubule | A cylindrical polymer of tubulin, 25 nm in diameter, that forms the main structural component of cilia, flagella, and mitotic spindles; microtubules provide tracks for organelle movements powered by the motors dynein and kinesin |
Centriole | A short cylinder of nine microtubule triplets located in the cell center (centrosome) and at the base of cilia and flagella; pericentrosomal material nucleates and anchors microtubules |
Microvillus (or filopodium) | A thin, cylindrical projection of the plasma membrane supported internally by a bundle of actin filaments |
Cilia/flagella | Organelles formed by an axoneme of nine doublet and two singlet microtubules that project from the cell surface and are surrounded by plasma membrane; the motor protein dynein powers bending motions of the axoneme; nonmotile primary cilia have sensory functions |
Glycogen particle | Storage form of polysaccharide |
Ribosome | RNA/protein particle that catalyzes protein synthesis |
Rough endoplasmic reticulum | Flattened, intracellular bags of membrane with associated ribosomes that synthesize secreted and integral membrane proteins |
Smooth endoplasmic reticulum | Flattened, intracellular bags of membrane without ribosomes involved in lipid synthesis, drug metabolism, and sequestration of Ca2+ |
Golgi apparatus | A stack of flattened membrane bags and vesicles that packages secretory proteins and participates in protein glycosylation |
Nucleus | Membrane-bounded compartment containing the chromosomes, nucleolus and the molecular machinery that controls gene expression |
Nuclear envelope | A pair of concentric membranes connected to the endoplasmic reticulum that surrounds the nucleus |
Nuclear pore | Large, gated channels across the nuclear envelope that control all traffic of proteins and RNA in and out of the nucleus |
Euchromatin | Dispersed, active form of interphase chromatin |
Heterochromatin | Condensed, inactive chromatin |
Nucleolus | Intranuclear site of ribosomal RNA synthesis and processing; ribosome assembly |
Lysosome | Impermeable, membrane-bound bags of hydrolytic enzymes |
Peroxisome | Membrane-bound bags containing catalase and various oxidases |
Mitochondria | Organelles surrounded by a smooth outer membrane and a convoluted inner membrane folded into cristae; they contain enzymes for fatty acid oxidation and oxidative phosphorylation of ADP |
Some Universal Principles of Living Cells
Overview of Eukaryotic Cellular Organization and Functions
Nucleus
The nucleus (Fig. 1-10) stores genetic information in extraordinarily long DNA molecules called chromosomes. Surprisingly, the coding portions of genes make up only a small fraction (<2%) of the 3 billion nucleotide pairs in human DNA, but more than 50% of the 97 million nucleotide pairs in a nematode worm. Regions called telomeres stabilize the ends of chromosomes, and centromeres ensure the distribution of chromosomes to daughter cells when cells divide. The functions of most of the remaining DNA are not yet known. The DNA and its associated proteins are called chromatin (Fig. 1-5). Interactions with histones and other proteins fold each chromosome compactly enough to fit inside the nucleus. During mitosis, chromosomes condense further into separate structural units that one can observe by light microscopy (Fig. 1-7). Between cell divisions, chromosomes are decondensed but occupy discrete territories within the nucleus.
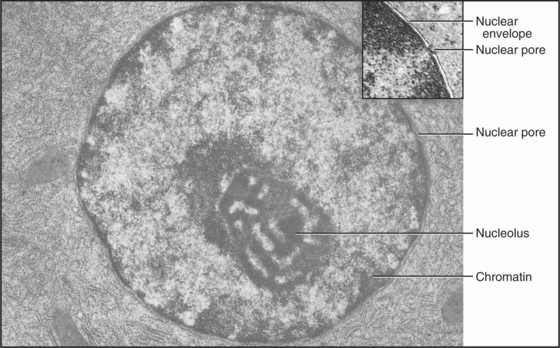
Figure 1-10 ELECTRON MICROGRAPH OF A THIN SECTION OF A NU-CLEUS.
(Courtesy of Don Fawcett, Harvard Medical School, Boston, Massachusetts.)
Proteins of the transcriptional machinery turn specific genes on and off in response to genetic, developmental, and environmental signals. Enzymes called polymerases make RNA copies of active genes. Messenger RNAs specify the amino acid sequences of proteins. Other RNAs have structural, regulatory, or catalytic functions. Most newly synthesized RNAs must be processed extensively before they are ready for use. Processing involves removal of noncoding intervening sequences, alteration of bases, or addition of specific structures at either end. For cytoplasmic RNAs, this processing occurs before RNA molecules are exported from the nucleus through nuclear pores. The nucleolus assembles ribosomes from more than 50 different proteins and 3 RNA molecules. Genetic errors resulting in altered RNA and protein products cause or predispose individuals to many inherited human diseases.
Cell Cycle
Cellular growth and division are regulated by an integrated molecular network consisting of protein kinases (enzymes that add phosphate to the side chains of proteins), specific kinase inhibitors, transcription factors, and highly specific proteases. When conditions inside and outside a cell are appropriate for cell division (Fig. 1-9B), changes in the stability of key proteins allow specific protein kinases to escape from negative regulators and to trigger a chain of events leading to DNA replication and cell division. Once DNA replication is initiated, specific destruction of components of these kinases allows cells to complete the process. Once DNA replication is complete, activation of the cell cycle kinases such as Cdk1 pushes the cell into mitosis, the process that separates chromosomes into two daugh-ter cells. Three controls sequentially activate Cdk1 through a positive feedback loop: (1) synthesis of a regulatory subunit, (2) transport into the nucleus, and (3) removal of inhibitory phosphate groups.
A key feature of the cell cycle is a series of built-in quality controls, called checkpoints (Fig. 1-9), which ensure that each stage of the cycle is completed successfully before the process continues to the next step. These checkpoints also detect damage to cellular constituents and block cell cycle progression so that the damage may be repaired. Misregulation of checkpoints and other cell cycle controls is a common cause of cancer. Remarkably, the entire cycle of DNA replication, chromosomal condensation, nuclear envelope breakdown, and reformation, including the modulation of these events by checkpoints, can be carried out in cell-free extracts in a test tube.
Ribosomes and Protein Synthesis
Ribosomes catalyze the synthesis of proteins, using the nucleotide sequences of messenger RNA molecules to specify the sequence of amino acids (Figs. 1-4, 1-6, and 1-11). If the protein being synthesized has a signal sequence for receptors on the endoplasmic reticulum (ER), the ribosome binds to the ER, and the protein is inserted into the ER membrane bilayer or into the lumen of the ER as it is synthesized. Otherwise, ribosomes are free in the cytoplasm, and newly synthesized proteins enter the cytoplasm for routing to various destinations.
Endoplasmic Reticulum
The endoplasmic reticulum is a continuous system of flattened membrane sacks and tubules (Fig. 1-11) that is specialized for protein processing and lipid biosynthesis. Motor proteins move along microtubules to pull the ER membranes into a branching network spread throughout the cytoplasm. ER also forms the outer bilayer of the nuclear envelope. ER pumps and channels regulate the cytoplasmic Ca2+ concentration, and ER enzymes metabolize drugs.
Ribosomes synthesizing proteins destined for insertion into cellular membranes or for export from the cell associate with specialized regions of the ER, called rough ER owing to the attached ribosomes (Fig. 1-6). These proteins carry signal sequences of amino acids that guide their ribosomes to ER receptors. As a polypeptide chain grows, its sequence determines whether the protein folds up in the lipid bilayer or translocates into the lumen of the ER. Some proteins are retained in the ER, but most move on to other parts of the cell.
Golgi Apparatus
The Golgi apparatus processes the sugar side chains of secreted and membrane glycoproteins and sorts the proteins for transport to other parts of the cell (Figs. 1-6 and 1-11). The Golgi apparatus is a stack of flattened, membrane-bound sacks with many associated vesicles. Membrane vesicles come from the ER and fuse with the Golgi apparatus. As a result of a series of vesicle-budding and fusion events, the membrane molecules and soluble proteins in the lumen pass through the stacks of Golgi apparatus from one side to the other. During this passage, Golgi enzymes, retained in specific layers of the Golgi apparatus by transmembrane anchors, modify the sugar side chains of secretory and membrane proteins. On the downstream side of the Golgi apparatus, processed proteins segregate into different vesicles destined for lysosomes or the plasma membrane. The Golgi apparatus is characteristically located in the middle of the cell near the nucleus and the centrosome.
Lysosomes
An impermeable membrane separates degradative enzymes inside lysosomes from other cellular components. Lysosomal proteins are synthesized by rough ER and transported to the Golgi apparatus, where enzymes recognize a three-dimensional site on the proteins’ surface that targets them for addition of the modified sugar, phosphorylated mannose (Fig. 1-6). Vesicular transport, guided by phosphomannose receptors, delivers lysosomal proteins to the lumen of lysosomes.
Plasma Membrane
The plasma membrane is the interface of the cell with its environment (Fig. 1-12). Owing to the hydrophobic interior of its lipid bilayer, the plasma membrane is impermeable to ions and most water-soluble molecules. Consequently, they cross the membrane only through transmembrane channels, carriers, and pumps, which provide the cell with nutrients, control internal ion concentrations, and establish a transmembrane electrical potential. A single amino acid change in one plasma membrane pump and Cl− channel causes cystic fibrosis.
Adhesive glycoproteins of the plasma membrane allow cells to bind specifically to each other or to the extracellular matrix. These selective interactions allow cells to form multicellular associations, such as epithelia. Similar interactions allow white blood cells to bind bacteria so that they can be ingested and digested in lysosomes. In cells that are subjected to mechanical forces, such as muscle and epithelia, adhesive proteins of the plasma membrane are reinforced by association with cytoskeletal filaments inside the cell. In skin, defects in these attachments cause blistering diseases.
ER synthesizes phospholipids and proteins for the plasma membrane (Fig. 1-6). After insertion into the lipid bilayer of the ER, proteins move to the plasma membrane by vesicular transport through the Golgi apparatus. Many components of the plasma membrane are not permanent residents; receptors for extracellular molecules, including nutrients and some hormones, can recycle from the plasma membrane to endosomes and back to the cell surface many times before they are degraded. Defects in the receptor for low-density lipoproteins cause arteriosclerosis.
Mitochondria
Mitochondrial enzymes convert most of the energy released from the breakdown of nutrients into the synthesis of ATP, the common currency for most energy-requiring reactions in cells (Fig. 1-11). This efficient mitochondrial system uses molecular oxygen to complete the oxidation of fats, proteins, and sugars to carbon dioxide and water. A less efficient glycolytic system in the cytoplasm extracts energy from the partial breakdown of glucose to make ATP. Mitochondria cluster near sites of ATP utilization, such as sperm tails, membranes engaged in active transport, nerve terminals, and the contractile apparatus of muscle cells.
Mitochondria form in a fundamentally different way from the ER, Golgi apparatus, and lysosomes (Fig. 1-6). Free ribosomes synthesize most mitochondrial proteins, which are released into the cytoplasm. Receptors on the surface of mitochondria recognize and bind signal sequences on mitochondrial proteins. Energy-requiring processes transport these proteins into the lumen or insert them into the outer or inner mitochondrial membranes.
DNA, ribosomes, and messenger RNAs located inside mitochondria produce a small number of the proteins that contribute to the assembly of the organelle. This machinery is left over from an earlier stage of evolution when mitochondria arose from symbiotic Bacteria (Fig. 1-1). Defects in the maternally inherited mitochondrial genome cause several diseases, including deafness, diabetes, and ocular myopathy.
Peroxisomes
Peroxisomes are membrane-bound organelles containing enzymes that participate in oxidative reactions. Like mitochondria, peroxisomal enzymes oxidize fatty acids, but the energy is not used to synthesize ATP. Peroxisomes are particularly abundant in plants as well as some animal cells. Peroxisomal proteins are synthesized in the cytoplasm and imported into the organelle using the same strategy as mitochondria but using different targeting sequences and transport machinery (Fig. 1-6). Genetic defects in peroxisomal biogenesis cause several forms of mental retardation.
Cytoskeleton and Motility Apparatus
A cytoplasmic network of three protein polymers—actin filaments, intermediate filaments, and microtubules (Fig. 1-13)—maintains the shape of a cell. Each polymer has distinctive properties and dynamics. Actin filaments and microtubules also provide tracks for the ATP-powered motor proteins that produce most cellular movements (Fig. 1-14), including cellular locomotion, muscle contraction, transport of organelles through the cytoplasm, mitosis, and the beating of cilia and flagella. The specialized forms of motility exhibited by muscle and sperm are exaggerated, highly organized versions of the motile processes used by most other eukaryotic cells.
Networks of cross-linked actin filaments anchored to the plasma membrane (Fig. 1-12) reinforce the surface of the cell. In many cells, tightly packed bundles of actin filaments support finger-like projections of the plasma membrane (Fig. 1-5). These filopodia or microvilli increase the surface area of the plasma membrane for transporting nutrients and other processes, including sensory transduction in the ear. Genetic defects in a membrane-associated, actin-binding protein called dystrophin cause the most common form of muscular dystrophy.
Actin filaments participate in movements in two ways. Assembly of actin filaments produces some movements, such as the extension of pseudopods. Other movements result from force produced by the motor protein myosin moving along actin filaments (Fig. 1-14). A family of different types of myosin uses the energy from ATP hydrolysis to produce movements. Muscles use a highly organized assembly of actin and myosin filaments to produce forceful, rapid, one-dimensional contractions. Myosin also drives the contraction of the cleavage furrow during cell division. External signals, such as chemotactic molecules, can influence both actin filament organization and the direction of motility. Genetic defects in myosin cause enlargement of the heart and sudden death.
Microtubules are rigid cylindrical polymers with two main functions. They serve as (1) mechanical reinforcing rods for the cytoskeleton and (2) the tracks for two classes of motor proteins. They are the only cytoskeletal polymer that can resist compression. The polymer has a molecular polarity that determines the rate of growth at the two ends and the direction of movement of motor proteins. Virtually all microtubules in cells have the same polarity relative to the organizing centers that initiate their growth (e.g., the centrosome) (Fig. 1-2). Their rapidly growing ends are oriented toward the periphery of the cell. Individual cytoplasmic microtubules are remarkably dynamic, growing and shrinking on a time scale of minutes.