Chapter 1 Introduction
Additional videos for this topic are available online at www.expertconsult.com.
The full text of this chapter can be accessed online at www.expertconsult.com.
Equipment Considerations and Image Formation
One of the primary physical components of an ultrasound machine is the transducer, which is connected by a cable to the other components, including the image screen or monitor and the computer processing unit. The transducer is placed on the skin surface and determines the imaging plane and structures that are imaged. Ultrasound is a unique imaging method in that sound waves are used rather than ionizing radiation for image production. An essential principle of ultrasound imaging relates to the piezoelectric effect of the ultrasound transducer crystal, which allows electrical signal to be changed to ultrasonic energy and vice versa. An ultrasound machine sends the electrical signal to the transducer, which results in the production of sound waves. The transducer is coupled to the soft tissues with acoustic transmission gel, which allows transmission of the sound waves into the soft tissues. These sound waves interact with soft tissue interfaces, some of which reflect back toward the skin surface and the transducer, where they are converted to an electrical current used to produce the ultrasound image. At soft tissue interfaces between tissues that have significant differences in impedance, there is sound wave reflection, which produces a bright echo. A sound wave that is perpendicular to the surface of an object being imaged will be reflected more than if it is not perpendicular. In addition to reflection, sound waves can be absorbed and refracted by the soft tissue interfaces. The absorption of a sound wave is enhanced with increasing frequency of the transducer and greater tissue viscosity.1
An important consideration in ultrasound imaging is the frequency of the transducer because this determines image quality. A transducer is designated by the range of sound wave frequencies it can produce, described in megahertz (MHz). The higher the frequency, the higher the resolution of the image; however, this is at the expense of sound beam penetration as a result of sound wave absorption.1 In contrast, a low-frequency transducer optimally assesses deeper structures, but it has relatively lower resolution. Transducers may also be designated as linear or curvilinear (Fig. 1-1). With a linear transducer, the sound wave is propagated in a linear fashion parallel to the transducer surface (Video 1-1). This is optimum in evaluation of the musculoskeletal system to assess linear structures, such as tendons, to avoid artifact. A curvilinear transducer may be used, although less commonly in evaluation of deeper structures because this increases the field of view (Video 1-2),
or it may provide guidance of a needle for biopsy or aspiration. A small footprint linear probe is very important for imaging the hand, ankle, and foot given the contours of these body parts that allow only limited contact with the probe surface (see Fig. 1-1C). A small footprint transducer with an offset is helpful when performing procedures on the distal extremities.
Scanning Technique
To produce an ultrasound image, the transducer is held on the surface of the skin to image the underlying structures. Ample acoustic transmission gel should be used to enable the sound beam to be transmitted from the transducer to the soft tissues and to allow the returning echoes to be converted to the ultrasound image. I prefer a layer of thick transmission gel over a more cumbersome gel standoff pad. Gel that is more like liquid consistency is also less ideal because the gel tends not to stay localized at the imaging site. The transducer should be held between the thumb and fingers of the examiner’s dominant hand, with the end of the transducer near the ulnar aspect of the hand (Fig. 1-2A). It is very important during imaging to stabilize or anchor the transducer on the patient with either the small finger or the heel of the imaging hand (see Fig. 1-2B). This technique is essential to maintain proper pressure of the transducer on the skin, to avoid involuntary movement of the transducer, and to allow fine adjustments in transducer positioning. Remember that the sound beam emitted from the transducer is focused relative to the short end of the transducer, and side-to-side movement of the transducer should only be a millimeter at a time.
Various terms describe manual movements of the transducer during scanning. The term heel-toe is used when the transducer is rocked or angled along the long axis of the transducer (Fig. 1-3A). The term toggle is used when the transducer is angled from side to side (see Fig. 1-3B). With both the heel-toe and toggle maneuvers, the transducer is not moved from its location, but rather the transducer is angled. The term translate is used when the transducer is moved to a new location while maintaining a perpendicular angle with the skin surface. The term sweep is used when the transducer is slid from side to side while maintaining a stable hand position, similar to sweeping a broom.
Image Appearance
Once the transducer is placed on the patient’s skin with intervening gel, a rectangular image (when using a linear transducer) appears on the monitor. The top of the image represents the superficial soft tissues that are in contact with the transducer, and the deeper structures appear toward the lower aspect of the image (Fig. 1-4). To understand the resulting ultrasound image, consider the sound beam as a plane or slice that extends down from the transducer along its long axis. It is this plane that is portrayed on the image. The left and right sides of the image can represent either end of the transducer, and this can usually be switched by using the left-to-right invert button on the ultrasound machine or by simply rotating the transducer 180 degrees. When imaging a structure in long axis, it is common to have the proximal aspect on the left side of the image and the distal aspect on the right.
Image optimization is essential to maximize resolution and clarity. The first step is to select the proper transducer and frequency. Higher-frequency transducers (10 MHz or greater) optimally evaluate superficial structures, whereas lower-frequency transducers are used for deep structures. Linear transducers are typically used, unless the area of interest is deep, such as the hip region, where a curvilinear transducer may be chosen. After the proper transducer is selected and placed on the patient, the next step is to adjust the depth of the sound beam; this is accomplished by a button or dial on the ultrasound machine. The depth of the sound beam is adjusted until the structure of interest is visible and centered in the image (Fig. 1-5A and B). The next step in optimization with many ultrasound machines is to adjust the focal zones of the ultrasound beam, if present on the ultrasound machine. This feature is typically displayed on the side of the image as a number of cursors or other symbols. It is optimum to reduce the number of focal zones to span the area of interest because increased focal zones will decrease the frame rate that produces a windshield-wiper effect. It is also important to move the depth of the focal zones to the depth where the structure is to be imaged to optimize resolution (see Fig. 1-5C). Some ultrasound machines have a broad focal zone that may not have to be moved. Finally, the overall gain can be adjusted by a knob on the ultrasound machine to increase or decrease the overall brightness of the echoes, which is in part determined by the ambient light in the examination room (see Fig. 1-5D). The gain should ideally be set where one can appreciate the ultrasound characteristics of normal soft tissues (as described later).
Sonographic Appearances of Normal Structures
Normal musculoskeletal structures have characteristic appearances on ultrasound imaging.2 Normal tendons appear hyperechoic with a fiber-like or fibrillar echotexture (see Fig. 1-4).3 At close inspection, the linear fibrillar echoes within a tendon represent the endotendineum septa, which contain connective tissue, elastic fibers, nerve endings, blood, and lymph vessels.3 Continuous tendon fibers are best appreciated when they are imaged long axis to the tendon. On such a long axis image, by convention the proximal aspect is on the left side of the image, with the distal aspect on the right. Normal muscle tissue appears relatively hypoechoic (Fig. 1-6). At closer inspection, the hypoechoic muscle tissue is separated by fine hyperechoic fibroadipose septa or perimysium, which surrounds the hypoechoic muscle bundles. The surface of bone or calcification is typically very hyperechoic, with posterior acoustic shadowing and possibly posterior reverberation if the surface of the bone is smooth and flat (see Fig. 1-6). The hyaline cartilage covering the articular surface of bone is hypoechoic and uniform (Fig. 1-7A and B), whereas the fibrocartilage, such as the labrum of the hip and shoulder, and the knee menisci are hyperechoic (see Fig. 1-7B). Ligaments have a hyperechoic, striated appearance that is more compact compared with tendons (Fig. 1-8). In addition, ligaments are also identified in that they connect two osseous structures. Often normal ligaments may appear relatively hypoechoic when surrounded by hyperechoic subcutaneous fat; however, a compact linear hyperechoic ligament can be appreciated when imaged in long axis perpendicular to the ultrasound beam.
Normal peripheral nerves have a fascicular appearance in which the individual nerve fascicles are hypoechoic, surrounded by hyperechoic connective tissue epineurium (Fig. 1-9).4 Hyperechoic fat is typically seen around larger peripheral nerves. In short axis, peripheral nerves display a honeycomb or speckled appearance, which allows their identification. Because peripheral nerves have a relatively mixed hyperechoic and hypoechoic echotexture, their appearance changes relative to the adjacent tissues. For example, the median nerve in the forearm, when surrounded by hypoechoic muscle, appears relatively hyperechoic; in contrast, more distally in the carpal tunnel, when it is surrounded by hyperechoic tendon, the median nerve appears relatively hypoechoic (see Fig. 5-3B in Chapter 5). The epidermis and dermis collectively appear hyperechoic, whereas the hypodermis shows hypoechoic fat and hyperechoic fibrous septa (see Fig. 1-7).
Sonographic Artifacts
One must be familiar with several artifacts common to musculoskeletal ultrasound. One such artifact is anisotropy.5 When a tendon is imaged perpendicular to the ultrasound beam, the characteristic hyperechoic fibrillar appearance is displayed. However, when the ultrasound beam is angled as little as 5 degrees relative to the long axis of such a structure, the normal hyperechoic appearance is lost; the tendon becomes more hypoechoic with increased angle (Figs. 1-10 to 1-13). This variation of ultrasound interaction with fibrillar tissues is called anisotropy, and it involves tendons and ligaments and, to a lesser extent, muscle. Because abnormal tendons and ligaments may also appear hypoechoic, it is important to focus on that segment of tendon or ligament that is perpendicular to the ultrasound beam, to exclude anisotropy. With a curved structure, such as the distal aspect of the supraspinatus tendon, the transducer is continually moved or angled to exclude anisotropy as the cause of a hypoechoic tendon segment (see Fig. 1-11) (Video 1-3). Anisotropy is noted both in long axis and short axis of ligaments and tendons (Video 1-4),
but it occurs when the sound beam is angled relative to the long axis of a structure (see Fig. 1-12). Therefore, to correct for anisotropy, the transducer is angled along the long axis of the imaged tendon or ligament; when imaging a tendon in long axis, the transducer is angled as a heel-toe maneuver (see Fig. 1-3A and Video 1-5),
whereas in short axis, the transducer is toggled (see Fig. 1-3B and Video 1-6).
Anisotropy can be used to one’s advantage in identification of a hyperechoic tendon or ligament in close proximity to hyperechoic soft tissues, such as in the ankle and wrist. When imaging a tendon in short axis, toggling the transducer will cause the tendon to become hypoechoic, thus allowing its distinction from the adjacent hyperechoic fat that does not demonstrate anisotropy (see Fig. 1-12). Once the tendon is identified, it is important to eliminate anisotropy to exclude pathology. Anisotropy is also helpful in identification of some ligaments, such as in the ankle, because they are often adjacent to hyperechoic fat (see Fig. 1-13). In addition, hyperechoic tendon calcifications can be made more conspicuous when they are surrounded by hypoechoic tendon from anisotropy with angulation of the transducer (see Fig. 3-62 in Chapter 3). When performing an interventional procedure, it is anisotropy that causes the needle to become less conspicuous when the needle is not perpendicular to the sound beam (see Fig. 9-7 in Chapter 9).
Another important artifact is shadowing. This occurs when the ultrasound beam is reflected, absorbed, or refracted.6 The resulting image shows an anechoic area that extends deep from the involved interface. Examples of structures that produce shadowing include interfaces with bone or calcification (Fig. 1-14), some foreign bodies (see Chapter 2), and gas. An object with a small radius of curvature or a rough surface will display a clean shadow, whereas an object with a large radius of curvature and a smooth surface will display a dirty shadow (resulting from superimposed reverberation echoes).7 Refractile shadowing may also occur at the edge of some structures, such as a foreign body or the end of a torn Achilles or patellar tendon (Fig. 1-15).8
Another type of artifact is posterior acoustic enhancement or increased through-transmission. This occurs during imaging of fluid (Figs. 1-16 and 1-17) and solid soft tissue tumors, such as peripheral nerve sheath tumors (see Fig. 2-59 in Chapter 2) and giant cell tumors of tendon sheath (Fig. 1-18).9 In these situations, the sound beam is relatively less attenuated compared with the adjacent tissues; therefore, the deeper soft tissues will appear relatively hyperechoic compared with the adjacent soft tissues.6
Another artifact with musculoskeletal implications is posterior reverberation. This occurs when the surface of an object is smooth and flat, such as a metal object or the surface of bone. In this situation, the sound beam reflects back and forth between the smooth surface and the transducer and produces a series of linear reflective echoes that extend deep to the structure.6 If the series of reflective echoes is more continuous deep to the structure, the term ring-down artifact is used, as may be seen with metal surfaces (Fig. 1-19). Ultrasound is ideal in evaluation of structures immediately overlying metal hardware because this reverberation artifact occurs deep to the hardware without obscuring the superficial soft tissues. Related to posterior reverberation is the comet-tail artifact, such as that seen with soft tissue gas (Fig. 1-20), which appears as a short segment of posterior bright echoes that narrows further from the source of the artifact.
One additional artifact to consider is beam-width artifact. This is essentially analogous to volume averaging and occurs if the ultrasound beam is too wide relative to the object being imaged. An example is imaging of a small calcification in which the relatively large beam width may eliminate shadowing. This effect can be reduced by adjusting the focal zone to the level of the object of interest.6
Miscellaneous Ultrasound Techniques
Several ultrasound techniques or applications available with some ultrasound machines can enhance scanning and diagnostic capabilities. One such method is spatial compound sonography.10 Unlike conventional ultrasound, sound beams with spatial compound sonography are produced at several different angles, with information combined to form a single ultrasound image. This improves tissue plane definition, but it has a smoothing effect, and motion blur is more likely because frames are compounded (Fig. 1-21). One must be aware that the use of spatial compounding may reduce the artifact produced by a foreign body, which may decrease its conspicuity (see Fig. 2-52 in Chapter 2).
Another ultrasound technique is tissue harmonic imaging. Unlike conventional ultrasound, which receives only the fundamental or transmitted frequency to produce the image, with tissue harmonic imaging, harmonic frequencies produced during ultrasound beam propagation through tissues are used to produce the image. This technique assists in evaluation of deep structures and also improves joint and tendon surface visibility.11 The technique may more clearly delineate the edge of a soft tissue mass (Fig. 1-22) or a fluid-filled tendon tear (Fig. 1-23).
One helpful technique available on some ultrasound machines is extended field of view. With this technique, an ultrasound image is produced by combining image information obtained during real-time scanning. This allows imaging of an entire muscle from origin to insertion; it is helpful in measuring large abnormalities (e.g., tumor or tendon tear) and in displaying and communicating ultrasound findings (Figs. 1-24 and 1-25).12 An alternative to extended field of view imaging that is available on some ultrasound equipment is the split-screen function, which essentially joins two images on the display screen that doubles the field of view.
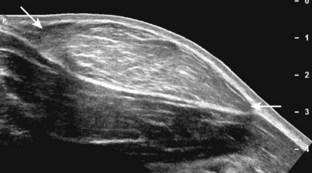
FIGURE 1-25 Extended field of view.
Ultrasound image shows full extent of a lipoma (between arrows).
A number of ultrasound techniques are relatively new, and their practical musculoskeletal applications are still being defined. One such technique is three-dimensional ultrasound, which acquires data as a volume (either mechanically or freehand) and thus enables reconstruction at any imaging plane (Fig. 1-26). This technique has been used to characterize rotator cuff tears and to quantify a volume of tissue such as tumor or synovial proliferation.13,14 An additional technique is fusion imaging, in which real-time ultrasound imaging can be superimposed on computed tomography (CT) or MRI; this has been used to assist with needle guidance for sacroiliac joint injections.15 One last technique is ultrasound elastography, which is used to assess the elastic properties of tissue. With this technique, compression of tissue produces strain or displacement within the tissue. Displacement is less when tissue is hard; it is displayed as blue on the ultrasound image, whereas soft tissue is displayed as red (Fig. 1-27). With regard to musculoskeletal applications, normal tendons appear as blue, whereas areas of tendinopathy, such as of the Achilles tendon or common extensor tendon of the elbow, appear as red.14,16–19 A future direction is the quantitative measurement of tissue elasticity using shear-wave ultrasound elastography.20
Color and Power Doppler
Most ultrasound machines have the option of color and power Doppler imaging, with possible spectral waveform analysis. Ultrasound uses the Doppler effect, in which the sound frequency of an object changes as the object travels toward or away from a point of reference, to obtain information about blood flow. Color flow imaging shows colored blood flow superimposed on a gray-scale image, in which two colors such as red and blue represent flow toward and away from the transducer, respectively (Fig. 1-28).21 Pulsed-wave or duplex Doppler ultrasound displays an ultrasound image and waveform (Fig. 1-29). There are important considerations to optimize the Doppler ultrasound. Reducing the width of the field of view and increasing the frame rate are helpful. To correct for aliasing (when the Doppler shift frequency of blood is greater than the detected frequency, which causes an error in frequency measurement), one can increase the pulse repetition frequency, lower the ultrasound frequency, or increase the angle between the sound beam and the flow direction toward perpendicular. Power Doppler is another method of color Doppler ultrasound that is more sensitive to blood flow (it shows small vessels and slow flow rates) compared with conventional color Doppler, and it assigns a color to blood flow regardless of direction (Fig. 1-30). Power Doppler is extremely sensitive to movement of the transducer, which produces a flash artifact. It is important to adjust the color gain optimally for Doppler imaging to avoid artifact if the setting is too sensitive and for false-negative flow if sensitivity is too low. To optimize power Doppler imaging, set the color background (without the gray-scale displayed) so that the lowest level of color nearly uniformly is present, with only minimal presence of the next highest color level.22
Increased blood flow on color or power Doppler imaging may occur with greater perfusion, inflammation, and neovascularity. In imaging soft tissues, color and power Doppler imaging are used to confirm that an anechoic tubular structure is a blood vessel and to confirm blood flow. When a mass is identified, increased blood flow may suggest neovascularity, possibly from malignancy (Fig. 1-31).23 Although the finding is nonspecific, a tumor without flow is more likely to be benign, and malignant tumors usually demonstrate increased flow and irregular vessels.24 With regard to superficial lymph nodes, either no flow or hilar flow is more common with benign lymph node enlargement, and spotted, peripheral, or mixed patterns of flow are more common with malignant lymph node enlargement (see Chapter 2).25 Color or power Doppler imaging is also helpful in the differentiation between complex fluid and a mass or synovitis; the former typically has no internal flow, and the latter may show increased flow.26 After treatment for inflammatory arthritis, color and power Doppler imaging can show interval decrease in flow, which would indicate a positive response.27 It is also important to use color Doppler imaging during a biopsy to ensure that major vessels are avoided.
Dynamic Imaging
One significant advantage of ultrasound over other static imaging methods, such as radiography, CT, and conventional MRI, is the dynamic capability. On a basic level, ultrasound evaluation can be directly guided by a patient’s history, symptoms, and findings at physical examination. In fact, regardless of the protocol followed for imaging a joint, it is essential that ultrasound is focused during one aspect of the examination over any area of point tenderness or focal symptoms.28 Once ultrasound examination is begun, the patient can directly give feedback with regard to pain or other symptoms with transducer pressure over an ultrasound abnormality. When a patient has a palpable abnormality, direct palpation under ultrasound visualization will ensure that the imaged abnormality corresponds to the palpable abnormality. Graded compression also provides additional information about soft tissue masses; lipomas are often soft and pliable (see Video 2-7).
1 Curry TSDJ, Murry RC. Christensen’s physics of diagnostic radiology, ed 4. Philadelphia: Lea and Febiger; 1990.
2 Erickson SJ. High-resolution imaging of the musculoskeletal system. Radiology. 1997;205:593–618.
3 Martinoli C, Derchi LE, Pastorino C, et al. Analysis of echotexture of tendons with US. Radiology. 1993;186:839–843.
4 Silvestri E, Martinoli C, Derchi LE, et al. Echotexture of peripheral nerves: correlation between US and histologic findings and criteria to differentiate tendons. Radiology. 1995;197:291–296.
5 Crass JR, van de Vegte GL, Harkavy LA. Tendon echogenicity: ex vivo study. Radiology. 1988;167:499–501.
6 Scanlan KA. Sonographic artifacts and their origins. AJR Am J Roentgenol. 1991;156:1267–1272.
7 Rubin JM, Adler RS, Bude RO, et al. Clean and dirty shadowing at US: a reappraisal. Radiology. 1991;181:231–236.
8 Hartgerink P, Fessell DP, Jacobson JA, et al. Full- versus partial-thickness Achilles tendon tears: sonographic accuracy and characterization in 26 cases with surgical correlation. Radiology. 2001;220:406–412.
9 Reynolds DL, Jr., Jacobson JA, Inampudi P, et al. Sonographic characteristics of peripheral nerve sheath tumors. AJR Am J Roentgenol. 2004;182:741–744.
10 Lin DC, Nazarian LN, O’Kane PL, et al. Advantages of real-time spatial compound sonography of the musculoskeletal system versus conventional sonography. AJR Am J Roentgenol. 2002;179:1629–1631.
11 Strobel K, Zanetti M, Nagy L, et al. Suspected rotator cuff lesions: tissue harmonic imaging versus conventional US of the shoulder. Radiology. 2004;230:243–249.
12 Lin EC, Middleton WD, Teefey SA. Extended field of view sonography in musculoskeletal imaging. J Ultrasound Med. 1999;18:147–152.
13 Kang CH, Kim SS, Kim JH, et al. Supraspinatus tendon tears: comparison of 3D US and MR arthrography with surgical correlation. Skeletal Radiol. 2009;38:1063–1069.
14 Klauser AS, Peetrons P. Developments in musculoskeletal ultrasound and clinical applications. Skeletal Radiol. 2009. Sep 3 [Epub ahead of print]
15 Klauser AS, De Zordo T, Feuchtner GM, et al. Fusion of real-time US with CT images to guide sacroiliac joint injection in vitro and in vivo. Radiology. 2010;256:547–553.
16 De Zordo T, Chhem R, Smekal V, et al. Real-time sonoelastography: findings in patients with symptomatic Achilles tendons and comparison to healthy volunteers. Ultraschall Med. 2010;31:394–400.
17 De Zordo T, Fink C, Feuchtner GM, et al. Real-time sonoelastography findings in healthy Achilles tendons. AJR Am J Roentgenol. 2009;193:W134–138.
18 De Zordo T, Lill SR, Fink C, et al. Real-time sonoelastography of lateral epicondylitis: comparison of findings between patients and healthy volunteers. AJR Am J Roentgenol. 2009;193:180–185.
19 Klauser AS, Faschingbauer R, Jaschke WR. Is sonoelastography of value in assessing tendons? Semin Musculoskelet Radiol. 2010;14:323–333.
20 Arda K, Ciledag N, Aktas E, et al. Quantitative assessment of normal soft-tissue elasticity using shear-wave ultrasound elastography. AJR Am J Roentgenol. 2011;197:532–536.
21 Boote EJ. AAPM/RSNA physics tutorial for residents: topics in US: Doppler US techniques: concepts of blood flow detection and flow dynamics. Radiographics. 2003;23:1315–1327.
22 Bude RO, Rubin JM. Power Doppler sonography. Radiology. 1996;200:21–23.
23 Bodner G, Schocke MF, Rachbauer F, et al. Differentiation of malignant and benign musculoskeletal tumors: combined color and power Doppler US and spectral wave analysis. Radiology. 2002;223:410–416.
24 Belli P, Costantini M, Mirk P, et al. Role of color Doppler sonography in the assessment of musculoskeletal soft tissue masses. J Ultrasound Med. 2000;19:823–830.
25 Wu CH, Shih JC, Chang YL, et al. Two-dimensional and three-dimensional power Doppler sonographic classification of vascular patterns in cervical lymphadenopathies. J Ultrasound Med. 1998;17:459–464.
26 Breidahl WH, Stafford Johnson DB, Newman JS, et al. Power Doppler sonography in tenosynovitis: significance of the peritendinous hypoechoic rim. J Ultrasound Med. 1998;17:103–107.
27 Ribbens C, Andre B, Marcelis S, et al. Rheumatoid hand joint synovitis: gray-scale and power Doppler US quantifications following anti-tumor necrosis factor-alpha treatment: pilot study. Radiology. 2003;229:562–569.
28 Jamadar DA, Jacobson JA, Caoili EM, et al. Musculoskeletal sonography technique: focused versus comprehensive evaluation. AJR Am J Roentgenol. 2008;190:5–9.