CHAPTER 31 Intercellular Junctions
The mechanical integrity of animal tissues such as epithelia, nerves, and muscles depends on the ability of the cells to interact with each other and the extracellular matrix. Plasma membrane specializations, called cellular junctions, mediate these interactions. Physical connections from the extracellular matrix or adjacent cells through these junctions and the associated cytoskeletal filaments inside cells impart mechanical strength to tissues.
Investigation of junctions began when microscopists and physiologists recognized that epithelial and muscle cells adhere to each other and the underlying extracellular matrix. They also discovered that some epithelia form a tight barrier between the luminal surface and the underlying tissue spaces. The physical basis of these interactions became clear during the 1960s, when electron micrographs of thin sections of vertebrate tissues revealed four types of intercellular junctions that connect the plasma membranes of adjacent cells (Table 31-1 and Fig. 31-1) and two types of junctions to bind to the extracellular matrix. Subsequent research established the molecular architecture of these junctions, each based on a different transmembrane protein:
Vertebrates use a selection of junctions that are suited to the physiological functions of each tissue. Columnar epithelial cells in the intestine interact with their neighbors using all four types of intercellular junctions (Fig. 31-1B-D). Belt-like tight junctions and adherens junctions encircle the apex of the cell. Desmosomes and gap junctions form patch-like lateral connections between the cells. Hemidesmosomes anchor the cells to the basal lamina. Stratified epithelial cells in the skin (Fig. 31-1A) emphasize desmosomes and intermediate filaments (Fig. 31-1B) to resist mechanical forces but also interact via claudins and adherens junctions. Desmosomes and adherens junctions link muscle cells to the surrounding basal lamina (see Fig. 29-18C). Gap junctions connect heart and smooth muscle cells but not skeletal muscle cells. Most nerve cells communicate chemically, but some use gap junctions for electrical communication.
Tight Junctions
Tight junctions, also called zonula occludens, occlude the extracellular space between epithelial cells, forming a tight, belt-like adhesive seal that selectively limits the diffusion of water, ions, and larger solutes as well as migration of cells (Fig. 31-2). Thus, they separate the interior of the body from the external world. Tight junctions also define the boundary between the biochemically distinct apical and basolateral domains of the plasma membrane of polarized epithelial cells. Many physiological processes (see Figs. 11-2 through 11-4) depend on the selective permeability of the two pathways across an epithelium: passive “paracellular” diffusion through tight junctions and transcellular movement made possible by the action of pumps, carriers, and channels located selectively in the apical and basolateral domains of the plasma membrane.
In electron micrographs of thin sections of tight junctions, the plasma membranes of adjacent cells appear to fuse together in a series of one or more contacts (Fig. 31-2). Early models of tight junctions proposed a fusion between the lipid bilayers of the two membranes to account for the barrier to ion diffusion, but freeze-fracture images revealed that the strands consist of integral membrane proteins. The contacts correspond to continuous strands of intramembranous particles that form a branching network in the plane of the lipid bilayer.
Transmembrane proteins forming the strands ob-served by freeze-fracture were difficult to identify until investigators found a monoclonal antibody that bound to the cytoplasmic side of the plasma membrane at tight junctions. Using this antibody, they isolated an integral membrane protein and named it occludin. The amino acid sequence of occludin suggested four transmembrane strands and two hydrophobic extracellular loops that are extremely rich in tyrosine and glycine residues (Fig. 31-3). The same group then discovered that mice lacking the single occludin gene survive with normal tight junctions, revealing the existence of other tight junction proteins. It is now believed that a family of more than 20 proteins, called claudins, constitutes the main structural proteins of tight junction strands. Claudins have four transmembrane sequences, but they are not related in sequence to occludin. Close association of claudins limits diffusion in the extracellular space between cells as well as diffusion of lipids and proteins in the plane of the membrane.
The cytoplasmic tails of claudins interact with numerous proteins with roles as scaffolds and in actin binding, signaling, and cell polarity. ZO-1, ZO-2, and ZO-3, peripheral membrane adapter proteins containing PDZ protein-interaction domains (see Fig. 25-11), interact with the long C-terminal cytoplasmic tail of occludin and JAM (junctional adhesion molecule). These ZO-adapters link the transmembrane proteins to cytoplasmic proteins including actin filaments, cingulin, and a small GTPase. ZO-2 and cingulin are specific for tight junctions, whereas ZO-1 also associates with cadherins in adherens junctions and connexins in gap junctions.
Tight junctions are the barrier that segregates different pumps, carriers, receptors, and lipids in the apical and basolateral domains of the plasma membrane. These domains allow polarized cells to create different extracellular environments in the basal and apical compartments. For example, intestinal epithelial cells take up nutrients from the lumen of the intestine and transport them into the extracellular space beneath the cells (see Fig. 11-2). Tight junctions and epithelial polarity are required for many physiological functions (see Figs. 11-3 and 11-4). Separating the apical and basal compartments also regulates some types of signaling. For instance, airway epithelial cells release the growth factor heregulin from the apical surface, but it activates cell growth only by activating the receptor tyrosine kinase erbB2 (see Fig. 25-4) on the basolateral surface when the epithelium is damaged or the tight junctions compromised.
The transepithelial barrier that is established by tight junctions is regulated by extracellular stimuli (e.g., hormones such as vasopressin and aldosterone and cytokines such as tumor necrosis factor; see Chapter 27), their downstream second messengers (e.g., Ca2+ and cyclic adenosine monophosphate [cAMP]; see Chapter 26), and effectors (e.g., protein kinases A and C; see Chapter 25). The mechanisms are not yet well understood, but posttranslational modifications of tight junctions might modulate their assembly. Another possibility is that tension on associated actin filaments might physically open passages through tight junctions. The metabolic state of the cell also influences tight junctions; depletion of ATP causes tight junctions to leak without destroying the barrier between the apical and basolateral domains of the plasma membrane. Cells migrating across epithelia, such as white blood cells moving from the blood to the connective tissue, open tight junctions locally without disrupting the tight seal across the epithelium (see Fig. 30-13). Migratory cells induce a localized increase in cytoplasmic Ca2+ in the epithelial cells that is required for opening the tight junctions.
Gap Junctions
For many years, the dominance of the cell theory in biology, which suggested that isolation of cells was a general principle, discouraged curiosity about the possibility of direct intercellular communication. Early electrophysiological experiments on nerves and skeletal muscles reinforced the widespread belief that cells were autonomous. By chance, the cells that were used in these experiments were electrically isolated and communicated exclusively by secreting chemical messengers that bound to receptors on target cells (see Figs 11-8 and 11-9). However, nerve and skeletal muscle cells were found to be exceptions to the general principle, which emerged only later, that cells in animal tissues communicate with each other by gap junctions. The generality of gap junctional communication means that sharing cytoplasmic components is common in animal cell biology. Cells in plant tissues also communicate with each other, but they use direct cytoplasmic connections, called plasmodesmata, rather than gap junctions (Box 31-1 and Fig. 31-4).
BOX 31-1 Plasmodesmata
Plants lack gap junctions, but many cells in plant tissues maintain cytoplasmic continuity with their neighbors through plasmodesmata, membrane-lined channels across the cell wall (Fig. 31-4). A strand of modified endoplasmic reticulum fills most of the pore. Specialized proteins on the cytoplasmic surfaces of the surrounding plasma membrane and central endoplasmic reticulum are thought to line the pore, although few molecular components of plasmodesmata have been identified. Most plasmodesmata form by incomplete cytokinesis, but secondary plasmodesmata can form independently.
In 1959, electrophysiological experiments on synapses between giant axons and the motor neurons that drive the flipper muscles of crayfish provided the first convincing evidence for direct electrical communication between cells. These electrical synapses transmit action potentials (see Fig. 11-6) directly from one cell to the next without the delay required for secretion and reception of a chemical transmitter. This, in turn, allows exceptionally fast responses to escape predators. Heart muscle cells were found to be connected by similar electrical junctions.
Over the next decade, three approaches revealed coupling between many nonexcitable cells. Using microelectrodes, physiologists established that plasma membrane depolarization of one cell was transmitted with little resistance to adjacent epithelial cells (Fig. 31-5), although the amplitude of the response declined with distance. Similarly, it was discovered that fluorescent molecules, radioactive tracers, and essential nutrients pass from the cytoplasm of one cell to the cytoplasm of neighboring cells. Electron microscopists associated low-resistance communication between cells with the presence of plasma membrane specializations that they called gap junctions owing to the regular 2-nm separation of the adjacent cell membranes (Fig. 31-6). Light microscopy with antibody probes is used to survey tissues for junctions, while fluorescent fusion proteins are the best approach to study gap junction assembly and dynamics.
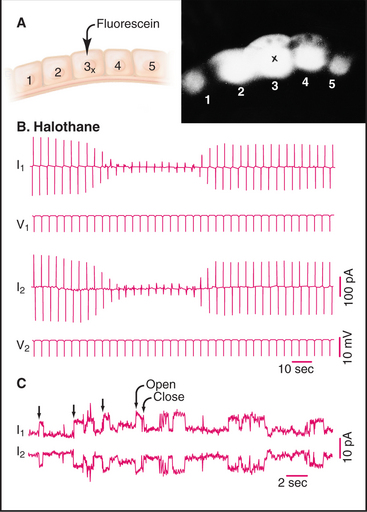
Figure 31-5 gap junction physiology. A, Drawing and fluorescence micrograph, showing the movement of a tracer dye between epithelial cells from the salivary gland of Chironomus. Cell 3 was injected with fluorescein (molecular weight: 330), which spread to adjacent cells via gap junctions. B–C, Electrical recordings from pairs of cells coupled by gap junctions.B, Two cells (1 and 2) were voltage-clamped (see the text that describes Fig. 11-6) and subjected alternately to small depolarizing voltage changes (V1, V2). Being electrically coupled, they responded with opposite currents (I1, I2). The anesthetic halothane closes most of the channels, reducing the current in response to depolarization. C, When the cells are held at a constant depolarizing voltage in the presence of halothane, current records reveal the opening and closing of individual gap junction channels as opposite step changes in current.
(A, From Lowenstein W: Physiol Rev 61:829, 1991.B, From Eghbali B, Kessler JA, Spray DC: Expression of gap junction channels in communication-incompetent cells. Proc Natl Acad Sci 87:1328–1331, 1990. C, Courtesy of Mark Ellisman, University of California, San Diego; reprinted with permission from Gaietta G, Deernick TJ, Adams SR, et al: Multicolor and electron microscopic imaging of connexin trafficking. Science 296:503–507, 2002. Copyright 2002 AAAS.)
Gap junctions are plaques that contain large intercellular channels that connect the cytoplasms of a pair of cells. These plaques exclude other transmembrane proteins and contain a few to thousands of channels. Half channels in each membrane are called connexons. They consist of six protein subunits, named connexins, which are proteins with four transmembrane α-helices (Fig. 31-7). Connexons assemble in vesicles along the secretory pathway. New connexons add around the periphery of gap junction plaques and old connexons are removed from the middle of plaques (Fig. 31-6C).
A hexagonal ring of connexins forms a central aqueous channel across the lipid bilayer. In the narrow intracellular gap, each connexon pairs with a connexon from the adjacent cell, forming a tight extracellular seal that precludes leakage of ions out of either cell. The use of six subunits creates a larger pore than tetrameric voltage-gated ion channels (see Fig. 10-7) or pentameric ligand-gated ion channels (see Fig. 10-12). The cylindrical transmembrane pore is 10 nm long with a diameter of 1.2 nm. This pore passes hydrophilic molecules up to about 1 kD in size, including ions (to establish electrochemical continuity between the cells), second messengers (to establish a common network of information), small peptides, and metabolites (to allow sharing of resources). Recent evidence shows that connexon hemichannels (the ring of six connexins in one plasma membrane) can open rarely for the nonspecific passage of ions and solutes as large as ATP.
Remarkably, gap junctions were invented twice during evolution. Connexins are found exclusively in chordates. The earliest metazoan branches (see Fig. 2-9) have a gene for proteins called innexins, for invertebrate connexins. Innexins have four transmembrane domains and form functional gap junctions but lack any sequence similarity to connexins. Vertebrates have a few innexin genes, expressed in the central nervous system.
In cells that express more than one connexin, the hexameric connexons may consist of one or more than one type of subunit. Most connexons pair with identical connexons on the partner cell to form homotypic gap junctional channels, but nonidentical pairs can form heterotypic channels with novel properties. Homotypic channels pass molecules equally well in both directions, but heterotypic channels can be asymmetrical. These hybrid channels may pass fluorescent tracers more readily in one direction than the other or react more sensitively to the transjunctional potential of one polarity than the other. This might explain the asymmetrical coupling that is sometimes observed between both excitable and nonexcitable cells, such as neuronal gap junctions, which pass action potentials in one direction but not the other.
Biophysicists have studied the properties of single connexon channels by patch-clamping pairs of cells with few channels, a state that can be achieved conveniently by expressing connexins in cells lacking them, or by measuring electrical properties of purified connexons incorporated into lipid bilayers (see Fig. 10-16A). Like other channels, connexons flip back and forth between two states: open and closed (Fig. 31-5). The structural basis for this difference in conductance is not yet established. The conductance of the open state depends on the connexin isoform and varies from about 30 pS to 300 pS. Given the permeability of gap junctions to relatively large solutes, it is surprising that their conductance is in the same range as narrower ligand- and voltage-gated ion channels. Both the greater length and the arrangement of charged residues lining the channel may contribute to the unexpectedly low conductance of connexons.
Gap junctional communication is conditional, depending on both the number of channels and the fraction that are open or closed. The fraction of open channels is usually less than 1.0; it is about 0.2 in heart and as low as 0.01 in one nerve cell that was tested. Many factors regulate the reversible opening and closing of connexon channels, including the transjunctional voltage, cytoplasmic H+ and Ca2+ concentrations, and protein kinases. Oleamide, a fatty acid amide produced by the brain, blocks gap junctional communication and induces sleep in animals. Organic alcohols (heptanol and octanol) and general anesthetics (halothane) can also close gap junction channels reversibly (see Fig. 31-5), but these agents are not specific for gap junctions. The transjunctional potential (i.e., the potential difference between the coupled cells) gates most connexons, regardless of the plasma membrane potentials of these cells. Like other voltage-gated channels, individual transitions are fast, but the response to potential changes, on the scale of seconds, is very slow in comparison with other channels (see Fig. 10-7). High concentrations of cytoplasmic Ca2+ (100 to 500 mM) and cytoplasmic acidification also close connexons. These effects of membrane potential, H+, and Ca2+ allow cells to terminate communication with neighboring cells that are damaged (depolarizing the plasma membrane and admitting high concentrations of Ca2+) or metabolically compromised (allowing Ca2+ to leak out of intracellular stores and acidifying the cytoplasm).
Second messengers generated by signaling pathways control gap junction activity in two ways. For example, on a time scale of hours, cAMP also promotes the assembly of gap junctions. On a time scale of seconds, cAMP activates protein kinase A, which phosphorylates the C-terminal tail of some connexins, increasing or decreasing the fraction of open channels (depending on the connexin isoform and the cell type). In the retina of the eye, the neurotransmitter dopamine) (see Fig. 11-7) regulates the size of a network of electrically coupled neurons. Dopamine activates a seven-helix receptor on these “horizontal cells,” stimulating the production of cAMP (see Fig. 26-1). This second messenger activates protein kinase A to phosphorylate connexons, reducing their open probability and the size of the neuronal network.
Cells in most metazoans communicate by gap junctions. Coupled cells in vertebrates include epithelial cells of the skin, endocrine glands, exocrine glands, gastrointestinal tract, and renal-urinary tract as well as smooth muscle, cardiac muscle, bone, some neurons, and glial cells. Epithelial cells can coordinate their activities with their neighbors, as in synchronizing the beats of cilia (see Fig. 38-14C). Fragments of viral proteins can spread from infected cells to neighboring cells, which then become targets for cytotoxic T lymphocytes (see Fig. 28-9). Gap junctions allow osteocytes buried deep in bone to maintain a cellular supply line to acquire nutrients from distant blood vessels (see Fig. 32-4). Passage of action potentials between cardiac and smooth muscle cells sets off waves of contraction (see Fig. 39-18). Electrical synapses between neurons can transmit action potentials at very high frequencies (>1000 per second). In some parts of the brain, gap junctions also coordinate action potentials in groups of neurons. Even white blood cells may form transient gap junctions with endothelial cells.
Mutations in connexin genes cause human disease and pathology in mice (Table 31-2). The defects are remarkably specific, considering that most connexins are expressed in several tissues. This might reflect situations in which other connexins cannot compensate or in which the absolute number of channels is crucial. Recessive mutations in the connexin-26 gene are the most common causes of inherited human deafness. As many as 1 in 30 people are carriers, and their mutations may contribute to hearing loss late in life. Connexin-26 participates in the transport of k+ in the epithelia supporting the sensory hair cells in the ear. Patients with one of a variety of mutations in the connexin-32 gene can suffer from degeneration of the myelin sheath around axons, an X-linked variant of Charcot-Marie-Tooth disease. Many human tissues express connexin-32, but the pathological processes are confined to myelin. The stability of myelin might depend on intracellular gap junctions between layers of the myelin sheath that provide a pathway between the metabolically active cell body and the deep layers of the sheath near the axon. (Defects in myelin membrane proteins cause other forms of Charcot-Marie-Tooth disease.) In contrast to humans, mice that lack connexin-32 have mild myelin defects but more serious defects in liver function (metabolic defects and a high incidence of tumors). Mice with null mutations in connexin-43, the main connexin of gap junctions in heart and other tissues, die shortly after birth. Their hearts beat, but a malformation of the heart is fatal. Other organs are only mildly abnormal.
Table 31-2 PHENOTYPES OF HUMANS AND MICE WITH MUTATIONS IN GAP JUNCTION SUBUNITS
Connexin | Species | Phenotype |
---|---|---|
Cx-26β2 | Human | Dominant and recessive mutations with deafness; skin disease |
Mouse | Embryonic lethal defect due to defective glucose transport across the placenta | |
Cx-30β6 | Human | Recessive deafness; skin disease |
Cx-31β3 | Human | Recessive deafness; skin disease |
Cx-32β1 | Human | X-linked point mutations, defective myelin, peripheral nerve degeneration; deafness |
Mouse | Defective liver glucose metabolism, liver tumors, mild nerve defect | |
Cx-37α4 | Mouse | Female infertility, defect in communication of granulosa cells with oocyte |
Cx-40α5 | Mouse | Partial block of impulse conduction in heart |
Cx-43α1 | Human | Deafness |
Mouse | Embryonic lethal heart defects (heterozygote mild heart conduction defect) | |
Cx-46α3 | Mouse | Cataracts in lens of the eye |
Cx-50α8 | Mouse | Cataracts in lens of the eye, small eyes |
Human | Cataracts in lens of the eye |
Note: Mutations are homozygous loss of function mutations unless noted otherwise. The nomenclature used here combines the Cx-“molecular mass in kDa” and molecular phylogeny αβ-number systems.
Adherens Junctions
Adherens junctions and desmosomes are two types of adhesive junctions using homophilic (like to like) interactions of cadherins (see Fig. 30-6) to bind epithelial cells to their neighbors. Cytoplasmic actin filaments reinforce adherens junctions (Fig. 31-8A), whereas cytoplasmic intermediate filaments anchor desmosomes (Fig. 31-8B).
Homophilic interactions between densely clustered E-cadherins bind adjacent cells together at adherens junctions. β-Catenin and a related protein called plakoglobin bind the cytoplasmic domains of E-cadherin (see Fig. 7-9F). β-Catenin not only regulates gene expression when it enters the nucleus as part of the Wnt signaling pathway (see Fig. 30-8) but also interacts with several cytoskeletal and signaling proteins associated with adherens junctions. α-Catenin has been a candidate to connect cadherins to actin filaments, since it can bind both β-catenin and actin filaments. However, these two interactions appear to be mutually exclusive, so the link between cadherins and actin is still under investigation.
Adherens junctions are the first connections that are established between developing sheets of epithelial cells. Contact begins when cadherins on the tips of filopodia engage partner cadherins of the same type on another cell. The contact spreads laterally as more cadherins are recruited along with associated actin filaments, as is illustrated by dorsal closure of the ectoderm by Drosophila embryos (see Fig. 38-5). These pioneering adherens junctions eventually allow like cells to associate in epithelial sheets (see Fig. 30-7) and to influence the maturation of the epithelium. Adherens junctions are a prerequisite for the tight junctions that allow epithelial cells to establish polarity with different proteins and lipids in the apical and basal plasma membranes. The shape of the cells depends on Rho family GTPases and protein kinases associated with the adherens junction, which regulate the assembly and contraction of the associated actin cytoskeleton. The junctions and polarity of the cells determine the orientation of the mitotic spindle and the plane of division. This allows for asymmetrical division of stem cells, such as those at the base of stratified epithelia (see Figs. 35-6 and 41-15). In mature columnar epithelia, a belt-like adherens junction, called the zonula adherens, encircles the cells near their apical surface (see Fig. 31-1D) and maintains the physical integrity of the epithelium.
Desmosomes
Desmosomes (desmos = “bound”, soma = “body”) provide strong adhesions between epithelial and muscle cells. In epithelia, these junctions are small, disk-shaped, “spot welds” between adjacent cells. Desmosomes in the heart are more complicated because they are mixed with adherens junctions (see Fig. 39-18). Cellular adhesion at desmosomes is mediated by two families of desmosomal cadherins, named desmogleins and desmocollins (see Fig. 30-5 and Table 30-2). These cadherins link the plasma membranes of adjacent cells and connect to cytoplasmic intermediate filaments via adapter proteins. The most distal of five extracellular CAD domains interact head to head with CAD1 domains from the partner cells and laterally with other cadherins in a dense tangle midway between the two plasma membranes (see Fig. 30-6).
Plakoglobin (also called γ-catenin, since it is similar to β-catenin) binds to the ICS domains of desmogleins and desmocollins, forming a link to desmoplakin, plakophilin, and intermediate filaments. Desmoplakin and accessory proteins link desmosomal cadherins to intermediate filaments. Desmoplakin I and II, dimeric proteins related to plectin (see Fig. 35-7), consist of a coiled-coil rod with globular domains at each end. N-terminal globular domains bind plakoglobin and desmosomal cadherins, whereas domains called “plakin repeats” at the C-terminus bind directly to the N-terminal, nonhelical domains of epidermal keratins. Mutations in this part of epidermal keratins can cause blistering skin diseases by compromising the integrity of desmosomes (see Fig. 35-6).
Although all desmosomes share a common plan, their molecular compositions vary in particular tissues. Mammals have four genes for desmogleins and three genes for desmocollins. Desmocollin mRNAs are also alternatively spliced (see Fig. 16-6). Desmoglein-2 and desmocollin-2 are found in most desmosomes. Expression of the other isoforms is more restricted. For example, in epidermis, desmoglein-1 and desmocollin-1 are found only in the upper layers, whereas desmoglein-3 is in the basal layers. This explains the pathology in autoimmune blistering diseases. Patients with pemphigus foliaceus make antibodies that react with desmoglein-1 and disrupt desmosomes in the upper layers of the epidermis, whereas patients with pemphigus vulgaris produce autoantibodies to desmoglein-3 that have the same effect on the basal layers. Antibodies are directly responsible; transfusion of human autoantibodies into a mouse reproduces the disease. Other organs are spared, owing to the restricted expression of these two isoforms. Mutations in these desmoglein genes in mice compromise desmosomes and cause skin blisters similar to pemphigus.
The development of animal tissues depends on desmosomes and their constituent proteins. Loss-of-function mutations can lead to mechanical failures; mutations in the plakoglobin gene can be lethal in mice and humans during embryogenesis, owing to disruption of the heart. Similarly, mutations in the desmoplakin gene cause skin and cardiac defects. Less direct evidence suggests that desmosomes might also transduce signals, perhaps deploying plakoglobin in a manner similar to that of β-catenin (see Fig. 30-8).
Adhesion to the Extracellular Matrix: Hemidesmosomes and Focal Contacts
Adhesion to the extracellular matrix is fundamentally different from intercellular adhesion because integrins, rather than homophilic interactions of cadherins, provide the transmembrane link between the cytoskeleton and ligands in the extracellular matrix (see Fig. 30-9). At focal contacts and related assemblies, transmembrane integrins link cytoplasmic actin filaments to the extracellular matrix (see Fig. 30-11).
Hemidesmosomes are another type of integrin-based adhesive junction that links cytoplasmic intermediate filaments to the basal lamina. The morphologic resemblance of hemidesmosomes to half of a conventional desmosome belies the fact that they are fundamentally different at the molecular level (Fig. 31-8). Like desmosomes, hemidesmosomes have a dense plaque on the cytoplasmic surface of the plasma membrane that anchors loops of intermediate filaments. The similarity ends there.
ACKNOWLEDGMENTS
Thanks go to James Anderson and Dan Goodenough for their suggestions on revisions to this chapter.
Cilia ML, Jackson D. Plasmodesmata form and function. Curr Opin Cell Biol. 2004;16:500-506.
Coulombe PA. A new fold on an old story: Attachment of intermediate filaments to desmosomes. Nat Struct Biol. 2002;9:560-562.
Dejana E. Endothelial cell-cell junctions. Nat Rev Mol Cell Biol. 2004;5:261-270.
Fleishman SJ, Unger VM, Yeager M, Ben-Tal N. A C-alpha model of the transmembrane alpha-helices of gap junction intercellular channels. Mol Cell. 2004;15:879-888.
Garrod DR, Merritt AJ, Nie Z. Desmosomal cadherins. Curr Opin Cell Biol. 2002;14:537-545.
Getsios S, Nuen AC, Green KJ. Working out the strength and flexibility of desmosomes. Nat Rev Mol Cell Biol. 2004;5:271-281.
Gonzalez-Mariscal L, Betanzoa A, Nava P, Jaramillo BE. Tight junction proteins. Prog Biophys Mol Biol. 2003;81:1-44.
Harris AL. Emerging issues in connexin channels: Biophysics fills the gap. Quart Rev Biophys. 2001;34:325-472.
Heinlein M, Epel BL. Macromolecular transport and signaling through plasmodesmata. Int Rev Cytol. 2004;235:93-164.
Jones JE, Hopkinson SB, Goldfinger LE. Structure and assembly of hemidesmosomes. BioEssays. 1998;20:488-494.
Knust E, Bossinger O. Composition and formation of intercellular junctions in epithelial cells. Science. 2002;298:1955-1959.
Payne AS, Hanakawa Y, Amagai M, Stanley JR. Desmosomes and disease: Pemphigus and bullous impetigo. Curr Opin Cell Biol. 2004;16:536-543.
Perez-Moreno M, Jamora C, Fuchs E. Sticky business: Orchestrat-ing cellular signals at adherens junctions. Cell. 2003;112:535-548.
Powell AM, Sakuma-Oyama Y, Oyama N, Black MM. Collagen XVII/BP180: A collagenous transmembrane protein component of the dermoepidermal anchoring complex. Clin Exp Derm. 2005;30:682-687.
Stout C, Goodenough DA, Paul DL. Connexins: Functions without junctions. Curr Opin Cell Biol. 2004;16:507-512.
Tsukita A, Furuse M. Claudin-based barrier in simple and stratified cellular sheets. Curr Opin Cell Biol. 2002;14:531-536.
Van Itallie CM, Anderson JM. The molecular physiology of tight junction pores. Physiology. 2004;19:331-338.
Wei C-J, Xu X, Lo CW. Connexins and cell signaling in development and disease. Annu Rev Cell Dev Biol. 2004;20:811-838.
Yap AS, Brieher WM, Gumbiner BM. Molecular analysis of cadherin-based adherens junctions. Annu Rev Cell Dev Biol. 1998;13:119-146.