Integumentary, Skeletal, and Muscular Systems
The construction of the tissues of the body involves developmental phenomena at two levels of organization. One is the level of individual cells, in which the cells that make up a tissue undergo increasing specialization through a process called cytodifferentiation (see discussion of restriction, determination, and differentiation, [p. 85]). At the next level of complexity, various cell types develop in concert to form specific tissues through a process called histogenesis. This chapter discusses the development of three important tissues of the body: skin, bone, and muscle. The histogenesis of each of these tissues exemplifies important aspects of development.
Integumentary System
The skin, consisting of the epidermis and dermis, is one of the largest structures in the body. The epidermis represents the interface between the body and its external environment, and its structure is well adapted for local functional requirements. Simple inspection of areas such as the scalp and palms shows that the structure of the integument varies from one part of the body to another. These local variations result from inductive interactions between the ectoderm and underlying mesenchyme. Abnormalities associated with the integumentary system are presented later in Clinical Correlation 9.1.
Epidermis
Structural Development
The outer layer of the skin begins as a single layer of ectodermal cells (Fig. 9.1A). As development progresses, the ectoderm becomes multilayered, and regional differences in structure become apparent.
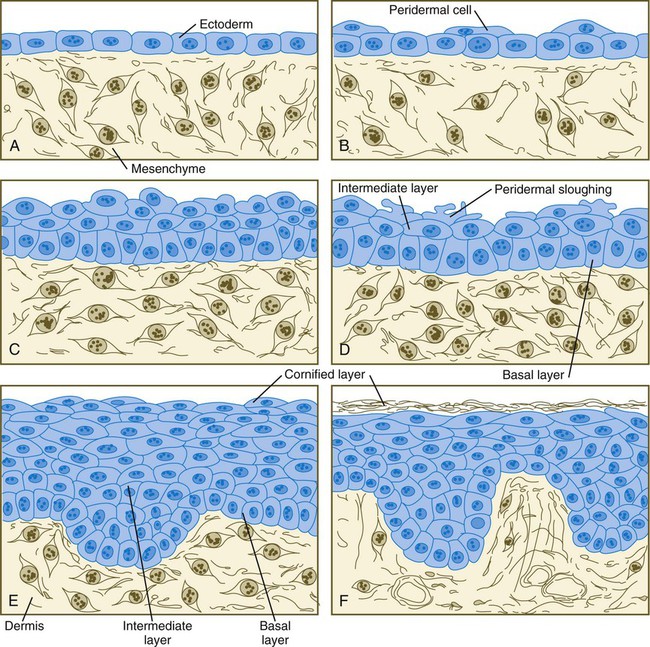
A, At 1 month. B, At 2 months. C, At

The first stage in epidermal layering is the formation of a thin outer layer of flattened cells known as the periderm at the end of the first month of gestation (Fig. 9.1B). Cells of the periderm, which is present in the epidermis of all amniote embryos, seem to be involved in the exchange of water, sodium, and possibly glucose between the amniotic fluid and the epidermis.
By the third month, the epidermis becomes a three-layered structure, with a mitotically active basal (or germinative) layer, an intermediate layer of cells (Fig. 9.1D) that represent the progeny of the dividing stem cells of the basal layer, and a superficial layer of peridermal cells bearing characteristic surface blebs (Fig. 9.2). Peridermal cells contain large amounts of glycogen, but the function of this glycogen remains uncertain.
Epidermal Differentiation
When the multilayered epidermis becomes established, a regular cellular organization and sequence of differentiation appear within it (Fig. 9.3). Stem cells* of the basal layer (stratum basale) divide and contribute daughter cells to the next layer, the stratum spinosum. The movement of epidermal cells away from the basal layer is preceded by a loss of adhesiveness to basal lamina components (e.g., fibronectin, laminin, and collagen types I and IV). These cellular properties can be explained by the loss of several integrins, which attach the basal cells to the underlying basal lamina. Cells of the stratum spinosum produce prominent bundles of keratin filaments, which converge on the patchlike desmosomes binding the cells to each other.
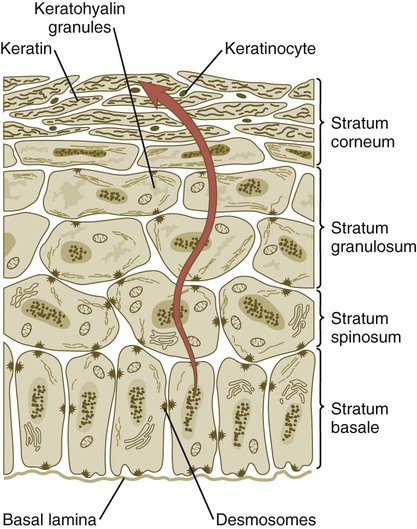
Cells arising in the stratum basale undergo terminal differentiation into keratinocytes as they move toward the surface. (Adapted from Carlson B: Patten’s foundations of embryology, ed 6, New York, 1996, McGraw-Hill.)
The formation of epidermal ridges is closely associated with the earlier appearance of volar pads on the ventral surfaces of the fingers and toes (Fig. 9.4). Volar pads first form on the palms at about weeks, and by
weeks, they have formed on the fingers. The volar pads begin to regress by about
weeks, but while they are present, they set the stage for the formation of the epidermal ridges, which occurs between 11 and 17 weeks. Similar events in the foot occur approximately 1 week later than those in the hand.
When the epidermal ridges first form, the tips of the digits are still smooth, and the fetal epidermis is covered with peridermal cells. Beneath the smooth surface, however, epidermal and dermal ridges begin to take shape (Fig. 9.5). Late in the fifth month of pregnancy, the epidermal ridges become recognizable features of the surface landscape.
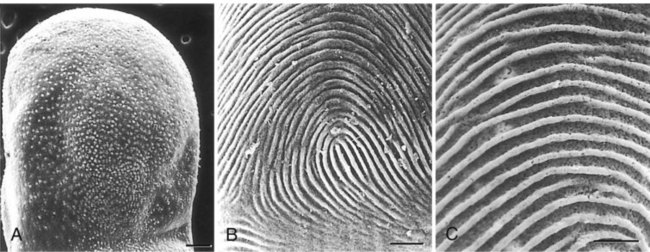
A, Low-power view of the palmar surface of a digit. B, Epidermal surface of the dermis of the fingertip showing the primary dermal ridges. C, Basal surface of the epidermis showing the epidermal ridges. Bars, 100 µm. (From Misumi Y, Akiyoshi T: Am J Anat 119:419, 1991.)
Dermis
The dermis arises from several sources. In the trunk, dorsal dermis arises from the dermatome of the somites, whereas ventral and lateral dermis and dermis of the limbs is derived from the lateral plate mesoderm. In the face, much of the cranial skin, and anterior neck, dermal cells are descendants of cranial neural crest ectoderm (see Fig. 12.9).
Ectodermal Wnt signaling, acting through the β-catenin pathway, specifies the dermomyotomal cells, as well as mesenchymal cells of the ventral somatopleure closest to the ectoderm, to become dermal cells, which express the dermal marker, Dermo 1 (see Fig. 9.8A). The future dermis is initially represented by loosely aggregated mesenchymal cells that are highly interconnected by focal tight junctions on their cellular processes. These early dermal precursors secrete a watery intercellular matrix rich in glycogen and hyaluronic acid.
Dermal-Epidermal Interactions
If ectoderm from one part of the body is combined with dermis from another area, the ectoderm differentiates into a regional pattern characteristic of underlying dermis, rather than a pattern appropriate for the site of origin of the ectoderm (Fig. 9.6). Cross-species recombination experiments have shown that, even in distantly related animals, skin ectoderm and mesenchyme can respond to each other’s inductive signals.
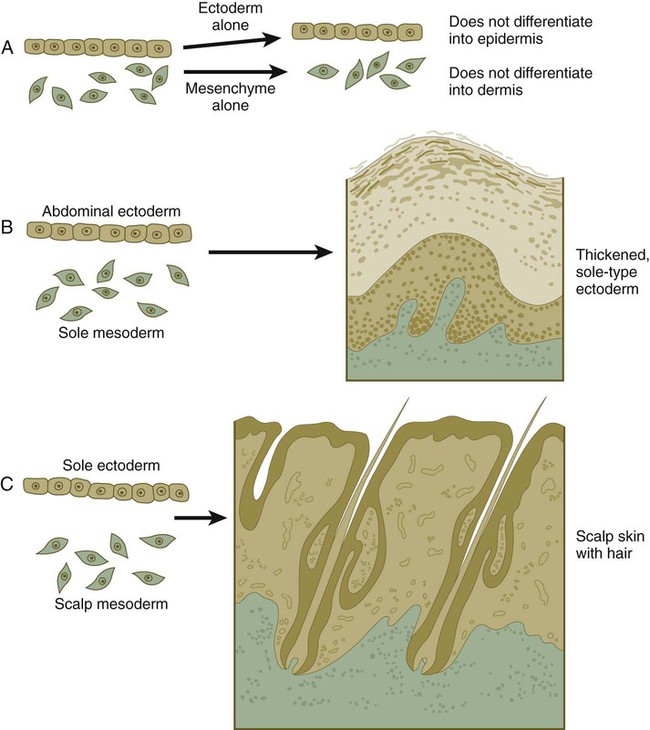
When separated (A), ectoderm and underlying mesenchyme do not differentiate. Recombinations (B and C) show that the dermis determines the nature of the ectodermal differentiation.
Epidermal Appendages
As a result of inductive influences by the dermis, the epidermis produces a wide variety of appendages, such as hair, nails, sweat and sebaceous glands, mammary glands, and the enamel component of teeth. (The development of teeth is discussed in Chapter 14.)
Hair
Hair formation is first recognizable at about the twelfth week of pregnancy as regularly spaced epidermal placodes associated with small condensations of dermal cells called dermal papillae (Fig. 9.7). Under the continuing influence of a dermal papilla, the placode forms an epidermal downgrowth (hair germ), which over the next few weeks forms an early hair peg. In succeeding weeks, the epidermal peg overgrows the dermal papilla, and this process results in the shaping of an early hair follicle. At this stage, the hair follicle still does not protrude beyond the outer surface of the epidermis, but in the portion of the follicle that penetrates deeply into the dermis, two bulges presage the formation of sebaceous glands, which secrete an oily skin lubricant (sebum), and are the attachment site for the tiny arrector pili muscle. The arrector pili is a mesodermally derived smooth muscle that lifts the hair to a nearly vertical position in a cold environment. In many animals, this increases the insulation properties of the hair. The developing hair follicle induces the adjacent dermal mesoderm to form the smooth muscle cells of this muscle. As the developing hair matures, a small bulge below the sebaceous gland marks an aggregation of epidermal stem cells (Fig. 9.7D).
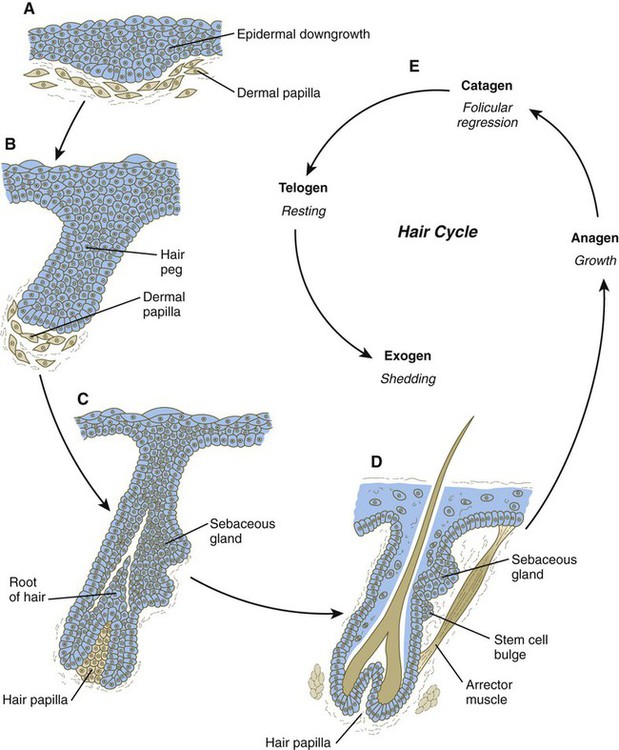
A, Hair primordium (12 weeks). B, Early hair peg (15 to 16 weeks). C, Bulbous hair follicle (18 weeks). D, Adult hair. E, The adult hair cycle.
The formation of a hair involves a series of inductive interactions mediated by signals that are only partly understood. When a dense condensation of dermal cells has formed beneath the ectoderm (Fig. 9.8A), the first of two dermal inductions results in the thickening of ectoderm in very regularly arranged locations to form epidermal placodes (Fig. 9.8B). Fibroblast growth factor (FGF) and Wnt (mainly Wnt-11) signaling from the dermis, along with the inactivation of local bone morphogenetic proteins (BMPs), stimulates the activation of other Wnts in the ectoderm to form an epidermal placode. The response of the ectoderm is to produce other Wnts, acting through β-catenin intermediates, and Edar, the receptor for the signaling molecule ectodysplasin. In the areas where hairs will not develop (interfollicular areas), placode formation is inhibited by locally produced BMPs and by the inhibition of Wnts by Dickkopf. How the epidermal placodes are spaced in such a geometrically regular fashion is still not well understood.
The newly formed epidermal placodes become the inducing agent and stimulate the aggregation of mesenchymal cells beneath the placode to form the dermal papilla (Fig. 9.8C). Sonic hedgehog, produced by the epidermal placode, seems to be involved in this induction, but the identity of other signals is unknown. Next, the dermal papilla initiates the second dermal induction by stimulating downgrowth of the cells of the epidermal placode into the dermis (Fig. 9.8D). Epidermal downgrowth, which involves considerable epidermal cellular proliferation, is stimulated by expression of sonic hedgehog by the epidermal cells and the subsequent expression of cyclin D1, part of the cell cycling pathway. Later formation of a hair is structurally and biochemically an extremely complex process, which, among other things, involves the expression of a range of Hox genes in specific locations and at specific times along the length of each developing hair.
Once formed, an individual hair follows a regular cycle of growth and shedding (see Fig. 9.7). During anagen, the first phase in the cycle, the hair is actively growing (around 10 cm per year). This phase can last up to 5 to 6 years. Then it enters catagen, a phase lasting 1 or 2 weeks, during which the hair follicle regresses to only a fraction of its original length. The hair stops growing in the resting phase (telogen), which lasts 5 to 6 weeks, after which time the hair is shed (exogen). Adjacent hairs are frequently in different phases of the hair cycle.
The pattern of epidermal appendages such as hairs has been shown experimentally to relate to patterns generated in the dermis. Other studies have compared patterns of scalp hairs between normal embryos and embryos with cranial malformations (Fig. 9.9) and have shown a correlation between whorls and the direction of hair growth and the tension on the epidermis at the time of formation of the hair follicles.
Nails
Toward the end of the third month, epidermal thickenings (primary nail field) on the dorsal surfaces of the digits mark the beginnings of nail development. Cells from the primary nail field expand proximally to undercut the adjacent epidermis (Fig. 9.10). Proliferation of cells in the proximal part of the nail field results in the formation of a proximal matrix, which gives rise to the nail plate that grows distally to cover the nail bed. The nail plate itself consists of highly keratinized epidermal cells. A thin epidermal layer, the eponychium, initially covers the entire nail plate, but it eventually degenerates, except for a thin persisting rim along the proximal end of the nail. The thickened epidermis underlying the distalmost part of the nail is called the hyponychium, and it marks the border between dorsal and ventral skin. Outgrowing fingernails reach the ends of the digits by about 32 weeks, whereas in toenails, this does not occur until 36 weeks.
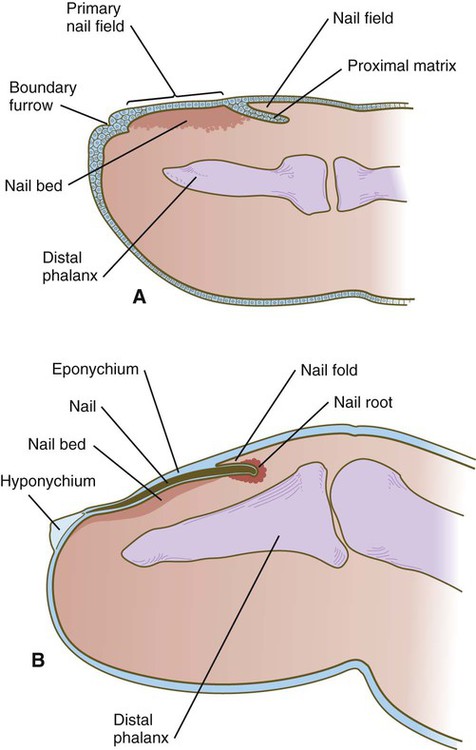
A, In the fourth month, the primary nail field overlies a mesenchymal nail bed and extends proximally as the proximal matrix. B, Close to term, the nail has grown close to the end of the fingertip. Much of the nail is covered by a thin eponychium, most of which will eventually degenerate.
Mammary Glands
As with many glandular structures, the mammary glands arise as epithelial (in this case, ectodermal) downgrowths into mesenchyme in response to inductive influences by the mesenchyme. The first morphological evidence of mammary gland development is the appearance of two bands of ectodermal thickenings called milk lines (part of the wolffian ridge [see p. 111]) running along the ventrolateral body walls in embryos of both genders at about 6 weeks (Fig. 9.11A). They are marked by the expression of various Wnts within the ectodermal cells. The thickened ectoderm of the milk lines undergoes fragmentation, and remaining areas form the primordia of the mammary glands. The craniocaudal level and the extent along the milk lines at which mammary tissue develops vary among species. Comparing the location of mammary tissue in cows (caudal), humans (in the pectoral region), and dogs (along the length of the milk line) shows the wide variation in location and number of mammary glands. In humans, supernumerary mammary tissue or nipples can be found anywhere along the length of the original milk lines (Fig. 9.11B). Individual mammary placodes form from aggregation and proliferation of ectodermal cells of the milk line under the inductive influence of the signaling molecule neuregulin-3. Their dorsoventral location is marked by the expression of the transcription factor Tbx-3.
Mammary ductal epithelial downgrowths (Fig. 9.12) are associated with two types of mesoderm: fibroblastic and fatty. The early epithelial downgrowth secretes parathyroid hormone–related hormone, which increases the sensitivity of the underlying mesenchymal cells to BMP-4. BMP-4 signals within the underlying mesenchyme have two principal effects (see Fig. 9.12B). First, they stimulate further downgrowth of the mammary epithelial bud. Second, they stimulate the expression of the transcription factor Msx-2, which inhibits the formation of hair follicles in the region of the nipple. Experimental evidence suggests that inductive interactions with the fatty component of the connective tissue are responsible for the characteristic shaping of the mammary duct system. As with many developing glandular structures, the inductive message seems to be mediated to a great extent by the extracellular matrix of the connective tissue.
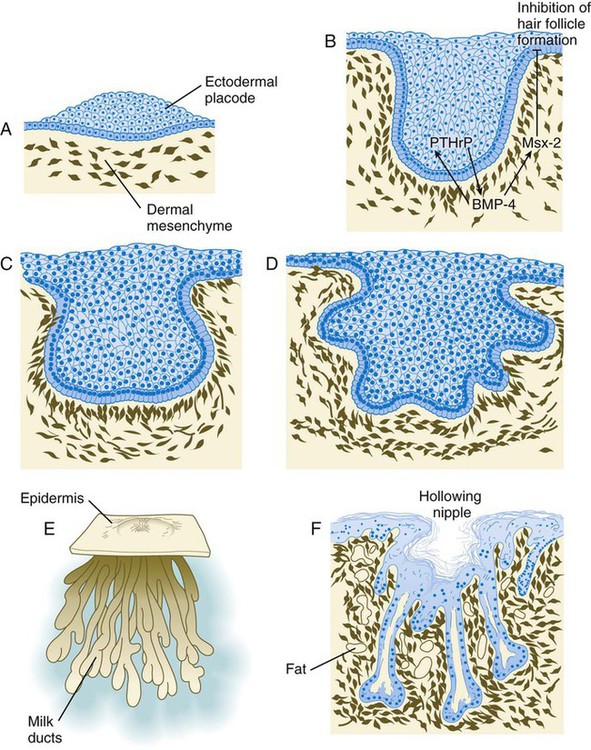
A, Sixth week. B, Seventh week. BMP-4, bone morphogenetic protein-4; PTHrP, parathyroid hormone–related protein. C, Tenth week. D, Fourth month. E, Sixth month. F, Eighth month.
The role of the mesoderm and testosterone receptors is well illustrated in experiments involving mice with a genetic mutant, androgen insensitivity syndrome. This is the counterpart of a human condition called the testicular feminization syndrome, in which genetic male individuals lack testosterone receptors. Despite having high circulating levels of testosterone, these individuals develop female phenotypes, including typical female breast development (Fig. 9.13A), because without receptors, the tissue cannot respond to the testosterone.
In vitro recombination experiments on mice with androgen insensitivity have been instrumental in understanding the role of the mesoderm in mediating the effects of testosterone on mammary duct development (Fig. 9.13B). If mutant mammary ectoderm is combined with normal mesoderm in the presence of testosterone, the mammary ducts regress, but normal ectoderm combined with mutant mesoderm continues to form normal mammary ducts despite being exposed to high levels of testosterone. This shows that the genetic defect in testicular feminization is expressed in the mesoderm.
The postnatal development of female mammary gland tissue is also highly responsive to its hormonal environment. The simple mammary duct system that was laid down in the embryo remains in an infantile condition until it is exposed to the changing hormonal environment at the onset of puberty (Fig. 9.14A). Increasing levels of circulating estrogens, acting on a base of growth hormone and insulinlike growth factor activity, stimulate the proliferation of the mammary ducts and enlargement of the pad of fatty tissue that underlie it (Fig. 9.14B). As is the case with testosterone effects, estrogen effects on the epithelium of the mammary ducts are mediated via paracrine influences from the mammary connective tissue stroma, which contains the estrogen receptors.
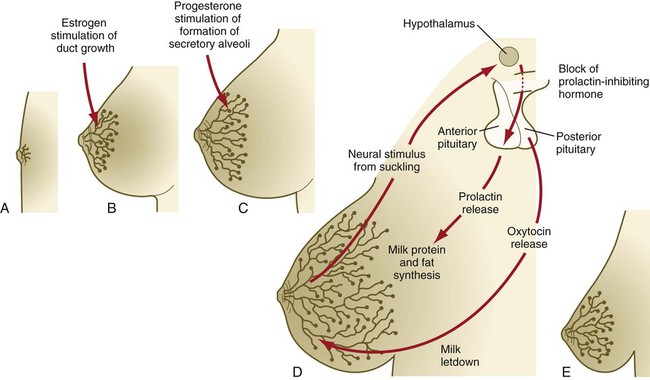
A, Newborn. B, Young adult. C, Adult. D, Lactating adult. E, Adult after lactation.
The next major change in the complete cycle of mammary tissue development occurs during pregnancy, although minor cyclical changes in mammary tissue are detectable in each menstrual cycle. During pregnancy, increased amounts of progesterone, along with prolactin and placental lactogen, stimulate the development of secretory alveoli at the ends of the branched ducts (Fig. 9.14C). With continuing development of the alveoli, the epithelial cells build up increased numbers of the cytoplasmic organelles, such as rough endoplasmic reticulum and the Golgi apparatus, which are involved in protein synthesis and secretion.
Lactation involves numerous reciprocal influences between the mammary glands and the brain; these are summarized in Figure 9.14D. Stimulated by prolactin secretion from the anterior pituitary, the alveolar cells synthesize milk proteins (casein and α-lactalbumin) and lipids. In a rapid response to the suckling stimulus, the ejection of milk is triggered by the release of oxytocin by the posterior portion of the pituitary. Oxytocin causes the contraction of myoepithelial cells, which surround the alveoli. Suckling also causes an inhibition of the release of luteinizing hormone–releasing hormone by the hypothalamus that results in the inhibition of ovulation and a natural form of birth control.
With cessation of nursing, reduced prolactin secretion and the inhibitory effects of nonejected milk in the mammary alveoli result in the cessation of milk production. The mammary alveoli regress, and the duct system of the mammary gland returns to the nonpregnant state (Fig. 9.14E).
Clinical Correlation 9.1 summarizes several types of anomalies that affect the integumentary system.
Skeleton
The deep skeletal elements of the body typically first appear as cartilaginous models of the bones that will ultimately be formed (Fig. 9.16). At specific periods during embryogenesis, the cartilage is replaced by true bone through the process of endochondral ossification. In contrast, the superficial bones of the face and skull form by the direct ossification of mesenchymal cells without an intermediate cartilaginous stage (intramembranous bone formation). Microscopic details of intramembranous and endochondral bone formation are presented in standard histology texts and are not repeated here.
To differentiate into defined skeletal elements, the mesenchymal precursor cells must often interact with elements of their immediate environment—typically epithelia with associated basal laminae—or components of the neighboring extracellular matrix. Details of the interactions vary among regions of the body. In the limb, a continuous interaction between the apical ectodermal ridge (see Chapter 10) and the underlying limb bud mesoderm is involved in the specification of the limb skeleton. An inductive interaction between the sclerotome and notochord or neural tube initiates skeletogenesis of the vertebral column. In the head, preskeletal cells of the neural crest may receive information at levels ranging from the neural tube itself, to sites along their path of migration, to the region of their final destination. Inductive interactions between regions of the brain and the overlying mesenchyme stimulate formation of the membrane bones of the cranial vault.
Regardless of the nature of the initial induction, the formation of skeletal elements begins along a common path, which diverges into osteogenic or chondrogenic programs, depending on the nature of the immediate environment. Shortly after induction, the preskeletal mesenchymal cells produce the cellular adhesion molecule N-cadherin, which promotes their transformation from a mesenchymal to an epitheliumlike morphology and their forming cellular condensations (Fig. 9.17). The growth factor transforming growth factor-β stimulates the synthesis of fibronectin and finally N-CAM, which maintains the aggregated state of the cells in the preskeletal condensation.
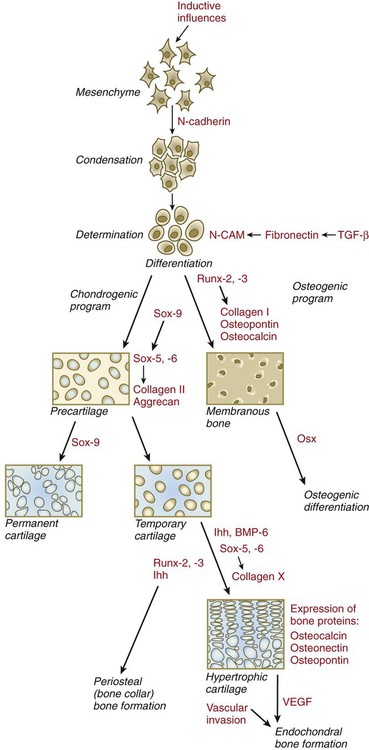
BMP-6, bone morphogenetic protein-6; CAM, cellular adhesion molecule; Ihh, Indian hedgehog; Osx, osterix; TGF-β, transforming growth factor-β; VEGF, vascular endothelial growth factor.
At this point, specific differentiation programs come into effect. If the skeletal element is destined to form membranous bone, the transcription factor Runx-2 sets off an osteogenic program (see Fig. 9.17). Osterix (Osx) is a downstream transcription factor from Runx-2 and is also required for the differentiation of osteoblasts. The protein encoded by the Runx2 gene has been shown to control the differentiation of mesenchymal cells into osteoblasts (bone-forming cells). These cells produce molecules characteristic of bone (type I collagen, osteocalcin, and osteopontin) and form spicules of intramembranous bone.
If the cellular condensation is destined to form cartilage, it follows the chondrogenic program. Under the influence of Sox-9, the chondroblasts begin to form type II collagen and secrete a cartilaginous matrix (see Fig. 9.17). Some embryonic cartilage (e.g., in the nose, ear, and intervertebral surfaces) remains as permanent cartilage and continues to express Sox-9. The cartilage that forms the basis for endochondral bone formation undergoes specific changes that ultimately promote bone formation around it. A first step is hypertrophy, which occurs under the influence of Runx-2 and the signaling factors Indian hedgehog and BMP-6. The formation of type X collagen is characteristic of hypertrophying cartilage. Then the hypertrophic chondrocytes themselves begin to produce bone proteins, such as osteocalcin, osteonectin, and osteopontin. They also express vascular endothelial growth factor, which stimulates the ingrowth of blood vessels into the hypertrophic cartilage. This sets the stage for the replacement of the eroded hypertrophic cartilage by true bone as osteoblasts accompany the invading capillaries. FGF-18, produced by the perichondrium, inhibits the maturation of the chondrocytes around the periphery of the mass of cartilage as those at the center are undergoing hypertrophy.
Axial Skeleton
Vertebral Column and Ribs
The earliest stages in establishing the axial skeleton are introduced in Chapter 6. Formation of the axial skeleton is more complex, however, than the simple subdivision of the paraxial mesoderm into somites and the medial displacement of sclerotomal cells to form primordia of the vertebrae. Each vertebra has a complex and unique morphology specified by controls operating at several levels and during several developmental periods.
According to the traditional view of vertebral development (see Fig. 6.13), the sclerotomes split into cranial and caudal halves, and the densely packed caudal half of one sclerotome joins with the loosely packed cranial half of the next to form the centrum of a vertebra. More recent morphological research suggests that vertebral development is more complex than this model.
The vertebral column is divided into several general areas (see Fig. 9.16): (1) an occipital region, which is incorporated into the bony structure of the base of the skull; (2) a cervical region, which includes the highly specialized atlas and axis that link the vertebral column to the skull; (3) the thoracic region, from which the true ribs arise; (4) the lumbar region; (5) a sacral region, in which the vertebrae are fused into a single sacrum; and (6) a caudal region, which represents the tail in most mammals and the rudimentary coccyx in humans. A typical vertebra arises from the fusion of several cartilaginous primordia. The centrum, which is derived from the ventromedial sclerotomal portions of the paired somites (see Fig. 6.10), surrounds the notochord and serves as a bony floor for the spinal cord (Fig. 9.18). The neural arches, arising from dorsal sclerotomal cells, fuse on either side with the centrum and, along with other neural arches, form a protective roof over the spinal cord. Incomplete closure of the bony roof results in a common anomaly called spina bifida occulta (see Fig. 11.43B). The costal process forms the true ribs at the level of the thoracic vertebrae. At other levels along the vertebral column, the costal processes become incorporated into the vertebrae proper.
The fundamental regional characteristics of the vertebrae are specified by the actions of discrete combinations of homeobox-containing genes (Fig. 9.19). Expression of the Hox genes begins with the first appearance of the presomitic mesoderm and for most genes persists until chondrification begins in the primordia of the vertebrae. Formation of the normal segmental pattern along the craniocaudal axis of the vertebral column is ensured by the fact that most vertebrae are specified by a unique combination of Hox genes. For example, in the mouse, the atlas (C1) is characterized by the expression of Hoxa1, Hoxa3, Hoxb1, and Hoxd4. The axis (C2) is specified by these four genes plus Hoxa4 and Hoxb4.
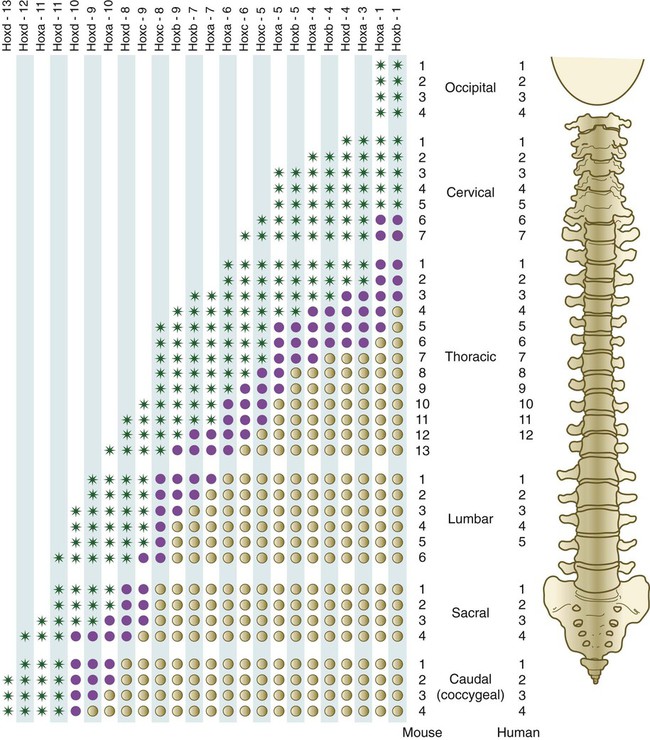
The vertebral column of the mouse (left) has one more thoracic and one more lumbar vertebra than the vertebral column of the human. Green asterisks indicate levels at which there is definite expression of the Hox gene indicated at the top of the column. Purple circles represent the caudal border where expression fades out. Tan circles represent areas of no expression of the Hox gene. (Based on studies by Kessel M, Balling R, Gruss P: Cell 61:301-308, 1990.)
A clear association exists between major regional boundaries in the axial skeleton and the anterior expression boundaries of certain Hox paralogues (Table 9.1). Retinoic acid (vitamin A) can cause shifts in cranial or caudal levels in the overall segmental organization of the vertebrae if it is applied at specific developmental periods. For example, if administered early, retinoic acid results in a cranial shift (the last cervical vertebra is transformed into the first thoracic vertebra), and later administration causes a caudal shift (thoracic vertebrae extend into the levels of the first two lumbar vertebrae). Such shifts in level are called homeotic transformations and are representative of the broad family of homeotic mutants described in Chapter 4.
Table 9.1
Regional Boundary | Hox Paralogue |
Occipital-cervical | Hox3 |
Cervical-thoracic | Hox6 |
Attached-floating ribs | Hox9 |
Thoracic-lumbar | Hox10 |
Lumbar-sacral | Hox11 |
Sacral-coccygeal | Hox13 |
Both the degree of control of axial level and the degree of redundancy of this control by paralogues of the Hox genes are illustrated by experiments in which either some or all of the components of a specific Hox paralogue are knocked out. When single Hox genes are knocked out, only minor morphological effects are noted. When all the members of a paralogous group are inactivated, however, profound effects appear. When all the Hox10 paralogues are knocked out, ribs form on all the lumbar and sacral vertebrae. This finding suggests that Hox10 represses the influence of the more anterior Hox genes. Similarly, Hox11 suppresses the influence of Hox10 and allows the sacrum to form. One of the striking features of axial development is the redundancy of the genes that pattern the vertebrae. A mutant of a specific Hox gene is likely to produce only a minor anatomical defect, whereas the nonfunction of an entire paralogous group produces major effects. Overall, a single paralogous group is involved in the patterning of 6 to 10 consecutive vertebrae, and the actions of at least 2 paralogous groups are involved in the formation of any individual vertebra. Control of posterior extension of the axial skeleton is balanced by the actions of the Cdx family of homeobox genes, which promote extension of the axial skeleton, and the Hox13 paralogue, which exerts a braking effect on such extension. In other structures, (e.g., limbs and external genitalia) Hox-13 gene products are associated with terminal growth. Some common anomalies of the vertebrae are discussed in Clinical Correlation 9.2.
Among the vertebrae, the axis and atlas have an unusual morphology and a distinctive origin (Fig. 9.22). The centrum of the atlas is deficient, but the area of the centrum is penetrated by the protruding odontoid process of the axis. The odontoid process consists of three fused centra that are presumably equivalent: (1) a half-segment from the centrum of a transitional bone (the proatlas) not found in humans, (2) the centrum that should have belonged to the atlas, and (3) the normal centrum of the axis. This arrangement permits a greater rotation of the head about the cervical spine. When the ubiquitously expressed Hoxa7 transgene was introduced into the germline of mice, the cranial part of the vertebral column was posteriorized. The base of the occipital bone was transformed into an occipital vertebra (the proatlas), and the atlas was combined with its centrum; the result was an axis that did not possess an odontoid process.
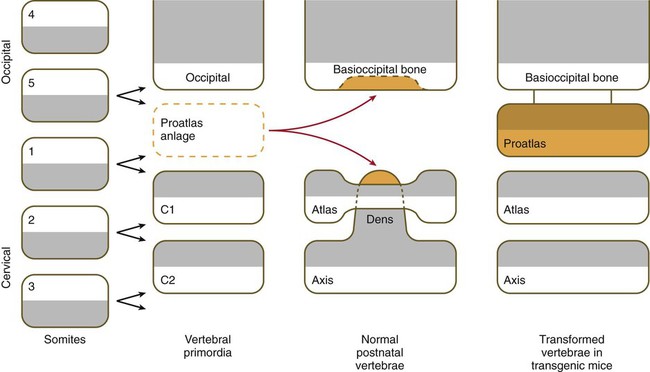
In normal development, cells from a proatlas anlage contribute to the formation of the basioccipital bone and the dens of the axis. The normal atlas forms an anterior arch (only a transient structure in other vertebrae) instead of a centrum. The cells that would normally form the centrum at the level of the atlas instead fuse with the axis to form the dens of the axis. In mice containing the Hoxa7 (A7) transgene, a proatlas forms, and the atlas and axis have the form of typical vertebrae (right column). (Based on Kessel M, Balling R, Gruss P: Cell 61:301-308, 1990.)
The ribs arise from zones of condensed mesenchymal cells lateral to the centrum. The proximal part of a rib (head, neck, and tubercle) arises from the central sclerotome (see Box 6.1). Because of the resegmentation of the somites as they form the vertebrae (see Fig. 6.13), the distal part (shaft) of the rib is derived from the lateral part of the adjacent cranial somite. By the time ossification in the vertebrae begins, the ribs separate from the vertebrae.
The formation of the proximal portions of the ribs depends on gene expression in the myotome. Products of the Hox-6 paralogous group promote the expression of two myogenic regulatory factors, Myf-5 and Myf-6 (see p. 184), in the myotomes of the thoracic level somites. Myf-5 and Myf-6 stimulate the formation of the growth factors, platelet-derived growth factor (PDGF) and FGF, which promote proximal rib growth in the sclerotome. Formation of the distal portion of the ribs requires BMP signals from the adjacent somatopleural mesoderm.
The sternum, which along with the connective tissue surrounding the distal ribs is derived from lateral plate mesoderm, arises as a pair of cartilaginous bands that converge at the ventral midline as the ventral body wall consolidates (Fig. 9.23). After the primordial sternal bands come together, they reveal their true segmental nature by secondarily subdividing into craniocaudal elements. Such secondary segmentation follows an early morphological and molecular course that closely parallels the formation of synovial joints (see p. 206). These segments ultimately fuse as they ossify to form a common unpaired body of the sternum. Several common anomalies of the sternum (e.g., split xiphoid process or split sternum [see Fig. 15.39]) are readily understood from its embryological development. Anterior borders of the Hox code that guide the development of the sternum from lateral plate mesoderm are offset from those in the paraxial mesoderm. Malformations of the xiphoid process are seen in mice mutant for Hoxc4 and Hoxa5, and mice mutant for Hoxb2 and Hoxb4 have split sternums.
The clavicle, which arises from neural crest and forms by an intramembranous mechanism, is one of the first bones in the body to become ossified, and ossification is well advanced by the eighth week. Studies of mice heterozygous for the Runx2 gene have shed light not only on the nature of the clavicle, but also on a poorly understood human syndrome. Such heterozygotes exhibit hypoplasia of the clavicle, delayed ossification of membrane bones (e.g., of the skull), and open anterior and posterior fontanelles in the skull. Cleidocranial dysplasia in humans exhibits all these conditions, as well as supernumerary teeth. Without clavicles, affected individuals can approximate their shoulders in the anterior midline (Fig. 9.24). The findings in this mutant suggest that the clavicle is a purely membranous bone and may be a unique class of bone, being neither truly axial nor appendicular in the usual sense.
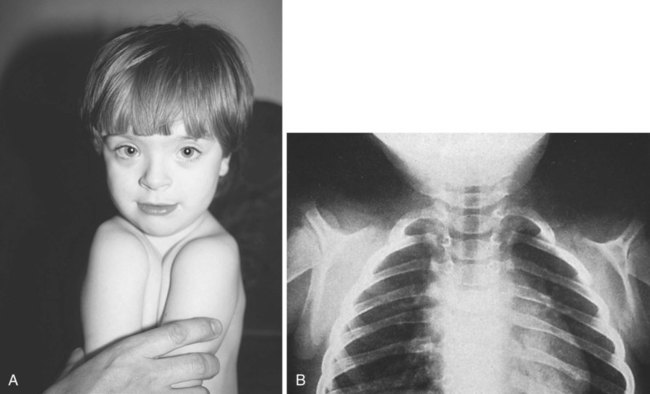
Absence of clavicles in individuals with mutants in Runx-2. A, In this boy, note the ability to approximate the shoulders without the clavicles present. B, In this radiograph, note the absence of clavicles. (A, From Turnpenny P, Ellard S: Emery’s elements of medical genetics, ed 14, Philadelphia, 2012, Churchill Livingstone; B, from the Robert J. Gorlin Collection, Division of Oral and Maxillofacial Pathology, University of Minnesota Dental School, courtesy of Dr. Ioannis Koutlas.)
The caudal end of the axial skeleton is represented by a well-defined, tail-like appendage during much of the second month (Fig. 9.25A). During the third month, the tail normally regresses, largely through cell death and differential growth, to persist as the coccyx, but rarely a well-developed tail persists in newborns (Fig. 9.25B).
Skull
The phylogenetic and ontogenetic foundation of the skull is represented by the chondrocranium, which is the cartilaginous base of the neurocranium (Fig. 9.26A). The fundamental pattern of the chondrocranium has been remarkably preserved in the course of phylogeny. It is initially represented by several sets of paired cartilages. One group (parachordals, hypophyseal cartilages, and trabeculae cranii) is closely related to midline structures. Caudal to the parachordal cartilages are four occipital sclerotomes. Along with the parachordal cartilages, the occipital sclerotomes, which are homologous with precursors of the vertebrae, fuse to form the base of the occipital bone. More laterally, the chondrocranium is represented by pairs of cartilage that are associated with epithelial primordia of the sense organs (olfactory organ, eyes, and auditory organ). Molecular signals from the preoral gut endoderm are required for chondrification of the chondrocranium rostral to the tip of the notochord, which is of neural crest origin, whereas caudal to the tip of the notochord, notochordal signals promote chondrification of the mesodermally derived posterior chondrocranium.
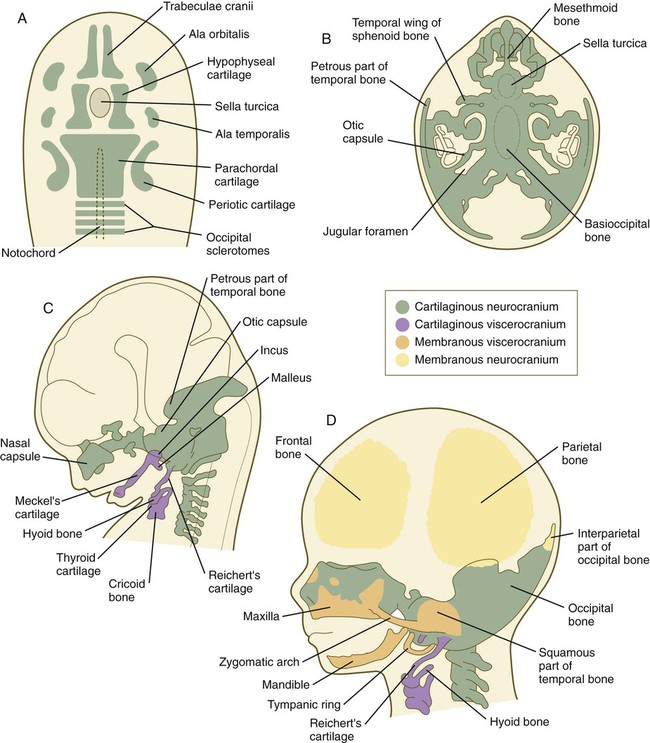
A, Basic skeletal elements of a 6-week embryo viewed from above. B, Chondrocranium of an 8-week embryo viewed from above. C, Lateral view of the embryo illustrated in B. D, Skull of a 3-month embryo. (Adapted from Carlson B: Patten’s foundations of embryology, ed 6, New York, 1996, McGraw-Hill.)
The individual primordial elements of the chondrocranium undergo several patterns of growth and fusion to form the structurally complex bones of the basicranium (the occipital, sphenoid, and temporal bones and much of the deep bony support of the nasal cavity) (Fig. 9.26B). In addition, some of these bones (e.g., the occipital and temporal bones) incorporate membranous components during their development, so in their final form, they are truly composite structures (see Fig. 9.26D). Other components of the neurocranium, such as the parietal and frontal bones, are purely membranous bones (Box 9.1).
Elements of the membranous neurocranium (the paired parietal and frontal bones and the interparietal part of the occipital bone) arise as flat, platelike aggregations of bony spicules (trabeculae) from mesenchyme that has been induced by specific parts of the developing brain. These bones remain separate structures during fetal development, and even at birth, they are separated by connective tissue sutures. Intersections between sutures where more than two bones meet are occupied by broader areas of connective tissue called fontanelles. The most prominent fontanelles are the anterior fontanelle, located at the intersection of the two frontal and two parietal bones, and the posterior fontanelle, located at the intersection of the parietal bones and the single occipital bone (Fig. 9.27).
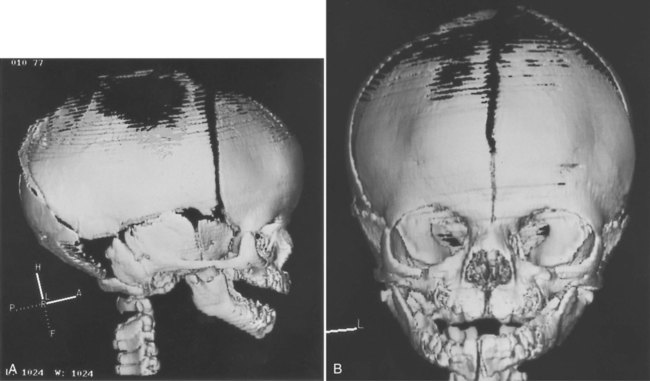

A, Lateral view. B, Frontal view. The midline fissure in the forehead area is the metopic suture, which normally becomes obliterated after birth. The irregular black area above that is the anterior fontanelle, one of the “soft spots” in a newborn’s head. (Courtesy of R.A. Levy, H. Maher, and A.R. Burdi, Ann Arbor, Mich.)
Similar to the neurocranium, the viscerocranium consists of two divisions: a cartilaginous viscerocranium and a membranous viscerocranium. In contrast to much of the neurocranium, the bones of the viscerocranium originate largely from neural crest–derived mesenchyme. Phylogenetically, the viscerocranium is related to the skeleton of the branchial arches (gill arches). Each branchial arch (more commonly called a pharyngeal arch in humans) is supported by a cartilaginous rod, which gives rise to numerous definitive skeletal elements characteristic of that arch (see Box 9.1). (Details of the organization and derivatives of the noncranial pharyngeal arch cartilages are discussed in Chapter 14 [see Fig. 14.36].)
The membranous viscerocranium consists of a series of bones associated with the upper and lower jaws and the region of the ear (see Fig. 9.26D). These arise in association with the first arch cartilage (Meckel’s cartilage) and take over some of the functions originally subserved by Meckel’s cartilage and many new functions, such as sound transmission in the middle ear. Clinical Correlation 9.3 discusses conditions resulting from skull deformities.
Appendicular Skeleton
The appendicular skeleton consists of the bones of the limbs and limb girdles. There are fundamental differences in organization and developmental control between the axial and the appendicular skeleton. The axial skeleton forms a protective casing around soft internal tissues (e.g., brain, spinal cord, pharynx), and the mesenchyme forming the bones is induced by the organs that the bones surround. In contrast, the bones of the appendicular skeleton form a central supporting core of the limbs. Although interaction with an epithelium (the apical ectodermal ridge of the limb bud [see Chapter 10]) is required for the formation of skeletal elements in the limb, morphogenetic control of the limb is inherent in the mesoderm, with the epithelium playing only a stimulatory role. All components of the appendicular skeleton begin as cartilaginous models, which convert to true bone by endochondral ossification later during embryogenesis. (Details of the formation of the appendicular skeleton are given in Chapter 10.)
Several defined genetic mutations result in prominent disturbances in the development of the appendicular skeleton. The most common form of dwarfism, achondroplasia, results from mutations of the FGF receptor 3 gene (FGFR3). This condition is characterized by short stature secondary to limb shortening, midface hypoplasia, a disproportionately large head and pronounced lumbar lordosis (Fig. 9.28). A more severe consequence of the same mutation is thanatophoric dysplasia, in which the shortening of the extremities is even more severe. The thorax is very narrow, and death from respiratory insufficiency usually occurs in infancy. A mutation of SOX-9 (see Fig. 9.17) causes campomelic dysplasia, characterized by pronounced bowing of the limbs, a variety of other skeletal anomalies, and sex reversal in XY males, resulting from a disruption of SOX-9 in sexual differentiation.
Muscular System
Three types of musculature—skeletal, cardiac, and smooth—are formed during embryonic development. Virtually all skeletal musculature is derived from the paraxial mesoderm, specifically the somites or somitomeres. Splanchnic mesoderm gives rise to the musculature of the heart (cardiac muscle) and the smooth musculature of the gut and respiratory tracts (Table 9.2). Other smooth muscle, such as that of the blood vessels and the arrector pili muscles, is derived from local mesenchyme.
Table 9.2
Embryological Origins of the Major Classes of Muscle
Embryological Origin | Derived Muscle | Innervation |
Somitomeres 1 through 3 and prechordal plate | Most extrinsic eye muscles | Cranial nerves III and IV |
Somitomere 4 | Jaw-closing muscles | Cranial nerve V (mandibular branch) |
Somitomere 5 | Lateral rectus muscle of eye | Cranial nerve VI |
Somitomere 6 | Jaw-opening and other second-arch muscles | Cranial nerve VII |
Somitomere 7 | Third-arch branchial muscles | Cranial nerve IX |
Somites 1 and 2 | Intrinsic laryngeal muscles and pharyngeal muscles | Cranial nerve X |
Occipital somites (1 through 7) | Muscles of tongue, larynx, and neck | Cranial nerves XI and XII, cranial cervical nerves |
Trunk somites | Trunk muscles, diaphragm, and limb muscles | Spinal nerves |
Splanchnic mesoderm | Cardiac muscle | Autonomic |
Splanchnic mesoderm | Smooth muscles of gut and respiratory tract | Autonomic |
Local mesenchyme | Other smooth muscle: vascular, arrector pili muscles | Autonomic |
From Carlson BM: Patten’s foundations of embryology, ed 6, New York, 1996, McGraw-Hill.
Skeletal Muscle
There is increasing evidence that certain cells of the epiblast are determined to become myogenic cells even before the somites are completely formed, but it is convenient to begin with the emergence of muscle precursor cells in the somites. For many decades, the origin of the skeletal musculature was in question, with the somites and lateral plate mesoderm being likely candidates. This issue was finally resolved by tracing studies involving cellular markers (Box 9.2), and it is now known that virtually all skeletal muscle originates in somites or somitomeres. Early steps in the determination of myogenic cells in somites are summarized in Figure 6.11.
Determination and Differentiation of Skeletal Muscle
The mature skeletal muscle fiber is a complex multinucleated cell that is specialized for contraction. Precursors of most muscle lineages (myogenic cells) have been traced to the myotome of the somite (see Fig. 6.10). Although these cells look like the mesenchymal cells that can give rise to many other cell types in the embryo, they have undergone a restriction event committing them to the muscle-forming line. Committed myogenic cells pass through several additional mitotic divisions before completing a terminal mitotic division and becoming postmitotic myoblasts.
Proliferating myogenic cells are kept in the cell cycle through the action of growth factors, such as FGF and transforming growth factor-β. With the accumulation of myogenic regulatory factors (see next section), myogenic cells upregulate the synthesis of the cell cycle protein p21, which irreversibly removes them from the cell cycle. Under the influence of other growth factors, such as insulinlike growth factor, the postmitotic myoblasts begin to transcribe the mRNAs for the major contractile proteins actin and myosin, but the major event in the life cycle of a postmitotic myoblast is its fusion with other similar cells into a multinucleated myotube (Fig. 9.32). The fusion of myoblasts is a precise process involving their lining up and adhering by calcium (Ca++)–mediated recognition mechanisms, involving molecules such as M-cadherin, and the ultimate union of their plasma membranes.
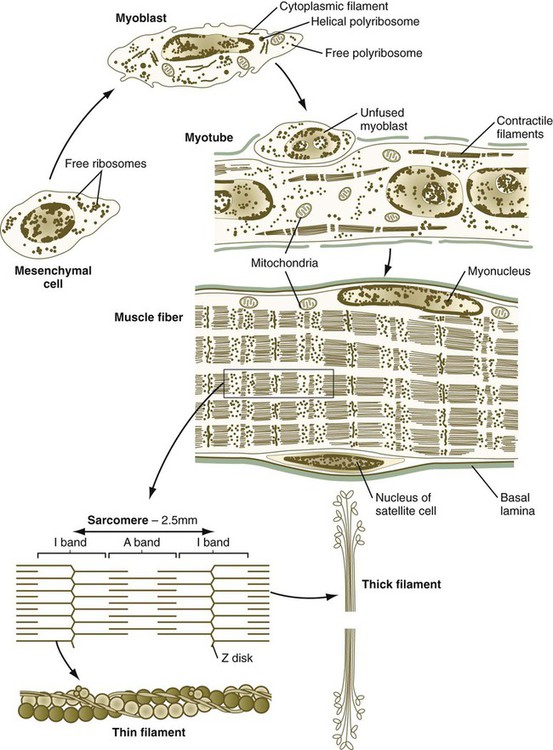
Important subcellular elements in a muscle fiber are also shown.
The development of a muscle fiber is not complete, however, with the peripheral migration of the nuclei of the myotube. The nuclei (myonuclei) of a multinucleated muscle fiber are no longer able to proliferate, but the muscle fiber must continue to grow in proportion to the rapid growth of the fetus and then the infant. Muscle fiber growth is accomplished by means of a population of myogenic cells, called satellite cells, which take up positions between the muscle fiber and the basal lamina in which each muscle fiber encases itself (see Fig. 9.32). Operating under a poorly understood control mechanism, possibly involving the Delta/Notch signaling system, satellite cells divide slowly during the growth of an individual. Some of the daughter cells fuse with the muscle fiber so that it contains an adequate number of nuclei to direct the continuing synthesis of contractile proteins required by the muscle fiber. After muscle fiber damage, satellite cells proliferate and fuse to form regenerating muscle fibers.
Muscle Transcription Factors
The first-discovered family of myogenic regulatory factors is a group of four basic helix-loop-helix transcription factors, sometimes called the MyoD family (Fig. 9.33). Another regulatory factor, called muscle enhancer factor-2 (MEF-2), works in concert with the MyoD family, but all these myogenic regulatory factors are capable of converting nonmuscle cells (e.g., fibroblasts, adipocytes, chondrocytes, retinal pigment cells) to cells expressing the full range of muscle proteins.
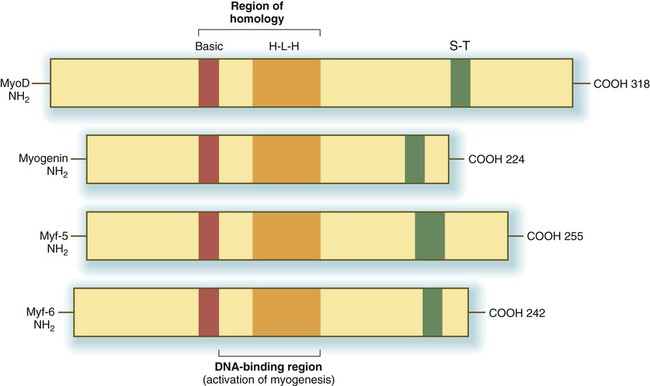
H-L-H, homologous helix-loop-helix regions; S-T, homologous serine/threonine-rich region.
As with many helix-loop-helix proteins, myogenic regulatory proteins of the MyoD family form dimers and bind to a specific DNA sequence (CANNTG), called the E box, in the enhancer region of muscle-specific genes. The myogenic specificity of these proteins is encoded in the basic region (see Fig. 9.33).
The regulatory activities of MyoD and other members of that family are themselves regulated by other regulatory proteins, which can modify their activities (Fig. 9.34). Many cells contain a transcriptional activator designated E12. When a molecule of E12 forms a heterodimer with a molecule of MyoD, the complex binds more tightly to the muscle-enhancer region of DNA than does a pure MyoD dimer. This increases the efficiency of transcription of the muscle genes. A transcriptional inhibitor called Id (inhibitor of DNA binding) can form a heterodimer with a molecule of MyoD. Id contains a loop-helix-loop region, but no basic region, which is the DNA-binding part of the molecule. The Id molecule has a greater binding affinity for a MyoD molecule than another molecule of MyoD and can displace one of the units of a MyoD dimer, thus resulting in more Id-MyoD heterodimers. These bind poorly to DNA and often fail to activate muscle-specific genes.
During muscle development, the myogenic regulatory factors of the MyoD family are expressed in a regular sequence (Fig. 9.35). In mice, the events leading to muscle formation begin in the somite, where Pax-3 and Myf-5, working through apparently separate pathways, activate MyoD and cause certain cells of the dermomyotome to become committed to forming muscle. With increased levels of MyoD, the mononuclear cells withdraw from the mitotic cycle and begin to fuse into myotubes. At this stage, myogenin is expressed. Finally, in maturing myotubes, Myf-6 (formerly called MRF-4) is expressed.
In knockout mice, the absence of a single myogenic regulatory factor (e.g., myf-5, MyoD) alone does not prevent the formation of skeletal muscle (although there may be other minor observable defects), but when myf-5 and MyoD are knocked out simultaneously, muscle fails to form. Another very instructive double knockout of Pax-3 and myf-5 produces mice that are totally lacking in muscles of the trunk and limbs, but the head musculature remains intact. This research shows that in the earliest stages of determination, different regulatory pathways are followed by muscle-forming cells of the head and trunk (see Fig. 9.41).
Histogenesis of Muscle
Not only are there fast and slow myoblasts, but also there are early and late cellular isoforms of myoblasts, which have different requirements for serum factors and nerve interactions in their differentiation. When the earliest myoblasts fuse into myotubes, they give rise to primary myotubes, which form the initial basis for an embryonic muscle. The differentiation of primary myotubes occurs before motor nerve axons have entered the newly forming muscle. Subsequently, smaller secondary myotubes arising from late myoblasts form alongside the primary myotubes (Fig. 9.36). By the time secondary myotubes form, early motor axons are present in the muscles, and there is evidence that the presence of nerves is required for the formation of secondary myotubes. A primary muscle fiber and its associated secondary muscle fibers are initially contained within a common basal lamina and are electrically coupled. These muscle fibers actively synthesize a wide variety of contractile proteins.
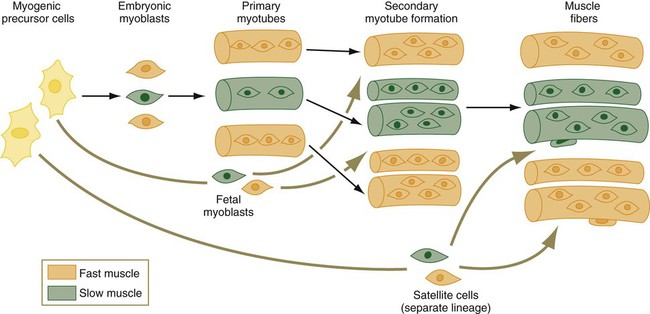
A family of embryonic myoblasts contributes to the formation of the primary myotubes, and fetal myoblasts contribute to secondary myotubes.
The myosin molecule is complex, consisting of two heavy chains and a series of four light chains (LCs) (Fig. 9.37). Mature fast fibers have one LC1, two LC2, and one LC3 subunits; slow muscle myosin contains two LC1 and two LC2 subunits. In addition, there are fast and slow forms (MHCf and MHCs) of the myosin heavy chain (MHC) subunits. The myosin molecules possess adenosine triphosphatase activity, and differences in this activity account partly for differences in the speed of contraction between fast and slow muscle fibers.
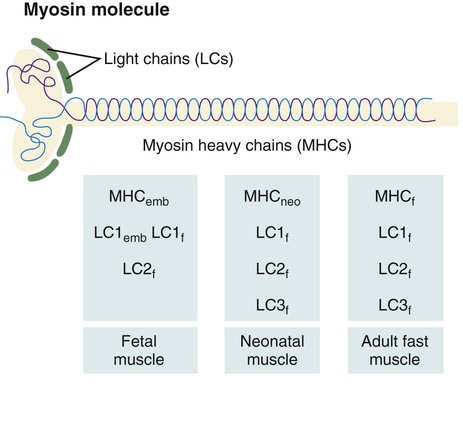
A schematic representation of the myosin molecule is also shown.
The myosin molecule undergoes a succession of isoform transitions during development. From the fetal period to maturity, a series of three developmental isoforms of the MHC (embryonic [MHCemb], neonatal [MHCneo], and adult fast [MHCf]) pass through a fast muscle fiber. (Developmental changes in the LC and MLC subunits are summarized in Figure 9.37.) Other contractile proteins of muscle fibers (e.g., actin, troponin) pass through similar isoform transitions. After injury to muscle in an adult, the regenerating muscle fibers undergo sets of cellular and molecular isoform transitions that closely recapitulate the transitions occurring in normal ontogenesis.
Morphogenesis of Muscle
Muscles of the Trunk and Limbs
Quail/chick grafting experiments have clearly shown that the major groups of skeletal muscles in the trunk and limbs arise from myogenic precursors located in the somites. In the thorax and abdomen, the intrinsic muscles of the back (the epaxial muscles) are derived from cells arising in the dorsal myotomal lip, whereas ventrolateral muscles (hypaxial muscles) arise from epithelially organized ventral buds of the somites. Tendons of the epaxial muscles arise from the syndetome layer within the somites (see Box 6.1), whereas tendons of the limb and hypaxial musculature arise from lateral plate mesoderm. In the limb regions, myogenic cells migrate from the epithelium of the ventrolateral dermomyotome early during development. More cranial myogenic cells originating in similar regions of the occipital somites migrate into the developing tongue and diaphragm. At the lumbar levels, precursors of the abdominal muscles also move out of the epithelium of ventrolateral somitic buds.
Early specification of the future hypaxial musculature within the epithelial somite is initially regulated by dorsalizing (possibly a member of the Wnt family) and lateralizing (BMP-4) signals from the ectoderm and lateral plate mesoderm. This process activates two early transcription factors (Six [sine oculis] and Eya [eyes absent]), which leads to a more intense expression of Pax3 and the expression of Lbx1, a homeobox gene that is exclusively expressed in the lateral dermomyotomal lips. Lbx1 may prevent the premature differentiation of the hypaxial musculature. It is highly likely that the prune belly syndrome (Fig. 9.38), which is characterized by the absence of the abdominal musculature, will be found to be caused by a molecular deficiency in this population of myogenic cells.
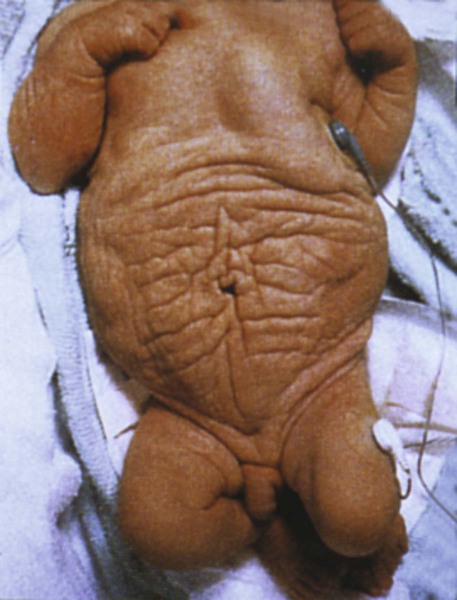
Note the wrinkled abdomen in the absence of the abdominal musculature. (From the Robert J. Gorlin Collection, Division of Oral and Maxillofacial Pathology, University of Minnesota Dental School, courtesy of Dr. Ioannis Koutlas.)
After their origin from the somites, the muscle primordia of the trunk and abdomen become organized into well-defined groups and layers (Fig. 9.39). (Morphogenesis of the limb muscles is discussed in Chapter 10.) The results of numerous experiments have shown fundamental differences in cellular properties between the cellular precursors of limb muscles and axial muscles. These differences are summarized in Table 9.3.
Table 9.3
Differences between Cellular Precursors of Axial and Limb Muscles
Axial Muscles | Limb Muscles |
Usual location in medial half of somite | Location in lateral half of somite |
Differentiation largely in situ | Migration into limb buds before differentiation |
Initial differentiation into mononucleate myocytes | Initial differentiation into multinucleate myotubes |
Myogenic determination factors (Myf-5, MyoD) expressed at or before the onset of myotome formation | Expression of myogenic determination genes delayed until limb muscle masses begin to coalesce |
Differentiation appears to be strongly influenced by neural tube and notochord | Migration and differentiation little influenced by axial structures |
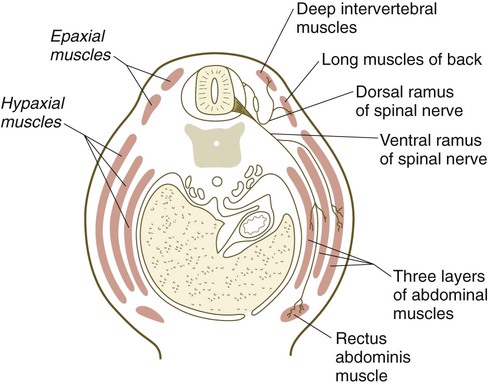
Muscles of the Head and Cervical Region
Myogenesis in the head differs significantly from that in the trunk (Fig. 9.40). Much of the cranial musculature, especially that associated with mastication, arises from cranial unsegmented paraxial mesoderm, equivalent to the somites. Other craniofacial muscles, especially those in the lower jaw and neck, arise from lateral splanchnic mesoderm, as does cardiac muscle. In the early postgastrulation period, the lateral splanchnic mesoderm (sometimes called the cardiocraniofacial morphogenetic field), associated with the future pharynx and probably responding to the same inductive signals from the pharyngeal endoderm, gives rise to both the lower cranial musculature and the secondary heart field. Early in the determination process, both these types of muscle are under the control of transcription factors (e.g., Isl-1, Tbx-1, and Nkx 2.5) that are different from those that control the early development of the trunk musculature. Different types of musculature develop under different sets of early controls before entering similar pathways of differentiation (Fig. 9.41).
Cardiac Muscle
Even early cardiac myoblasts contain relatively large numbers of myofibrils in their cytoplasm, and they are capable of undergoing pronounced contractions. In the embryo, the mononucleated cardiac myocytes face a difficult problem: The cells of the developing heart must continue to contract while the heart is increasing in mass. This functional requirement necessitates that cardiac myocytes undergo mitosis even though their cytoplasm contains many bundles of contractile filaments (Fig. 9.42). Cells of the body often lose their ability to divide when their cytoplasm contains structures characteristic of the differentiated state. Cardiac myocytes deal with this problem by partially disassembling their contractile filaments during mitosis. In contrast to skeletal muscle, cardiac myocytes do not undergo fusion, but rather remain as individual cells, although they may become binucleated. Cardiac myocytes keep in close structural and functional contact through intercalated disks, which join adjacent cells to one another.
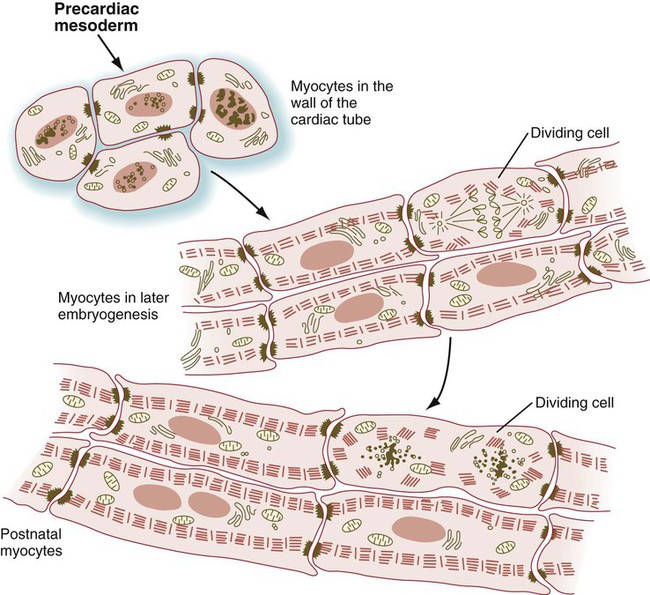
During mitosis, the contractile filaments undergo a partial disassembly. (Adapted from Rumyantsev P: Cardiomyocytes in processes of reproduction, differentiation and regeneration [in Russian], Leningrad, 1982, Nauka.)
Later in development, a network of cardiac muscle cells undergoes an alternative pathway of differentiation characterized by increased size, a reduction in the concentration of myofibrils, and a greatly increased concentration of glycogen in the cytoplasm. These cells form the conducting system, parts of which are called Purkinje fibers (see p. 434). Purkinje fibers also express a different profile of contractile protein isoforms from either atrial or ventricular myocytes.
Summary
The epidermis starts as a single layer of ectoderm, to which a single superficial layer of peridermal cells is added. As other layers are added, three cell types migrate from other sources: (1) melanoblasts (pigment cells) from the neural crest, (2) Langerhans’ cells (immune cells) from precursors in the bone marrow, and (3) Merkel cells (mechanoreceptors) from the neural crest.
In the multilayered epidermis, unspecialized cells from the stratum basale differentiate as they move through the various layers toward the surface of the epidermis. The cells produce increasing amounts of intracellular keratins and filaggrin; the latter is involved in the interconnections of the keratinocytes, the final differentiated form of the epidermal cell.
In the trunk, the dermis arises from mesodermal cells derived from the dermatome of the somites. Dermal-epidermal interactions underlie the formation of epidermal appendages such as hairs. In mammary glands, hormonal influences are important in the development of the duct system after the ductal epithelium is induced.
Skeletal tissue arises from the mesenchyme of either mesodermal or neural crest origin. There are two major subdivisions of the skeleton: the axial skeleton of the trunk and the appendicular skeleton of the limbs.
The fundamental organization of the cranial components of the vertebral column is closely associated with expression of the homeobox-containing genes. Superimposed on this is the induction of many components of the axial skeleton by underlying ectodermal (usually neural) structures. Individual vertebrae are composite structures consisting of components derived from two adjoining somites.
The skull consists of two subdivisions: the neurocranium, which surrounds the brain; and the viscerocranium, which surrounds the oral cavity. The base of the neurocranium (chondrocranium) is initially represented by several sets of paired cartilage. These later become transformed into bone. Most bones surrounding the brain are formed by intramembranous bone, which differentiates directly from mesenchyme. The viscerocranium is also derived from cartilaginous and membranous components.
Skeletal muscle fibers undergo a sequence of differentiation from mononuclear myoblasts. First, they fuse to form multinucleated myotubes, and then they mature into skeletal muscle fibers. Mononucleated reserve cells (satellite cells) can proliferate and fuse to growing or mechanically stressed muscle fibers.
Pax-3 and myf-5 (a member of the MyoD transcription factor family) stimulate myogenic progenitor cells of the trunk to form myoblasts. Other regulatory factors can activate (e.g., E12) or inhibit (e.g., Id) the activities of muscle regulatory factors. Early myogenic cells are kept in the cell cycle by growth factors such as FGF and transforming growth factor-β. Myoblasts are characterized by the expression of MyoD, and growth factors, such as insulinlike growth factor, promote their fusion and differentiation into myotubes, which express myogenin.
The first multinucleated muscle fibers to form are primary myotubes. Secondary myotubes form around them. Innervation by motor nerve fibers is necessary for the full differentiation of muscle fibers. During the differentiative process, several sets of isoforms of myosin subunits and other contractile proteins appear in sequence in the muscle fibers.
Skeletal muscles of the limbs and trunk arise from cellular precursors in the somites. The cranial musculature arises from the somitomeres. Dorsal and ventral muscles of the trunk arise from precursors located in different regions of the somites. The limb musculature also arises from cells in the ventrolateral regions of the somites. These cells migrate into the limb buds and express Pax-3 while migrating.
Cardiac muscle arises from splanchnic mesoderm. Cardiomyocytes differ from skeletal muscle cells in that they can divide mitotically after they are highly differentiated and contain contractile filaments.
*Many types of tissues contain a population of stem cells, which have a high capacity for proliferation. Some of the daughter cells remain as stem cells, but other daughter cells become what in the epidermis are called transit-amplifying cells. These cells, which are located in the stratum basale and to some extent in the stratum spinosum, are capable of a few more mitotic divisions before permanently withdrawing from the cell cycle. The postmitotic cells are sometimes called committed cells. In the epidermis, these are the cells that undergo keratinization.