Hematopoiesis
After completion of this chapter, the reader will be able to:
2. Discuss the evolution and formation of blood cells from embryo to fetus to adult, including anatomic sites and cells produced.
3. Predict the likelihood of encountering active marrow from biopsy sites when given the patient’s age.
4. Relate normal and abnormal hematopoiesis to the various organs involved in the hematopoietic process.
5. Explain the stem cell theory of hematopoiesis, including the names of various stem cells and progenitor cells and their lineage associations.
6. Discuss the roles of hematopoietic growth factors in differentiation and maturation sequences of hematopoietic progenitor cells, including nonspecific and lineage-specific factors.
7. Describe general morphologic changes that occur during cell maturation.
8. Define apoptosis and discuss the relationship between apoptosis, growth factors, and stem cell differentiation.
9. Discuss the roles of various cytokines and hematopoietic growth factors in the process of hematopoiesis.
10. Discuss therapeutic applications of cytokines and hematopoietic growth factors.
Hematopoietic Development
Mesoblastic Phase (Yolk Sac Phase)
Hematopoiesis is considered to begin around the nineteenth day of embryologic development after fertilization. Progenitor cells of mesenchymal origin migrate from the aorta-gonad-mesonephros region of the developing aorta-splanchnopleure to the yolk sac.1 The cells arising from the aorta-gonad-mesonephros region give rise to hematopoietic stem cells (HSCs), but not to primitive erythroblasts. The primitive erythroblasts found in the yolk sac arise from mesodermal cells, which initially line the cavity of the yolk sac. These primitive cells migrate from the periphery into the central cavity of the yolk sac, where they develop into primitive erythroblasts.2–5 The remaining cells surrounding the cavity of the yolk sac are called angioblasts and form the future blood vessels.2–5 The yolk sac phase of hematopoiesis is characterized by the development of primitive erythroblasts that produce measurable amounts of hemoglobins, including Portland, Gower-1, and Gower-2 (see Chapter 10). Yolk sac hematopoiesis does not contribute significantly to definitive hematopoiesis.4 This phase of hematopoiesis occurs intravascularly, or within a developing blood vessel.
Hepatic Phase
The hepatic phase of hematopoiesis begins at 4 to 5 gestational weeks and is characterized by recognizable clusters of developing erythroblasts, granulocytes, and monocytes.5 The developing erythroblasts signal the beginning of definitive hematopoiesis with a decline in primitive hematopoiesis of the yolk sac. In addition, lymphoid cells begin to appear.6,7 Hematopoiesis during this phase occurs extravascularly, with the liver remaining the major site of hematopoiesis during fetal life and retaining activity until 1 to 2 weeks after birth. Hematopoiesis in the aorta-gonad-mesonephros region and the yolk sac disappears during this stage. Hematopoiesis in the fetal liver reaches its peak by the third month of development (Figure 7-1). The developing spleen, kidney, thymus, and lymph nodes contribute to the hematopoietic process during this phase. The thymus, the first fully developed organ in the fetus, becomes the major site of T-cell production, whereas the kidney and spleen produce B cells.
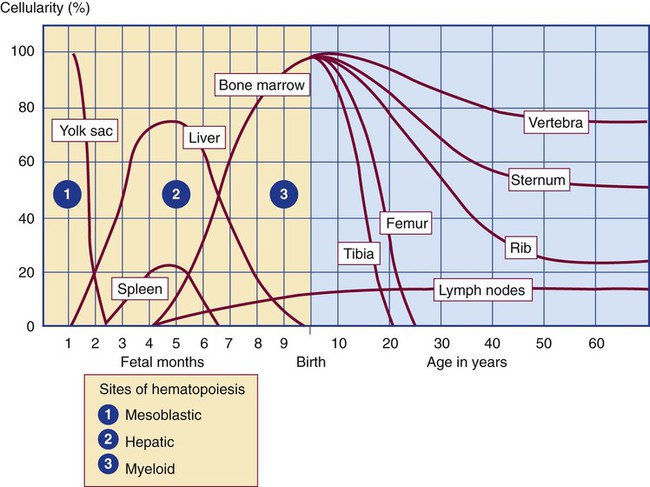
Production of megakaryocytes also begins during the hepatic phase. The spleen gradually decreases granulocytic production and involves itself solely in lymphopoiesis. During the hepatic phase, detectable levels of hemoglobin (Hb) F, Hb A, and Hb A2 may be present.8
Medullary (Myeloid) Phase
During the fifth month of fetal development, hematopoiesis begins in the developing bone marrow cavity. This transition is called medullary hematopoiesis because it occurs in the medulla or inner part of the bone marrow. During this phase, mesenchymal cells, which are a type of embryonic tissue, migrate into the core of the bone and differentiate into skeletal and hematopoietic blood cells.9,10 Hematopoietic activity, especially myeloid activity, is apparent during this stage of development, and the myeloid-to-erythroid ratio approaches the adult level of 3 : 1 by 21 weeks of gestation.11 By the end of the sixth month, the bone marrow becomes the primary site of hematopoiesis. Measurable levels of erythropoietin (EPO), granulocyte colony-stimulating factor (G-CSF), granulocyte-monocyte colony-stimulating factor (GM-CSF), fetal hemoglobin, Hb A2, and adult hemoglobin can be detected. In addition, cells at various stages of maturation can be seen in all three lineages.
Adult Hematopoietic Tissue
Bone Marrow
A central space is created within the cavities of these bones by resorption of cartilage and endosteal bone. Mesenchymal cells migrate into the space and eventually differentiate into three cell types, which give rise to the blood and bone marrow matrix cells (reticular cells and adipose tissue). Reticular cells are formed on the exterior surfaces of the venous sinuses and extend long, narrow branches into the perivascular space, creating a meshlike network; this provides a supportive skeletal network for developing hematopoietic cells, macrophages, and mast cells.10 During infancy and early childhood, the bone marrow consists primarily of red active marrow. Between 5 and 7 years of age, adipocytes become more abundant and begin to occupy the spaces in the long bones previously dominated by active marrow. The process of replacing the active marrow by adipose tissue (yellow marrow) during development is called retrogression and eventually results in restriction of the active marrow to the flat bones, sternum, vertebrae, pelvis, ribs, skull, and proximal portion of the long bones (Figure 7-2). Areas located within the bone marrow cavity where red marrow has been replaced by yellow marrow consist of an mixture of adipocytes, undifferentiated mesenchymal cells, and macrophages. Inactive yellow marrow is also scattered throughout active red marrow and is capable of reverting back to active marrow in cases of increased demand on the bone marrow.8 Such cases might be excessive blood loss or increased erythrocyte destruction in the bone marrow by toxic chemicals or irradiation.
Red Marrow
The red marrow is composed of extravascular cords that contain all of the developing blood cell lineages, stem and progenitor cells, adventitial cells, and macrophages (Figures 7-3 and 7-4). The cords are separated from the lumen of the sinusoids by endothelial and adventitial cells and are located between the trabeculae of spongy bone. Trabeculae are projections of calcified bone radiating out from the cortical bone into the marrow space and provide support for the developing marrow. The hematopoietic cells tend to develop in specific niches within the cords. Normoblasts develop in small clusters adjacent to the outer surfaces of the vascular sinuses (Figure 7-5); in addition, some normoblasts are found surrounding iron-laden macrophages (Figure 7-6). Megakaryocytes are located close to the vascular walls of the sinuses, which facilitates the release of platelets into the lumen of the sinusoids. Immature myeloid (granulocytic) cells through the metamyelocyte stage are located deep within the cords. As these maturing granulocytes proceed along their differentiation pathway, they move closer to the vascular sinuses.9
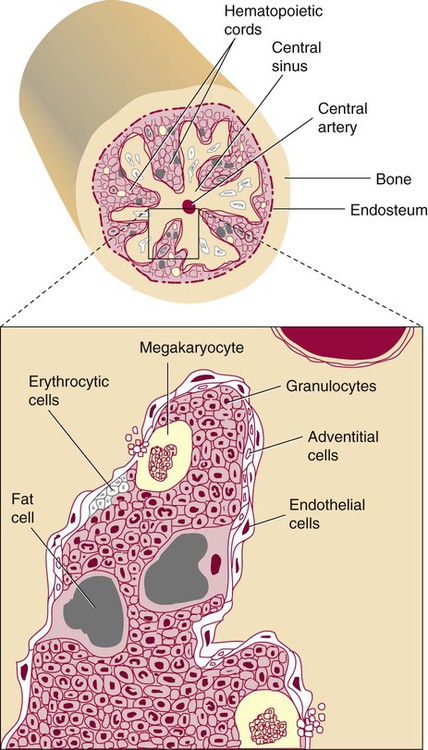
As blood cells come in contact with endothelial cells, they bind to the surface via a receptor-mediated process. Cells pass through pores in the endothelial cytoplasm and are released into the circulation.12
Marrow Circulation
The nutrient and gas requirements of the marrow are supplied by the nutrient and periosteal arteries, which enter via the bone foramina. The nutrient artery supplies blood only to the marrow.10 It coils around the central longitudinal vein, which passes along the bone canal. In the marrow cavity, the nutrient artery divides into ascending and descending branches that also coil around the central longitudinal vein. The arteriole branches that enter the inner lining of the cortical bone (endosteum) form sinusoids (endosteal beds), which connect to periosteal capillaries that extend from the periosteal artery.13 The periosteal arteries provide nutrients for the osseous bone and the marrow. Their capillaries connect to the venous sinuses located in the endosteal bed, which empty into a larger collecting sinus that opens into the central longitudinal vein.14 Blood exits the marrow via the central longitudinal vein, which runs the length of the marrow. The central longitudinal vein exits the marrow through the same foramen where the nutrient artery enters. Hematopoietic cells located in the endosteal bed receive their nutrients from the nutrient artery.
Hematopoietic Microenvironment
The hematopoietic inductive microenvironment plays an important role in stem cell differentiation and proliferation.15,16 It is responsible for supplying a semifluid matrix, which serves as an anchor for the developing hematopoietic cells. The matrix is responsible for maintaining differentiation and proliferation and provides a supporting tissue in the hematopoietic inductive microenvironment. Stromal cells in the matrix are of several types: (1) endothelial cells, (2) adipocytes, (3) macrophages, (4) osteoblasts, (5) osteoclasts, and (6) reticular cells (fibroblasts). Endothelial cells are broad flat cells that form a single continuous layer along the inner surface of the bone marrow sinus.15 They regulate the flow of particles entering and leaving hematopoietic spaces. Adipocytes are large cells with a single fat vacuole; they secrete various steroids that influence erythropoiesis and maintain bone integrity.14 They also play a role in regulating the volume of the marrow in which active hematopoiesis occurs.14 Macrophages function in phagocytosis and secretion of various cytokines that regulate hematopoiesis and are located throughout the marrow space.17 Other cells involved in cytokine production include endothelial cells, adipocytes, and fibroblasts. Osteoblasts are bone-forming cells, and osteoclasts are bone-resorbing cells. Reticular cells are associated with the formation of reticular fibers that form a lattice that supports the vascular sinuses and developing hematopoietic cells. Stromal cells are believed to be derived from fibroblasts. They play a role in support and regulation of hematopoietic stem/progenitor cell survival and differentiation.15
The extracellular matrix of the bone marrow contains proteoglycans or glycosaminoglycans, fibronectin, collagen, laminin, hemonectin, and thrombospondin.15 Proteoglycans are expressed on the endothelial cell surface and mediate progenitor binding to the stroma. Fibronectin, collagen, laminin, hemonectin, and thrombospondin function as adhesion molecules, promoting the adhesion of HSCs to the extracellular matrix.
Liver
The liver consists of two lobes situated beneath the diaphragm in the abdominal cavity. The position of the liver with regard to the circulatory system is optimal for gathering, transferring, and eliminating substances via the bile.18 Anatomically, liver cells are arranged in radiating hepatic lobules emanating from a central vein (Figure 7-7). Adjacent to the longitudinal lobes of the liver and separated only by a small space are sinusoids, which are lined by two types of cells: Kupffer cells and epithelial cells. Kupffer cells are macrophages, removing cellular and foreign debris from the blood that circulates through the liver; they also are responsible for protein synthesis.19 The epithelial cells are arranged in the lining so as to be separated from one another by a noncellular area; this arrangement allows plasma to have direct access to the hepatocytes. This unusual organization of the liver and its location in the body enables it to be involved in many varied functions.
Liver Pathophysiology
The liver is often involved in blood-related diseases. In porphyrias, the liver exhibits enzymatic deficiencies that result in the accumulation of the various intermediary porphyrins. In severe hemolytic anemias and red blood cell (RBC) dysplasias, the conjugation of bilirubin and the storage of iron are increased. The liver sequesters membrane-damaged RBCs and removes them from the circulation. The liver is capable of extramedullary hematopoietic production in case of bone marrow shutdown.14 It is directly affected by storage diseases of the monocyte/macrophage (Kupffer) cells as a result of enzymatic deficiencies that cause hepatomegaly with ultimate dysfunction of the liver (Gaucher disease, Niemann-Pick disease, Tay-Sachs disease; see Chapter 28).
Spleen
The spleen is the largest lymphoid organ in the body. The spleen is located directly beneath the diaphragm behind the fundus of the stomach in the upper left quadrant of the abdomen. It is vital but not essential for life and functions as an indiscriminate filter of the circulating blood. In a healthy individual, the spleen contains about 350 mL of blood.18
The exterior surface of the spleen is surrounded by a layer of peritoneum and inwardly by a connective tissue capsule. The capsule projects inwardly, forming trabeculae that divide the spleen into discrete regions. Located within these regions are three types of splenic tissue: (1) white pulp, (2) red pulp, and (3) a marginal zone. The white pulp consists of scattered follicles with germinal centers containing lymphocytes, macrophages, and dendritic cells. Aggregates of lymphocytes surround splenic arteries that pass through these germinal centers. Adjacent to the splenic arteries is a region called the periarteriolar lymphatic sheath. This area consists of lymphoid nodules containing primarily B lymphocytes. Activated B lymphocytes are found in the germinal centers.19
The marginal zone surrounds the white pulp and forms a reticular meshwork containing blood vessels, macrophages, and specialized B cells. The red pulp is composed primarily of vascular sinusoids and sinuses separated by cords of tissue (cords of Billroth) containing specialized macrophages that are loosely connected to the dendritic process, creating a spongelike region that functions as a filter for blood passing through the region.19 As RBCs pass through the cords of Billroth, there is a decrease in the flow of blood, which leads to stagnation and depletion of the RBCs’ glucose supply. These cells are subject to increased damage and stress that may lead to their removal from the spleen. The spleen uses two methods for removing senescent RBCs from the circulation: (1) culling, in which the cells are phagocytosed with subsequent degradation of cell organelles, and (2) pitting, in which splenic macrophages remove inclusions or damaged surface membrane from the circulating RBCs. The spleen synthesizes immunoglobulin M in the germinal centers, and it serves as a storage site for platelets. In a healthy individual, approximately 30% of the total platelet count is sequestered in the spleen.20
The spleen has a rich blood supply, receiving approximately 350 mL/min. Blood enters the spleen through the central splenic artery located at the hilum and branches outward through the trabeculae. The branches enter all three regions of the spleen: the white pulp with its dense accumulation of lymphocytes, the marginal zone, and the red pulp. The venous sinuses, which are located in the red pulp, unite and leave the spleen as splenic veins (Figures 7-8 and 7-9).21
Spleen Pathophysiology
In the rapid-transit pathway, blood cells enter the splenic artery and pass directly to the sinuses in the red pulp and continue to the venous system to exit the spleen. When splenomegaly occurs, the spleen becomes enlarged and is palpable. This occurs as a result of many conditions, such as chronic leukemias, genetic defects in RBCs, hemoglobinopathies, Hodgkin disease, thalassemia, malaria, and the myeloproliferative disorders. Often splenectomy is beneficial in cases of excessive destruction of RBCs, such as severe hereditary spherocytosis, storage disorders, and autoimmune hemolytic anemias, when treatment with corticosteroids does not effectively suppress hemolysis.22,23 Splenectomy also may be indicated in severe cases of agnogenic myeloid metaplasia associated with splenomegaly, severe refractory hemolytic anemia, thrombocytopenia, or qualitative platelet function defect syndromes.22,23 After splenectomy, platelet and leukocyte counts increase transiently.22 In sickle cell anemia, repeated splenic infarcts caused by sickled RBCs trapped in the small-vessel circulation of the spleen cause tissue damage and necrosis, which often results in autosplenectomy (see Chapter 26).
Hypersplenism is an enlargement of the spleen resulting in some degree of pancytopenia despite the presence of a hyperactive bone marrow. The most common cause is congestive splenomegaly secondary to cirrhosis of the liver and portal hypertension. Other causes include thrombosis, vascular stenosis, other vascular deformities such as aneurysm of the splenic artery, and cysts.24
Lymph Nodes
Lymph nodes are organs of the lymphatic system located along the lymphatic capillaries that parallel, but are not part of, the circulatory system. The nodes are bean-shaped structures (1 to 5 mm in diameter) that occur in groups or chains at various intervals along lymphatic vessels. They may be superficial (inguinal, axillary, cervical, supratrochlear) or deep (mesenteric, retroperitoneal). Lymph is the fluid portion of blood that escapes into the connective tissue and is characterized by a low protein concentration and the absence of RBCs. Afferent lymphatic vessels carry circulating lymph to the lymph nodes. Lymph is filtered by the lymph nodes and exits via the efferent lymphatic vessels located in the hilus of the lymph node.25
Similar in structure to the spleen, lymph nodes consist of an outer capsule that forms trabeculae and provides support for macrophages and the predominant population of lymphocytes. Lymph nodes can be divided into two basic regions: an outer region called the cortex and an inner region called the medulla. Trabeculae radiate through the cortex and the medulla, dividing the interior of the lymph node into specific areas (Figure 7-10). In the cortical region, these areas are known as cortical nodules and contain follicles. These follicles contain foci of B-cell proliferation termed germinal centers.9,18 The cortical nodules are arranged in circles along the outer cortex region of the lymph node. Located between the cortex and the medulla is a region called the paracortex, which contains predominantly T cells and numerous macrophages. The medullary cords lie toward the interior of the lymph node. These cords consist primarily of B lymphocytes and plasma cells.23 Lymph nodes have three main functions: (1) they play a role in the formation of new lymphocytes from the germinal centers, (2) they are involved in the processing of specific immunoglobulins, and (3) they filter particulate matter, debris, and bacteria entering the lymph node via the lymph.
Thymus
At birth, the thymus is an efficient, well-developed organ. It consists of two lobules, each measuring 0.5 to 2 cm in diameter. It is located in the upper part of the anterior mediastinum at about the level of the great vessels of the heart.9,18 It resembles other lymphoid tissue in that the lobules are subdivided into two areas: the cortex (a peripheral zone) and the medulla (a central zone) (Figure 7-11). Both areas are populated with the same cellular components—lymphocytes, mesenchymal cells, reticular cells, and many macrophages—although in different proportions. The cortex is characterized by a blood supply system that is unique in that it consists only of capillaries. Its function seems to be that of a “waiting zone,” which is densely populated with progenitor lymphoid cells that migrated from the bone marrow. These cells have no identifiable surface markers when they enter the thymus but give rise to T cells that later express surface antigens and move toward the medulla. Eventually, they leave the thymus to populate specific regions of other lymphoid tissue, such as the T cell–dependent areas of the spleen, lymph nodes, and other lymphoid tissues that are deficient in immunocompetent T lymphocytes. It is theorized that the cytoplasmic processes of the epithelial reticular cells contain secretory products, thymic hormones, thymic factor, and thymic humoral hormones (proteins/peptides extracted from the thymus) that promote differentiation of pre-T (nonmarked) from mature T lymphocytes. The cells that are not marked die in the cortex as a result of apoptosis and are phagocytosed by macrophages before release. The medulla contains only 5% mature T lymphocytes and seems to be a holding zone for conditioned cells until the cells are needed by the peripheral lymphoid tissues.26 The thymus also contains myriad cell types, including B cells, dendritic cells, eosinophils, neutrophils, and myeloid cells.19
Gross examination indicates that the size of the thymus is related to age. The thymus weighs 12 to 15 g at birth, increases to 30 to 40 g at puberty, and decreases to 10 to 15 g at later ages. It is hardly recognizable in old age, having atrophied (Figure 7-12). The thymus retains the ability to produce new T cells, however, as has been shown after irradiation treatment that may accompany bone marrow transplantation.
Stem Cells and Cytokines
Stem Cell Theory
In 1961 Till and McCulloch27 conducted a series of experiments in which they irradiated spleens and bone marrows of mice, creating a state of aplasia. These aplastic mice were given an intravenous injection of marrow cells. Colonies of HSCs were seen 7 to 8 days later in the spleens of the irradiated (recipient) mice. These colonies were called colony-forming units–spleen (CFU-S). These investigators later showed that these colonies were capable of self-renewal and the production of differentiated progeny. The CFU-S represents what we now refer to as committed myeloid progenitors or colony-forming unit–granulocyte, erythrocyte, monocyte, and megakaryocyte (CFU-GEMM).27,28 These cells are capable of giving rise to multiple lineages of blood cells.
Stem cells by definition can be characterized as follows: (1) they are capable of self-renewal, (2) they give rise to differentiated progeny, and (3) they are able to reconstitute the hematopoietic system of a lethally irradiated host. The undifferentiated HSCs can differentiate into progenitor cells committed to either lymphoid or myeloid lineages. These lineage-specific progenitor cells consist of (1) the common lymphoid progenitor, which proliferates and differentiates into lymphocytes of T, B, and natural killer lineages; and (2) the common myeloid progenitor, which proliferates and differentiates into individual granulocytic, erythrocytic, monocytic, and megakaryocytic lineages. The resulting limited lineage-specific precursors give rise to morphologically recognizable, lineage-specific precursor cells (Figure 7-13 and Table 7-1). Despite the limited numbers of HSCs in the bone marrow, more than 1 to 5 × 109 each of erythrocytes and leukocytes are produced each hour for the entire life span of an individual.15 Most of the cells in normal bone marrow are precursor cells at various stages of maturation.
TABLE 7-1
Culture-Derived Colony-Forming Units (CFUs)
Abbreviation | Cell Line |
CFU-GEMM | Granulocyte, erythrocyte, megakaryocyte, monocyte |
CFU-E | Erythrocyte |
CFU-Meg | Megakaryocyte |
CFU-M | Monocyte |
CFU-GM | Granulocyte, monocyte |
CFU-BASO | Myeloid to basophil |
CFU-EO | Myeloid to eosinophil |
CFU-G | Myeloid to neutrophil |
CFU-pre-T | T lymphocyte |
CFU-pre-B | B lymphocyte |
HSCs are directed to one of three possible fates: (1) self-renewal, (2) differentiation, or (3) apoptosis.29 When the HSC divides, it gives rise to two identical daughter cells. Both daughter cells may follow the path of differentiation, leaving the stem cell pool (symmetric division), or one daughter cell may return to the stem cell pool and the other daughter cell may follow the path of differentiation (asymmetric division) or undergo apoptosis. Many theories have been proposed to describe the mechanisms that determine the fate of the stem cell. Till and McCulloch proposed that hematopoiesis is a random process whereby the HSCs randomly commits to self-renewal or differentiation.27 This model is also called the stochastic model of hematopoiesis. Later studies suggested that the microenvironment in the bone marrow determines whether the stem cell will self-renew or differentiate (instructive model of hematopoiesis).29 Current thinking is that the ultimate decision made by the stem cell can be described by both the stochastic and instructive models of hematopoiesis. The initial decision to self-renew or differentiate is probably stochastic, whereas lineage differentiation that occurs later is determined by various signals from the hematopoietic inductive microenvironment in response to specific requirements of the body.
The multilineage priming model suggests that HSCs receive signals from the hematopoietic inductive microenvironment to amplify or repress genes associated with commitment to multiple lineages that are expressed only at low levels. The implication is that the cell’s fate is determined by intrinsic and extrinsic factors. Extrinsic regulation involves proliferation and differentiation signals from specialized niches located in the hematopoietic inductive microenvironment via direct cell-to-cell or cellular-extracellular signaling molecules.29 Some of the cytokines released from the hematopoietic inductive microenvironment include factors that regulate proliferation and differentiation, such as stem cell factor (SCF), thrombopoietin (TPO), and Flt3 ligand. Intrinsic regulation involves genes such as SCL (TAL1), which is expressed in cells in the hemangioblast, a bipotential progenitor cell of mesodermal origin that gives rise to hematopoietic and endothelial lineages, and GATA2, which is expressed in later-appearing HSCs. Both of these genes are essential for primitive and definitive hematopoiesis.29 In addition to factors involved in differentiation and regulation, there are regulatory signaling factors, such as Notch-1 and Notch-2, which allow HSCs to respond to hematopoietic inductive microenvironment factors, altering cell fate.30
Stem Cell Cycle Kinetics
The bone marrow is estimated to be capable of producing approximately 3 billion erythrocytes, 2.5 billion platelets, and 1.5 billion granulocytes per kilogram of body weight daily. The determining factor controlling the rate of production is physiologic need. Stem cells exist in the marrow in the ratio of 1 per 1000 nucleated blood cells. Stem cells are capable of many mitotic divisions when stimulated by appropriate cytokines. When mitosis has occurred, the cell may reenter the cycle or go into a resting phase, termed G0. Some cells in the resting phase reenter the active cell cycle and divide one additional time, whereas other cells are directed to terminal differentiation (Figure 7-14).
From these data, a mitotic index can be calculated to establish the percentage of cells in mitosis in relation to the total number of cells. Factors affecting the mitotic index include the duration of mitosis and the length of the resting state. Normally, the mitotic index is approximately 1% to 2%. An increased mitotic index implies increased proliferation. An exception to this rule is in the case of megaloblastic anemia, in which mitosis is prolonged.31 An understanding of the mechanism of the generative cycle aids in understanding the mode of action of specific drugs used in the treatment and maintenance management of proliferative disorders.
Stem Cell Phenotypic and Functional Characterization
The identification and origin of stem cells can be determined by immunophenotypic analysis using flow cytometry. The earliest identifiable human HSCs capable of initiating long-term cultures are CD34+, CD38−, HLA-DRlow, Thy1low, and Lin−.30 This population of marrow cells is enriched in primitive progenitors. The expression of CD38 and HLA-DR is associated with a loss of “stemness.” The acquisition of CD33 and CD38 is seen on committed myeloid progenitors,30 and the expression of CD10 and CD38 is seen on committed lymphoid progenitors.30 The expression of CD7 is seen on T-lymphoid progenitor cells and natural killer cells, and the expression of CD19 is seen on B-lymphoid progenitors (see Chapter 33).30
Functional characterization of HSCs can be accomplished through in vitro techniques using long-term culture assays. These involve the enumeration of colony-forming units (e.g., CFU-GEMM, colony-forming unit–granulocyte-monocyte, burst-forming unit–erythroid) on semisolid media, such as methylcellulose. Primitive progenitor cells, such as the high proliferative potential colony-forming cell and the long-term colony initiating cell, also have been identified. These hematopoietic precursor cells give rise to colonies that can survive for 5 to 8 weeks and be replated.30 In vivo functional assays also are available and require transplantation of cells into syngeneic, lethally irradiated animals followed by transference of the engrafted bone marrow cells into a secondary recipient.30 These systems allow the ability of the stem cells to proliferate and differentiate to be characterized and may serve as models for developing clinically applicable techniques for gene therapy and stem cell transplantation.
Cytokines and Growth Factors
A group of specific glycoproteins called hematopoietic growth factors or cytokines regulates the proliferation, differentiation, and maturation of hematopoietic precursor cells. Cytokines are a diverse group of soluble proteins that have direct and indirect effects on hematopoietic cells. Classification of cytokines has been difficult because of their overlapping and redundant properties. The terms cytokine and growth factor are often used synonymously; cytokines include interleukins (ILs), lymphokines, monokines, interferons, chemokines, and colony-stimulating factors (CSFs).32 Cytokines are responsible for stimulation or inhibition of production, differentiation, and trafficking of mature blood cells and their precursors.33 Many of these cytokines exert a positive influence on stem cells and progenitor cells with multilineage potential (e.g., IL-1, IL-3, IL-6, IL-9, IL-11, GM-CSF, and kit ligand).33 Cytokines that exert a negative influence on hematopoiesis include transforming growth factor-β, tumor necrosis factor-α, and the interferons.30
Apoptosis refers to programmed cell death, a normal physiologic process that eliminates unwanted, abnormal, or harmful cells. Apoptosis differs from necrosis, which is accidental death from trauma. When cells do not receive the appropriate cytokines necessary to prevent cell death, apoptosis is initiated. In some disease states, apoptosis is “turned on,” which results in early cell death, whereas in others the mechanisms involved in apoptosis fail to initiate this process, which allows uncontrolled proliferation of cells.33,34
Colony-Stimulating Factors
CSFs are produced by many different cells. They have a high specificity for their target cells and are active at low concentrations.32 The names of the individual factors indicate the predominant cell lines that respond to their presence: The primary target of G-CSF is the granulocyte, and GM-CSF targets the granulocyte-monocyte cell line. The biologic activity of CSFs was first identified by their ability to induce hematopoietic colony formation in semisolid media. In addition, it was shown in cell culture experiments that although a particular CSF may show specificity for one cell lineage, it is often capable of influencing other cell lineages as well. This is particularly true when multiple growth factors are combined.35 Although G-CSF stimulates the proliferation of granulocyte progenitors, it also works synergistically with IL-3 to enhance megakaryocyte colony formation.35
Stem Cell Factor, Flt3 Ligand, Granulocyte-Monocyte Colony-Stimulating Factor, Interleukin-3, and Interleukin-6
Ogawa36 described early-acting growth factors (multilineage), intermediate-acting growth factors (multilineage), and late-acting growth factors (lineage restricted). Kit ligand, also known as SCF, is an early-acting growth factor; its cell surface receptor is the product of the c-kit gene. The receptor c-kit is a type I tyrosine kinase receptor that is expressed on HSCs and is downregulated with differentiation. The binding of SCF to its receptor (c-kit) induces a series of signals that are sent via signal transduction pathways from the cytoplasmic receptor of the HSC to the nucleus of the HSC, stimulating the cell to proliferate. As HSCs differentiate and mature, the expression of c-kit decreases. Activation of kit ligand by SCF is essential in the early stages of hematopoiesis.33,37
Flt3 seems to act at an even earlier stage of HSC development than SCF. Flt3 stands for c-fms-like tyrosine kinase.38 SCF and Flt3 work synergistically with IL-3, G-CSF, and GM-CSF to promote early HSC proliferation.
Other early-acting CSFs that are multilineage include IL-3, GM-CSF, and IL-6. IL-3 regulates blood cell production by controlling the production, differentiation, and function of granulocytes and macrophages.39 GM-CSF induces expression of specific genes and stimulates HSC proliferation, differentiation, and functional activation.40 IL-6 is a pleiotropic growth factor with stimulatory effects on myeloid and lymphoid cell lineages.41
Interleukins
Cytokines originally were named according to their specific function, such as lymphocyte-activating factor (now called IL-1), but continued research showed that a particular cytokine may have multiple actions. A group of scientists began calling some of the cytokines interleukins, numbering them in the order in which they were identified (e.g., IL-1, IL-2). Figure 7-15 illustrates the hematopoietic system and the sites of action of some of the cytokines. These factors are discussed in more detail in subsequent chapters. Characteristics shared by interleukins include the following:
1. They are proteins that exhibit multiple biologic activities, such as the regulation of autoimmune and inflammatory reactions and hematopoiesis.
2. They have synergistic interactions with other cytokines and growth factors.
3. They are part of interacting systems with amplification potential.
Table 7-2 lists the sources of cytokines and sites of activity.
TABLE 7-2
Selected Cytokines, Sources, Target, and Clinical Application
Cytokine | Source | Target | Clinical Application |
IL-1 | Monocytes/macrophages, endothelial cells, fibroblasts, epithelial cells, T cells | Fibroblasts, endothelial cells, basophils | Used in cases of inflammation, such as fever, and as a defense mechanism during infection43,46 |
IL-2 | T cells | T, B, NK cells | Enhances migration of cells with antitumor activity into tissues; induces release of IL-6, tumor necrosis factor, and interferon, which may have antitumor effects47 |
IL-3 | T cells, monocytes/macrophages, mast cells | Macrophages, neutrophils, eosinophils, basophils, mast cells, megakaryocytes, T and B cells | Used in treatment of refractory anemia with and without ringed sideroblasts; also used to stimulate megakaryopoiesis48 |
IL-4 | T cells, B cells, macrophages, basophils, mast cells | Macrophages, myeloid progenitors, T and B cells, mast cells, fibroblasts | Used to potentiate antitumor activity, especially in cases of gastric carcinoma49 |
IL-5 | T cells | T and B cells, eosinophils | |
IL-6 | Monocytes/macrophages, endothelial cells, T cells, fibroblasts, osteoblasts | Fibroblasts, myeloid precursors, T and B cells, mast cells, fibroblasts | Pleiotropic cytokine with wide range of biologic activities in immune regulation and hematopoiesis |
IL-7 | BM stromal cells, spleen, thymus | Pre-B cells, early T cells, CLL, Sézary cells, ALL | Enhances allocytolytic activity of lymphokine-activated killer cells50; is considered to be a promoter of thymopoiesis and immune recovery51 |
IL-8 | Monocytes/macrophages, fibroblasts, neutrophils, endothelial cells, synovial cells, chondrocytes | Chemoattractant for neutrophils, T cells, eosinophils | Active in neutrophil chemotaxis and degranulation; acts as a principal mediator of inflammation that then can be acted on by IL-452 |
IL-9 | T cells | T cells, macrophages, mast cells | Potent lymphoid cell factor, stimulating growth of certain T cells and mast cells53,54 |
IL-10 | T and B cells, macrophages | Lymphoid and myeloid cells | Synergistic activity of IL-4 and IL-10 causes antiinflammatory effect on human placental cells in vitro55 |
IL-11 | BM stromal cells, fibroblasts | Progenitor cells and stromal cells | Has shown activity in preclinical studies in association with myelosuppression, cancer therapy, neutropenia and thrombocytopenia56; stimulates growth and survivability of certain B and T cells57 |
IL-12 | Macrophages | T, NK cells | Used in stimulating proliferation of peripheral blood mononuclear cells and tumor-infiltrating lymphocytes in melanoma patients57; capable of reversing immunosuppression mediated by the neoplastic agent paclitaxel58 |
IL-13 | T cells, basophils, stromal cells | Monocytes, B lymphocytes | Pleiotropic cytokine that has important antiinflammatory and immunoregulatory activities50 |
IL-14 | T cells, T and B lymphoma cells | Pre-B, T, NK cells | CD4+ cell growth factor; proinflammatory; enhances lymphocyte chemotaxis59 |
IL-15 | Monocytes/macrophages, epithelial cells, skeletal muscle cells, BM and thymic stromal cells | T, B, and NK cells, mast cells | rhIL-15 has clinical applications in immunodeficiency diseases and BM transplantation, where it might be advantageous to accelerate peripheral expansion and promote localization of T cells60 |
IL-16 | CD8+ T cells, mast cells | T and B cells | Chemoattracts CD4 T cells61 |
IL-17 | CD4+ cells | Macrophages | May be useful in further delineating mechanisms of G-CSF regulation50 |
IL-18 | Monocytes/macrophages, liver | Tumor cells, pathogens | |
G-CSF | Endothelial cells, placenta, monocytes/macrophages, fibroblasts | All granulocytes, macrophages, endothelial cells, fibroblasts, leukemic myeloblasts | Stimulates granulocyte production and granulocyte activation62; may be used to treat neutropenia and to mobilize CD34+ stem cells in the peripheral blood63 |
M-CSF | B and T cells, endothelial cells, monocytes/macrophages, fibroblasts, epithelial cells, osteoblasts | Monocytes/macrophages, osteoblasts | Used to stimulate monocytes/macrophages production and activity62 |
GM-CSF | B, T, and NK cells, osteoblasts, endothelial cells, fibroblasts, monocytes/macrophages | All granulocytes, megakaryocytes, stem cells, BFU-E, monocytes/macrophages, leukemic myeloblasts | May be used to treat neutropenia in patients receiving chemotherapy and in those undergoing BM transplantation64 |
EPO | Kidney, liver | BFU-E, CFU-E | Used to stimulate growth and proliferation of erythroid precursors; used clinically to treat anemia associated with renal failure, chemotherapy, and BM infiltration by cancer64 |
TPO | Kidney, liver, spleen, BM stroma, muscle, brain | Megakaryocytes, platelets, c-mpl+ blasts | Essential for maturation of megakaryocytes and enhances platelet production; used to speed recovery of blood platelets after cytoreductive therapies65 |
c-kit ligand | Fibroblasts, BM stroma, hepatocytes | Primitive progenitors | Also referred to as stem cell factor/steel factor; used to stimulate myeloid, erythroid, and lymphoid progenitors66 |
Flt3 ligand | BM fibroblasts and stroma cells | Acts synergistically with other growth factors to stimulate T cells, B cells, NK cells, and dendritic cells67 | Stimulates primitive progenitor cells68 |
Lineage-Specific Hematopoiesis
Erythropoiesis
Erythropoiesis occurs in the bone marrow and is a complex, regulated process for maintaining adequate numbers of erythrocytes in the peripheral blood. The CFU-GEMM gives rise to the earliest identifiable colony of RBCs, called the burst-forming unit–erythroid (BFU-E). The BFU-E produces a large multiclustered colony that resembles a cluster of grapes containing brightly colored hemoglobin. These colonies range from a single large cluster to 16 or more clusters. BFU-Es contain only a few receptors for EPO, and their cell cycle activity is not influenced significantly by the presence of exogenous EPO. BFU-Es under the influence of IL-3, GM-CSF, TPO, and kit ligand develop into colony-forming unit–erythroid (CFU-E) colonies.28 The CFU-E has many EPO receptors and has an absolute requirement for EPO. Some CFU-Es are responsive to low levels of EPO and do not have the proliferative capacity of the BFU-E.42
EPO is a lineage-specific glycoprotein that prevents apoptosis of erythroid precursors. EPO is produced in the renal peritubular interstitial cells or renal tubular cells.42 In addition, a small amount of EPO is produced by the liver.37 EPO induces hemoglobin synthesis and serves as a differentiation factor causing the CFU-E to differentiate into pronormoblasts, the earliest visually recognized erythrocyte precursors in the bone marrow.43 Erythropoiesis is discussed in detail in Chapter 8.
Leukopoiesis
Leukopoiesis can be divided into two major categories: myelopoiesis and lymphopoiesis. Factors that promote differentiation of the CFU-GEMM into neutrophils, monocytes, eosinophils, and basophils include GM-CSF, G-CSF, monocyte colony-stimulating factor (M-CSF), IL-3, IL-5, IL-11, and kit ligand. GM-CSF stimulates the proliferation and differentiation of neutrophil and macrophage colonies from the colony-forming unit–granulocyte-monocyte. G-CSF and M-CSF stimulate neutrophil differentiation and monocyte differentiation from the colony-forming unit–granulocyte and colony-forming unit–monocyte.42 IL-3 is a multilineage stimulating factor that stimulates the growth of granulocytes, monocytes, megakaryocytes, and erythroid cells. Eosinophils require GM-CSF, IL-5, and IL-3 for differentiation. The requirements for basophil differentiation are less clear, but it seems to depend on the presence of IL-3 and kit ligand. Growth factors promoting lymphoid differentiation include IL-2, IL-7, IL-12, and IL-15 and to some extent IL-4, IL-10, IL-13, IL-14, and IL-16.38 Leukopoiesis is discussed further in Chapter 12.
Megakaryopoiesis
Earlier influences on megakaryopoiesis include GM-CSF, IL-3, IL-6, IL-11, kit ligand, and TPO.34 The stimulating hormonal factor TPO (also known as mpl ligand), along with IL-11, controls the production and release of platelets. The liver is the main site of production of TPO.44,45 Megakaryopoiesis is discussed in Chapter 13.
Analytical and Therapeutic Applications
Clinical use of growth factors approved by the U.S. Food and Drug Administration has contributed numerous options in the treatment of hematologic malignancies and solid tumors. In addition, growth factors can be used as priming agents to increase the yield of HSCs during apheresis for transplantation protocols. Advances in molecular biology have resulted in cloning of the genes that are responsible for the synthesis of various growth factors and the recombinant production of large quantities of these proteins. Table 7-2 is a concise overview of some growth factors, physiologic roles, and applications. Many more examples can be found in the literature.
Summary
• Hematopoiesis is the formation, development, and specialization of all functional blood cells.
• Hematopoiesis progresses through the mesoblastic, hepatic, and medullary phases.
• Organs that function at some point in hematopoiesis include the liver, spleen, lymph nodes, thymus, and bone marrow.
• The bone marrow is the primary site of hematopoiesis at birth and throughout life.
• In certain situations, blood cell production may occur outside the bone marrow; such production is termed extramedullary.
• The microenvironment in the bone marrow is essential for stem cell differentiation and proliferation.
• Monophyletic theory suggests that all blood cells arise from a common progenitor.
• As cells mature, certain morphologic characteristics of maturation allow specific lineages to be recognized. General characteristics of maturation include decrease in cell size, decrease in nuclear size, loss of nucleoli, condensation of nuclear chromatin, and decreased basophilia in cytoplasm. Some morphologic changes are unique to specific lineages (e.g., loss of the nucleus in RBCs).
• Cytokines and growth factors play a major role in determining differentiation of stem cells.
• Cytokines include ILs, CSFs, interferons, and others.
• Some cytokines exert influence on stem cells with multilineage potential, some are lineage specific, and some function only in combination with other cytokines.
• Cytokines are necessary to prevent premature apoptosis.
• Cytokines have contributed new options in the treatment of bone marrow malignancies, leukemias, and aplastic anemias.
Review Questions
1. The process of formation and development of blood cells is termed:
2. During midfetal life, the primary source of blood cells is the:
3. Which of the following organs is responsible for the conditioning of T lymphocytes?
4. The best source of active bone marrow from a 20-year-old would be:
5. Physiologic programmed cell death is termed:
6. Which organ is the site of sequestration of platelets?
7. The term for the earliest hematopoietic cell is:
8. Which of the following cells is not a product of the CFU-GEMM?
9. Which of the following hematopoietic growth factors is produced in the kidney?