Head and Neck
Among the earliest vertebrates, the cranial region consisted of two principal components: (1) a chondrocranium, associated with the brain and the major sense organs (nose, eye, ear); and (2) a viscerocranium, a series of branchial (pharyngeal) arches associated with the oral region and the pharynx (Fig. 14.1A). As vertebrates became more complex, the contributions of the neural crest to the head became much more prominent, and the face and many dermal (intramembranously formed) bones of the skull (dermocranium) were added. With the early evolution of the face, the most anterior of the branchial arches underwent a transformation to form the upper and lower jaws and two of the middle ear bones, the malleus and the incus. Along with an increase in complexity of the face (Fig. 14.1B) came a corresponding increase in complexity of the forebrain (telencephalon and diencephalon). From structural and molecular aspects, the rostral (anteriormost) part of the head shows distinctly different characteristics from the pharyngeal region, as follows:
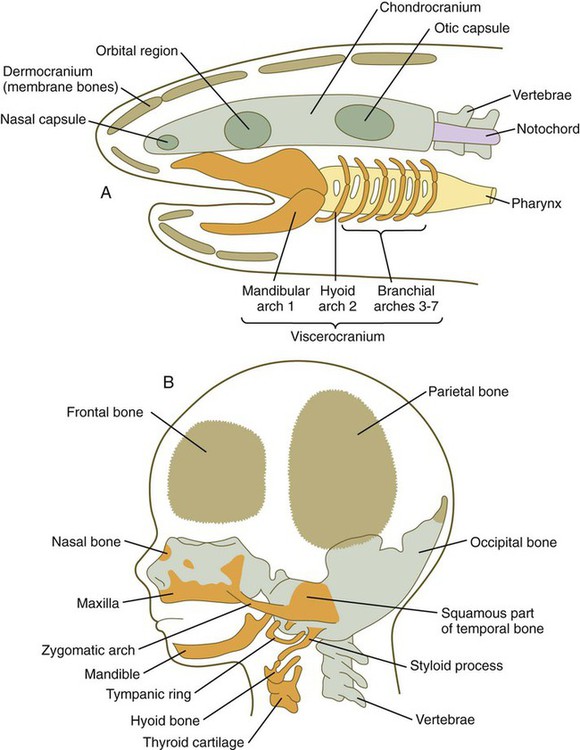
A, Skull of a primitive aquatic vertebrate, showing the chondrocranium (green), viscerocranium (orange), and dermocranium (brown). B, Human fetal head, showing the distribution of the same components of the cranial skeleton.
1. The pharyngeal region and hindbrain are highly segmented (see Fig. 14.3), whereas segmentation is less evident in the forebrain and rostral part of the head.
2. Structural segmentation in the pharyngeal region is associated with complex segmental patterns of gene expression (see Fig. 11.12).
3. Formation of the forebrain and associated structures of the rostral part of the head depends on the actions of specific genes (e.g., Lim1 [see Fig. 5.9], Emx1, Emx2, Otx1, and Otx2) and inductive signaling by the prechordal mesoderm or anterior visceral endoderm.
4. Much of the connective tissue and skeleton of the rostral (phylogenetically newer) part of the head is derived from the neural crest. The anterior end of the notochord, terminating at the hypophysis, constitutes the boundary between the mesodermally derived chondrocranium and the more rostral neural crest–derived chondrocranium. Neural crest cells are also prominent contributors to the ventral part of the pharyngeal region.
In previous chapters, the development of certain components of the head (e.g., the nervous system, neural crest, bones of the skull) is detailed. The first part of this chapter provides an integrated view of early craniofacial development to show how the major components are interrelated. The remainder of the chapter concentrates on the development of the face, pharynx, and pharyngeal arch system. Clinical Correlations 14.1 and 14.2, which appear later in the chapter, present malformations associated with the head and neck.
Early Development of the Head and Neck
Development of the head and neck begins early in embryonic life and continues until the cessation of postnatal growth in the late teens. Cephalization begins with the rapid expansion of the rostral end of the neural plate. Very early, the future brain is the dominant component of the craniofacial region. Beneath the brain, the face, which does not take shape until later in embryogenesis, is represented by the stomodeum (Fig. 14.2). In the early embryo, the stomodeum is sealed off from the primitive gut by the oropharyngeal membrane, which breaks down by the end of the first embryonic month (see Fig. 6.23). Surrounding the stomodeum are several tissue prominences that constitute the building blocks of the face (see Fig. 14.6). In keeping with its origin from the anterior neural ridge and its later serving as the tissue of origin of Rathke’s pouch, the ectoderm of the oropharyngeal membrane is first characterized by its expression of the homeodomain transcription factor Pitx-2. In the rostral midline is the frontonasal prominence, which is populated by mesenchymal cells derived from forebrain and some midbrain neural crest. On either side of the frontonasal prominence, ectodermal nasal placodes, which arose from the anterior neural ridge (see p. 95), develop into horseshoe-shaped structures, each consisting of a nasomedial process, also derived from forebrain neural crest, and a nasolateral process, derived from midbrain neural crest. Farther caudally, the stomodeum is bounded by maxillary and mandibular processes, which are also filled with neural crest–derived mesenchyme.
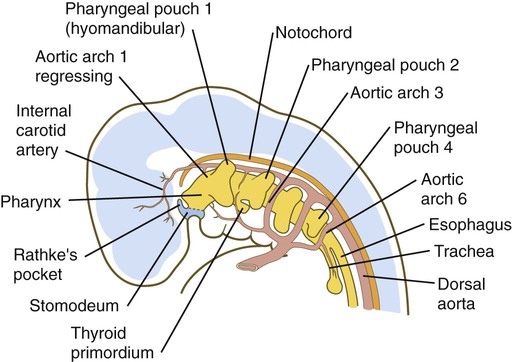
The future cervical region is dominated by the pharyngeal apparatus, consisting of a series of pharyngeal pouches, arches, and clefts. Many components of the face, ears, and glands of the head and neck arise from the pharyngeal region. Also prominent are the paired ectodermal placodes (see Fig. 13.1), which form much of the sensory tissue of the cranial region.
Tissue Components and Segmentation of Early Craniofacial Region
The early craniofacial region consists of a massive neural tube beneath which lie the notochord and a ventrally situated pharynx (see Fig. 14.2). The pharynx is surrounded by a series of pharyngeal arches. Many of the tissue components of the head and neck are organized segmentally. Figure 14.3 illustrates the segmentation of the tissue components of the head. As discussed in earlier chapters, distinct patterns of expression of certain homeobox-containing genes are associated with morphological segmentation in some cranial tissues, particularly the central nervous system (see Fig. 11.12). The chain of events between segmental patterns of gene expression and the appearance of morphological segmentation in parts of the cranial region remains incompletely understood.
Fundamental Organization of the Pharyngeal Region
Because many components of the face are derived from the pharyngeal region, an understanding of the basic organization of this region is important. In a 1-month-old embryo, the pharyngeal part of the foregut contains four lateral pairs of endodermally lined outpocketings called pharyngeal pouches and an unpaired ventral midline diverticulum, the thyroid primordium (Fig. 14.4). If the contours of the ectodermal covering over the pharyngeal region are followed, bilateral pairs of inpocketings called pharyngeal grooves, which almost make contact with the lateralmost extent of the pharyngeal pouches, are seen (see Fig. 14.4C).
Alternating with the pharyngeal grooves and pouches are paired masses of mesenchyme called pharyngeal (branchial) arches. Central to each pharyngeal arch is a prominent artery called an aortic arch, which extends from the ventral to the dorsal aorta (see Chapter 17 and Fig. 14.2). The mesenchyme of the pharyngeal arches is of dual origin. The mesenchyme of the incipient musculature originates from mesoderm, specifically the somitomeres. Much of the remaining pharyngeal arch mesenchyme, especially that of the ventral part, is derived from the neural crest, whereas mesoderm makes various contributions to the dorsal pharyngeal arch mesenchyme.
Establishing the Pattern of the Craniofacial Region
Very early in development, the cranial neural tube becomes segmented on the basis of molecular instructions, based largely on Hox gene expression (see Fig. 11.12), and this coding spills over into the neural crest cells that leave the neural tube (see Fig. 12.8). More recently, investigators have recognized that the pharyngeal endoderm also exerts a profound patterning influence on facial development. Patterning of the pharyngeal endoderm itself is heavily based on its exposure to retinoic acid. Formation of the first pharyngeal pouch does not require retinoic acid, but pharyngeal pouches 3 and 4 have an absolute requirement for retinoic acid, whereas pouch 2 needs some, but not so much, exposure to retinoic acid.
Formation of the pharyngeal arches depends on signals from the pharyngeal pouches. Even though neural crest cells are major contributors to the underlying tissues of the pharyngeal arches, experiments have shown that neural crest is not required for the formation or patterning of the pharyngeal arches. In almost all aspects of lower facial morphogenesis, the development of neural crest derivatives depends on signals from cranial ectoderm, but the ectoderm is prepatterned by signals (importantly fibroblast growth factor-8 [FGF-8]) emanating from the pharyngeal endoderm (Fig. 14.5A).
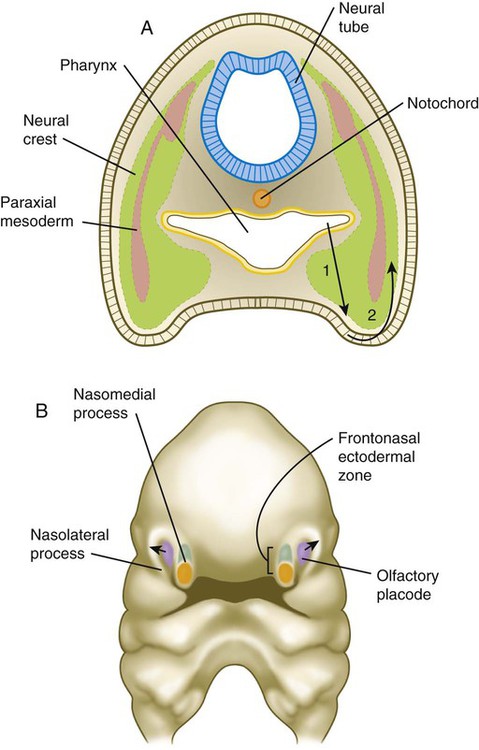
A, In the pharynx, pouch endoderm signals to the ectoderm, which signals to underlying neural crest cells. B, The frontonasal ectodermal zone, which produces signals important for the development of the midface. Dorsal cells (green) express fibroblast growth factor-8 (FGF-8), and ventral cells (orange) express sonic hedgehog (shh). The olfactory placode (violet) induces the nasolateral processes through FGF signaling.
Development of individual pharyngeal arches depends on various sets of molecular instructions. The first arch, which forms the upper and the lower jaws, is not included in the overall Hox code that underlies development of the remainder of the arches and determines their anteroposterior identity (see Fig. 12.8). Within individual pharyngeal arches, a code based on the homeobox-containing Dlx genes heavily influences dorsoventral patterning (see p. 301). Other molecular influences also strongly affect patterning of aspects of pharyngeal arch development. A major force in the patterning of pharyngeal arch 1 is endothelin-1 (Edn-1), which is secreted by the ectoderm of the arches and combines with its receptor (Ednr) on migrating neural crest cells. Although expressed on all of the pharyngeal arches, Edn-1 exerts its most prominent effect on the development of the first arch through its effects on Dlx expression.
A prominent feature of the early developing face is the unpaired frontonasal prominence, which constitutes the rostralmost part of the face (see Fig. 14.6). Originating over the bulging forebrain, the frontonasal process is filled with cranial neural crest. These neural crest cells are targets of a signaling center in the overlying ectoderm, called the frontonasal ectodermal zone (see Fig. 14.5B). This signaling center, which itself is induced by sonic hedgehog (shh) emanating from the forebrain, is an area where dorsal ectodermal cells expressing FGF-8 confront ventral ectodermal cells, which express shh. This confluence of ectodermal signals acts on the underlying neural crest cells to shape the tip of the snout. Mammals and other species with broad faces have bilateral frontonasal ectodermal zones, located at the tips of the nasomedial processes (see Fig. 14.6). In birds, which have a narrow midface tapering into a beak, the two frontonasal ectodermal zones fuse into a single signaling center. In avian embryos, transplantation of the facial ectodermal zone into an ectopic region results in the formation of a second beak.
Cellular Migrations and Tissue Displacements in the Craniofacial Region
Early craniofacial development is characterized by several massive migrations and displacements of cells and tissues. The neural crest is the first tissue to exhibit such migratory behavior, with cells migrating from the nervous system even before closure of the cranial neural tube (see Chapter 12). Initially, segmental groups of neural crest cells are segregated, especially in the pharyngeal region (see Fig. 14.3). These populations of cells become confluent, however, during their migrations through the pharyngeal arches. Much of the detailed anatomy of the facial skeleton and musculature is based on the timing, location, and interactions of individual streams of neural crest and mesodermal cells. Recognition of this level of detail (which is beyond the scope of this text) is important in understanding the basis underlying many of the numerous varieties of facial clefts that are seen in pediatric surgical clinics.
The early cranial mesoderm consists mainly of the paraxial and prechordal mesoderm (see Fig. 14.3). Although the paraxial mesoderm rostral to the occipital somites has been traditionally considered to be subdivided into somitomeres (see Fig. 6-8), some embryologists now classify it as being unsegmented mesoderm (see Fig. 14.3). Mesenchymal cells originating in the paraxial mesoderm form the connective tissue and skeletal elements of the caudal part of the cranium and the dorsal part of the neck. Within the pharyngeal arches, cells from the paraxial mesoderm initially form a mesodermal core, which is surrounded by cranial neural crest cells (see Fig. 14.5A). Myogenic cells from paraxial mesoderm undergo extensive migrations to form the bulk of the muscles of the cranial region. Similar to their counterparts in the trunk and limbs, these myogenic cells become integrated with local connective tissue to form muscles. Another similarity with the trunk musculature is that morphogenetic control seems to reside within the connective tissue elements of the muscles, rather than in the myogenic cells themselves. In the face and ventral pharynx, this connective tissue is of neural crest origin.
Another set of tissue displacements important in the cranial region is the joining of cells derived from the ectodermal placodes with cells of the neural crest to form parts of sense organs and ganglia of certain cranial nerves (see Fig. 13.1).
Development of the Facial Region
Formation of the Face and Jaws
Structures of the face and jaws originate from several primordia that surround the stomodeal depression of a 4- to 5-week-old human embryo (Fig. 14.6). These primordia consist of the following: an unpaired frontonasal prominence; paired nasomedial and nasolateral processes, which are components of the horseshoe-shaped olfactory (nasal) primordia; and paired maxillary processes and mandibular prominences, both components of the first pharyngeal arches. The upper jaw contains a mixed population of neural crest cells derived from the forebrain and midbrain, whereas the lower jaw contains mesenchymal cells derived from midbrain and hindbrain (rhombomeres 1 and 2) neural crest. The specific morphology of facial skeletal elements is determined by signals passed from the pharyngeal endoderm to the facial ectoderm and then to the neural crest precursors of the facial bones. Narrow zones of pharyngeal endoderm control the morphogenesis of specific portions of the skeleton of the lower face. FGF-8 signaling from the facial ectoderm plays a key role in patterning of the facial skeleton.
The frontonasal process is a prominent structure in the earliest phases of facial development, and its formation is the result of an exquisitely sensitive signaling system that begins with the synthesis of retinoic acid in a localized region of ectoderm opposite the forebrain and continues with the action of shh produced by the ventral forebrain. The action of shh, through the mediation of the most rostral wave of neural crest cells, underlies the establishment of the frontonasal ectodermal zone, located at the tips of the nasomedial processes (see p. 298). The signaling molecules (FGF-8 and shh) emanating from this zone stimulate cell proliferation in the neural crest mesenchyme of the frontonasal process. In the absence of such signaling, cell death in the region increases, and cell proliferation decreases, resulting in various midfacial defects (see Clinical Correlation 14.1). Retinoic acid is unusual in that both deficiencies and excess amounts can cause very similar defects. From 4 to 5 weeks, the frontonasal process is a dominant structure of the early face (see Fig. 14.6), but with subsequent growth of the maxillary process and the nasomedial and nasolateral processes, it recedes from the oral region. The nasolateral process develops as a result of FGF signaling emanating from the nasal pit.
As with the limb buds, outgrowth of the frontonasal prominence and the maxillary and mandibular processes depends on mesenchymal-ectodermal interactions. In contrast to the limb, however, the signaling system (FGF and shh) is concentrated in the apical ectoderm of these processes, where it may act as a morphogenetic organizer and a stimulus for mesenchymal outgrowth of the facial primordia. The homeobox-containing gene Msx1 is expressed in the rapidly proliferating mesenchyme at the tips of the facial primordia. The parallel with expression of Msx1 in the subectodermal region of the limb (see p. 200) suggests that similar mechanisms operate in outgrowing limb and facial primordia. Hox genes are not expressed in the first arch, and the presence of Otx-2 distally, coupled with the absence of Hox proximally, provides the molecular basis for the development of the first arch.
Through differential growth between 4 and 8 weeks (see Fig. 14.6), the nasomedial and maxillary processes become more prominent and ultimately fuse to form the upper lip and jaw (Fig. 14.7). As this is occurring, the frontonasal prominence, which was a prominent tissue bordering the stomodeal area in the 4- and 5-week-old embryo, is displaced as the two nasomedial processes merge. The merged nasomedial processes form the intermaxillary segment, which is a precursor for (1) the philtrum of the lip, (2) the premaxillary component of the upper jaw, and (3) the primary palate.
Between the maxillary process and the nasal primordium (nasolateral process) is a nasolacrimal groove (naso-optic furrow) that extends to the developing eye (see Fig. 14.6). The ectoderm of the floor of the nasolacrimal groove thickens to form a solid epithelial cord, which detaches from the groove. The epithelial cord undergoes canalization and forms the nasolacrimal duct and, near the eye, the lacrimal sac. The nasolacrimal duct extends from the medial corner of the eye to the nasal cavity (inferior meatus) and, in postnatal life, acts as a drain for lacrimal fluid. This connection explains why people can have a runny nose when crying. Meanwhile, the expanding nasomedial process fuses with the maxillary process, and over the region of the nasolacrimal groove, the nasolateral process merges with the superficial region of the maxillary process. The region of fusion of the nasomedial and maxillary processes is marked by an epithelial seam, called the nasal fin. Mesenchyme soon penetrates the nasal fin, and the result is a continuous union between the nasomedial and maxillary processes.
The lower jaw is formed in a simpler manner. The bilateral mandibular prominences enlarge, and their medial components merge in the midline, to form the point of the lower jaw. The midline dimple that is seen in the lower jaw of some individuals is a reflection of variation in the degree of merging of the mandibular prominences. A prominent cartilaginous rod called Meckel’s cartilage differentiates within the lower jaw (see Fig. 14.36D). Derived from neural crest cells of the first pharyngeal arch, Meckel’s cartilage forms the basis around which membrane bone (which forms the definitive skeleton of the lower jaw) is laid down. Experimental evidence indicates that the rodlike shape of Meckel’s cartilage is related to the inhibition of further chondrogenesis by the surrounding ectoderm. If the ectoderm is removed around Meckel’s cartilage, large masses of cartilage form instead of a rod. These properties are similar to the inhibitory interactions between ectoderm and chondrogenesis in the limb bud. A later-acting influence in growth of the lower jaw is the planar cell polarity pathway, which influences outgrowth of Meckel’s cartilage. If this pathway is disrupted, the mandible will not develop to its normal length.
Formation of the Palate
The early embryo possesses a common oronasal cavity, but in humans the palate forms between 6 and 10 weeks to separate the oral from the nasal cavity. The palate is derived from three primordia: an unpaired median palatine process and a pair of lateral palatine processes (Figs. 14.8 and 14.9).
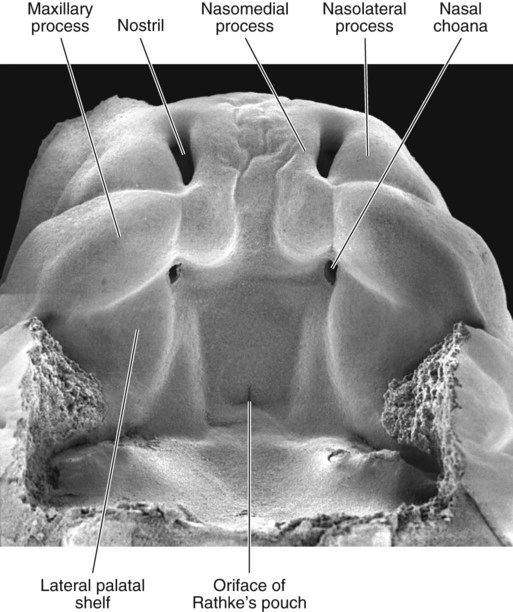
The lower jaw has been removed, and one is looking toward the roof of the oronasal cavity. (From Steding G: The anatomy of the human embryo, Basel, 2009, Karger; courtesy of Dr. J. Männer.)
The median palatine process is an ingrowth from the newly merged nasomedial processes. As it grows, the median palatine process forms a triangular bony structure called the primary palate. In postnatal life, the skeletal component of the primary palate is referred to as the premaxillary component of the maxilla. The four upper incisor teeth arise from this structure (Fig. 14.10).
Formation of the palate involves (1) growth of the palatal shelves, (2) their elevation, (3) their fusion, and (4) removal of the epithelial seam at the site of fusion. The lateral palatine processes, which are the precursors of the secondary palate, first appear as outgrowths of the maxillary processes during the sixth week. At first, they grow downward on either side of the tongue (Fig. 14.11). Similar to other facial primordia, outgrowth of the palatal shelves involves ectodermal-mesenchymal interactions and specific growth factors. FGF-10 produced in the mesenchyme of the forming palatal shelf is bound to an FGF receptor in the ectoderm (Fig. 14.12). This process stimulates the release of shh from the ectoderm. Shh causes the release of BMP-2 in the mesenchyme. BMP-2 and Msx-1, which interacts with BMP-4, stimulate proliferation of the mesenchymal cells of the palatal shelf and its resultant growth. During week 7, the lateral palatine processes (palatal shelves) dramatically dislodge from their positions alongside the tongue and become oriented perpendicularly to the maxillary processes. The apices of these processes meet in the midline and begin to fuse.
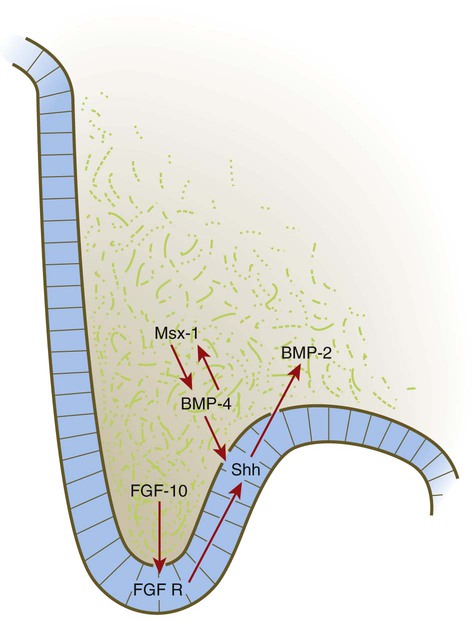
BMP, bone morphogenetic protein; FGF, fibroblast growth factor; FGF R, fibroblast growth factor receptor; Shh, sonic hedgehog.
Another structure involved in formation of the palate is the nasal septum (see Figs. 14.8 and 14.11). This midline structure, which is a downgrowth from the frontonasal prominence, reaches the level of the palatal shelves when the palatal shelves fuse to form the definitive secondary palate. Rostrally, the nasal septum is continuous with the primary palate.
At the gross level, the palatal shelves fuse in the midline, but rostrally they also join the primary palate. The midline point of the fusion of the primary palate with the two palatal shelves is marked by the incisive foramen (see Fig. 14.10).
1. What causes the disappearance of the midline epithelial seam?
2. What signals result in the diverse pathways of differentiation of the epithelium on either side of the palate?
The disappearance of the midline epithelial seam after the approximation of the palatal shelves involves several fundamental developmental processes (Fig. 14.13). Some of the epithelial cells at the fusion seam undergo apoptosis and disappear. Others may migrate out from the plane of fusion and become inserted into the epithelial lining of the oral cavity. Still other epithelial cells undergo a morphological transformation into mesenchymal cells. Transforming growth factor-β3 (TGF-β3) is expressed by the ectodermal cells of the distal rim of the palatal shelves just before fusion and loses prominence shortly thereafter. It plays an important role in stimulating apoptosis of the epithelial cells at the fusion seam. In TGF-β3–mutant mice, the lateral palatal shelves approximate in the midline, but the epithelial seam fails to disappear, and the mice develop isolated cleft palate.
Formation of the Nose and Olfactory Apparatus
The human olfactory apparatus first becomes visible at the end of the first month as a pair of thickened ectodermal nasal placodes located on the frontal aspect of the head (Fig. 14.14A). Similar to the formation of the lens placodes, the formation of the nasal placodes requires the expression of Pax-6 and the action of retinoids produced in the forebrain. In the absence of Pax-6 expression, neither the nasal placodes nor the lens placodes form. The nasal placodes originate from the anterolateral edge of the neural plate before its closure.
Soon after their formation, the nasal placodes form a surface depression (the nasal pits) surrounded by horseshoe-shaped elevations of mesenchymal tissue with the open ends facing the future mouth (see Fig. 14.6). The two limbs of the mesenchymal elevations are the nasomedial and nasolateral processes. Formation of the thickened nasal processes depends on the retinoid-stimulated production of FGF-8, which stimulates proliferation of the mesenchymal cells within the nasal processes. The source of these retinoids is the epithelium of the nasal pit itself. Meanwhile, production of retinoids by the forebrain diminishes. As a consequence, the frontonasal prominence, which depends on forebrain retinoids for supporting proliferation of its mesenchymal cells, is reduced. As the nasal primordia merge toward the midline during weeks 6 and 7, the nasomedial processes form the tip and crest of the nose along with part of the nasal septum, and the nasolateral processes form the wings (alae) of the nose. The receding frontonasal process contributes to part of the bridge of the nose.
Meanwhile, the nasal pits continue to deepen toward the oral cavity and form substantial cavities themselves (see Fig. 14.14). By weeks, only a thin oronasal membrane separates the oral cavity from the nasal cavity. The oronasal membrane soon breaks down, thereby making the nasal cavities continuous with the oral cavity through openings behind the primary palate called nasal choanae (see Fig. 14.9). Shortly after breakdown of the oronasal membrane, however, the outer part of the nasal cavity becomes blocked with a plug of epithelial cells, which persists until the end of the fourth month. With the fusion of the lateral palatal shelves, the nasal cavity is considerably lengthened and ultimately communicates with the upper pharynx.
At 6 to 7 weeks, a pair of epithelial ingrowths can be seen in each side of the nasal septum near the palate. Developing as invaginations from the medial portion of the nasal placode, these diverticula, known as vomeronasal organs (see Fig. 14.11B), reach a maximum size of about 6 to 8 mm at around the sixth fetal month and then begin to regress, leaving small cystic structures. In most mammals and many other vertebrates, the vomeronasal organs, which are lined with a modified olfactory epithelium, remain prominent and are involved in the olfaction of food in the mouth or sexual olfactory stimuli (e.g., pheromones).
The dorsalmost epithelium of the nasal pits undergoes differentiation as a highly specialized olfactory epithelium (see Fig. 14.14). Differentiation of the olfactory organ and the vomeronasal organ requires the action of FGF-8, which is produced in a signaling zone that surrounds the nasal pit. Beginning in the embryonic period and continuing throughout life, the olfactory epithelium is able to form primitive sensory bipolar neurons, which send axonal projections toward the olfactory bulb of the brain. Preceding axonal ingrowth, some cells break free from this epithelium and migrate toward the brain. Some of these cells may synthesize a substrate for the ingrowth of the olfactory axons. Other cells migrating from the olfactory placode (specifically, the vomeronasal primordium) synthesize luteinizing hormone–releasing hormone and migrate to the hypothalamus, the site of synthesis and release of this hormone in adults. The embryonic origin of these cells in the olfactory placode helps to explain the basis for Kallmann’s syndrome, which is characterized by anosmia and hypogonadotropic hypogonadism. Cells of the olfactory placode also form supporting (sustentacular) cells and glandular cells in the olfactory region of the nose. Physiological evidence shows that the olfactory epithelium is capable of some function in late fetal life, but full olfactory function is not attained until after birth.
Formation of the Salivary Glands
Starting in the sixth week, the salivary glands originate as solid, ridgelike thickenings of the oral epithelium (Fig. 14.15). Extensive epithelial shifts in the oral cavity make it difficult to determine the germ layer origins of the salivary gland epithelium. The parotid glands are probably derived from ectoderm, whereas the submandibular and sublingual glands are thought to be derived from endoderm.
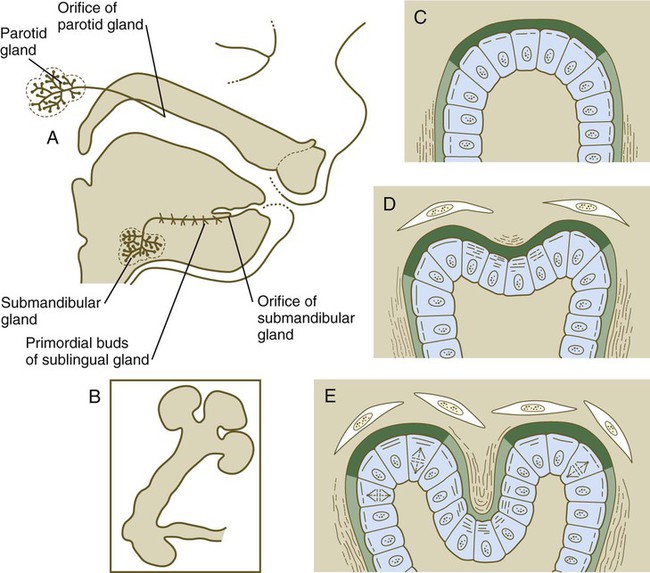
A, Salivary gland development in an 11-week-old human embryo. B, Development of salivary gland epithelium in vitro. C, Accumulation of newly synthesized glycosaminoglycans (dark green) in the basal lamina at the end of a primary lobule. D, Early cleft formation is associated with the contraction of bundles of microfilaments in the apices of the epithelial cells lining the cleft. Collagen fibers (wavy lines) are lined up lateral to the lobule and in the newly forming cleft. E, As the cleft deepens, glycosaminoglycan synthesis is reduced in the cleft, and collagen deposition continues. (C through E show the relationship between disposition of the extracellular matrix and lobulation of the glandular primordium.)
Clinical Correlation 14.1 presents malformations of the face and oral regions.
Formation of the Teeth
Patterning of the Dentition
Each human tooth has a distinctive morphology, and each type of tooth forms in a characteristic location. For many years, virtually nothing was known about the patterning of the dentition, but analysis of certain types of genetically modified mice has provided some concrete clues to the molecular basis of dental patterning. Both the dental field overall and the patterning of the dentition take shape very early in craniofacial development, before any overt indication of tooth formation. The expression of Pitx-2 (a bicoid-related transcription factor; see Fig. 4.1) outlines first the entire ectodermal dental field, and later the epithelium of the individual tooth germs. The homeobox-containing genes Dlx-1 and Dlx-2 are expressed in the maxillary arch and in the proximal part of the mandibular arch. When both these genes are knocked out in mice, the upper jaw develops without molar teeth, although molars develop in the lower jaws. The incisor teeth in both jaws develop normally. Another homeobox-containing gene, Barx-1, is induced by FGF-8 in proximal ectoderm of the mandibular process, and in the formation of molar teeth in knockouts it may compensate for the absence of Dlx-1 and Dlx-2 in the lower jaw. FGF-8 acts proximally to restrict Barx-1 and Dlx-2 to guide the molar-producing domain, and BMP-4 acts distally to activate Msx-1 and Msx-2 in guiding the formation of incisor teeth.
Mammals have only a single row of teeth in each jaw. This is controlled by two overlapping gradients of opposite polarity along the lingual-buccal axis (Fig. 14.20). On the buccal side of the jaw, a high concentration of BMP-4 stimulates the expression of Msx-1 in the dental mesenchyme in the normal process of tooth development, thus accounting for the development of the normal teeth. On the lingual side of the jaw, a high concentration of the transcription factor Osr-2 (the mammalian equivalent of the Drosophila pair-rule gene odd skipped; see Fig. 4.1) inhibits the BMP-Msx axis and consequently tooth formation in that area. When Osr-2 is inactivated, BMP-4–Msx-1 activity on the lingual side of the jaw is not inhibited, and supernumerary teeth form on the lingual side of the normal row of teeth (see Fig. 14.20B). Enhancing or inhibiting the function of many of the other genes involved in tooth development can also lead to the formation of supernumerary teeth, but these genes do not cause expansion of the entire dental field as does Osr-2.
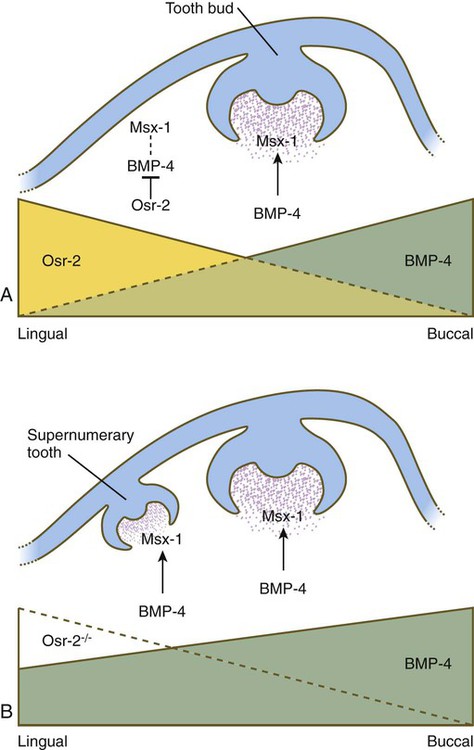
Normally, a higher concentration of Osr-2 in the lingual region suppresses the tooth-inducing activity of the bone morphogenetic protein-4 (BMP-4)–Msx-1 pathway (A). In mutants (B), the absence of Osr-2 activity permits the formation of an extra row of teeth on the lingual side of the normal row. (Based on Cobourne MT and Sharpe PT: Semin Cell Dev Biol 21:314-324, 2010.)
Stages of Tooth Development
Although each tooth has a specific time sequence and morphology of development, certain general developmental stages are common to all teeth (Fig. 14.21). As the dental lamina grows into the neural crest mesenchyme, epithelial primordia of the individual teeth begin to take shape as tooth buds. In keeping with their interactive mode of development, the tooth buds are associated with condensations of mesenchymal cells. The tooth bud soon expands, passing through a mushroom-shaped cap stage before entering the bell stage (see Fig. 14.21D).
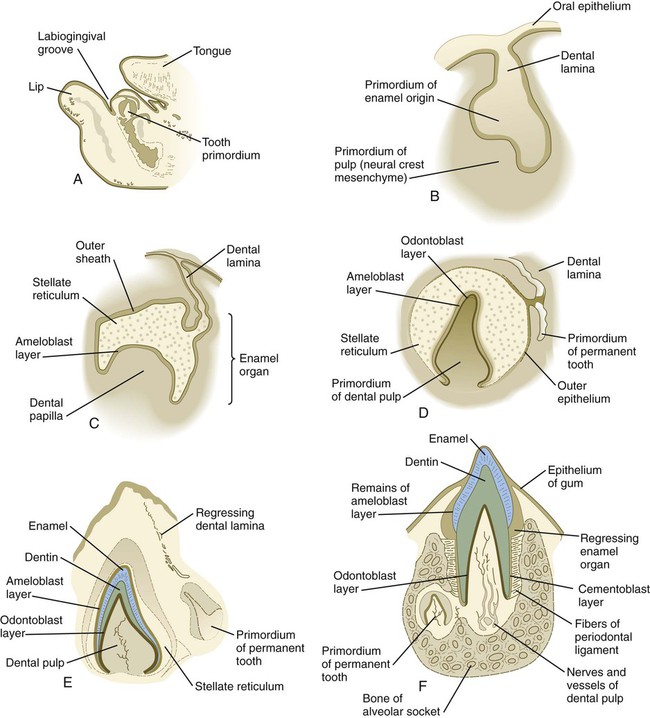
A, Parasagittal section through the lower jaw of a 14-week-old human embryo showing the relative location of the tooth primordium. B, Tooth primordium in the bud stage in a 9-week-old embryo. C, Tooth primordium at the cap stage in an 11-week-old embryo, showing the enamel organ. D, Central incisor primordium at the bell stage in a 14-week-old embryo before deposition of enamel or dentin. E, Unerupted incisor tooth in a term fetus. F, Partially erupted incisor tooth showing the primordium of a permanent tooth near one of its roots. (After Patten B: Human embryology, ed 3, New York, 1968, McGraw-Hill.)
By the bell stage, the tooth primordium already has a complex structure, even though it has not formed any components of the definitive tooth. The epithelial component, called the enamel organ, is still connected to the oral epithelium by an irregular stalk of dental lamina, which soon begins to degenerate. The enamel organ consists of an outer sheath of epithelium, a mesenchymelike stellate reticulum, and an inner epithelial ameloblast layer. Ameloblasts are the cells that begin to secrete the enamel of the tooth. The initial formation of ameloblasts depends on the actions of the transcription factor Tbx-1. Within the concave surface of the enamel organ is a condensation of neural crest mesenchyme called the dental papilla. Cells of the dental papilla opposite the ameloblast layer become transformed into columnar epithelial cells called odontoblasts (Fig. 14.22). These cells secrete the dentin of the tooth. Attached to the dental lamina close to the enamel organ is a small bud of the permanent tooth (see Fig. 14.21E and F). Although delayed, it goes through the same developmental stages as the deciduous tooth.
Late in the bell stage, the odontoblasts and ameloblasts begin to secrete precursors of dentin and enamel, beginning first at the future apex of the tooth. Over several months, the definitive form of the tooth takes shape (see Fig. 14.21). Meanwhile, a condensation of mesenchymal cells forms around the developing tooth. Cells of this structure, called the dental sac, produce specialized extracellular matrix components (cementum and the periodontal ligament) that provide the tooth with a firm attachment to the jaw. While these events are occurring, the tooth elongates and begins to erupt through the gums (gingiva).
Tissue Interactions in Tooth Development
Teeth are formed by a series of inductive interactions. Tissue recombination experiments have shown that the thickened ectoderm of the dental lamina initiates tooth formation. Early in development, dental ectoderm can induce nondental cranial neural crest mesenchyme to participate in the formation of a tooth, but predental neural crest mesenchyme cannot induce nondental ectoderm to form a tooth. Research suggests that the transcription factor Lef-1 may stimulate the predental surface ectoderm to secrete FGF-8, which induces the underlying mesenchyme to express Pax-9 (Fig. 14.23). In the absence of Pax-9 expression, tooth development does not proceed past the bud stage. BMP-2 and BMP-4, also produced by the surface ectoderm, inhibit the action of FGF-8. Investigators have suggested that such inhibition is the basis for the non–tooth-forming spaces between the developing teeth. More recent research has shown that lateral inhibition through the Delta/Notch system is also involved in dental spacing. Another characteristic transcription factor induced in the mesenchyme beneath the thickened dental lamina is Msx-1.
Slightly later in dental development, BMP-4, instead of functioning as an inhibitor, acts along with FGF-8 and shh to stimulate the mesenchyme of the tooth bud to express a variety of characteristic molecules, including the following: the transcription factors Msx-1, Msx-2, and EGR-1; the extracellular matrix molecules tenascin and syndecan; and BMP-4. If the overlying ectoderm is separated from the neural crest mesenchyme, the predental mesenchyme will not develop into a dental papilla, and a tooth will not form. The role of BMP-4 as an inducer was shown by adding a small bead soaked in BMP-4 to a small mass of cultured predental neural crest mesenchyme (see Fig. 14.23B). Under the influence of the BMP-4 released from the bead, the mesenchyme began to express Msx-1, Msx-2, Egr-1, and BMP-4. The mesenchyme did not produce tenascin or syndecan, however, thus showing that other signals in addition to BMP-4 are required to achieve the full inductive response.
Under the inductive influence of the dental mesenchyme, now called the dental papilla, a small group of ectodermal cells at the tip of the dental papilla ceases dividing. This mass of cells, called the enamel knot (Fig. 14.24), serves as a signaling center that regulates the shape of the developing tooth. Through the production of several signaling molecules, including shh, FGF-4, and BMP-2, BMP-4, and BMP-7, the enamel knot stimulates the proliferation of cells in the dental cap down and away from itself. By serving as a fixed point in this process, the enamel knot determines the site of the tip of a cusp in the developing tooth. In the case of molar teeth, which have multiple cusps, secondary enamel knots form—one for each cusp. The location and spacing of secondary enamel knots are determined by two molecules, both induced by BMPs. p21 is strongly expressed at sites where secondary enamel knots form, and ectodin is expressed in the intervening spaces. In the absence of ectodin, the secondary enamel knots and the resulting cusps of the molar teeth become massive because of the lack of its restraining influence. Ultimately, the cells of the enamel knot undergo apoptosis, possibly under the influence of BMP-4, which stimulates cell death in several other developmental systems. The appearance of apoptosis terminates the inductive signaling from this structure.
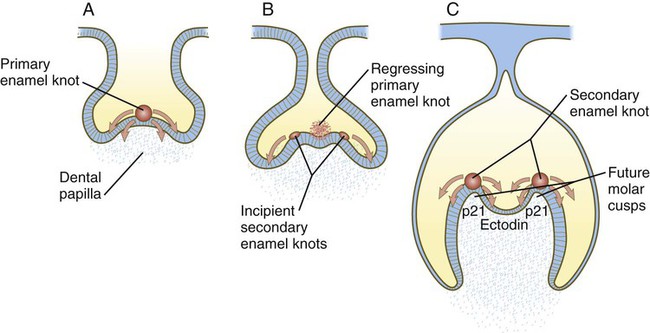
A, The primary enamel knot induces proliferation on either side. B, Two secondary enamel knots form on each side of the regressing primary enamel knot. C, Under the influence of the secondary enamel knots, the future cusps of the molar begin to form.
Formation of Dentin and Enamel
Late in their differentiation, odontoblasts withdraw from the cell cycle, elongate, and start secreting predentin from their apical surfaces, which face the enamel organ. The production of predentin signals a shift in the patterns of synthesis from type III collagen and fibronectin to type I collagen and other molecules (e.g., dentin phosphoprotein, dentin osteocalcin) that characterize the dentin matrix. The first dentin is laid against the inner surface of the enamel organ at the apex of the tooth (see Fig. 14.22B). With the secretion of additional dentin, the accumulated material pushes the odontoblastic epithelium from the odontoblast-ameloblast interface.
Tooth Eruption and Replacement
Each tooth has a specific time of eruption and replacement (Table 14.1). With the growth of the root, the enamel-covered crown pushes through the oral epithelium. The sequence of eruption begins with the central incisor teeth, usually a few months after birth, and continues generally stepwise until the last of the deciduous molars forms at the end of the second year. A total of 20 deciduous teeth are formed.
Table 14.1
Usual Times of Eruption and Shedding of Deciduous and Permanent Teeth
Teeth | Eruption | Shedding |
Deciduous | ||
Central incisors | 6-8 mo | 6-7 yr |
Lateral incisors | 7-10 mo | 7-8 yr |
Canines | 14-18 mo | 10-12 yr |
First molars | 12-16 mo | 9-11 yr |
Second molars | 20-24 mo | 10-12 yr |
Permanent | ||
Central incisors | 7-8 yr | |
Lateral incisors | 8-9 yr | |
Canines | 11-13 yr | |
First premolars | 10-11 yr | |
Second premolars | 11-12 yr | |
First molars | 6-7 yr | |
Second molars | 12-13 yr | |
Third molars | 15-25 yr |
Meanwhile, the primordium of the permanent tooth is embedded in a cavity extending into the bone on the lingual side of the alveolar socket in which the tooth is embedded (see Fig. 14.21F). As the permanent tooth develops, its increasing size causes resorption of the root of the deciduous tooth. When a sufficient amount of the root is destroyed, the deciduous tooth falls out, leaving room for the permanent tooth to take its place. The sequence of eruption of the permanent teeth is the same as that of the deciduous teeth, but an additional 12 permanent teeth (for a total of 32) are formed without deciduous counterparts.
Clinical Correlation 14.2 presents malformations of the teeth and dentition.
Development of the Pharynx and Its Derivatives
Considering the complexity of the structural arrangements of the embryonic pharynx, it is not surprising that many different structures originate in the pharyngeal region. This complexity provides many opportunities for abnormal development, as discussed in Clinical Correlation 14.3 at the end of this section. This section details aspects of later development that lead to the formation of specific structures. Adult derivatives of the regions of the pharynx and pharyngeal arches are summarized in Figure 14.34.
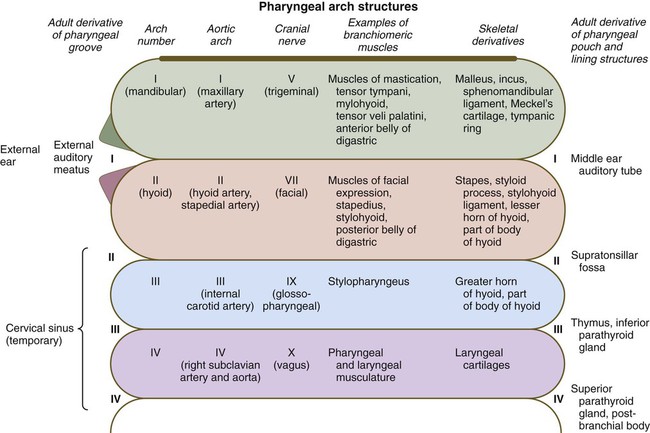
External Development of the Pharyngeal Region
Externally, the pharyngeal (branchial) region is characterized by four pharyngeal arches and grooves interposed between the arches (Fig. 14.35; see Fig. 14.4). These structures give rise to a diverse array of derivatives.
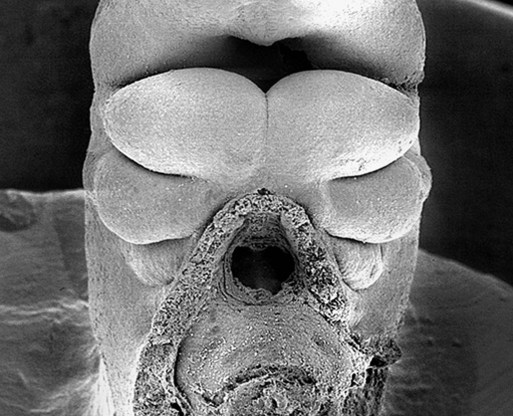
The stomodeum is the dark area at the top of the figure. Beneath that, three pairs of pharyngeal arches are prominent. (From Steding G: The anatomy of the human embryo, Basel, 2009, Karger; courtesy of Dr. J. Männer.)
Pharyngeal Arches
As mentioned earlier, the endoderm of the foregut is the main driver in organizing development of the pharynx. Under the influence of various concentrations of retinoic acid (see p. 297), combinations of Hox genes determine the craniocaudal identity of the segments of the pharynx. The first pharyngeal arch develops independently of Hox genes, whereas expression of Hoxa-2 and Hoxa-3 is required for formation of the second and third pharyngeal arches. Similar to the pharyngeal arches, the pharyngeal pouches, as well as having individual identities, are characterized by highly regionalized molecular patterns in the dorsoventral and the craniocaudal axes. Signals from the pharyngeal endoderm pattern the pharyngeal arches even before cranial neural crest cells enter the arches (see Fig. 14.5). Tbx-1 expression in the early pharyngeal endoderm influences FGF-8 signaling, and in its absence pharyngeal pouches do not form normally, and a sequence of malformations reminiscent of DiGeorge’s syndrome occurs.
In addition to being packed with mesenchyme (mainly of neural crest origin except for the premuscle mesoderm, which migrates from the somitomeres), each pharyngeal arch is associated with a major artery (aortic arch) and a cranial nerve (see Fig. 14.34). Each also contains a central rod of precartilaginous mesenchyme, which is transformed into characteristic adult skeletal derivatives. Understanding the relationship between the pharyngeal arches and their innervation and vascular supply is very important, because tissues often maintain their relationship with their original nerve as they migrate out or become displaced from their site of origin in the pharyngeal arch system.
The first pharyngeal arch (mandibular) contributes mainly to structures of the face (both mandibular and maxillary portions) and ear (see Fig. 14.34). Its central cartilaginous rod, Meckel’s cartilage, is a prominent component of the embryonic lower jaw until it is surrounded by locally formed intramembranous bone, which forms the definitive jaw. During later development, the distal part of Meckel’s cartilage undergoes resorption because of extensive apoptosis of the chondrocytes. More dorsally, Meckel’s cartilage forms the sphenomandibular ligament, the anterior ligament of the malleus, and the malleus (Fig. 14.36). In addition, the incus arises from a primordium of the quadrate cartilage. The first-arch musculature is associated with the masticatory apparatus, the pharynx, and the middle ear. A common feature of these muscles is their innervation by the trigeminal nerve (cranial nerve V).
The molecular basis for development of the first pharyngeal arch is quite different from that of the other arches, starting with the origin of the neural crest that populates it. The neural crest cells that populate the first arch are derived from rhombomeres 1 and 2 and midbrain, which are anterior to the expression domain of the Hox genes. Cellular precursors of the first-arch mesenchyme are instead associated with the expression of Otx-2. The role of signaling molecules and transcription factors, such as Dlx and Msx, is discussed earlier (see pp. 299-301).
Patterning of the second pharyngeal arch is strongly influenced by the homeobox gene Hoxa2. When this gene is knocked out in mice, skeletal derivatives of the second arch fail to form. The second arch in such mutant animals contains mirror-image duplicates of many of the proximal bones of the first-arch skeleton. This may be a result of the response of the mutant second-arch mesenchyme to an ectodermal signal from the first pharyngeal cleft that plays a role in patterning the first arch. The formation of mirror-image first-arch structures is analogous to the formation of mirror-image supernumerary limbs after transplantation of the zone of polarizing activity in the limb bud (see p. 198). The finding that only proximal structures are affected reflects the different genetic control of proximal and distal segments of the arches.
Pharyngeal Grooves
The first pharyngeal groove is the only one that persists as a recognizable adult structure: the external auditory meatus. Grooves II and III become covered by the enlarged external portion of the second arch (a phylogenetic homologue of the operculum [gill cover] of fish). The enlargement of the second arch is caused by the presence of a signaling center in the ectoderm at its tip; such a signaling center is not present in arches 3 or 4. As in the facial primordia, this signaling center produces shh, FGF-8, and BMP-7, which stimulate growth of the underlying mesenchyme. During the period of their overshadowing by the hyoid (second) arch, grooves II and III are collectively known as the cervical sinus (Fig. 14.37; see Fig. 13.27). As development progresses, the posterior ectoderm of the second arch fuses with ectoderm of a swelling (cardiac swelling) just posterior to the fourth arch, thus causing the cervical sinus to disappear and the external contours of the neck to become smooth.
Internal Development of the Stomodeal and Pharyngeal Regions
Pharynx and Pharyngeal Pouches
As with their corresponding first pharyngeal clefts, the first pharyngeal pouches become intimately involved in the formation of the ear. The end of each pouch expands to become the tympanic cavity of the middle ear, and the remainder becomes the auditory (eustachian) tube, which connects the middle ear with the pharynx (see Fig. 13.24).
The third pharyngeal pouch is a more complex structure, consisting of a solid, dorsal epithelial mass and a hollow, elongated ventral portion (see Fig. 14.37). Through yet incompletely known mechanisms, the third pouch endoderm is specified very early into a parathyroid/thymus primordium. In a manner reminiscent of dorsoventral patterning of the neural tube (see p. 222), delineation of the common endodermal primordium into parathyroid and thymic segments occurs in response to opposing shh and BMP-4 gradients, with high levels of shh promoting a parathyroid fate and high BMP-4 leading to thymic development. Future parathyroid cells can be recognized by their expression of Gcm-2 (Glial cells missing) transcription factor, whereas future thymus cells express Foxn-1. By 5 weeks of gestation, cells identifiable as parathyroid tissue can be recognized in the endoderm of the solid dorsal mass. The ventral elongation of the third pouch differentiates into the epithelial portion of the thymus gland. The primordia of the thymus and the parathyroid glands lose their connection with the third pharyngeal pouch and migrate caudally from their site of origin. Although the parathyroid III primordia initially comigrate with the thymic primordia, they ultimately continue to migrate toward the midline. There they join with the thyroid gland and pass the parathyroid primordia of the fourth pouch to form the inferior parathyroid glands. The third pharyngeal pouch disappears.
The fourth pharyngeal pouch is organized much as the third, with a solid, bulbous, dorsal parathyroid IV primordium. It also contains a small ventral epithelial outpocketing, which contributes a minor component to the thymus in some species. In humans, the thymic component of the fourth pouch is vestigial. At the ventralmost part of each fourth pouch is another structure called the postbranchial (ultimobranchial) body (see Fig. 14.37). Neural crest cells migrate into the postbranchial bodies and ultimately become the secretory component of these structures.
Thyroid
The unpaired primordium (thyroid bud) of the thyroid gland appears in the ventral midline of the pharynx between the first and second pouches (see Fig. 14.37). Starting during the fourth week as an endodermal thickening just caudal to the median tongue bud (tuberculum impar), the thyroid primordium soon elongates to form a prominent downgrowth called the thyroid diverticulum. The pathway of caudal extension of the bilobed thyroid diverticulum is determined by the pattern of arteries in the neck and extension and continues during pharyngeal development. During its caudal migration, the tip of the thyroid diverticulum expands and bifurcates to form the thyroid gland itself, which consists of two main lobes connected by an isthmus. For some time, the gland remains connected to its original site of origin by a narrow thyroglossal duct.
Hypophysis
The hypophysis (pituitary gland) develops from two initially separate ectodermal primordia that secondarily unite. One of the primordia, called the infundibular process, forms as a ventral downgrowth from the floor of the diencephalon. The other primordium is Rathke’s pouch, a midline outpocketing from the stomodeal ectoderm that extends toward the floor of the diencephalon as early as the fourth week. An inductive event from the overlying diencephalon, mediated first by BMP-4 and then by FGF-8, stimulates the formation of a Rathke’s pouch primordium in the dorsal stomodeal ectoderm and provides cues for molecular events that stimulate cell proliferation. Through the action of Hesx-1 (homeobox gene expressed in embryonic stem cells, formerly called Rpx) and the Lim-type homeobox-containing genes Lhx3 and Lhx4, Rathke’s pouch primordium forms the definitive Rathke’s pouch (Fig. 14.38). In the early embryo, the cells in Rathke’s pouch originate in the anterior ridge of the neural plate.
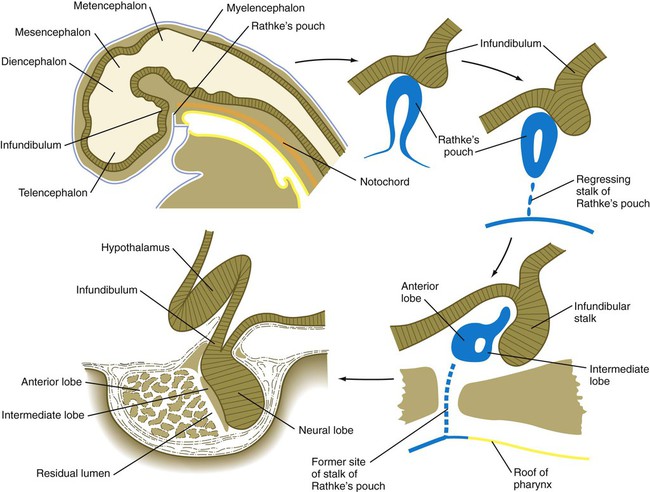
Upper left, Reference diagram showing a sagittal section through a 4-week-old human embryo.
The infundibular process is intimately related to the hypothalamus (see Fig. 1.15), and certain hypothalamic neurosecretory neurons send their processes into the infundibular process, which ultimately becomes the neural lobe of the hypophysis. Throughout development, the histological structure of the infundibulum retains a neural character.
As development proceeds, Rathke’s pouch elongates toward the infundibulum (see Fig. 14.38). While its blind end partially enfolds the infundibulum like a double-layered cup, the stalk of Rathke’s pouch begins to regress. The outer wall of the cup thickens and assumes a glandular appearance in the course of its differentiation into the pars distalis (anterior lobe) of the hypophysis. The inner layer of the cup, which is closely adherent to the neural lobe, becomes the pars intermedia (intermediate lobe). It remains separated from the anterior lobe by a slitlike residual lumen, which represents all that remains of the original lumen of Rathke’s pouch.
With the progression of pregnancy, the hypophysis undergoes a phase of cytodifferentiation. Late in the fetal period, specific cell types begin to produce small amounts of hormones. Molecular cascades underlying the differentiation of specific cell types in the pituitary are being discovered (Box 14.1).
Thymus and Lymphoid Organs
The thymus and the bursa or mammalian equivalent are commonly referred to as central lymphoid organs. The lymphoid structures that are seeded by B and T lymphocytes are called peripheral lymphoid organs. (Figure 14.39 shows the development and function of the lymphoid system.) Small cervical thymus glands have been discovered in the neck in mice. How their function fits into that of the overall lymphoid system remains to be determined.
Formation of the Tongue
In 5-week-old embryos, the tongue is represented by a pair of lateral lingual swellings in the ventral regions of the first pharyngeal arches and two median unpaired swellings. The tuberculum impar is located between the first and second arches, and the copula (yoke) unites the second and third arches (Figs. 14.40A and B and 14.41). The foramen cecum, which marks the original location of the thyroid primordium, serves as a convenient landmark delineating the border between the original tuberculum impar and the copula. Caudal to the copula is another swelling that represents the epiglottis.
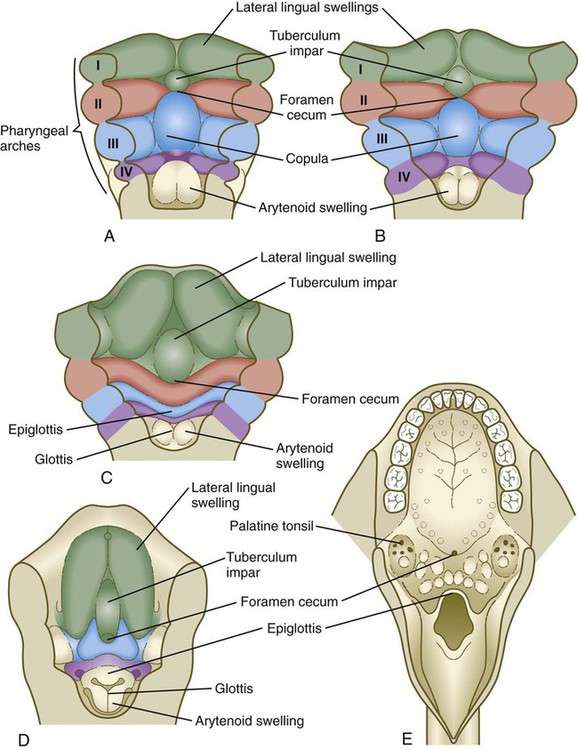
A, At 4 weeks. B, Late in the fifth week. C, Early in the sixth week. D, Middle of the seventh week. E, Adult.
Growth of the body of the tongue is accomplished by a great expansion of the lateral lingual swellings, with a minor contribution by the tuberculum impar (Fig. 14.40C and D). The root of the tongue is derived from the copula, along with additional ventromedial tissue between the third and fourth pharyngeal arches.
Clinical Correlation 14.3 presents anomalies and syndromes involving the pharynx and pharyngeal arches.
Summary
The early craniofacial region arises from the rostral portions of the neural tube, the notochord, and the pharynx, which is surrounded by a series of paired aortic arches. Between the aortic arches and the overlying ectoderm are large masses of neural crest–derived and mesodermally derived mesenchyme. Some of these components show evidence of anatomical segmentation or segmental patterns of gene expression.
Massive migrations of segmental groups of neural crest cells provide the mesenchyme for much of the facial region. The musculature of the craniofacial region is derived from somitomeric mesoderm or the occipital somites. The connective tissue component of the facial musculature is of neural crest origin.
The pharyngeal (branchial) region is organized around paired mesenchymal pharyngeal arches, which alternate with endodermally lined pharyngeal pouches and ectodermally lined pharyngeal grooves.
The face and lower jaw arise from an unpaired frontonasal prominence and paired nasomedial, maxillary, and mandibular processes. Through differential growth and fusion, the nasomedial processes form the upper jaw and lip, and the frontonasal prominence forms the upper part of the face. The expanding mandibular processes merge to form the lower jaw and lip. A nasolacrimal groove between the nasolateral and maxillary processes ultimately becomes canalized to form the nasolacrimal duct, which connects the orbit to the nasal cavity.
The palate arises from the fusion of an unpaired median palatine process and paired lateral palatine processes. The former forms the primary palate, and the latter forms the secondary palate. Fusion of the lateral palatal shelves involves removal of the midline epithelial seam by a combination of apoptosis, migration, and transformation of epithelial cells into mesenchyme.
The olfactory apparatus begins as a pair of thickened ectodermal nasal placodes. As these sink to form nasal pits, they are surrounded by horseshoe-shaped nasomedial and nasolateral processes. The nasomedial processes form the bridge and septum of the nose, and the nasolateral processes form the alae of the nose. The deepening nasal pits break into the oral cavity, and only later are the nasal cavities separated from the oral cavity by the palate.
The salivary glands arise as epithelial outgrowths of the oral epithelium. Through a series of continuing interactions with the surrounding mesenchyme, the expanding glandular epithelium branches and differentiates.
Teeth form from interactions between oral ectoderm (dental lamina) and neural crest mesenchyme. A developing tooth is first a tooth bud, which then passes through a cap and bell stage. Late in the bell stage, ectodermal cells (ameloblasts) of the epithelial enamel organ begin to form enamel, and the neural crest–derived epithelium (odontoblasts) begins to secrete dentin. Precursors of the permanent teeth form dental primordia along the more advanced primary teeth.
Malformations of the face are common. Many, such as cleft lip and cleft palate, represent the persistence of the structural arrangements that are normal for earlier embryonic stages. Others, such as holoprosencephaly and hypertelorism, result from growth disturbances in the frontonasal process. Most facial malformations seem to be multifactorial in origin, involving genetic susceptibility and environmental causes.
Components of the pharynx (pharyngeal grooves, pharyngeal arches, and pharyngeal pouches) give rise to a wide variety of structures. The first arch gives rise to the upper and lower jaws and associated structures. The first groove and pouch, along with associated mesenchyme from the first and second arches, form the many structures of the external and middle ear. The second, third, and fourth pharyngeal grooves become obliterated and form the outer surface of the neck, and the components of the second through fourth arches form the pharyngeal skeleton and much of the musculature and connective tissue of the pharyngeal part of the neck. The endoderm of the third and fourth pouches forms the thymus and parathyroid glands. The thyroid gland arises from an unpaired ventral endodermal outgrowth of the upper pharynx.
The tongue originates from multiple ventral swellings in the floor of the pharynx. The bulk of the tongue comes from the paired lateral lingual swellings in the region of the first pharyngeal arches. The unpaired tuberculum impar and copula also contribute to the formation of the tongue. The tongue musculature, along with the hypoglossal nerve (cranial nerve XII), which supplies the muscles, arises from the occipital somites. General sensory innervation of the tongue (from cranial nerves V, IX, and X) corresponds with the embryological origin of the innervated part of the tongue. Cranial nerves VII and IX innervate the taste buds on the tongue.
Many malformations of the lower face and jaw are related to hypoplasia of the first pharyngeal arches. Cysts, sinuses, and fistulas of the neck are commonly caused by abnormal persistence of pharyngeal grooves or pouches. Ectopic glandular tissue (thyroid, thymus, or parathyroid) is explained by the persistence of tissue rests along the pathway of migration of the glands. Certain syndromes (e.g., DiGeorge’s syndrome), which seemingly affect disparate organs, can be attributed to neural crest defects.