Chapter 35 General Anesthetics
Abbreviations | |
---|---|
CNS | Central nervous system |
ED50 | Median effective dose |
GABA | γ-Aminobutyric acid |
IV | Intravenous |
MAC | Minimum alveolar concentration |
N2O | Nitrous oxide |
NMDA | N-methyl-D-aspartate |
Pco2 | Carbon dioxide tension (partial pressure) |
Therapeutic Overview
The primary therapeutic considerations are summarized in the Therapeutic Overview Box.
Therapeutic Overview |
---|
Requirements of Anesthetic Drugs |
Inhalational |
Chemical stability |
Minimal irritation upon inhaling |
Speed of onset (time to loss of consciousness) |
Ability to produce analgesia, amnesia, and muscle relaxation |
Minimal side effects, especially cardiovascular and respiratory depression and toxicity to the liver |
Speed and safety of emergence |
Minimal metabolism |
Intravenous |
Chemical stability |
No pain at injection site |
Speed of onset |
Minimal side effects |
Ability to produce analgesia, amnesia, and muscle relaxation |
Speed and safety of emergence |
Rapid metabolism or redistribution |
Mechanisms of Action
The molecular basis for the anesthetic action of inhalational agents is poorly understood. Although most inhalational anesthetics contain an ether (-O-) link and a halogen (Fig. 35-1), no obvious structure-activity relationships have been defined, suggesting that they do not exert their effects through specific cell-surface receptors, unlike most other therapeutic agents acting on the central nervous system (CNS).
The potency of an inhalational anesthetic is expressed in terms of the minimum alveolar concentration (MAC), which is a concentration that prevents 50% of patients from responding to a painful stimulus, such as a skin incision. MAC is analogous to the median effective dose (ED50) and is used to express the relative potency of gaseous drugs. Meyer and Overton observed that the potencies of anesthetic agents correlate highly with their lipid solubilities, as measured by the oil:gas partition coefficient (Table 35-1; Fig. 35-2). Indeed, this relationship holds not only for agents in clinical use but also for inert gases that are not used clinically, such as xenon and argon. This correlation has given rise to several theories of anesthetic action, none of which has been substantiated.
Most IV anesthetic agents contain ring structures (Figure 35-3), have well-documented effects at specific cell-surface receptors, and include benzodiazepines, barbiturates, opioids, and several other compounds.
The barbiturate anesthetics include thiopental and methohexital, while the benzodiazepine anesthetics include diazepam and midazolam. These agents act at two distinct recognition sites on the GABAA receptor Cl− channel complex to potentiate GABA-mediated Cl− conductance and neuronal inhibition (see Chapter 31).
Several opioids used for anesthesia include fentanyl and its analogs sufentanil and remifentanil. Although morphine was used for many years, these newer high-potency compounds are gradually replacing it. The depressant action of the opioids on neuronal activity is mediated by the μ opioid receptor and those of mixed-action opioids by μ and κ opioid receptors (see Chapter 36).
Pharmacokinetics
In a mixture of gases, the partial pressure of an anesthetic agent is directly proportional to its fractional concentration in the mixture (Dalton’s Law). Thus, as depicted in Figure 35-4, in a mixture of 70% N2O, 25% O2, and 5% halothane, which might be used during mask induction of anesthesia, the partial pressures of the component gases are 532, 190, and 38 mm Hg, respectively, at 1 atmosphere (760 mm Hg) of pressure.
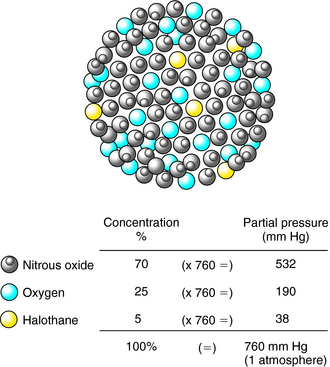
FIGURE 35–4 The partial pressure of a gas in a mixture of gases is directly proportional to its concentration.
The rate of induction of anesthesia by inhalational agents is affected by numerous factors, including those that reduce alveolar ventilation, which represents the product of the rate of respiration and tidal volume less the pulmonary dead space (Table 35-2). Thus if a patient is administered respiratory depressants such as barbiturates or opioid analgesics preoperatively, the rate of respiration or tidal volume decreases, thereby reducing alveolar ventilation in the absence of assisted ventilation. Alveolar dead space is substantial in patients with pulmonary diseases such as emphysema and atelectasis, which result in decreased alveolar ventilation and rate of anesthesia induction.
TABLE 35–2 Factors Affecting the Rate of Induction with an Inhalational Anesthetic
Condition | Rate of Induction |
---|---|
Increased concentration of anesthetic in inspired gas mixture | Increased |
Increased alveolar ventilation | Increased |
Increased solubility of anesthetic in blood (blood:gas partition coefficient) | Decreased |
Increased cardiac output | Decreased |
The path followed by an inhalational anesthetic during induction of, and emergence from, anesthesia is diagrammed in Figure 35-5. Induction is facilitated by factors that maintain a high partial pressure of the anesthetic in the inspired gas mixture, alveolar space, and arterial blood to deliver as much of the gas to the brain as quickly as possible. The alveolar membrane poses no barrier to gases, permitting unhindered diffusion in both directions. Therefore, once the anesthetic gas reaches the alveolar space, it obeys the law of mass action and moves down its partial-pressure gradient into arterial blood. At the initiation of anesthetic administration, the partial pressure of the anesthetic in the alveolar space is much higher than that in blood. Thus the partial pressure gradient between the alveolar space and the arteriolar blood is high, and initially the gas moves rapidly into blood. As the partial pressure of the anesthetic agent in blood increases, the gradient between the alveolar space and blood decreases and uptake slows (Fig. 35-6).
Another important factor in the rate of rise of the arterial partial pressure of an anesthetic gas is its solubility in blood. This relationship is expressed as the blood:gas partition coefficient. The higher the solubility is of an anesthetic gas in blood, the more must be dissolved to produce a change in partial pressure (because partial pressure is inversely proportional to solubility). This relationship is illustrated for N2O and halothane in Figure 35-7.
Nitrous oxide has a blood:gas partition coefficient of 0.47, so relatively little must be dissolved in blood for its partial pressure in blood to rise. This also is true for desflurane and sevoflurane. In contrast, blood serves as a large reservoir for halothane, retaining at equilibrium 2.3 parts for every 1 part in the alveolar space. Induction therefore depends not on dissolving the anesthetic in blood but on raising arterial partial pressure to drive the gas from blood to brain. Therefore the rate of rise of arterial partial pressure and speed of induction are fastest for gases that are least soluble in blood (see Fig. 35-6). The blood:gas partition coefficients of inhalational anesthetics are listed in Table 35-1.
The transfer of an anesthetic from arterial blood to brain depends on factors analogous to those involved in movement of gas from alveoli to arterial blood. These include the partial pressure gradient between blood and brain, the solubility of the anesthetic in brain, and cerebral blood flow. The brain is part of the vessel-rich group of tissues that compose 9% of body mass but receive 75% of cardiac output. The anesthetic uptake curve levels off (see Fig. 35-6), reflecting attainment of equilibrium by the vessel-rich group of tissues. In contrast, the muscle group constitutes 50% of body mass but receives only 18% of cardiac output. Fat represents 19% of body mass and receives 5% of cardiac output, whereas the vessel-poor group including bone and tendon, accounts for 22% of body mass yet receives less than 2% of cardiac output. Thus approximately 41% of total body mass receives a mere 7% of cardiac output. As a consequence, in most surgical procedures, poorly perfused tissues do not contribute meaningfully to the apparent volume of distribution of the inhalational anesthetic, and true total equilibration does not occur. The importance of tissue perfusion as a factor determining the uptake of an anesthetic is illustrated for halothane in Figure 35-8.
When administration of an anesthetic is terminated, the anesthetic gas flows from the venous blood to the alveolar space (see Fig. 35-5). Factors that affect the rate of elimination of an inhalational anesthetic are analogous to those that determine its rate of uptake. Therefore the rate of loss of an anesthetic gas during emergence from anesthesia is directly proportional to its rate of uptake, and emergence is a mirror image of induction.
Although inhaled anesthetics are cleared from the body largely via the lung, most undergo some degree of hepatic metabolism, and several metabolites have been implicated in organ toxicity. The extensive metabolism of methoxyflurane, 50% to 60% of an administered dose, results in the release of fluoride ions, which can reach nephrotoxic concentrations during long surgical procedures; therefore methoxyflurane is no longer used. The extent of biotransformation of other inhalational anesthetics ranges from approximately 15% for halothane to negligible amounts for N2O (see Table 35-1). Inhalational anesthetics that are not appreciably metabolized generally exhibit less-toxic sequelae.
When IV anesthetics are administered, movement of drug from blood to brain determines its onset of action. A good IV anesthetic drug should be effective within one “arm-to-brain blood circulation.” The short-acting compounds propofol and etomidate are the fastest to induce anesthesia, that is, 30 to 50 seconds from injection to loss of the eyelash reflex, or one arm-to-brain circulation. On the other hand, a benzodiazepine requires several minutes to induce a similar response. Because blood flow to the brain is also important, the onset of action may be delayed in a patient with extremely low cardiac output and therefore a relatively low blood flow to the brain.
The duration of effect of a single induction dose of an IV anesthetic is determined by its rate of redistribution or metabolism. Redistribution from the brain into less-well-perfused tissues (i.e., abdominal viscera, skeletal muscle) is the predominant mechanism responsible for termination of action. Redistribution can occur within minutes of induction of anesthesia with a single dose of anesthetic, resulting in recovery of reflex activity and consciousness. The IV induction agents have varying speeds of onset, durations of action, and rates of redistribution. The pharmacokinetic and physicochemical characteristics of the ideal IV anesthetic are listed in Box 35-1.
Thiopental has been used widely for IV induction because of its rapid and smooth onset and its short duration of action. It is highly lipid soluble, rapidly crosses the blood-brain barrier, and is rapidly redistributed from brain to other body tissues. These pharmacokinetic characteristics preclude its use as a maintenance agent for lengthy procedures. However, because of its long terminal elimination half-life (Table 35-3), thiopental accumulates in the body, and its duration of action increases with repeated administration, causing some patients to remain unconscious after surgery is completed. Thiopental is metabolized primarily in the liver to H2O-soluble metabolites that are excreted in the urine. The pharmacokinetic properties of other barbiturates used as IV anesthetics, such as methohexital, are generally similar to those of thiopental.
Induction of anesthesia with the benzodiazepine diazepam is relatively slow, often taking several minutes. It has a long redistribution half-life (30 to 60 minutes), a long duration of action, and a long terminal elimination half-life (see Table 35-3). It is metabolized by the microsomal enzyme system in liver, and most of its metabolites are pharmacologically active and have long half-lives (see Chapter 31). Midazolam is an H2O-soluble benzodiazepine twice as potent as diazepam. It takes midazolam 2 to 3 minutes to induce anesthesia, which is faster than diazepam but slower than thiopental.
Morphine, the prototypical opioid analgesic, is given subcutaneously or intramuscularly in doses of 8 to 15 mg to allay anxiety and ease pain before, during, and after surgery. It is administered IV in substantially higher doses in combination with an inhalational or IV anesthetic for the induction and maintenance of anesthesia, especially for cardiac or other major surgery. Because of its low lipophilicity, morphine crosses the blood-brain barrier slowly, and plasma concentrations may not accurately reflect those in brain. Morphine is metabolized in the liver, primarily to morphine-6-glucuronide, which retains considerable morphine-like activity but has limited access to the CNS.
Remifentanil, the newest potent and ultrashort-acting μ opioid receptor agonist, has a rapid onset of action, is metabolized rapidly by plasma and tissue esterases, and has a terminal elimination half-life of 10 to 15 minutes. It is often one of the components in “balanced-anesthesia,” where it is given as a continuous infusion, titrating to the desired effect. Because remifentanil is so short-acting, patients should be given a longer-acting opioid or other analgesic 10 to 15 minutes before emergence from anesthesia. Other opioids commonly used in anesthesia differ from morphine in their potency, rate of onset, and duration of action but are generally similar in their pharmacological activity (see Table 35-3 and Chapter 36).
The physicochemical properties of some IV anesthetic drugs render them insoluble in H2O at physiological pH, necessitating use of solvents or adjusting the pH of the injectate (see Table 35-3), either of which can lead to problems. The alkaline pH of a 2.5% solution of thiopental makes it unsuitable for mixing with acidic drugs, especially opioids and muscle relaxants. Thiopental solutions also cause tissue damage if injected intraarterially or extravascularly. Acidic etomidate solutions can cause pain and thrombophlebitis after intravascular injection. All alcohol-based solvents and buffers are venous irritants, causing pain when injected IV. Thus a diazepam solution is sometimes mixed with a solution of the local anesthetic lidocaine to make the injection less painful. Midazolam, in contrast, is H2O soluble and poses no special problems for IV administration. Propofol emulsion causes pain on injection, a problem that may be resolved by newer formulations.
Relationship of Mechanisms of Action to Clinical Response
Because doses of inhalational anesthetics are additive, a 0.5 MAC of compound “A” can be combined with a 0.5 MAC of compound “B” to give an inspired-gas mixture that has a MAC of 1.0. For example, 1.0 MAC of halothane is 0.75% (5.7 mm Hg, or 5.7 torr at 1.0 atmosphere of pressure), and 1.0 MAC of isoflurane is 1.15% (8.7 mm Hg). Therefore an inspired gas mixture containing 0.375% halothane and 0.575% isoflurane has a MAC of 1.0.
Pharmacovigilance: Side Effects, Clinical Problems, and Toxicity
Respiratory and Cardiovascular Effects
All inhalational anesthetics reduce spontaneous respiration in a concentration-dependent manner by depressing medullary centers in the brainstem. They decrease the responsiveness of chemoreceptors in respiratory centers to elevations in carbon dioxide tension (Pco2) in blood and cerebrospinal fluid, which normally serve as a potent stimulus for increasing minute ventilation. The result is a shift to the right and flattening of the Pco2 ventilation-response curve (Fig. 35-9). Thus the ventilatory response to hypercapnia is attenuated. Opioids also reduce the responsiveness of brainstem chemoreceptors to elevations in Pco2 and shift the Pco2 ventilation-response curve in a similar manner. When an opioid is given concurrently with an inhalational anesthetic, the effects of the two on respiration are additive and often synergistic, as shown in Figure 35-9. CO2 exerts a local effect on the cerebral vasculature by dilating small vessels. The resultant increase in intracranial pressure is a cause for concern in patients with head trauma.
All inhalational anesthetics depress the force of myocardial contraction in a concentration-dependent manner in isolated heart preparations. In patients, the effects on myocardial function varies depending on the agent, the concentration needed for surgical anesthesia, and the drug’s effects on the sympathetic nervous system. Nitrous oxide has minimal effects on cardiovascular function, whereas halothane significantly depresses most cardiovascular variables. The cardiovascular effects of other anesthetics fall between those of N2O and halothane.
Renal blood flow and glomerular filtration rate are decreased during general anesthesia, resulting in decreased urine formation. Enflurane and sevoflurane undergo some metabolism in the liver and release free fluoride ions, which can be nephrotoxic in sufficiently high concentrations during lengthy surgical procedures. It is best not to use either agent in patients with impaired renal function. Halothane, though metabolized to an appreciable extent (see Table 35-1), does not release significant amounts of free fluoride.
Halogenated inhalational anesthetics, and halothane in particular, can precipitate malignant hyperthermia in genetically susceptible patients. Depolarizing neuromuscular blocking agents, notably succinylcholine (see Chapter 12), can also trigger this reaction, which is manifest as a sustained contraction of the musculature with a dramatic increase in O2 consumption and an increased body temperature. The syndrome results from a failure of the sarcoplasmic reticulum to re-sequester Ca++, preventing the dissociation of actin and myosin filaments of muscle. The resultant hyperthermia is an emergency requiring prompt treatment, including rapid cooling and administration of the skeletal muscle relaxant dantrolene (see Chapter 12). Overall, malignant hyperthermia occurs in 1 in 15,000 to 1 in 50,000 cases. The combined use of halothane and succinylcholine is associated with the highest incidence, and the combined use of a halogenated anesthetic other than halothane and a non-depolarizing muscle relaxant is associated with the lowest incidence.
Central Nervous System Effects
As indicated, N2O lacks sufficient potency to produce surgical levels of anesthesia safely by itself. It can produce analgesia comparable to that produced by a therapeutic dose of morphine at concentrations of 20% and can cause amnesia at concentrations of 60%. At a concentration of 40%, N2O can induce a state of behavioral disinhibition and raucousness in patients not receiving other drugs. It is this action of N2O that has given the agent the name “laughing gas.” Most often, N2O is administered in combination with a halogenated anesthetic to lower the anesthetic requirement for the latter and to promote rapid induction (see Fig. 35-6).
Nitrous oxide diffuses into enclosed air-filled cavities in the body, where it exchanges with nitrogen. Because of a difference in their blood:gas partition coefficients, blood can carry much more N2O than nitrogen. Nitrous oxide diffuses out of blood and into air-filled cavities approximately 35 times faster than nitrogen leaves those cavities and enters the blood. This results in an increase in pressure and distention of enclosed air-filled, nitrogen-containing spaces. This situation might be encountered in patients with an occlusion of the middle ear, pneumothorax, obstructed intestine, or air emboli in the bloodstream, or after a pneumoencephalogram. These conditions, if not absolute contraindications to the use of N2O, are at least signals for caution.
General side effects, clinical problems, and toxicities associated with the use of the benzodiazepines and opioids are presented in Chapter 31 and Chapter 36, respectively.
Postoperative nausea and vomiting are common side effects with opioids. The rapid IV administration of morphine can evoke histamine release from mast cells, which in turn causes arterial and venous dilatation and hypotension. This effect can be prevented by pretreatment with H1 and H2 receptor blockers, such as diphenhydramine and cimetidine, respectively (see Chapter 14).
New Horizons
Efforts to reduce the rising cost of healthcare in the United States may result in 70% to 75% of all surgical procedures being performed in ambulatory surgical facilities. Surgery in hospitals will be reserved for patients requiring the most intensive medical care. This trend has important implications in terms of drug development. Because most surgical patients are discharged within hours of their surgery, the effects of anesthetic drugs have to be dissipated rapidly and completely, enabling the patient to have a clear sensorium and no residual postoperative nausea or impairment of motor function, judgment, or memory. This requires inhalational anesthetic agents that have a fast onset and offset of action like propofol. Therefore IV drugs that are inactivated rapidly by simple mechanisms (such as plasma esterase activity) will be relied on more heavily for general anesthesia, because their effects disappear within moments of terminating drug administration. Newer drugs should possess the characteristics of the ideal agent listed in Box 35-1.
Bovill JG. Inhalation anaesthesia: From diethyl ether to xenon. Handb Exp Pharmacol. 2008;182:121-142.
Campagna JA, Miller KW, Forman A. Mechanisms of actions of inhaled anesthetics. N Engl J Med. 2003;348:2110-2124.
Hendrickx JF, DeWolf A. Special aspects of pharmacokinetics of inhalation anesthesia. Handb Exp Pharmacol. 2008;182:159-186.
Henthorn TK. The effects of altered physiological states on intravenous anesthetics. Handb Exp Pharmacol. 2008;182:363-377.
Olkkola KT, Ahonen J. Midazolam and other benzodiazepines. Handb Exp Pharmacol. 2008;182:335-360.
Vanlersberghe C, Camu F. Propofol. Handb Exp Pharmacol. 2008;182:227-252.