Fetal Period and Birth
After the eighth week of pregnancy, the period of organogenesis (embryonic period) is largely completed, and the fetal period begins. By the end of the embryonic period, almost all the organs are present in a grossly recognizable form. The external contours of the embryo show a very large head in proportion to the rest of the body and greater development of the cranial than of the caudal part of the body (Figs. 18.1 and 18.2).
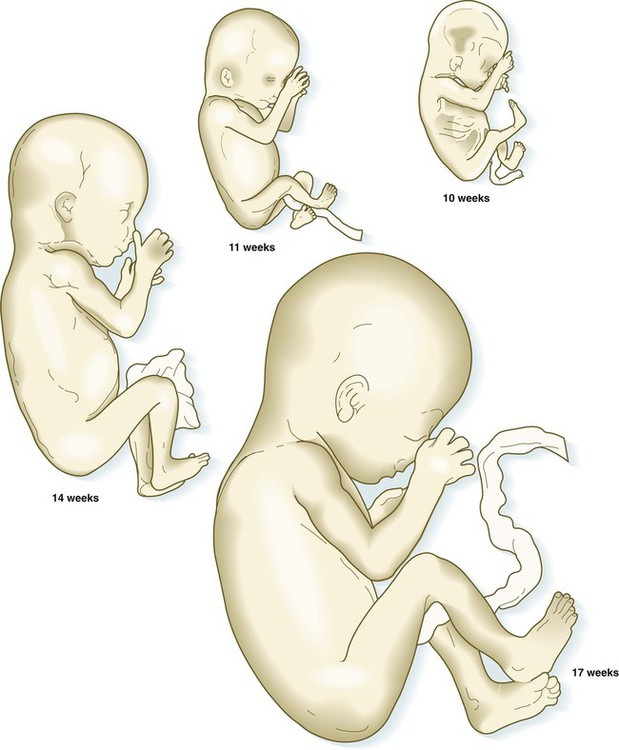
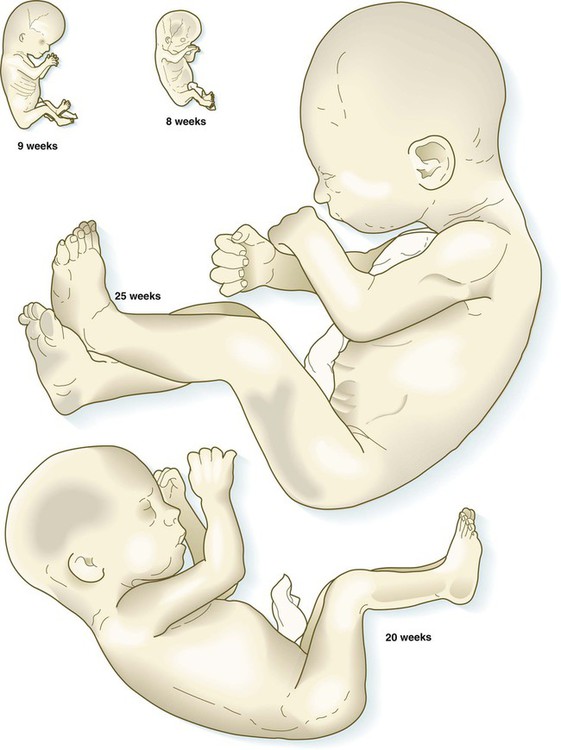
The fetuses from 8 through 17 weeks are drawn actual size. The fetuses from 20 and 25 weeks are drawn two-thirds actual size.
This chapter emphasizes the functional development of the fetus and the adaptations that ensure a smooth transition to independent living after the fetus has passed through the birth canal and the umbilical cord is cut. Techniques that are used to monitor the functional state of the fetus are also described in Clinical Correlation 18.1, later in the chapter.
Growth and Form of the Fetus
Despite the intense developmental activity that occurs during the embryonic period (3 to 8 weeks), the absolute growth of the embryo in length and mass is not great (Fig. 18.3). The fetal period (9 weeks to birth), however, is characterized by rapid growth. The change in proportions of the various regions of the body during the prenatal and postnatal growth periods is as striking as the absolute growth of the embryo. The early dominance of the head is reduced as development of the trunk becomes a major factor in the growth of the early fetus. Even later, a relatively greater growth of the limbs changes the proportions of various regions of the body. During the early fetal period, the entire body is hairless and very thin because of the absence of subcutaneous fat (Fig. 18.4). By midpregnancy, the contours of the head and face approach those of the neonate, and the abdomen begins to fill out. Beginning at around week 27, the deposition of subcutaneous fat causes the body to round out. (Some major developmental landmarks during the fetal period are summarized in the table on pp. xii and xiii.)
Fetal Physiology
Circulation
The circulation of the human embryo can be first studied at about 5 weeks by means of ultrasound. At that time, the heart beats at a rate of approximately 100 beats/minute. This probably represents an inherent atrial rhythm. The pulse rate increases to about 160 beats/minute by 8 weeks and then decreases to 150 beats/minute by 15 weeks, with a further slight decline near term. The pulse rate in utero is remarkably constant, and embryos exhibiting bradycardia (slow pulse rate) often die before term. Near term, the pulse rate varies to some extent if conditions in the uterus change or if the embryo is stressed. This variation is related to the functional establishment of the autonomic innervation of the heart (Fig. 18.5).
Quantitative studies have shown a good correlation between blood flow and functional needs of various regions of the embryo. Approximately 40% of the combined cardiac output goes to the head and upper body and supplies the relatively great needs of the developing brain. Another 30% of the combined cardiac output goes to the placenta via the umbilical arteries for replenishment. Figure 18.6 shows the relative amounts of blood that enter and leave the heart via various vascular channels. (The general qualitative pattern of blood flow in a human fetus is presented in Fig. 17.30.)
Fetal Movements and Sensations
Ultrasonography has revolutionized the analysis of fetal movements and behavior because the fetus can be examined virtually undisturbed (except for an increase in vascular activity induced by the ultrasound) for extended periods. Earlier studies of fetal movements were principally concerned with the development of reflex responses, and the information was obtained largely by the analysis of newly aborted fetuses (see Chapter 11). Although valuable information on maturation of reflex arcs was obtained in this manner, many of the movements elicited were not those normally made by the fetus in utero.
The undisturbed embryo does not show any indication of movement until about weeks. The first spontaneous movements consist of slow flexion and extension of the vertebral column, with the limbs being passively displaced. Within a short time, a large repertoire of fetal movements evolves. After study by numerous investigators, a classification of fetal movements has been suggested (Box 18.1). The first fetal movements are followed in a few days by startle and general movements. Shortly thereafter, isolated limb movements are added (Fig. 18.7). Movements associated with the head and jaw appear later. Toward the end of the fourth month, the fetus begins a pattern of periods of activity, followed by times of inactivity. Many women first become aware of fetal movements at this time. Between the fourth and fifth months, the fetus becomes capable of gripping firmly onto a glass rod. Although weak protorespiratory movements are possible, they cannot be sustained.
Continuous ultrasound monitoring for extended periods reveals patterns involving many types of movements (Fig. 18.8). At different weeks of pregnancy, some movements are predominant, whereas others are in decline or are just beginning to take shape. Analysis of anencephalic fetuses has shown that although many movements occur, they are poorly regulated. These movements start abruptly, are maintained at the same force, and then stop abruptly. These abnormal patterns of movements are considered evidence for strong supraspinal modulation of movement in the fetus.
Fetal Digestive Tract
Individual epithelial cell types, including Brunner’s glands, which protect the duodenal lining from gastric acid, appear in the small intestine early in the second trimester. Although the presence of most enzymes or proenzymes characteristic of the intestinal lining can be shown histochemically during the midfetal period, the amounts of these substances are generally quite small. Activity of some of the enzymes secreted by the exocrine pancreatic tissue can also be shown between 16 and 22 weeks’ gestation. Meconium, a greenish mixture of desquamated intestinal cells, swallowed lanugo hair, and various secretions, begins to fill the lower ileum and colon late in the fourth month (Fig. 18.9).
Fetal Kidney Function
Intrauterine renal function is unnecessary for fetal life because embryos with bilateral renal agenesis survive in utero. Bilateral renal agenesis, however, is commonly associated with oligohydramnios (see Chapter 7), thus indicating that the overall balance of amniotic fluid requires a certain amount of fetal renal function.
Endocrine Function in the Fetus
Among the fetal endocrine glands, the adrenal remains the most enigmatic. By 6 to 8 weeks of development, the inner cortex enlarges greatly to form a distinct fetal zone, which later in pregnancy occupies about 80% of the gland. By the end of pregnancy, the adrenal glands weigh 4 g each, the same mass as that of the adult glands (Fig. 18.10). The fetal adrenal cortex produces 100 to 200 mg of steroids each day, an amount several times higher than that of the adult adrenal glands. The main hormonal products of the fetal adrenal are Δ5-3β-hydroxysteroids, such as dehydroepiandrosterone, which are inactive alone, but are converted to biologically active steroids (e.g., estrogens, especially estrone) by the placenta and liver. The fetal adrenal cortex depends on the presence of pituitary ACTH; in its absence, the fetal adrenal cortex is small. If exogenous ACTH is administered, the fetal adrenal cortex persists after birth.
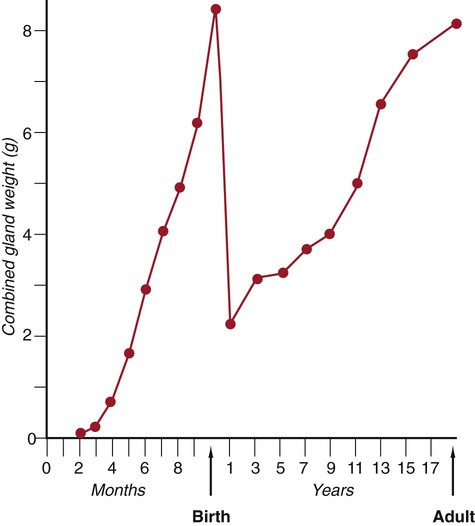
After birth, the weight of the gland decreases dramatically with the reorganization of the cortex of the gland. (Based on data from Neville AM, O’Hare MJ: The human adrenal cortex, Berlin, 1982, Springer.)
Despite the prominence of the fetal adrenal cortex, its specific functions during pregnancy are still unclear. The large fetal zone of the adrenal cortex produces a steroid precursor for estrogen biosynthesis by the placenta. Fetal adrenal hormones influence maturation of the lungs (as prolactin has also been postulated to do), liver, and epithelium of the digestive tract (Box 18.2). In sheep, products of the adrenal cortex prepare the fetus for independent postnatal life and influence the initiation of parturition, but the situation in primates is considerably less clear. Shortly after birth, the fetal adrenal cortex rapidly involutes (see Fig. 18.10). Within 1 month after birth, the weight of each gland is reduced by 50%, and the volume of the fetal cortex decreases from 70% of total adrenal volume to approximately 3%. By 1 year of age, each gland weighs only 1 g. The mass of the adrenal glands does not return to that of the late fetus until adulthood.
One of the earliest placental hormones produced is human chorionic gonadotropin (HCG) (see Chapter 7). One later function of HCG is to stimulate steroidogenesis by the placenta. The synthesis of HCG by the syncytiotrophoblast of the placenta is regulated by the production of gonadotropin-releasing hormone by cells of the cytotrophoblast. Synthesis of this hormone by the placenta supplants its normal production by the hypothalamus and is probably an adaptation that allows earlier and more local control of HCG than could be accomplished by the hypothalamus.
Clinical Correlation 18.1 discusses the clinical study and manipulation of the fetus.
Parturition
Parturition, the process of childbirth, occurs approximately 38 weeks after fertilization (Fig. 18.16). The process of childbirth consists of three distinct stages of labor. The first, the stage of dilation, begins with the onset of regular, hard contractions of the uterus and ends with complete dilation of the cervix. Although the contractions of the uterine smooth muscle may seem to be the dominant process in the first stage of labor, the most important components are the effacement and dilation of the cervix. During the entire pregnancy, the cervix functions to retain the fetus in the uterus. For childbirth to proceed, the cervix must change consistency from a firm, almost tubular structure to one that is soft, distensible, and not canal-like. This change involves a reconfiguration and removal of much of the cervical collagen. Although many of the factors underlying the reconfiguration of the cervix during the first stage of labor remain undefined, considerable evidence exists for an important role of prostaglandin F2α in the process. Although there is great variation, the average length of the first stage of labor is approximately 12 hours.
In humans, there is less dependence on activity of the pituitary-adrenal cortical axis for the initiation of parturition. More recent research on primate embryos has suggested that corticotropin-releasing hormone (CRH), which is normally released by the hypothalamus, is produced in significant amounts by the placenta, starting at about 12 weeks of pregnancy (Fig. 18.17). Some of the placental CRH stimulates the fetal adenohypophysis to release ACTH. ACTH stimulates the adrenal cortex to produce cortisol, which is necessary for many maturation processes in the fetus (see Box 18.2). Much of the CRH acts directly on the fetal adrenal cortex, however, and stimulates it to produce dehydroepiandrosterone sulfate, which the placenta uses directly as a substrate for the synthesis of estrogen.
Adaptations to Postnatal Life
Circulatory Changes at Birth
Because of closure of the ductus arteriosus, increased pulmonary venous flow, and loss of 25% to 50% of the peripheral vasculature (placental circulation) when the umbilical cord is cut, the blood pressure in the left atrium becomes slightly increased over that in the right atrium. This increase leads to physiological closure of the interatrial shunt, with the result that all the blood entering the right atrium empties into the right ventricle (Fig. 18.18). Structural closure of the valve at the foramen ovale is prolonged, occurring over several months after birth. Before complete structural obliteration of the interatrial valve, it possesses the property of “probe patency,” which allows a catheter inserted into the right atrium to pass freely through the foramen ovale into the left atrium. As structural fusion of the valve to the interatrial septum progresses, the property of probe patency is gradually reduced and ultimately disappears. In approximately 20% of individuals, structural closure of the interatrial valve is not completed, thus leading to the normally asymptomatic condition of probe patent foramen ovale.
After the postnatal pattern of the circulation is fully established, obliterated vessels or shunts that were important circulatory channels in the fetus either are replaced by connective tissue strands, forming ligaments, or are represented by relatively smaller vessels (see Fig. 18.18; Fig. 18.19). These changes are summarized in Table 18.1. In early postnatal life, the umbilical vein can still be used for exchange transfusions (in cases of hemolytic disease resulting from erythroblastosis fetalis) before its lumen becomes obliterated.
Table 18.1
Postnatal Derivatives of Prenatal Circulatory Shunts or Vessels
Prenatal Structure | Postnatal Derivative |
Ductus arteriosus | Ligamentum arteriosum |
Ductus venosus | Ligamentum venosum |
Interatrial shunt | Interatrial septum |
Umbilical vein | Ligamentum teres |
Umbilical arteries | Distal segments, lateral umbilical ligaments; proximal segments, superior vesical arteries |
Summary
The fetal period is characterized by intense growth in length and mass of the embryo. With time, the trunk grows relatively faster than the head, and later the limbs show the greatest growth. The early fetus is thin because of the absence of subcutaneous fat. By midpregnancy, subcutaneous fat is deposited.
At 5 weeks’ gestation, the heart beats at 100 beats/minute; the heart rate increases to 160 beats/minute by 8 weeks and then declines slightly during the remainder of pregnancy. Some different physiological properties of the fetal heart can be explained by the presence of fetal isozymes in the cardiac muscle. The patency of the ductus arteriosus in the fetus is actively maintained through the actions of prostaglandin E2.
The fetal lungs are filled with fluid, but they must be prepared for full respiratory function within moments after birth. The fetus begins to make anticipatory breathing movements as early as 11 weeks. Fetal breathing is affected by maternal physiological conditions, such as eating and smoking. Disproportionate growth in diameter of the upper airway is important in allowing a newborn to take the first breath. The secretion of pulmonary surfactant begins at about 24 weeks, but large amounts are not synthesized until just a few weeks before birth. Premature infants with a deficiency of pulmonary surfactant often have respiratory distress syndrome.
Fetal movements begin at about
weeks and increase in complexity thereafter. The maturation of fetal movements mirrors the structural and functional maturation of the nervous system. Diurnal rhythms in fetal activity appear at 20 to 22 weeks. The fetus has alternating periods of sleep and wakefulness. Near term, the fetus responds to vibroacoustic stimuli, and by 30 weeks, the pupillary light reflex can be elicited.
The fetal digestive tract is nonfunctional in the usual sense, but maturation of enzyme systems for digestion and absorption occurs. Spontaneous rhythmical movements of the small intestine begin by 7 weeks’ gestation. Meconium begins to fill the lower intestinal tract by midpregnancy. By term, the fetus typically swallows more than half a liter of amniotic fluid per day.
Fetal kidneys produce small amounts of dilute urine. Fetal endocrine glands produce small amounts of hormones that can be histochemically shown in glandular tissue early in the fetal period, but several months often pass before the same hormones can be measured in the blood. The fetal adrenal cortex is very large and produces 100 to 200 mg of steroids per day. The exact functions of the fetal adrenal gland are poorly understood, but fetal cortisol seems to prepare certain organ systems for the transition to independent life after birth. The placenta continues to produce a variety of hormones throughout most of pregnancy.
Many new diagnostic techniques have considerably improved access to the fetus. Among the imaging techniques, ultrasonography has emerged as the most widely used in obstetrics. Through sampling techniques such as amniocentesis and chorionic villus sampling, fluids or cells of the embryo and fetus can be removed for analysis. These techniques allow certain manipulations on the fetus (e.g., fetal blood transfusions, fetal surgery for certain anomalies).
Parturition occurs in three stages of labor. The first is the stage of dilation, which culminates with effacement of the cervix. The second stage culminates with expulsion of the infant. The third stage represents the period between delivery of the infant and expulsion of the placenta. The mechanisms underlying the initiation of parturition in the human remain poorly understood.
After birth and cutting of the umbilical cord, a newborn must quickly adapt to an independent existence in terms of breathing and cardiac function. After the first breaths and severing of the umbilical cord, the pulmonary circulation opens. In response to increased flow into the left atrium, the interatrial shunt undergoes physiological closure, and the ductus arteriosus undergoes a reflex closure. Closure of the ductus venosus in the liver is more prolonged.