Chapter 6 EEG-Correlated fMRI in Epilepsy
Current State of the Art
Introduction
EEG-correlated fMRI, commonly referred to as EEG-fMRI, is a magnetic resonance imaging (MRI) technique that uses the simultaneously recorded electroencephalogram (EEG) as a marker of brain activity. EEG is a measure of a neuronal activity, whereas fMRI reflects hemodynamic changes, in particular blood oxygen level–dependent (BOLD) signal, which is the main contrast mechanisms exploited by fMRI. Although it is clear that BOLD signal change is indirectly linked to neuronal activity, it is not a clear relationship, and the nature of this “neurovascular coupling” is not fully understood. The study of the hemodynamic correlates of pathological EEG patterns observed in epilepsy by use of EEG-fMRI has developed since the mid-1990s and indeed provided the original impetus for this development. While the initial motivation for combining the two modalities was to overcome some of the deficiencies in each technique, in particular the problem of EEG source localization (the inverse solution) and the low temporal resolution of fMRI, EEG-fMRI uniquely allows the study of the haemodynamic correlates of spontaneous brain activity and in particular individual interictal discharges and seizures.
Interictal Activity in Focal Epilepsy
Using an interictal epileptic discharge (IED) triggered technique, whereby images were only acquired following the observation of an EEG event of interest and compared voxel by voxel to images acquired following periods of background EEG, initial studies in selected patients with focal epilepsy revealed significant BOLD increases in a large proportion of cases that were reproducible,1 although the statistical tests applied varied widely2–8 (see Table 6-1). It should be noted that the early studies focused on the identification of BOLD signal increases, neglecting the possibility of BOLD decreases, which were subsequently shown to be of considerable interest (see discussion later in this chapter).
The continuous EEG-fMRI acquisition approach was made possible by the use of EEG processing methods to correct scanning-related artifact9 (see the section on technical aspects at the end of this chapter for further discussion). The analysis of this type of data requires the creation of a model of the BOLD signal over the entire experiment by identifying images that coincide with EEG events of interest, such as IED.10 This model is then used to find voxels with BOLD signal time courses using correlation. A map of the IED-correlated BOLD signal change is then created across the brain (for examples, see Figures 6-1 and 6-2). The identification of the events of interest is usually made by human observers and suffers from the well-documented limitations of this approach.11 In addition to the limits of an observer-derived EEG model per se, not all patients will have IEDs in the scanner, thus presenting a further difficulty. In the two largest case series of continuously recorded EEG-fMRI in focal epilepsy,12,13 around 50% of patients had IEDs during scanning. Approximately 60% of these had a BOLD signal change recorded in the vicinity of the electroclinical localization of seizure onset (known as concordant BOLD signal change; see Figure 6-1 for illustration). This figure represents the “yield” of EEG-fMRI in a given study. It is improved by modeling runs of IEDs rather than single events in addition to improvement in the EEG model by adequate spike detection and classification by the observer.11,13,14 The patterns of IED-related BOLD signal changes can be complex, with clusters commonly observed in close proximity to or overlapping the presumed seizure onset zone and remote from the epileptogenic zone and irritative zone regions of BOLD decrease most often observed at remote sites. The interpretation of these remote changes remains one of the active areas in EEG-fMRI research.
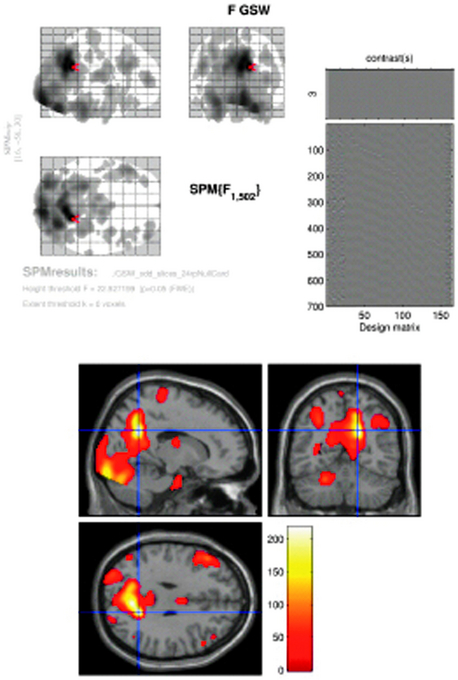
Figure 6–2 SPM{F} from a patient with frequent GSW discharges. Top left: BOLD response correlated with GSW in glass brain display (the red arrow marks the global maxima). Top right: design matrix: first column shows the effect of interest (GSW) convolved with canonical HRF; subsequent columns represent motion and cardiac regressors. At the bottom: BOLD response overlaid onto normalized T1-weighted MRI in MNI space (p < 0.05 corrected). Note bilateral thalamic and right cingulate gyrus activation, right precuneus (global maxima showed by cross hair), bilateral middle frontal gyrus, right occipital lobe (lingual gyrus) deactivation (localization confirmed using Talairach coordinates in Talairach Daemon, http://ric.uthscsa.edu/project/talairachdaemon.html).
Image courtesy of Dr. A. E. Vaudano.
Deviations from the normal time course, however, have been observed in epilepsy. Although the BOLD response, both positive and negative (to external stimuli), has been studied extensively and is well documented in reference to neuronal activity in healthy subjects, some have suggested that this relationship may be altered in the case of interictal epileptiform discharges.16–18 A formal study investigating this possibility found that the inclusion of noncanonical time courses does not lead to an important increase in yield.12 A more recent study suggests noncanonical HRFs associated with IEDs are often remote from the presumed seizure onset zone,19 but whether this represents artifact, propagation of epileptiform activity (time-locked activity) or another phenomenon is not clear. Others have, however, emphasized the importance of intersubject variability in the HRF and routinely use individualized HRFs to analyze IED-correlated fMRI,16,20 and the issue is still under debate. Early BOLD signal change (time locked to IEDs) has been reported in focal epilepsy,21 and BOLD activation has also been reported in the thalamus prior to the onset of generalized spike and wave discharges.22 It is conceivable that these reflect neuronal activity time-locked but preceding an event observed on the scalp EEG and for example may represent a phenomenon similar to observed preictal BOLD signal change in humans25 and preceding the occurrence of epileptic spikes in an animal model.26 Another possibility is that this is a reflection of abnormal neurovascular coupling.
Furthermore the relationship between blood perfusion and BOLD changes linked to epileptiform discharges in focal (one case) and generalized epilepsies are consistent with preservation of neurovascular coupling in epilepsy.23,24
LOCALIZATION OF BOLD CHANGES IN FOCAL EPILEPSY
As has been discussed earlier, IED-correlated BOLD signal change may be colocalized with the irritative or epileptogenic zones.27 We will now discuss the efforts to validate EEG-fMRI localization by comparing other noninvasive localization methods and the gold standard of intracranial recording.
Some reports, predominantly within larger series, of “concordance” of IED-correlated BOLD response with seizure onset were identified by intracranial recording,3,4,12,13 but the only more systematic study was limited to a five-case series. Here it was found that where EEG-fMRI activations were identified, at least one active electrode was on intracranial recording in the same location. The same series made a comparison with source analysis from standard scalp EEG.28
Comparison of noninvasive EEG source localization and EEG-fMRI activations have been made using both spike-triggered fMRI and continuous acquisition. An initial study observed that dipolar sources were often remote (2 to 6 cm) from EEG-fMRI localization.29 This was corroborated by a separate study that suggested distributed source analysis might be a more effective way of comparing EEG source and BOLD activation.30 A recent systematic study using calculated measurements over the cortex revealed IED-associated BOLD clusters that were highly concordant with distributed sources in most patients’ recordings, but also that other EEG-fMRI sources were present that were not concordant with the distributed sources,31 particularly negative BOLD responses. An initial study observed that multi-dipolar sources were generally in anatomical (lobar level) agreement with EEG-fMRI localization based on BOLD increases, and lesser agreement with BOLD decreases.32
Despite comparative studies made between modalities as described earlier, the role of EEG-fMRI in presurgical evaluation remains undefined. The only attempt to assess its practical benefit suggests a limited role in evaluating the group of patients where surgery was not able to be offered.33 Eight patients from this group had significant IED-correlated BOLD signal increase concordant with presumed seizure focus when studied with EEG-fMRI. Of these, four had a unifocal activation, which narrowed the location of the seizure onset zone and was concordant with intracranial recordings in two. In four patients, multifocal BOLD activation concordant with electroclinical evaluation was observed. Larger studies comparing multimodal invasive and noninvasive investigation in addition to postsurgical outcome are required to complete the picture.
BOLD CHANGES ASSOCIATED WITH SPECIFIC PATHOLOGIES IN FOCAL EPILEPSY
It is known in lesional epilepsy that the irritative zone and epileptogenic zone may extend beyond the anatomical boundary of pathological abnormality, and in addition, both in vitro and animal models suggest abnormal subpopulations of neurons within dysplastic areas.34 EEG-fMRI has therefore been used as a tool to evaluate the hemodynamic response in areas of cortical malformation and other pathologies.35–39
In general, EEG-fMRI studies of malformations of cortical development have shown variability in BOLD response within pathologically abnormal regions with broadly concordant activations reported in both gray matter heterotopia35 and focal cortical dysplasia.38 In both these studies, BOLD decreases were observed remote to the area of pathological abnormality. Other studies of malformations of cortical development (MCD) have supported these findings.37 The frequent IED-related BOLD decreases, particularly in MCD, have been attributed to loss of neuronal inhibition (in the presence of normal neurovascular coupling) in the regions surrounding the abnormality or abnormalities in neurovascular coupling itself. The significance of these deactivations will be discussed in more detail later.
A recent study of IED-related BOLD signal change in cavernomas36 suggested BOLD signal change both within the area of anatomical abnormality and within areas remote to it. Caution is required in interpreting BOLD signal change in these very vascular lesions as T2* sequences used in EEG-fMRI are very sensitive to hemosiderin within the lesions.
EEG-fMRI results in tuberous sclerosis reflect a similar pattern. In a recent study exclusively using pediatric patients, it was found that BOLD activation was variable between tubers and extended beyond the border of tubers as identified on structural images once again supporting the view that EEG-fMRI may be a useful technique in assessing the epileptic network beyond areas of structural abnormality.40
EEG-fMRI AND THE PEDIATRIC EPILEPSIES
The pediatric population has been generally less well studied with EEG-fMRI than adults (although some of the studies mentioned earlier have included several children). The experiments are lengthy and can be difficult to tolerate, particularly because they require subjects to remain still for the duration of the procedure. However, intracranial recording is also difficult in children, and invasive procedures such as this may be less acceptable to the pediatric population, meaning that the need for development of noninvasive techniques for localization of the seizure onset zone is even more pressing.41
Two series have used EEG-fMRI in groups of children with focal epilepsies of mixed etiology illustrating that the experiments are tolerated, and results may also show concordance with the seizure onset zone.42,43 In the most recent of these studies, it was found that deactivations are more common and more widespread than in adults, although a common pattern was not identified in this group.44 Questions remain regarding whether this is a function of age and the developing brain, of the particular epilepsy syndrome under study, or whether it in fact is related to pharmacological sedation, the use of which is necessary in children undergoing EEG-fMRI. Previous studies have suggested that benzodiazepine sedation may affect BOLD response to IEDs.45
Specific syndromes are now being studied in children. Two groups of children with benign epilepsy of childhood with centrotemporal spikes (BECCTS) have undergone EEG-fMRI,32,46 and both studies reported BOLD signal change concordant with the electroclinical localization in addition to the tuberous sclerosis study mentioned earlier. A recent study of children with generalized spike-and-wave activity is discussed later.22
ICTAL STUDIES IN FOCAL EPILEPSY
Clinical seizure onset has been used as an event marker in fMRI studies without the use of EEG,25,47,48 which has revealed concordance of seizure-related fMRI activation with electroclinical localization in some cases.
In the few ictal EEG-fMRI studies in focal epilepsy, activations have been more widespread than those observed interictally, usually extending beyond the seizure onset zone. A large increase in BOLD signal was observed, time-locked to a focal subclinical seizure in electroclinically concordant gray matter structures.49 In a patient with multiple simple partial seizures, BOLD activation was revealed, concordant with the electroclinically determined seizure focus. In addition, widespread deactivation was observed on the contralateral side and in other areas of the brain, and increases in the area of activation with cumulative numbers of seizures were analyzed.50
One benefit of recording seizures has been the opportunity to observe preictal changes in BOLD signal. PET and SPECT data suggest preictal changes in cerebral metabolism, and studies of cerebral blood flow support this.51 An fMRI study without simultaneous EEG in three patients suggested BOLD signal change may occur up to minutes prior to the electroclinical onset of seizures,25 a phenomenon that requires further evaluation.
EEG-fMRI AS A TOOL TO STUDY THE NEUROBIOLOGY OF EPILEPSY
In focal epilepsy, initial investigations have focused on temporal lobe epilepsy, the most uniform and best-studied syndrome. An initial series of 19 patients with temporal lobe epilepsy showed the majority had BOLD signal change in the affected temporal lobe, although the activations were usually neocortical, and deactivations often occurred in extratemporal regions.52
Given the observation that the remote deactivations occur in temporal lobe epilepsy, a further study in a group of patients with temporal lobe epilepsy showed that deactivation of the precuneus is common and associated with activation in the ipsilateral hippocampus in relation to interictal temporal lobe spikes.53 This effect was not observed in a similarly selected extratemporal lobe epilepsy group and was thought to reflect a suspension of the precuneus (a region commonly activated in an awake resting state in contrast to reduced conscious or task states54 (and correlated with alpha activity on the EEG55), specifically in response to temporal lobe spikes. The authors pointed out that this may reflect a subclinical suspension of the awake resting state analogous to the cortical deactivation/thalamic activation, which occurs in response to generalized spike-and-wave (GSW) discharge. See discussion later in the chapter.
Methods to assess the functional and structural connectivity between activated regions, such as dynamic causal modeling (which assesses causality in functional connectivity or effective connectivity)56 and diffusion tensor imaging have recently been combined to investigate putative propagation of epileptiform activity,24 and the continued evaluation of connectivity in the epileptic network is an exciting area of evolving research.
Beyond the BOLD changes related to epilepsy-specific stereotypical discharges, such as focal interictal spikes, there had been limited study of other electrophysiological abnormalities. Inspired by methods employed in the study of the hemodynamic correlates of normal brain rhythms using EEG-fMRI,55,57,58 EEG frequency-band-based approaches have been used to study patients with epilepsy, but these studies are restricted to case reports at present.37,59 In the first of these, delta power associated EEG-fMRI was correlated with the seizure onset zone, confirmed by intracranial recording.
EVOLVING ANALYSIS METHODOLOGY
As we have seen, significant BOLD changes are not revealed in an important proportion of cases in which pathological EEG activity is observed during scanning. Methods designed to improve the sensitivity and specificity of EEG-fMRI include the use of automated approaches to spike detection and classification14 and most recently a more symmetric fusion of EEG and fMRI.60
A major limitation of EEG-fMRI is the failure of the technique to register EEG abnormalities in a large proportion of cases studied, depending on the selection criteria. In such cases, the correlation approach cannot be used. This has led to the exploration of data-driven (i.e., without reference to any other data) fMRI analysis in an attempt to identify patterns of BOLD signal specifically linked to the IZ and EZ, such as temporal cluster analysis (TCA)61,62 and independent component analysis (ICA).63
Generalized Epilepsies
In generalized epilepsy syndromes, the aim of EEG-fMRI studies has been mainly an improved understanding of the neurobiology. One of the earliest observations was of four absence seizures, which were found to be associated with a striking pattern of widespread cortical BOLD decrease and thalamic BOLD increase using continuous EEG-fMRI. This was consistent with a reduction of cortical activity during GSW and a key role for the thalamus,64 although there were case reports of EEG-fMRI in generalized epilepsy before this.65 Archer et al. revealed a pattern of BOLD decrease in the posterior cingulate and bilateral precentral BOLD increase in relation to brief epochs of GSW in five patients using triggered EEG-fMRI.66 Continuous EEG-fMRI in larger series of patients revealed a varied pattern of GSW-related cortical BOLD changes dominated by decreases and changes in the thalamus dominated by increases67–69 (Figure 6-2 illustrates this finding). By grouping the results for cases with IGE on one hand and secondarily generalized epilepsy on the other, Hamandi et al. were able to demonstrate that GSW is commonly associated with BOLD decreases in the precuneus/posterior cingulate and bilateral BOLD increases in the thalamus, and that there is considerable overlap between the patterns in the two groups. Interestingly, similar thalamic activations were observed in 55% of a group of patients with focal epilepsy with bilateral spikes, compared to just 12.5% in a group with unilateral spikes.70
It was proposed that involvement of the precuneus reflects a subclinical manifestation of the suspension of consciousness observed in association with generalized seizures, in particular absence seizures, due to that region’s presumed role in consciousness54,71 It is important to note that the type of analysis employed in these studies, based on correlation, do not allow to infer causal links72–74
Recently, it was shown that thalamic hemodynamic changes can systematically precede the onset of GSW by as much as 6 seconds in some children, which may reflect the key role of the thalamus in the generation of generalized discharges.22
Technical Aspects
Despite the development of a dynamic commercial market for MR-compatible EEG recording equipment, EEG-fMRI experiments remain a challenge, and the hardware and software technology necessary for the acquisition of good-quality EEG-fMRI data for application in epilepsy and increasingly in other areas of neuroscience research remains an area of active development. For this reason, the main technological issues related to the acquisition and postprocessing of EEG-fMRI data for subsequent analysis and interpretation are discussed in the following section.
SAFETY
The main safety concern in carrying out simultaneous EEG-fMRI experiments is the risk of heating of the EEG components and of induced currents due to the effect of RF and to a lesser extent gradient switching. To minimize these risks, methods such as the inclusion of current limiting resistors (e.g., 10 kΩ inserted at each electrode for a 1.5 T scanner in one study) and the twisting together of EEG leads to minimize large loops being formed within the scanner have been recommended.75 Commercial MR-compatible EEG systems are now available that incorporate these features into their design. A crucial consideration when placing wires in contact with the body is the type of RF transmit coil used and the length of wire exposed to the electrical component of the field. The important point is that EEG-fMRI should be limited to head-only RF transmit coils, given the current state of technology and in the absence of further studies addressing this problem.76
DATA QUALITY
If EEG is acquired by standard methods in the MRI scanner, in the majority of cases the signal becomes uninterpretable during image acquisition due to the presence of repetitive artifact waveforms superimposed on the physiological signal due to the switching of gradients during EPI sequence acquisition2,9 (Figure 6-3). The first attempts at recording EEG inside MR scanners revealed the presence of significant pulse artifacts (often referred to as the BCG [ballistocardiogram] artifact).77 This effect has been shown to be common across subjects.78 The pulse artifact amplitude can reach 50 μV (at 1.5T) and may resemble epileptic spikes introducing an obvious complication in the study of epilepsy. The precise mechanism through which the circulatory system exposed to a strong magnetic field gives rise to these artifacts remains uncertain, but it is thought to represent a combination of the motion of the electrodes and leads (induction) and the Hall effect (voltage induced by flow of conducting blood in proximity of electrodes).79 This effect is proportional to the scanner’s main field strength.
In addition to artifacts on EEG, interaction between EEG and MRI systems results in artifacts caused by electrodes and leads on the images acquired,77 and this has affected the choice of EEG component materials.5,80,81 Radio-frequency fields radiating from the EEG recording equipment placed in the vicinity of the scanner can cause severe image degradation and may therefore require shielding.
STRATEGIES FOR THE REDUCTION AND CORRECTION OF ARTIFACTS ON THE EEG
Reduction and Correction of the Image Acquisition Artifact
Filtering,84,85 template subtraction, and/or ICA/PCA86 methods have all been used to remove the image artifact following acquisition of the EEG. Simple filtering led to some improvement in image quality, but template subtraction has been shown to be much more effective,87 and this method is used most widely at present (including its incorporation into commercially available MRI-compatible EEG systems). Postprocessing methods to reduce image acquisition artifacts can be categorized as filtering, template subtraction methods, or PCA/ICA.
The most commonly used image acquisition EEG artifact reduction method is based on average template artifact subtraction (AAS) method.9 It enables the artifact to be separated from physiological signal by averaging the EEG over repeated epochs (based on the fMRI scanning rate) and therefore relies on accurate knowledge of the timing of the scanner signal (for instance, by synchronizing scanner and EEG clocks). Subsequent subtraction from the ongoing EEG depends critically on the sampling rate, the number of averaging epochs, and the precision of their timing.
Numerous refinements of the AAS method have been proposed, notably to deal with the residual artifact when averaging of signal is suboptimal due to subject movement changing the artifact.88,89 Possibly the most important practical development has been the demonstration that synchronized MR acquisition and EEG digitization led to significantly improved EEG quality, and in particular over a wider frequency range, when combined with an AAS-like method.90
Reduction and Correction of the Pulse Artifact
The standard artifact reduction algorithm is based on subtraction of a running average estimate of the artifact based on QRS complex detection, in principle similar to the method used to reduce imaging artifact.78
Spatial EEG filtering methods have been proposed as an alternative means of correction based on temporal principal components analysis (PCA) or independent components analysis (ICA).86,87,91–95
1. Symms MR, Allen PJ, Woermann FG, et al. Reproducible localization of interictal epileptiform discharges using EEG-triggered fMRI. Phys Med Biol. 1999;44:N161-N168.
2. Warach S, Ives JR, Schlaug G, et al. EEG-triggered echo-planar functional MRI in epilepsy. Neurology. 1999;47:89-93.
3. Seeck M, Lazeyras F, Michel CM, et al. Non-invasive epileptic focus localization using EEG-triggered functional MRI and electromagnetic tomography. Electroencephalography and Clin Neurophysiol. 1998;106:508-512.
4. Lazeyras F, Blanke O, Perrig S, et al. EEG-triggered functional MRI in patients with pharmacoresistant epilepsy. J Magn Reson Imaging. 2000;12:177-185.
5. Krakow K, Woermann FG, Symms MR, et al. EEG-triggered functional MRI of interictal epileptiform activity in patients with partial seizures. Brain. 1999;122(Pt 9):1679-1688.
6. Patel MR, Blum A, Pearlman JD, et al. Echo-planar functional MR imaging of epilepsy with concurrent EEG monitoring. Am J Neuroradiol. 1999;20:1916-1919.
7. Jäger L, Werhahn KJ, Hoffmann A, et al. Focal epileptiform activity in the brain: detection with spike-related functional MR imaging–preliminary results. Radiology. 2002;223:860-869.
8. Krakow K, Lemieux L, Messina D, et al. Spatio-temporal imaging of focal interictal epileptiform activity using EEG-triggered functional MRI. Epileptic Disord. 2001;3:67-74.
9. Allen PJ, Josephs O, Turner R. A method for removing imaging artifact from continuous EEG recorded during functional MRI. Neuroimage. 2000;12:230-239.
10. Lemieux L, Salek-Haddadi A, Josephs O, et al. Event-related fMRI with simultaneous and continuous EEG: description of the method and initial case report. Neuroimage. 2001;14:780-787.
11. Salek-Haddadi A, Lemieux L, Merschhemke M, Diehl B, Allen PJ, Fish DR. EEG quality during simultaneous functional MRI of interictal epileptiform discharges. Magn Reson Imaging. 2003;21:1159-1166.
12. Salek-Haddadi A, Diehl B, Hamandi K, et al. Hemodynamic correlates of epileptiform discharges: an EEG-fMRI study of 63 patients with focal epilepsy. Brain Res. 2006;1088:148-166.
13. Al Asmi A, Bénar CG, Gross DW, et al. fMRI activation in continuous and spike-triggered EEG-fMRI studies of epileptic spikes. Epilepsia. 2003;44:1328-1339.
14. Liston AD, De Munck JC, Hamandi K, et al. Analysis of EEG-fMRI data in focal epilepsy based on automated spike classification and signal space projection. Neuroimage. 2006;31:1015-1024.
15. Friston KJ, Frith CD, Frackowiak RSJ, Turner R. Characterizing dynamic brain responses with fMRI: a multivariate approach. Neuroimage. 1995;2:166-172.
16. Bénar CG, Gross DW, Wang Y, et al. The BOLD response to interictal epileptiform discharges. Neuroimage. 2002;17:1182-1192.
17. Salek-Haddadi A, Friston KJ, Lemieux L, Fish DR. Studying spontaneous EEG activity with fMRI. Brain Res Brain Res Rev. 2003;43:110-133.
18. Gotman J, Kobayashi E, Bagshaw AP, Benar CG, Dubeau F. Combining EEG and fMRI: a multimodal tool for epilepsy research. J Magn Reson Imaging. 2006;23:906-920.
19. Lemieux L, Laufs H, Carmichael D, Paul JS, Walker MC, Duncan JS. Noncanonical spike-related BOLD responses in focal epilepsy. Hum Brain Mapp. 2008;29(3):329-345.
20. Kang JK, Benar CG, Al Asmi A, et al. Using patient-specific hemodynamic response functions in combined EEG-fMRI studies in epilepsy. Neuroimage. 2003;20:1162-1170.
21. Hawco CS, Bagshaw AP, Lu Y, Dubeau F, Gotman J. BOLD changes occur prior to epileptic spikes seen on scalp EEG. Neuroimage. 2007;35:1450-1458.
22. Moeller F, Siebner HR, Wolff S, et al. Changes in activity of striato-thalamo-cortical network precede generalized spike wave discharges. Neuroimage. 2008;39(4):1839-1849.
23. Stefanovic B, Warnking JM, Kobayashi E, et al. Hemodynamic and metabolic responses to activation, deactivation and epileptic discharges. Neuroimage. 2005;28:205-215.
24. Hamandi K, Laufs H, Noth U, Carmichael DW, Duncan JS, Lemieux L. BOLD and perfusion changes during epileptic generalised spike wave activity. Neuroimage. 2008;39:608-618.
25. Federico P, Abbott DF, Briellmann RS, Harvey AS, Jackson GD. Functional MRI of the pre-ictal state. Brain. 2005;128:1811-1817.
26. Mäkiranta M, Ruohonen J, Suominen K, et al. BOLD signal increase precedes EEG spike activity—a dynamic penicillin induced focal epilepsy in deep anesthesia. Neuroimage. 2005;27:715-724.
27. Rosenow F, Lüders H. Presurgical evaluation of epilepsy. Brain. 2001;124:1683-1700.
28. Benar CG, Grova C, Kobayashi E, et al. EEG-fMRI of epileptic spikes: concordance with EEG source localization and intracranial EEG. Neuroimage. 2006;30:1161-1170.
29. Lemieux L, Krakow K, Fish DR. Comparison of spike-triggered functional MRI BOLD activation and EEG dipole model localization. Neuroimage. 2001;14:1097-1104.
30. Bagshaw AP, Kobayashi E, Dubeau F, Pike GB, Gotman J. Correspondence between EEG-fMRI and EEG dipole localisation of interictal discharges in focal epilepsy. Neuroimage. 2006;30:417-425.
31. Grova C, Daunizeau J, Kobayashi E, et al. Concordance between distributed EEG source localization and simultaneous EEG-fMRI studies of epileptic spikes. Neuroimage. 2008;39:755-774.
32. Boor R, Jacobs J, Hinzmann A, et al. Combined spike-related functional MRI and multiple source analysis in the non-invasive spike localization of benign rolandic epilepsy. Clin Neurophysiol. 2007;118:901-909.
33. Zijlmans M, Huiskamp G, Hersevoort M, Seppenwoolde JH, van Huffelen AC, Leijten FS. EEG-fMRI in the preoperative work-up for epilepsy surgery. Brain. 2007;130(Pt 9):2343-2353.
34. Najm IM, Tilelli CQ, Oghlakian R. Pathophysiological mechanisms of focal cortical dysplasia: a critical review of human tissue studies and animal models. Epilepsia. 2007;48(Suppl 2):21-32.
35. Kobayashi E, Bagshaw AP, Grova C, Gotman J, Dubeau F. Grey matter heterotopia: what EEG-fMRI can tell us about epileptogenicity of neuronal migration disorders. Brain. 2006;129:366-374.
36. Kobayashi E, Bagshaw AP, Gotman J, Dubeau F. Metabolic correlates of epileptic spikes in cerebral cavernous angiomas. Epilepsy Res. 2007;73:98-103.
37. Diehl B, Salek-Haddadi A, Fish DR, Lemieux L. Mapping of spikes, slow waves, and motor tasks in a patient with malformation of cortical development using simultaneous EEG and fMRI. Magn Reson Imaging. 2003;21:1167-1173.
38. Federico P, Archer JS, Abbott DF, Jackson GD. Cortical/subcortical BOLD changes associated with epileptic discharges: an EEG-fMRI study at 3 T. Neurology. 2005;64:1125-1130.
39. Salek-Haddadi A, Lemieux L, Fish DR. Role of functional magnetic resonance imaging in the evaluation of patients with malformations caused by cortical development. Neurosurg Clin N Am. 2002;13:63-69.
40. Jacobs J, Rohr A, Moeller F, et al. Evaluation of epileptogenic networks in children with tuberous sclerosis complex using EEG-fMRI. Epilepsia. 2008;49(5):816-825.
41. Cross JH. Epilepsy surgery in childhood. Epilepsia. 2002;43(Suppl 3):65-70.
42. De Tiege X, Laufs H, Boyd SG, et al. EEG-fMRI in children with pharmacoresistant focal epilepsy. Epilepsia. 2007;48:385-389.
43. Jacobs J, Jacobs J, Boor R, et al. Localization of epileptic foci in children with focal epilepsies using 3-tesla simultaneous EEG-fMRI recordings. Clin Neurophysiol. 2007;118:e50-e51.
44. Jacobs J, Kobayashi E, Boor R, et al. Hemodynamic responses to interictal epileptiform discharges in children with symptomatic epilepsy. Epilepsia. 2007;48:2068-2078.
45. Ricci GB, De Carli D, Colonnese C, et al. Hemodynamic response (BOLD/fMRI) in focal epilepsy with reference to benzodiazepine effect. Magn Reson Imaging. 2004;22:1487-1492.
46. Lengler U, Kafadar I, Neubauer BA, Krakow K. FMRI correlates of interictal epileptic activity in patients with idiopathic benign focal epilepsy of childhood: a simultaneous EEG-functional MRI study. Clin Neurophysiol. 2007;118:e70.
47. Jackson GD, Opdam HI. Ictal fMRI: methods and models. Adv Neurol. 2000;83:203-211.
48. Detre JA, Sirven JI, Alsop DC, O’Connor MJ, French JA. Localization of subclinical ictal activity by functional magnetic resonance imaging: correlation with invasive monitoring. Ann Neurol. 1995;38:618-624.
49. Salek-Haddadi A, Merschhemke M, Lemieux L, Fish DR. Simultaneous EEG-correlated ictal fMRI. Neuroimage. 2002;16:32-40.
50. Kobayashi E, Hawco CS, Grova C, Dubeau F, Gotman J. Widespread and intense BOLD changes during brief focal electrographic seizures. Neurology. 2006;66:1049-1055.
51. Baumgartner C, Serles W, Leutmezer F, et al. Preictal SPECT in temporal lobe epilepsy: regional cerebral blood flow is increased prior to electroencephalography-seizure onset. J Nucl Med. 1998;39:978-982.
52. Kobayashi E, Bagshaw AP, Benar CG, et al. Temporal and extratemporal BOLD responses to temporal lobe interictal spikes. Epilepsia. 2006;47:343-354.
53. Laufs H, Hamandi K, Salek-Haddadi A, Kleinschmidt AK, Duncan JS, Lemieux L. Temporal lobe interictal epileptic discharges affect cerebral activity in “default mode” brain regions. Hum Brain Mapp. 2007;28(10):1023-1032.
54. Raichle ME, MacLeod AM, Snyder AZ, Powers WJ, Gusnard DA, Shulman GL. A default mode of brain function. PNAS. 2001;98:676-682.
55. Laufs H, Kleinschmidt A, Beyerle A, et al. EEG-correlated fMRI of human alpha activity. Neuroimage. 2003;19:1463-1476.
56. Friston KJ, Harrison L, Penny W. Dynamic causal modelling. Neuroimage. 2003;19:1273-1302.
57. Laufs H, Krakow K, Sterzer P, et al. Electroencephalographic signatures of attentional and cognitive default modes in spontaneous brain activity fluctuations at rest. Proc Natl Acad Sci U.S.A. 2003;100:11053-11058.
58. Mantini D, Perrucci MG, Del Gratta C, Romani GL, Corbetta M. Electrophysiological signatures of resting state networks in the human brain. PNAS. 2007;104:13170-13175.
59. Laufs H, Hamandi K, Walker MC, et al. EEG-fMRI mapping of asymmetrical delta activity in a patient with refractory epilepsy is concordant with the epileptogenic region determined by intracranial EEG. Magn Reson Imaging. 2006;24:367-371.
60. Daunizeau J, Grova C, Marrelec G, et al. Symmetrical event-related EEG/fMRI information fusion in a variational Bayesian framework. Neuroimage. 2007;36:69-87.
61. Morgan VL, Gore JC, Abou-Khalil B. Cluster analysis detection of functional MRI activity in temporal lobe epilepsy. Epilepsy Res. 2007;76:22-33.
62. Hamandi K, Salek Haddadi A, Liston A, Laufs H, Fish DR, Lemieux L. fMRI temporal clustering analysis in patients with frequent interictal epileptiform discharges: comparison with EEG-driven analysis. Neuroimage. 2005;26:309-316.
63. Rodionov R, De Martino F, Laufs H, et al. Independent component analysis of interictal fMRI in focal epilepsy: comparison with general linear model-based EEG-correlated fMRI. Neuroimage. 2007;38:488-500.
64. Salek-Haddadi A, Lemieux L, Merschhemke M, Friston KJ, Duncan JS, Fish DR. Functional magnetic resonance imaging of human absence seizures. Ann Neurol. 2003;53:663-667.
65. Baudewig J, Bittermann HJ, Paulus W, Frahm J. Simultaneous EEG and functional MRI of epileptic activity: a case report. Clin Neurophysiol. 2001;112:1196-1200.
66. Archer JS, Abbott DF, Waites AB, Jackson GD. fMRI “deactivation” of the posterior cingulate during generalized spike and wave. Neuroimage. 2003;20:1915-1922.
67. Aghakhani Y, Bagshaw AP, Benar CG, et al. fMRI activation during spike and wave discharges in idiopathic generalized epilepsy. Brain. 2004;127:1127-1144.
68. Gotman J, Grova C, Bagshaw A, Kobayashi E, Aghakhani Y, Dubeau F. Generalized epileptic discharges show thalamocortical activation and suspension of the default state of the brain. PNAS. 2005;102:15236-15240.
69. Hamandi K, Salek-Haddadi A, Laufs H, et al. EEG-fMRI of idiopathic and secondarily generalized epilepsies. Neuroimage. 2006;31:1700-1710.
70. Aghakhani Y, Kobayashi E, Bagshaw AP, et al. Cortical and thalamic fMRI responses in partial epilepsy with focal and bilateral synchronous spikes. Clin Neurophysiol. 2006;117:177-191.
71. Cavanna AE, Trimble MR. The precuneus: a review of its functional anatomy and behavioural correlates. Brain. 2006;129:564-583.
72. Meeren HKM, Pijn JP, Van Luijtelaar ELJM, Coenen AML, Lopes da Silva FH. Cortical focus drives widespread corticothalamic networks during spontaneous absence seizures in rats. J Neurosci. 2002;22:1480-1495.
73. Steriade M, Dossi RC, Nunez A. Network modulation of a slow intrinsic oscillation of cat thalamocortical neurons implicated in sleep delta waves: cortically induced synchronization and brainstem cholinergic suppression. J Neurosci. 1991;11:3200-3217.
74. Avoli M, Gloor P. Interaction of cortex and thalamus in spike and wave discharges of feline generalized penicillin epilepsy. Exp Neurol. 1982;76:196-217.
75. Lemieux L, Allen PJ, Franconi F, Symms MR, Fish DR. Recording of EEG during fMRI experiments: patient safety. Magn Reson Med. 1997;38:943-952.
76. Konings MK, Bartels LW, Smits HF, Bakker CJ. Heating around intravascular guidewires by resonating RF waves. J Magn Reson Imaging. 2000;12:79-85.
77. Ives JR, Warach S, Schmitt F, Edelman RR, Schomer DL. Monitoring the patient’s EEG during echo planar MRI. Electroencephalogr Clin Neurophysiol. 1993;87:417-420.
78. Allen PJ, Polizzi G, Krakow K, Fish DR, Lemieux L. Identification of EEG events in the MR scanner: the problem of pulse artifact and a method for its subtraction. Neuroimage. 1998;8:229-239.
79. Wendt REIII, Rokey R, Vick GWIII, Johnston DL. Electrocardiographic gating and monitoring in NMR imaging. Magn Reson Imaging. 1988;6:89-95.
80. Bonmassar G, Schwartz DP, Liu AK, Kwong KK, Dale AM, Belliveau JW. Spatiotemporal brain imaging of visual-evoked activity using interleaved EEG and fMRI recordings. Neuroimage. 2001;13:1035-1043.
81. Bonmassar G, Purdon PL, Jääskeläinen IP, et al. Motion and ballistocardiogram artifact removal for interleaved recording of EEG and EPs during MRI. Neuroimage. 2002;16:1127-1141.
82. Goldman RI, Stern JM, Engel JJr, Cohen MS. Acquiring simultaneous EEG and functional MRI. Clin Neurophysiol. 2000;111:1974-1980.
83. Anami K, Mori T, Tanaka F, et al. Stepping stone sampling for retrieving artifact-free electroencephalogram during functional magnetic resonance imaging. Neuroimage. 2003;19:281-295.
84. Hoffmann A, Jäger L, Werhahn KJ, Jaschke M, Noachtar S, Reiser M. Electroencephalography during functional echo-planar imaging: detection of epileptic spikes using post-processing methods. Magn Reson Med. 2000;44:791-798.
85. Sijbers J, Michiels I, Verhoye M, Van Audekerke J, Van der Linden A, Van Dyck D. Restoration of MR-induced artifacts in simultaneously recorded MR/EEG data. Magn Reson Imaging. 1999;17:1383-1391.
86. Mantini D, Perrucci MG, Cugini S, Ferretti A, Romani GL, Del Gratta C. Complete artifact removal for EEG recorded during continuous fMRI using independent component analysis. Neuroimage. 2007;34:598-607.
87. Bénar CG, Aghakhani Y, Wang Y, et al. Quality of EEG in simultaneous EEG-fMRI for epilepsy. Clin Neurophysiol. 2003;114:569-580.
88. Negishi M, Abildgaard M, Nixon T, Constable RT. Removal of time-varying gradient artifacts from EEG data acquired during continuous fMRI. Clin Neurophysiol. 2004;115:2181-2192.
89. Niazy RK, Beckmann CF, Iannetti GD, Brady JM, Smith SM. Removal of fMRI environment artifacts from EEG data using optimal basis sets. Neuroimage. 2005;28:720-737.
90. Mandelkow H, Halder P, Boesiger P, Brandeis D. Synchronization facilitates removal of MRI artifacts from concurrent EEG recordings and increases usable bandwidth. Neuroimage. 2006;32:1120-1126.
91. Scarff CJ, Reynolds A, Goodyear BG, Ponton CW, Dort JC, Eggermont JJ. Simultaneous 3-T fMRI and high-density recording of human auditory evoked potentials. Neuroimage. 2004;23:1129-1142.
92. Otzenberger H, Gounot D, Foucher JR. Optimisation of a post-processing method to remove the pulse artifact from EEG data recorded during fMRI: an application to P300 recordings during e-fMRI. Neurosci Res. 2007;57:230-239.
93. Otzenberger H, Gounot D, Foucher JR. P300 recordings during event-related fMRI: a feasibility study. Brain Res Cogn Brain Res. 2005;23:306-315.
94. Briselli E, Garreffa G, Bianchi L, et al. An independent component analysis-based approach on ballistocardiogram artifact removing. Magn Reson Imaging. 2006;24:393-400.
95. Srivastava G, Crottaz-Herbette S, Lau KM, Glover GH, Menon V. ICA-based procedures for removing ballistocardiogram artifacts from EEG data acquired in the MRI scanner. Neuroimage. 2005;24:50-60.