Chapter 17 Disturbances of Smell and Taste
The senses of smell and taste play important roles in human safety, nutrition, and quality of life. In a study of 750 consecutive patients presenting to our center with chemosensory complaints, 68% reported altered quality of life, 46% reported changes in appetite or body weight, and 56% described adverse influences on daily living or psychological well-being (Deems et al., 1991a). In another study of 445 such patients, at least one hazardous event, such as food poisoning or failure to detect fire or leaking natural gas, was reported by 45.2% of those with anosmia, 34.1% of those with severe hyposmia, 32.8% of those with moderate hyposmia, 24.2% of those with mild hyposmia, and 19.0% of those with normal olfactory function (Santos et al., 2004). In a longitudinal study of 1162 older persons without dementia, mortality risk was 36% higher in those with low compared to high scores on a 12-item odor identification test after adjusting for such variables as sex, age, and education (Wilson et al., 2010). Of particular importance to the neurologist is the fact that chemosensory function can provide unique insight into neurological health. Thus, olfactory disturbances are among the early preclinical or presymptomatic signs of Alzheimer disease (AD) and sporadic Parkinson disease (PD) (Ross et al., 2008). Indeed, recent research suggests that a standardized odor identification test is as effective in detecting PD as a single-photon emission computed tomography (SPECT) scan employing [123I]ioflupane (DaTSCAN) (Deeb et al., 2010)
It is critical for the physician to realize that most complaints of decreased “taste” function reflect decreased olfactory function (Deems et al., 1991a). Flavor sensations such as cola, coffee, chocolate, strawberry, pizza, licorice, steak sauce, and vanilla depend upon stimulation of the olfactory receptors by molecules that enter the nasal pharynx during deglutition, a process called retronasal olfaction. Such “taste” sensations disappear when the olfactory epithelium is severely damaged, leaving intact only sensations from free nerve endings of the trigeminal nerve (CN V) and such taste bud–mediated sensations as sweet, sour, salty, bitter, and metallic. The ability to taste is much more resilient to pathological or trauma-related alterations than the ability to smell, largely reflecting the redundant innervation of the taste buds from multiple cranial nerves (i.e., CN VII, IX, X) (Deems et al., 1991a).
Anatomy and Physiology
Olfaction
The olfactory receptor cells, which number around 6 million in the human, are located within a pseudostratified columnar neuroepithelium that also contains sustentacular or supporting cells, basal cells (the precursors of other cell types within the epithelium), and the poorly understood microvillar cells. This epithelium lines the cribriform plate and sectors of the superior septum, the middle turbinate, and the superior turbinate and is supported by a highly vascularized lamina propria that contains Bowman glands, the major source of the overlying mucus and enzymes that detoxify xenobiotic agents. It is into this mucus that each of the bipolar receptor cells projects 3 to 30 receptor-bearing cilia that interact with odorant molecules. These cells are unique, since they serve as both a receptor cell and a first-order neuron and can regenerate to some degree from basal cells after being damaged. Moreover, they exhibit the most diverse molecular phenotype of any neuron, expressing a wide range of receptor protein types and cell-surface antigens. A photomicrograph of the surface of the olfactory epithelium is shown in Fig. 17.1.
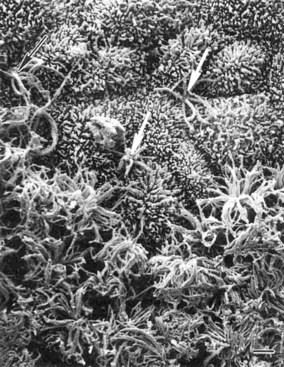
(From Menco, B.Ph.M., Morrison, E.E., 2003. Morphology of the mammalian olfactory epithelium: form, fine structure, function, and pathology. In: Doty, R.L. (Ed.), Handbook of Olfaction and Gustation, second ed. Marcel Dekker, New York, pp. 32-97, with permission.)
Unlike nearly all other central nervous system (CNS) neurons, the granule cells, as well as the largely dopaminergic periglomerular cells, undergo replacement over time (Altman, 1969). Astrocyte-like stem cells within the anterior subventricular zone of the brain generate large numbers of neuroblasts, some of which undergo restricted chain migration along the rostral migratory stream (Rousselot et al., 1994). This migration largely terminates within the granule cell layer of the olfactory bulb, from which some differentiating neuroblasts migrate more peripherally, thereby repopulating periglomerular cells. The architecture of the olfactory bulb, including its main cell types, is presented schematically in Fig. 17.2.
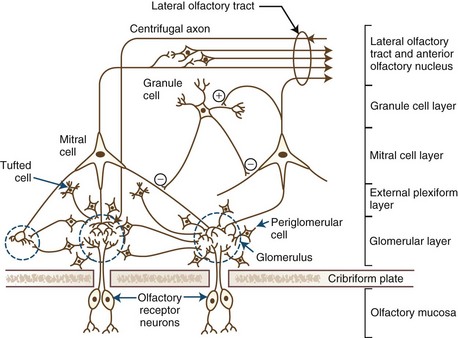
Fig. 17.2 Schematic of olfactory bulb structures, neurons, and layers.
(From Alloway, K.D., Pritchard, T.C., 2007. Medical Neuroscience. Hayes Barton, Raleigh, North Carolina, with permission.)
The relative roles of central brain structures in odor perception are poorly understood. The piriform cortex appears to encode higher-order representations of odor quality, identity, and familiarity. This brain region also plays a role in odor learning and memory, as well as in coordinating information between olfaction, taste, and vision (Gottfried et al., 2002). The entorhinal cortex preprocesses information entering the hippocampus, whereas the amygdala seems to respond to the intensity of emotionally significant odors. The rostral regions of the orbitofrontal cortex are involved in odor memory, whereas the caudal regions are associated with odor detection. The processing of hedonic information about odors seems to occur within the orbitofrontal cortex, with pleasant odors activating the medial orbitofrontal cortex and unpleasant odors activating the lateral orbitofrontal cortex.
Gustation
Taste plays a critical role in identifying substances in foods and beverages, such as sugars and poisonous alkaloids, that promote or disrupt homeostasis. The taste receptor cells are found within the taste buds, small flask-like structures located on the surface of the oral epithelium (Fig. 17.3). These cells extend microvilli into the lumen of the bud near its apical opening, termed the taste pore. Like olfactory receptor cells, taste receptors die and become replaced at various intervals from basal cells within the bud.
Humans possess approximately 7500 taste buds, most of which are found on lingual protuberances called papillae (Fig. 17.4). Taste buds innervated by the chorda tympani division of the facial nerve (CN VII) are found on the fungiform papillae, which are most dense on the tip and lateral margins of the anterior tongue. The palatine branch of the greater superficial petrosal division of CN VII innervates the taste buds on the soft palate. Some taste buds found on the anterior foliate papillae, located on a sector of the tongue’s posterior lateral margins, may also be innervated by branches of the chorda tympani nerve. Most foliate buds, as well as the buds on the circumvallate papillae—six to eight large structures that resemble flattened hills across the “chevron” of the posterior tongue—are innervated by the glossopharyngeal nerve (CN IX). Taste buds within the oral pharynx are supplied by the vagus (CN X) nerve. The small and somewhat pointed filiform papillae, which cover the entire tongue, harbor no taste buds. Although not involved in taste perception as such, the trigeminal nerve (CN V) participates in the formation of flavor via free nerve endings in the oral mucosa signaling sensations of touch, pain, and temperature. Thus, the fizziness of carbonated soft drinks and the warmth of coffee are dependent upon the stimulation of this nerve.
Individuals differ markedly in terms of the number and distribution of their taste buds. Although some physiology textbooks suggest that different regions of the tongue are responsible for the four basic taste qualities, this is an oversimplification of the facts. In general, the front of the tongue is more sensitive than other tongue regions to all taste qualities, although in the case of bitter, the back of the tongue is typically much more sensitive. The relative average sensitivity of tongue regions to the four prototypical taste qualities is shown in Fig. 17.5, although it must be emphasized that significant individual differences exist.
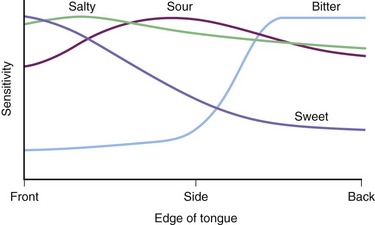
Fig. 17.5 Relative sensitivity of the edge of the tongue to the four classic taste qualities. Sensitivity reflects the reciprocal of the threshold value and is plotted as a ratio of maximal sensitivity = 1. Threshold data are from Hänig (1901). Note that all regions of the tongue that were evaluated were responsive to some degree to all stimuli, but that the anterior tongue was most sensitive to sweet, sour, and salty and least sensitive to bitter. The rear (base) of the tongue was relatively more sensitive to bitter.
(Adapted from Boring, E.G., 1942. Sensation and Perception in the History of Experimental Psychology. Appleton-Century Crofts, New York.)
The specific receptors involved in sensing taste stimuli have now been identified (Hoon et al., 1999). A small family of three G protein–coupled receptors (GPCRs)—T1R1, T1R2, and T1R3—encode sweet and umami (monosodium glutamate–like) sensations. Bitter sensations are mediated by the T2R receptors, a family of about 30 GPCRs that are expressed on cells different from those that express the sweet and umami receptors. The salty sensation of sodium chloride arises from the entrance of Na+ ions into the cells via specialized membrane channels such as the amiloride-sensitive Na+ channel. Although sour taste has been suggested to depend upon a range of receptors, PKD2L1 is likely the primary sour taste receptor.
The nerves innervating the taste buds converge centrally onto the nucleus of the solitary tract of the brainstem. Although species differences exist, the afferent taste nerve fibers can be classified electrophysiologically into categories based upon their relative responsiveness to sweet, sour, bitter, and salty-tasting stimuli. In the hamster, for example, sucrose-best, HCl-best, and NaCl-best fibers have been observed (Frank et al., 1988). Despite the fact that fibers are generally “tuned” for rather specific stimuli, they can nonetheless respond to other stimuli. For example, a few sucrose-best fibers also respond to NaCl and HCl. NaCl-best fibers and HCl-best fibers are less tightly tuned than sucrose-best fibers, with more fibers responding to multiple classes of stimuli.
Chemosensory Testing
Three general classes of sensory tests are available for quantifying human chemosensory function: psychophysical, electrophysiological, and psychophysiological (Doty, 2007). Psychophysical tests include tests where subjects make a conscious response, such as in tests of odor adaptation, detection, recognition, identification, discrimination, memory, hedonics, and suprathreshold scaling of various sensory dimensions. Electrophysiological tests measure minute stimulus-induced electrical changes from sensory receptors or the brain in the absence of verbal or other consciously overt subject responses. Included are summated electrical potentials from the surface of the olfactory epithelium (termed the electro-olfactogram), tongue, or scalp (changes in the electroencephalogram, such as event-related potentials or summated total power). Clinically, psychophysical tests have been most widely employed, in part due to reliability, practicality, and cost.
The most widely used psychophysical tests are those of identification and detection (Doty, 2007). In identification tests, a subject is typically asked to identify, usually from a list of alternatives, the quality of the sensation experienced when sniffing or tasting a stimulus. A response is required even if no sensation is perceived, a procedure called forced-choice responding. For example, in the most popular odor identification test, the University of Pennsylvania Smell Identification Test (UPSIT), the subject is provided with a series of 40 microencapsulated (scratch and sniff) odors and in each case asked to choose the name of the odor from four response alternatives (Doty et al., 1984b) (Fig. 17.6). The number of correct answers determines the degree of deficit and allows for both overall classification of function (i.e., normosmia, mild microsmia, moderate microsmia, severe microsmia, anosmia) and a relative percentile classification based upon age- and sex-related norms. Malingering can be discerned by improbable responses in the forced-choice situation, such as not correctly identifying any odors or otherwise identifying odors at a rate significantly below the expected chance performance of 25%. In an odor detection threshold test, a subject is typically presented with an odor and one or more blanks in random fashion and asked to identify which stimulus is stronger or otherwise discernable from the other stimuli. A common procedure is to present stronger stimuli when a miss occurs and weaker stimuli when a hit occurs, following a defined algorithm. This is termed a staircase procedure, and the threshold is defined as a set number of reversals of the staircase. With the exception of tests of hedonics and suprathreshold scaling, scores on tests of odor identification and detection, as well as discrimination and memory, are highly correlated, with the size of the correlations being dependent upon the less reliable of the intercorrelated tests (Doty et al., 1994). It is for this reason that a rather complete characterization of smell function can be obtained by simply using a reliable odor identification test.
Although very brief tests can be useful in screening, they have significant limitations. Short tests do not allow for the detection of malingering and are generally less sensitive than longer tests. As a general rule, the more trials contained in a test, the higher its reliability and sensitivity (Doty et al., 1995). Despite the fact that some very brief tests are reliable, their reliability is associated with less sensitivity and specificity, as brief tests can only clump patients into very broad dysfunction categories. This is analogous to the ability of a flashlight to determine blindness in a patient. While this may allow for accurate detection of absolute blindness, it does not allow for assessing varying degrees of less than total blindness.
Disorders of Olfaction
Many factors influence the ability to smell: age, sex, smoking behavior, reproductive state, nutrition, toxic exposures, head trauma, and numerous diseases (Table 17.1). Men generally perform less well than women on olfactory tests. Age is a major correlate of smell dysfunction, with significant decrements occurring in over 50% of those between 65 and 80 years of age and in 75% of those 80 years of age and older (Doty et al., 1984a) (Fig. 17.7). Such losses help explain why many elderly find food distasteful and succumb to nutritional deficiencies and, in rare instances, natural gas poisoning.
Table 17.1 Disorders and Conditions Associated with Compromised Olfactory Function, as Measured by Olfactory Testing
22q11 Deletion syndrome | Lubag |
HIV/AIDS | Medications |
Adenoid hypertrophy | Migraine |
Adrenal cortical insufficiency | MS |
Age | Multiple system atrophy |
Alcoholism | Multi-infarct dementia |
Allergies | Narcolepsy with cataplexy |
AD | Neoplasms, cranial/nasal |
ALS | Nutritional deficiencies |
Anorexia nervosa | Obstructive pulmonary disease |
Asperger syndrome | Obesity |
Ataxias | OCD |
Attention deficit/hyperactivity disorder | Orthostatic tremor |
Bardet-Biedl syndrome | Panic disorder |
Chemical exposure | PD |
COPD | Parkinson dementia complex of Guam |
Congenital | Pick disease |
Creutzfeldt-Jakob disease | PTSD |
Cushing syndrome | Pregnancy |
Cystic fibrosis | Pseudohypoparathyroidism |
Degenerative ataxias | Psychopathy |
Diabetes | Radiation (therapeutic, cranial) |
Down syndrome | REM behavior disorder |
Epilepsy | Refsum disease |
Facial paralysis | Renal failure/end-stage kidney disease |
FTLD | Restless legs syndrome |
Gonadal dysgenesis (Turner syndrome) | Rhinosinusitis/polyposis |
Guamanian ALS/PD/dementia syndrome | Schizophrenia |
Head trauma | Seasonal affective disorder |
Herpes simplex encephalitis | Sjögren syndrome |
Hypothyroidism | Stroke |
HD | Tobacco smoking |
Iatrogenesis | Toxic chemical exposure |
Kallmann syndrome | Upper respiratory infections |
Korsakoff psychosis | Usher syndrome |
Leprosy | Vascular disorders (e.g., aneurysms, hemorrhages) |
Liver disease | Vitamin B12 deficiency |
AD, Alzheimer disease; AIDS, acquired immunodeficiency syndrome; ALS, amyotrophic lateral sclerosis; COPD, chronic obstructive pulmonary disease; FTLD, frontotemporal lobar degeneration; HD, Huntington disease; HIV, human immunodeficiency virus; MS, multiple sclerosis; OCD, obsessive compulsive disorder; PD, Parkinson disease; PTSD, posttraumatic stress disorder; REM, rapid eye movement.
The three most common causes of long-lasting or permanent smell loss of patients who present to smell and taste centers are, in order of frequency, upper respiratory infections, head trauma, and chronic rhinosinusitis (Deems et al., 1991a). Congenital, iatrogenic, and toxic chemical exposures are the next most common causes. These etiologies can result in permanent damage to the olfactory neuroepithelium, decreased number of receptor cells, and replacement of sensory epithelium with other types of epithelia. Susceptibility to such damage likely increases from reduction or inhibition of mucociliary transport by a range of factors including diet, drugs, disease, genetics, and age-related changes in nasal function and normal defense mechanisms.
Symptoms of the common cold and influenza are readily apparent to the patient, but it is important to remember that most viral infections are either entirely asymptomatic or so mild that they go unrecognized. Thus, during seasonal epidemics, the number of serologically documented influenza or arboviral encephalitis infections exceeds the number of acute cases by several hundred-fold (Stroop, 1995). For these and other reasons, many idiopathic cases of smell dysfunction likely reflect unrecognized viral infections. Smell dysfunction has been reported in rare instances following influenza vaccine inoculations (Fiser and Borotski, 1979). This may reflect a subtle but defining influence on an already compromised olfactory epithelium, although coincidental viral infection cannot be excluded from consideration. Under certain circumstances, some viruses can enter the brain after incorporation into the olfactory receptor cells, possibly catalyzing neurodegenerative disease (Doty, 2008). Such viruses as herpes simplex types 1 and 2, polio, the Indiana strain of wild-type vesicular stomatitis, rabies, mouse hepatitis, Borna disease, and canine distemper viruses are neurotropic for peripheral olfactory structures.
Loss of smell function from head trauma usually reflects coup-contrecoup movement of the brain that shears off the olfactory fila at the level of the cribriform plate (Doty et al., 1997). In most cases, scar tissue forms, precluding reconnection of axons from regenerating receptor cells. Fractures of the cribriform plate or other elements of the skull are rare in such cases and are not a prerequisite for the smell loss. In general, the more severe the head trauma, the higher the likelihood that smell loss is present.
A major development in neurology is the discovery that a number of neurodegenerative diseases are associated with smell loss early in their course, most notably AD and PD. In most cases, the smell loss precedes the presentation of the classic clinical phenotype by several years. Interestingly, a number of disorders often confused with these two diseases are unaccompanied by meaningful olfactory dysfunction, making smell testing potentially useful as an aid in differential diagnosis. For AD, major affective disorder is an example (McCaffrey et al., 2000). For PD, such examples include progressive supranuclear palsy (PSP) (Doty et al., 1993), essential tremor (Busenbark et al., 1992), MPTP-induced parkinsonism (Doty et al., 1992), and vascular parkinsonism (Katzenschlager et al., 2004). The relative severity of olfactory dysfunction in a range of neurodegenerative diseases and in schizophrenia is shown in Table 17.2.
Table 17.2 Relative Degree of Olfactory Dysfunction in Various Neurological Diseases on an Arbitrary Scale
Disease | Relative Severity of Smell Loss |
---|---|
Idiopathic PD, AD, DLB, Guam PD-dementia complex, idiopathic rapid eye movement sleep behavior disorder | ++++ |
HD, Down syndrome, PARK8 PD | +++ |
Multiple system atrophy (type-P), PARK1 PD, pallidopontonigral degeneration, drug-induced PD?, schizophrenia, semantic dementia?, X-linked dystonia-parkinsonism (Lubag), narcolepsy | ++ |
Motor neuron disease, SCA2 PD, Friedreich ataxia, PARK3, corticobasal degeneration, FTD | + |
Major affective disorder, essential tremor, vascular parkinsonism, MPTP-induced parkinsonism, idiopathic dystonia, SCA3 PD, PSP; PARK2 | 0 |
Key: ++++ marked damage; + mild damage; 0 normal. Note that most of the values are based on relatively small patient numbers except for idiopathic PD.
AD, Alzheimer disease; DLB, dementia with lewy bodies; FTD, frontotemporal dementia; HD, Huntington disease; PD, Parkinson disease; PSP, progressive supranuclear palsy; SCA, spinocerebellar atrophy.
Modified and updated from Hawkes, C.H., Doty, R.L., 2009. The Neurology of Olfaction. Cambridge University Press, Cambridge, with permission.
It is noteworthy that the olfactory loss is present in idiopathic rapid eye movement sleep behavior disorder (iRBD) as well as in PD, since individuals with iRBD frequently develop PD. The fact that rapid eye movement(REM) behavior disorder is seen not only in its idiopathic form but in association with narcolepsy led has led to findings that narcolepsy—independent of REM behavior disorder—is associated with a significant impairment in olfactory function (Buskova et al., 2010; Stiasny-Kolster et al., 2007). Orexin A, also called hypocretin-1, is significantly decreased or undetectable in the cerebrospinal fluid of patients with narcolepsy and cataplexy. The orexin-containing hypothalamic neurons project throughout the entire olfactory system (from the olfactory epithelium to the olfactory cortex) (Caillol et al., 2003). Thus, damage to these projections may potentially impair olfactory performance in narcoleptic patients. The intranasal administration of orexin A (hypocretin-1) to narcoleptic patients with cataplexy has been found to improve their olfactory function, implying that mild olfactory impairment is not only a primary feature of this disorder but that CNS orexin deficiency could be a possible mechanism for this loss (Baier et al., 2008).
Disorders of Taste
As noted in the beginning of the chapter, most patients with complaints of taste loss have olfactory dysfunction, not taste dysfunction. This reflects the greater fragility of the olfactory system and the dependence of flavor sensations upon retronasal stimulation of this system. Impairment of whole-mouth gustatory function is rare outside of generalized metabolic disturbances, such as from diabetes, chronic renal failure, end-stage liver disease, thyroid disease, hypothyroidism, medications, and vitamin and mineral deficiencies. Nonetheless, taste perception can be altered by (1) viral invasion of one or more taste nerves, (2) the release of foul-tasting materials from the nasal and oral cavities secondary to medical conditions and oral appliances (e.g., rhinosinusitis, gingivitis, purulent sialadenitis), (3) transport problems of tastants to the taste buds (e.g., scaring of the lingual surface, mucosal drying, inflammatory conditions, infections), (4) damage to the taste buds (e.g., invasive carcinomas, local trauma), (5) damage to the taste nerves (e.g., chorda tympani damage from Bell palsy, middle ear infections or operations), and (6) damage to taste-related CNS structures from disorders such as multiple sclerosis, tumors, epilepsy, and stroke. Lesions caudal to the pons produce ipsilateral deficits, whereas lesions within the pons proper can produce ipsilateral, contralateral, or bilateral deficits. Both ipsilateral and contralateral taste deficits have been noted in patients with lesions of the insular cortex, reflecting the bilateral representation of taste function at this level (Pritchard et al., 1999). Unlike CN VII, CN IX is relatively protected along its path, although iatrogenic interventions can result in CN IX injury (e.g., from tonsillectomy, bronchoscopy, laryngoscopy, radiation therapy), and this nerve is not immune to damage from tumors, vascular lesions, and infection. On rare occasion, epilepsy or migraine is associated with a gustatory prodrome or aura, and some tastes may actually trigger seizures or migraine attacks.
The influence of medications on taste function is well established. Over 250 medications have been implicated in taste dysfunction, including antineoplastic agents, antirheumatic drugs, antibiotics, and blood pressure medications (Doty et al., 2008). Terbinafine, a popular antifungal, can produce long-lasting loss of sweet, sour, bitter, and salty taste perception (Doty and Haxel, 2005). A recent double-blind study found that eszopiclone, a widely used sleep medication, induces a bitter dysgeusia in approximately two-thirds of individuals tested (Doty et al., 2009). This sensation was related to the time since drug administration, was stronger for women than for men, and correlated with both saliva and blood levels of the drug.
Treatment and Management
Management of chemosensory disorders is condition specific. Optimism for prognosis is warranted for most patients with obstructive or inflammatory disorders (e.g., allergic rhinitis, glossitis, polyposis, intranasal or intraoral neoplasms) for which medical or surgical interventions are available. In cases of rhinosinusitis, for example, an oral taper of prednisone can initially be used to quell general inflammation, followed by topical administration of the nasal spray or drops in the inverted head position, such as the Moffett position (Canciani and Mastella, 1988), increasing the likelihood of the material reaching the olfactory epithelium. Candidiasis or other oral infections can be quelled with topical antifungal and antibiotic treatments. Some salty or bitter dysgeusias respond to chlorhexidine mouthwash, possibly as a result of its strong positive charge (Wang et al., 2009). Patients with excessive oral dryness, including dryness due to medications, often benefit from the use of mints, lozenges, or sugarless gum, as well as from oral pilocarpine or artificial saliva.
Medications that induce distortions of smell or taste can often be discontinued and other types of medications or modes of therapy substituted. Unfortunately, little empirical data are available for most drugs, and some drug-related effects on the taste system appear to be long lasting and not reversed by short-term drug discontinuance (Doty et al., 2008). There is suggestion that some antioxidants such as α-lipoic acid may be effectual in some cases of hyposmia, hypogeusia, dysosmia, dysgeusia, and burning mouth syndrome (Hummel et al., 2002), although strong scientific evidence for its efficacy is lacking. Despite being widely mentioned in the medical literature, zinc and vitamin A therapies offer unlikely benefit for olfactory disturbances except when frank deficiencies are present, although both of these agents may improve taste dysfunction secondary to hepatic deficiencies (Deems et al., 1991b). A recent report that theophylline improved smell function was not double blinded and lacked a control group, failing to take into account that some meaningful improvement occurs without treatment (Henkin et al., 2009). Indeed, the percentage of patients reported to be responsive to the treatment was about the same as that noted by others to show spontaneous improvement over a similar time period. Similar issues are inherent in a recent claims of efficacy for acupuncture and transcranial magnetic stimulation. There are claims that some antiepileptics and antidepressants (e.g., amitriptyline) may be of value in treating some chemosensory disturbances, particularly following head trauma. However, in the case of amitriptyline, there is clear evidence that it can distort taste function, possibly from its anticholinergic effects (Schiffman et al., 1999). A recent study suggests that donepezil (acetylcholinesterase inhibitor) improved odor identification scores in patients with AD, and that such scores correlated with overall clinician-based impressions of change scales (CIBIC-plus), leading the authors to suggest that tests of smell identification function may be useful in assessing treatment responses to this medication (Velayudhan and Lovestone, 2009).
It is of interest that repeated exposure to odorants may in fact increase sensitivity to them in both animals and humans, providing a rationale for therapies in which multiple odors are smelled before and after going to bed (Hummel et al., 2009). However, double-blind studies with appropriate controls are needed to confirm the effectiveness of this approach. Importantly, spontaneous recovery over time occurs in some instances, providing hope to at least some patients. In a longitudinal study of 542 patients presenting to our center with smell loss from a variety of causes, modest improvement occurred over an average time period of 4 years in about half of the participants (London et al., 2008). Nonetheless, normal age-related function returned in only 11% of the anosmic and 23% of the hyposmic patients. The amount of dysfunction present at the time of presentation, not etiology, was the best predictor of prognosis. Other predictors were patient age and the time between dysfunction onset and initial testing.
Alloway K.D., Pritchard T.C. Medical Neuroscience. Raleigh NC: Hayes Barton; 2007.
Altman J. Autoradiographic and histological studies of postnatal neurogenesis. IV. Cell proliferation and migration in the anterior forebrain, with special reference to persisting neurogenesis in the olfactory bulb. J Comp Neurol. 1969;137(4):433-457.
Baier P.C., Weinhold S.L., Huth V., et al. Olfactory dysfunction in patients with narcolepsy with cataplexy is restored by intranasal orexin A (hypocretin-1). Brain. 2008;131(Pt 10):2734-2741.
Boring E.G. Sensation and perception in the history of experimental psychology. New York: Appleton-Century Crofts; 1942.
Busenbark K.L., Huber S.I., Greer G., et al. Olfactory function in essential tremor. Neurology. 1992;42:1631-1632.
Buskova J., Klaschka J., Sonka K., et al. Olfactory dysfunction in narcolepsy with and without cataplexy. Sleep Med. 2010;11:558-561.
Caillol M., Aioun J., Baly C., et al. Localization of orexins and their receptors in the rat olfactory system: possible modulation of olfactory perception by a neuropeptide synthesized centrally or locally. Brain Res. 2003;960(1-2):48-61.
Canciani M., Mastella G. Efficacy of beclomethasone nasal drops, administered in the Moffett’s position for nasal polyposis. Acta Paediatr Scand. 1988;77:612-613.
Deeb J., Shah M., Muhammed N., et al. A basic smell test is as sensitive as a dopamine transporter scan: comparison of olfaction, taste, and DaTSCAN in the diagnosis of Parkinson’s disease. Q J Med. 2010. QJM Advance Access published August 23, 2010. doi:10.1093/qjmed/hcq142
Deems D.A., Doty R.L., Settle R.G., et al. Smell and taste disorders, a study of 750 patients from the University of Pennsylvania Smell and Taste Center. Arch Otolaryngol Head Neck Surg. 1991;117(5):519-528.
Deems R.O., Friedman M.I., Friedman L.S., et al. Clinical manifestations of olfactory and gustatory disorders associated with hepatic and renal disease. In: Getchell T.V., Doty R.L., Bartoshuk L.M., et al. Smell and Taste in Health and Disease. New York: Raven Press; 1991:805-827.
Doty R.L. Office procedures for quantitative assessment of olfactory function. Am J Rhinol. 2007;21(4):460-473.
Doty R.L. The olfactory vector hypothesis of neurodegenerative disease: is it viable? Ann Neurol. 2008;63(1):7-15.
Doty R.L., Golbe L.I., McKeown D.A., et al. Olfactory testing differentiates between progressive supranuclear palsy and idiopathic Parkinson’s disease. Neurology. 1993;43(5):962-965.
Doty R.L., Haxel B.R. Objective assessment of terbinafine-induced taste loss. Laryngoscope. 2005;115(11):2035-2037.
Doty R.L., McKeown D.A., Lee W.W., et al. A study of the test-retest reliability of ten olfactory tests. Chem Senses. 1995;20:645-656.
Doty R.L., Shah M., Bromley S.M. Drug-induced taste disorders. Drug Saf. 2008;31(3):199-215.
Doty R.L., Shaman P., Applebaum S.L., et al. Smell identification ability: changes with age. Science. 1984;226:1441-1443.
Doty R.L., Shaman P., Dann M. Development of the University of Pennsylvania Smell Identification Test: a standardized microencapsulated test of olfactory function. Physiol Behav. 1984;32:489-502.
Doty R.L., Singh A., Tetrud J., et al. Lack of major olfactory dysfunction in MPTP-induced parkinsonism. Ann Neurol. 1992;32(1):97-100.
Doty R.L., Smith R., McKeown D.A., et al. Tests of human olfactory function: principal components analysis suggests that most measure a common source of variance. Percept Psychophys. 1994;56(6):701-707.
Doty R.L., Treem J., Tourbier I., et al. A double-blind study of the influences of eszopiclone on dysgeusia and taste function. Pharmacol Biochem Behav. 2009;94:312-318.
Doty R.L., Yousem D.M., Pham L.T., et al. Olfactory dysfunction in patients with head trauma. Arch Neurol. 1997;54(9):1131-1140.
Fiser D.J., Borotski L. Anosmia after administration of influenza vaccine. Medicinski Pregled. 1979;32:455-457.
Frank M.E., Bieber S.L., Smith D.V. The organization of taste sensibilities in hamster chorda tympani nerve fibers. J Gen Physiol. 1988;91(6):861-896.
Gottfried J.A., Deichmann R., Winston J.S., et al. Functional heterogeneity in human olfactory cortex: an event-related functional magnetic resonance imaging study. J Neurosci. 2002;22(24):10819-10828.
Hänig D.P. Zur Psychophysik des Geschmachssinns. Phil Stud. 1901;17:576-623.
Hawkes C.H., Doty R.L. The Neurology of Olfaction. Cambridge: Cambridge University Press; 2009.
Henkin R.I., Velicu I., Schmidt L. An open-label controlled trial of theophylline for treatment of patients with hyposmia. Am J Med Sci. 2009;337(6):396-406.
Hoon M.A., Adler E., Lindemeier J., et al. Putative mammalian taste receptors: a class of taste-specific GPCRs with distinct topographic selectivity. Cell. 1999;96(4):541-551.
Hummel T., Heilmann S., Huttenbriuk K.B. Lipoic acid in the treatment of smell dysfunction following viral infection of the upper respiratory tract. Laryngoscope. 2002;112(11):2076-2080.
Hummel T., Rissom K., Reden J., et al. Effects of olfactory training in patients with olfactory loss. Laryngoscope. 2009;119(3):496-499.
Katzenschlager R., Zijlmans J., Evans A., et al. Olfactory function distinguishes vascular parkinsonism from Parkinson’s disease. J Neurol Neurosurg Psychiatry. 2004;75(12):1749-1752.
London B., Nabet B., Fisher A.R., et al. Predictors of prognosis in patients with olfactory disturbance. Ann Neurol. 2008;63(2):159-166.
McCaffrey R.J., Duff K., Solomon G.S. Olfactory dysfunction discriminates probable Alzheimer’s dementia from major depression: a cross-validation and extension. J Neuropsychiatry Clin Neurosci. 2000;12(1):29-33.
Menco B.Ph.M., Morrison E.E. Morphology of the mammalian olfactory epithelium: form, fine structure, function, and pathology. In: Doty R.L., editor. Handbook of Olfaction and Gustation. New York: Marcel Dekker; 2003:32-97.
Pritchard T.C., Macaluso D.A., Eslinger P.J. Taste perception in patients with insular cortex lesions. Behav Neurosci. 1999;113(4):663-671.
Ross G.W., Petrovitch H., Abbott R.D., et al. Association of olfactory dysfunction with risk for future Parkinson’s disease. Ann Neurol. 2008;63(2):167-173.
Rousselot P., Lois C., Alvarez-Buylla A. Embryonic (PSA) N-CAM reveals chains of migrating neuroblasts between the lateral ventricle and the olfactory bulb of adult mice. J Comp Neurol. 1994;351(1):51-61.
Santos D.V., Reiter E.R., DiNardo L.J., et al. Hazardous events associated with impaired olfactory function. Arch Otolaryngol Head Neck Surg. 2004;130:317-319.
Schiffman S.S., Zervakis J., Suggs M.S., et al. Effect of medications on taste: example of amitriptyline HCl. Physiol Behav. 1999;66(2):183-191.
Stiasny-Kolster K., Clever S.C., Moller J.C., et al. Olfactory dysfunction in patients with narcolepsy with and without REM sleep behaviour disorder. Brain. 2007;130:442-449.
Stroop W.G. Viruses and the olfactory system. In: Doty R.L., editor. Handbook of Olfaction and Gustation. New York: Marcel Dekker; 1995:367-393.
Velayudhan L., Lovestone S. Smell identification test as a treatment response marker in patients with Alzheimer disease receiving donepezil. J Clin Psychopharmacol. 2009;29(4):387-390.
Wang M.F., Marks L.E., Frank M.E. Taste coding after selective inhibition by chlorhexidine. Chem Senses. 2009;34(8):653-666.
Wilson R.S., Yu L., Bennett D.A. Odor identification and mortality in old age. Chem Senses. 2010;36:63-67.