Chapter 440 Development of the Hematopoietic System
Hematopoiesis in the Human Embryo and Fetus
Each hematopoietic organ houses distinct populations of cells. At 18-20 wk, the fetal liver is predominantly an erythropoietic organ, and the marrow produces both erythrocytes and neutrophils. The types of leukocytes present in the fetal liver and marrow differ with gestation. Macrophages precede neutrophils in the marrow, and the ratio of macrophages to neutrophils decreases as gestation progresses. Regardless of gestational age or anatomic location, production of all hematopoietic tissues begins with pluripotent stem cells capable of both self-renewal and clonal maturation into all blood cell lineages. Progenitor cells differentiate under the influence of hematopoietic growth factors (Table 440-1). Fetal hematopoietic growth factor production is independent of maternal growth factor production (Fig. 440-1).
Erythrocytes in the fetus are larger than in adults, and at 22-23 wk gestation the mean corpuscular volume can be as high as 135 fL (Fig. 440-2, upper panel). Similarly the mean corpuscular hemoglobin is very high at 22-23 wk and falls relatively linearly with advancing gestation (see Fig. 440-2, lower panel). In contrast, the mean corpuscular hemoglobin concentration is constant throughout gestation at 34 ± 1 g/fL. Although the size and quantity of hemoglobin in erythrocytes diminish during gestation, the hematocrit and blood hemoglobin concentration gradually increase (Fig. 440-3).
Concentrations of platelets in the blood increase gradually between 22 and 40 wk gestation (Fig. 440-4), but the platelet size, assessed by mean platelet volume, remains constant at 8 ± 1 fL. No differences are observed between males and females in fetal and neonatal reference ranges for erythrocyte indices, hematocrit, hemoglobin, platelet counts, or mean platelet volume measurements.
Fetal Thrombopoiesis
Thrombopoietin (TPO) is the physiologic regulator of platelet production and acts as a stimulator of all stages of megakaryocyte growth and development (see Table 440-1). The gene encoding TPO is located on the long arm of chromosome 3. TPO-mRNA is expressed primarily in liver and kidney and, to a lesser extent, in marrow stroma. TPO is a primary, but not exclusive, regulator of platelet production and stimulates the proliferation and survival not only of megakaryotic progenitors but also of erythroid, myeloid, and multipotent progenitors. Recombinant TPO (rTPO) supports the growth of megakaryocytic colonies of neonates and children, and progenitors of preterm neonates are more sensitive to rTPO than are progenitors of term neonates.
Hemoglobins in the Fetus and Neonate
The various globin chains differ in both the number and sequence of amino acids, and the synthesis of these chains is directed by separate genes (Fig. 440-5). Two sets of genes for the α chains are located on human chromosome 16. Two pairs of alleles provide the genetic information for the structure of the α chain. The β, γ, and δ genes are closely linked on chromosome 11.
Within the red blood cell (RBC) mass of an embryo, fetus, child, and adult, 6 different hemoglobins may normally be detected (Fig. 440-6): the embryonic hemoglobins, Gower-1, Gower-2, and Portland; the fetal hemoglobin, HbF; and the adult hemoglobins, HbA and HbA2. The electrophoretic mobilities of hemoglobins vary with their chemical structures. The time of appearance and quantitative relationships among the hemoglobins are determined by complex developmental processes.
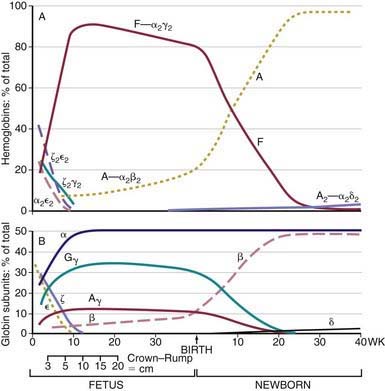
Figure 440-6 Changes in hemoglobin tetramers (A) and in globin subunits (B) during human development from embryo to early infancy.
(From Polin RA, Fox WW: Fetal and neonatal physiology, ed 2, Philadelphia, 1998, WB Saunders, p 1769.)
Fetal Hemoglobin
HbF contains γ polypeptide chains in place of the β chains of HbA. Its resistance to denaturation by strong alkali is the basis for determining the presence of fetal RBCs in the maternal circulation (the Kleihauer-Betke test). After the 8th wk, HbF is the predominant hemoglobin; at 24 wk of gestation it constitutes 90% of the total hemoglobin. During the 3rd trimester, a gradual decline occurs, so that at birth HbF averages 70% of the total hemoglobin. Synthesis of HbF decreases rapidly postnatally (Fig. 440-7), and by 6-12 mo of age only a trace is present.
Alterations of the Hemoglobins by Disease
HbF levels may be influenced by various factors. Because the HbF level is elevated during the 1st year of life, knowledge of its normal decline is important (see Figs. 440-6 and 440-7). In persons heterozygous for β-thalassemia (β-thalassemia trait), postpartum decrease of HbF is delayed; about 50% of such persons have elevated levels of HbF (>2.0%) in later life. In homozygous thalassemia (Cooley anemia) and in hereditary persistence of HbF, large amounts of HbF characteristically are found. In patients with major β-chain hemoglobinopathies (HbSS, HbSC), HbF usually is increased, particularly during childhood. Preterm infants treated with human recombinant EPO increase HbF production during active erythropoiesis. Moderate elevations of HbF can occur in many diseases accompanied by hematologic stress, such as hemolytic anemias, leukemia, and aplastic anemia, because of a minor population of RBCs that contain increased amounts of HbF. Tetramers of γ chains (γ4 or Hb Barts) or β chains (β4, HbH) may be found in α-thalassemia syndromes.
The normal adult level of HbA2 (2.0-3.4%) is seldom altered. Levels of HbA2 >3.4% are found in most persons with the β-thalassemia trait and in persons with megaloblastic anemias secondary to vitamin B12 and folic acid deficiency. Decreased HbA2 levels are found in persons with iron-deficiency anemia (Chapter 455) and α-thalassemia (Chapter 462).
Andrews NC. Genes determining blood cell traits. Nat Genetics. 2009;11:1161-1162.
Bishara N, Ohls RK. Current controversies in the management of the anemia of prematurity. Semin Perinatol. 2009;33:29-34.
Christensen RD, Jopling J, Henry E, Wiedmeier SE. The erythrocyte indices of neonates, defined using data from over 12,000 patients in a multihospital healthcare system. J Perinatol. 2008;28:24-28.
Dame C, Juul S. The switch from fetal to adult erythropoiesis. Clin Perinatol. 2000;27:507-526.
Deutsch VR, Toner A. Megakaryocyte development and platelet production. Br J Haematol. 2006;134:453-466.
Jopling J, Henry E, Wiedmeier SE, et al. Reference ranges for hematocrit and blood hemoglobin concentration during the neonatal period: data from a multihospital healthcare system. Pediatr. 2009;123:e333-e337.
Kaushansky K. Lineage-specific hematopoietic growth factors. N Engl J Med. 2006;354:2034-2045.
Sola-Visner MC, Christensen RD, Hutson AD, et al. Megakaryocyte size and concentration in the bone marrow of thrombocytopenic and nonthrombocytopenic neonates. Pediatr Res. 2007;61:479-484.
Spangrude GJ, Perry SS, Slayton WB. Early stages of hematopoietic differentiation. Ann N Y Acad Sci. 2003;996:186-194.
Vats A, Bielby RC, Tolley NS, et al. Stem cells. Lancet. 2005;366:592-602.
Wiedmeier SE, Henry E, Sola-Visner MC, et al. Platelet reference ranges for neonates, defined using data from over 47,000 patients in a multihospital healthcare system. J Perinatol. 2009;29:130-136.
Yoshimoto M, Yoder MC. Developmental biology: birth of the blood cell. Nature. 2009;457:801-813.