CHAPTER 343 Cerebral Blood Flow and Metabolism and Cerebral Ischemia
Cerebral Metabolism
Overview
Although many aspects of human cerebral metabolism are common to the metabolism of other tissues and organs in the body, there are a few fundamental differences. First, the brain is an unusual organ in having the highest energy requirement by mass. Even though it constitutes less than 2% of body weight, the adult brain receives 25% of cardiac output at rest and uses 20% of the total energy produced by the body.1 In children, the figures are even more impressive, with up to 50% of the energy consumption of the body being accounted for by the brain. Much of this energy allocation is devoted to activities connected to neural signaling, most of all (>50%) to the work of adenosine triphosphate (ATP)-driven ion pumps, particularly sodium-potassium adenosine triphosphatase (Na+,K+-ATPase), which maintains and restores the transmembrane Na+/K+ gradients that are repeatedly diminished by the propagation of action potentials and synaptic transmission.2,3 Other signaling-related costs pertain to neurotransmitter synthesis and reuptake from the synaptic cleft and axoplasmic transport. The remainder of energy expenditure is due to so-called housekeeping activities, such as the synthesis of molecules for general cellular purposes.4 These energy demands necessitate that the brain have reliable mechanisms to adequately protect its supply of oxygen and glucose from blood and ensure that it is tightly matched with demand (i.e., the level of neural activity). Second, the metabolism of the brain is distinguished by the singular contribution of astrocytes. Third, the brain possesses a BBB. Fourth, the brain exhibits highly developed metabolic compartmentalization, which refers to the fact that astrocytes and neuronal cells are so metabolically specialized that certain substrates and synthesized products are restricted to a particular cell type or “compartment” even though they may be required for the function of another. As detailed later, this feature necessitates close interaction and trafficking of molecules between cells of different type.5–8 More is said on this interdependence later.
Cerebral Metabolic Rate
The global cerebral metabolic rate (CMR) is conventionally expressed in terms of the consumption of glucose (CMRGlc) or oxygen (CMRO2), which respectively measures 25 to 30 µmol/100 g per minute and 130 to 180 µmol/100 g per minute in a resting human adult.9,10 CMRO2 is considered a function of mitochondrial activity and can be calculated from CBF and the arteriovenous oxygen content difference. Notably, CMRGlc is considerably higher during the first few years of life because of rapid brain growth and myelination, with a gradual reduction to adult levels by the end of the second decade.11 Good correlation between CBF, CMRO2, and CMRGlc is seen during rest, with the ratio between CMRO2 and CMRGlc being maintained at around 6 : 1. However, with neural activation, this relationship is altered.
The metabolism of the brain exhibits considerable variation on multiple levels: by region, activation state, cell type, and subcellular location. Regional variance is reflected in the local metabolic rates for oxygen and glucose (LCMRO2 and LCMRGlc), as well as in the levels of some metabolic enzymes. On the whole, a wider and much higher range of LCMRGlc is exhibited by cerebral gray matter than by white matter, with the highest values recorded in physiologically more active areas such as the auditory cortex, inferior colliculus, and somatosensory cortex.12,13 Consistent with this disparity, gray matter exceeds white matter in the activity of the key enzyme of mitochondrial energy metabolism, cytochrome oxidase.14,15 At the cellular level, neuronal energy needs are estimated to greatly exceed that of glial cells, even though neurons are significantly outnumbered by glia.2 The higher metabolic activity of neurons relative to glial cells is suggested by their higher mitochondrial density and expression of cytochrome oxidase,16 as well as by their heightened sensitivity to hypoxic or hypoglycemic injury. Within the neuron itself, there is further evidence of heterogeneity of metabolic activity, with dendrites and synaptic terminals having higher cytochrome oxidase activity than cell bodies and axons.16
Energy Capture and Transfer
ATP is the principal energy currency of all living cells, including neurons and glial cells. The energy of catabolic processes is captured in the two high-energy phosphate bonds of ATP, largely through the process of mitochondrial oxidative phosphorylation (Fig. 343-1). Only minor contributions derive from substrate-level phosphorylation in the glycolytic pathway and the tricarboxylic acid (TCA; also known as the citric acid or Kreb’s) cycle. In contrast, ATP utilization occurs mostly in the cytosol through cleavage of the terminal orthophosphate group of ATP by hydrolytic enzymes, collectively referred to as ATP hydrolases or “ATPases” for short, to liberate adenosine diphosphate (ADP). ADP can then undergo similar breakdown to release adenosine monophosphate (AMP). ATP can also be directly hydrolyzed to AMP and inorganic pyrophosphate (PPi). All these hydrolytic reactions are energy producing, or exergonic, and are coupled to many energy-requiring, or endergonic, reactions that could not otherwise proceed because of unfavorable thermodynamics. The high energy demand in the brain results in fast cycling between ATP, ADP, and inorganic phosphate (Pi), which requires fast transport between the mitochondria and cytosol. When ATP utilization is rapid or ATP generation is inhibited, the brain is assisted in the maintenance of ATP supply by the following buffer systems:
Reaction 1, catalyzed by creatine kinase, yields ATP from phosphocreatine (PCr), a storage form of high-energy phosphate. PCr also functions as a shuttle for the transfer of high-energy phosphate from mitochondria to the cytosol. The importance of PCr in energy homeostasis is underscored by the fact that the total creatine pool (as Cr and PCr) in the brain is at least 3 times larger than the adenosine nucleotide pool (AMP, ADP, and ATP). Reaction 2, catalyzed by adenylate kinase, re-creates ATP from ADP. Although other nucleoside triphosphates, such as guanosine triphosphate (GTP), cytidine triphosphate, and uridine triphosphate (UTP), serve functions similar to those of ATP, their regeneration from the corresponding nucleoside diphosphates occurs at the expense of ATP.17 Therefore, directly or indirectly, ATP drives all endergonic reactions of the cell.
Choice of Metabolic Substrates
Although the brain harnesses energy from a variety of substrates, it relies predominantly on glucose. Indeed, measurement of cerebral arteriovenous levels of a range of substrates and their metabolic products in mature humans under normal steady-state conditions has established that glucose is the only energy substrate that is taken up by endothelial cells of the BBB in more than trivial amounts.18 The virtual parity of the respiratory quotient under normal conditions demonstrates the overwhelming but not complete consumption of this glucose by oxidation. The brain also obtains some energy from the metabolism of other substrates such as amino acids and endogenously produced carbohydrates and lactate. Recent studies have even suggested that glial-derived lactate may actually be the preferred fuel of neurons,19,20 but on a whole-organ basis, no single metabolic process apart from glucose oxidation has the yield to support the intense activity of the brain for more than very brief periods. Therefore, with the brain possessing just low stores of glucose,8 moderate to severe hypoglycemia can result in rapid deterioration in the level of consciousness and essential cerebral functions.21 A notable exception occurs in fasting individuals and nursing babies, in whom the imposition of hypoglycemia and high fat metabolism can lead to the dominant use of ketone bodies by the brain for cellular fuel. In this regard, it is interesting that the rate of transport of ketone bodies across the BBB is the limiting step in terms of their cerebral metabolism.22,23 However, even though the oxidation of ketone bodies may provide up to 75% of the total cerebral energy supply,24 it is unable to serve as a complete replacement for the oxidation of glucose.
Metabolism of Glucose
Glucose and its metabolites occupy key positions at the intersection point of a number of essential catabolic and anabolic pathways. As discussed earlier, glucose is the main substrate for energy production, which occurs via glycolytic and TCA cycle metabolism (see Fig. 343-1). In addition, phosphorylated glucose can be condensed into glycogen to serve as the main energy reserve in the brain. Furthermore, glucose enters the synthetic pathways of three key neurotransmitters: glutamate, γ-aminobutyric acid (GABA), and acetylcholine, as well as that of a range of amino acids via TCA cycle intermediates. Finally, metabolism of glucose via the pentose phosphate pathway provides both ribose 5-phosphate for the synthesis of nucleotides and the reducing molecule nicotinamide adenine dinucleotide phosphate (NADPH) for lipid synthesis and antioxidant defense.
Glucose and Oxygen Delivery
Glucose is an extremely hydrophilic molecule, and its delivery to brain cells involves facilitated transport, which is tightly regulated in a cell- and region-specific manner by the glucose transporter proteins (GLUTs) of the solute carrier family 2 transport protein group.25,26 Many of the 13 known GLUT isoforms have been identified in brain but, in most cases, have not been recognized to have any defined role in glucose transport.27–30 Cerebral glucose uptake is predominantly mediated by GLUT1 and GLUT3. GLUT1 exists in two molecular weights of 45 and 55 kD as a result of differing extents of glycosylation. Glucose enters the brain through the 55-kD GLUT1 transporters of microvascular endothelial cells, which are variably distributed between the luminal and abluminal cell membranes, as well as a sizable intracellular pool, probably as a mechanism to modulate cerebral glucose uptake according to metabolic demands.26,31 The 45-kD GLUT1 is usually localized to glial cells, the choroid plexus, and the ependyma26 and has just limited expression in neurons, except in response to stress from conditions such as hypoxia or hypoglycemia.32,33 Neurons express the higher affinity, higher capacity GLUT3 transporter.34
At the average rate of global CBF (55 mL/100 g per minute), the oxygen extraction fraction (i.e., the proportion of oxygen extracted by the brain relative to the amount delivered to it in arterial blood) is approximately 0.5 (or 50%), as opposed to the glucose extraction fraction of about 0.1 (10%).10 Thus, the supply of glucose is typically far in excess of requirements, which leaves plenty of reserve for periods of high metabolic demand.
Energy Production from Glucose
Glycolysis is the main pathway for glucose metabolism (see Fig. 343-1) and takes place in the cytosol with the net formation of 2 equivalents of ATP and 2 equivalents of pyruvate from 1 equivalent of glucose:
Depending on the redox state of the cell, the resultant pyruvate can take one of two paths. Under normal aerobic conditions, pyruvate and the reduced form of nicotinamide adenine dinucleotide (NADH) are taken up by mitochondria, where their oxidation by the TCA cycle and respiratory chain provide for the vast bulk of ATP production (see Fig. 343-1). In the absence of oxygen, reoxidation of NADH through the respiratory chain is blocked and must instead occur by the reductive conversion of pyruvate to lactate by lactate dehydrogenase (LDH), or else glycolysis cannot continue.
Pyruvate enters the mitochondrial matrix to undergo stepwise oxidation in the TCA cycle to carbon dioxide and water, with much of the resulting release of energy made available to the mitochondrial respiratory chain as reducing equivalents. Usually, the pyruvate is converted to acetyl coenzyme A (acetyl CoA) by pyruvate dehydrogenase (see Fig. 343-1).
Acetyl CoA then enters the TCA cycle, where the first step involves its condensation with oxaloacetate to yield citrate. In the next few steps, citrate is isomerized and oxidatively decarboxylated to yield α-ketoglutarate, which in turn is oxidatively decarboxylated to form succinate. The remaining steps involve the regeneration of oxaloacetate from succinate (see Fig. 343-1). With each round of the cycle, two carbon atoms enter as acetyl CoA and two carbon atoms leave as CO2. Because an acetyl group is more reduced than CO2, the completed cycle involves four oxidation-reduction reactions that give rise to three molecules of NADH and one of reduced flavin adenine dinucleotide (FADH2). In addition, conversion of succinyl CoA to succinate involves the cleavage of an energy-rich thioester bond, which is coupled to the phosphorylation of guanosine diphosphate to form GTP. If the GTP is not used for protein synthesis or signal transduction, its γ-phosphate group can readily be transferred to ADP to form ATP.
The NADH and FADH2 from each of the preceding stages in the oxidation of glucose are used by mitochondria for the generation of ATP by oxidative phosphorylation (see Fig. 343-1). The driving force of oxidative phosphorylation is the electron transfer potential of NADH or FADH2 relative to O2. Electrons donated by NADH are passed sequentially down a “respiratory” chain of three large protein complexes embedded in the inner mitochondrial membrane, from NADH-ubiquinone (Q) reductase (complex I) to cytochrome reductase (complex III) to cytochrome-c oxidase (complex IV). Transfer of electrons between complexes I and III and between complexes III and IV is accomplished by reduced ubiquinone (QH2) and cytochrome c, respectively. In contrast to NADH, QH2 is the entry point for electrons from FADH2. At complex IV (cytochrome-c oxidase), the electrons are consumed in a reaction with oxygen, the final electron sink, to form water. This electron flow leads to translocation of H+ from the mitochondrial matrix to the intermembrane space, and the resultant concentration gradient creates a proton motive force or transmembrane electrochemical potential (Δψm) to drive an inner membrane–bound ATP synthase (complex V). In this fashion, oxidation of NADH and FADH2 theoretically drives the formation of 3 and 2 ATP molecules, respectively. On tallying up the energy yield from each stage of the metabolism of glucose, it can be seen that a molecule of glucose that undergoes complete oxidation may theoretically yield up to 38 molecules of ATP, the majority of which are contributed by oxidative phosphorylation.
In each of the stages of generation of ATP from glucose, energy supply is coupled to energy demand by enzymes whose activities are subject to feedback regulation by one or more downstream ionic or molecular species. The main site of regulation of glycolysis is at the level of phosphofructokinase (PFK), an enzyme that catalyzes the ATP-dependent addition of a phosphate group to fructose 6-phosphate early in the pathway (see Fig. 343-1). Increases in levels of ATP (a fundamental end product of glucose metabolism), citrate (the first molecule generated in the Krebs cycle), and H+ (which is produced in numerous downstream reactions) indicate a relatively robust energy supply and exert a negative feedback effect on PFK, which dampens further glycolysis. Conversely, increases in AMP, cyclic AMP (cAMP), ADP, K+, NH4+, and Pi tend to accompany a rundown of cellular energy and thereby stimulate the activity of PFK. At the stage of acetyl CoA synthesis, the pyruvate dehydrogenase complex is directly stimulated by increasing levels of its substrate pyruvate and inhibited by its end products acetyl CoA and NADH (see Fig. 343-1). It is also responsive to energy status, as shown by the [NADH]/[NAD+], [acetyl CoA]/[CoA] and [ATP]/[ADP] ratios. Increases in these ratios result in the phosphorylation and deactivation of pyruvate dehydrogenase. The rate of the TCA cycle is immediately dependent on the availability of the oxidized form of nicotinamide adenine dinucleotide (NAD+), which in turn is dependent on the availability of ADP and hence ultimately on the rate of utilization of ATP. The enzymes of the TCA cycle are also individually regulated. For example, the dehydrogenases of the TCA cycle are activated by Ca2+, which increases in concentration during the work of muscle contraction. The most important factor regulating the overall rate of oxidative phosphorylation is the level of ADP. As ADP levels rise (reflecting higher consumption or inadequate production of ATP), oxidative phosphorylation is stimulated; that is, the respiratory chain is activated by a need for ATP synthesis. Taken together, these complex and multiple regulatory mechanisms reflect the precise control of glucose metabolism in response to the prevailing cellular conditions.
Other Metabolic Fates of Glucose
Amino Acid and Neurotransmitter Synthesis
Glutamate can be converted back to α-ketoglutarate by oxidative deamination to undergo further oxidation in the TCA cycle for the purpose of energy generation35 or redirected toward the synthesis of other amino acids, GABA, fatty acids, or glutathione.
Glycerol Synthesis
New evidence indicates that glucose undergoes conversion to free glycerol within the brain. In theory, this could occur through reduction of the glycolytic intermediate dihydroxyacetone phosphate to glycerol 3-phosphate by glycerol-3-phosphate dehydrogenase, followed by dephosphorylation to glycerol by a phosphatase. The natural operation of this pathway is suggested by the presence of the necessary enzymes in the brain. Glycerol 3-phosphate has been demonstrated in astrocytes and neurons,36 as well as in the cytosol of oligodendrocytes.37 More importantly, it has recently been reported that 13C-labeled glycerol accumulates in the media of cultured astrocytes and cerebellar granule cells and in the brains of rats within 5 minutes after treatment with [U-13C] glucose.38
Storage as Glycogen
The brain converts a limited amount of glucose into glycogen to form its principal energy reserve. Although there is typically 3 to 4 times more glycogen than free glucose in the brain, it still amounts to no more than approximately 4 µmol/g, and were it to serve as the sole fuel source, it would be consumed completely in no more than a few minutes. Instead, more contemporary evidence suggests that it exists as a metabolic buffer system and is metabolized slowly, so complete turnover of brain glycogen stores normally takes 3 to 5 days.39
Glycogenesis requires the action of glycogen synthase on glucose subunits that have first undergone phosphorylation by ATP. Although both astrocytes and neurons possess the necessary enzymes, synthesis of glycogen is normally confined to astrocytes. Storage of glycogen is also almost exclusive to astrocytes and serves to augment neuronal energy requirements during periods of intense activity and pathologic shortages of glucose. Glycogen undergoes glycogenolysis by the enzyme glycogen phosphorylase to form lactate under stimulation by neurotransmitters such as norepinephrine, vasoactive intestinal polypeptide (VIP), histamine, serotonin, and certain metabolic by-products of neuronal activity such as K+ and adenosine.40 It has been hypothesized that this lactate is transferred from astrocytes to neurons for use as an energy substrate.41,42 In contrast to the metabolism of glucose, release of glucose equivalents from glycogen does not require prior “priming” with ATP.
Metabolic Contributions of Brain Structural Elements
Astrocytes
A growing body of research has resulted in a revision of the traditional view of astrocytes as a passive supporting act to neurons. The proliferation and development of astrocytes are indeed driven by trophic cues associated with neuronal activity, but the growth and survival of neurons are in turn dependent on astrocytes.43 In terms of metabolism, astrocytes form a highly active compartment that is separate from that of neurons but with which it interacts in a dynamic and essential fashion. This interaction occurs via the narrow extracellular space (ECS) and involves the shuttling of ionic and molecular metabolites and neurotransmitters such as lactate and glutamate. Despite being essentially nonexcitable, astrocytes have also been implicated in the integration of neuronal input, modulation of synaptic activity, and even long-range signaling. Neurotransmitter-evoked elevations in astrocytic calcium can trigger the release of chemical transmitters that can cause sustained modulatory actions on neighboring neurons. Astrocytes are also involved in brain water homeostasis and induction of the BBB (see review by Bennaroch5).
Before considering the influence of astrocytes on cerebral metabolism, it is relevant to consider their structural relationship to each other and to other cells in the brain. In the mammalian brain, neurons make up no more than 50% of the cerebral cortical volume and in most regions are outnumbered 10 : 1 by astrocytes.44 Each astrocyte typically defines a nonoverlapping three-dimensional domain and is polarized such that one or more astrocytic processes contact a capillary while many more are entwined within the neuropil and engage with hundreds to thousands of synapses. Where astrocytic processes abut capillaries, they are specialized into structures known as end-feet. These end-feet are so numerous that almost the entire surface of the capillaries is covered.45 The potential significance of this interposition of astrocytes between neurons and the capillary (endothelium) has been recognized since the late 19th century.44 It puts astrocytes in a special position to both sense neuronal signaling and capture glucose directly from the capillary, thereby permitting them to govern excitation-metabolism coupling. Another noteworthy feature of astrocytes is their ample connections with each other and the ECS through gap junctions and hemichannels composed mainly of connexin.43,46,47
As alluded to before, astrocytes play a leading role in the flux of glucose into neurons for energy. The glucose taken up by astrocytes may have one of two primary fates: it may be converted to lactate via astrocytic glycolysis, or it may be converted via glycogenesis to the glucose storage polymer glycogen. By contrast, in adult neurons, aerobic glycolysis results in the formation of pyruvate, not lactate, and glycogen metabolism and storage normally do not occur. That glycogen, the storage form of glucose, is located almost entirely in astrocytes is one indication of the dominant position of astrocytes in the metabolic processing of glucose. However, it should be noted that glycogenolysis in astrocytes is dictated by specific neurotransmitters and neuromodulators, thus giving neurons tight rein over this energy store.48 As elaborated on earlier, astrocytic glycolysis is also stimulated by neuronal activation, which leads to the production of lactate. It has been proposed that this lactate is secreted into the ECS and is taken up and oxidized by activated neurons as their main energy source. Notably, 1 molecule of lactate entering the TCA cycle to undergo oxidation can yield 17 ATP molecules under normoxic conditions, which is about half the energy production of aerobic glucose metabolism. Although controversial, the existence of astrocyte-neuron lactate shuttling is based on evidence. For instance, immunohistochemistry has identified a selective distribution of LDH isoforms, with neurons predominantly expressing LDH1, the form that is enriched in lactate-consuming tissues, and astrocytes expressing LDH5, the form that is enriched in lactate-producing tissues.6 Localization of the lactate monocarboxylate transporters MCT-1 and MCT-4 to astrocytes and MCT-2 to neurons demonstrates the capacity for lactate exchange between these cells.49 When primary neuronal cultures are in the presence of lactate and glucose, they preferentially consume lactate as their oxidative substrate.
The fidelity and safety of glutamate-mediated neurotransmission are dependent on very efficient uptake and modification of glutamate by astrocytes (Fig. 343-2). They keep the extracellular glutamate concentration very low by the rapid Na+-dependent GLT1 (predominant in the cortex and hippocampus) and GLAST (predominant in the cerebellum) cotransporter-mediated removal of the neurotransmitter from the synapse, thereby enabling quick termination of glutamate signaling before excitotoxic neuronal injury can occur.50 Astrocytes can handle this influx of glutamate in several different ways. They possess the necessary aminotransferases to transfer the α-amino group of glutamate to oxaloacetate or pyruvate to yield α-ketoglutarate and either aspartate or alanine. The resultant α-ketoglutarate can be oxidized for energy metabolism in the TCA cycle. Indeed, cultured astrocytes have been reported to use glutamate as an energy substrate even in the presence of glucose.51 Glutamate can also be converted into α-ketoglutarate by glutamate dehydrogenase–catalyzed oxidative deamination. However, the most common means by which astrocytes inactivate glutamate is by conversion to glutamine through the attachment of ammonium ions (see Fig. 343-2). This last reaction is endergonic and requires glutamine synthase, an enzyme limited to astrocytes, and serves the additional important function of removing ammonia.52
Being electrically “inert,” the glutamine can then be safely released to neurons for recycling into glutamate by neuronal mitochondrial glutaminase. This cycling between glutamine and glutamate is commonly referred to as the glutamine-glutamate shuttle (see Fig. 343-2). The sodium that is cotransported into astrocytes during the uptake of glutamate (glutamate/Na+ ratio, 1 : 3) stimulates the activity of Na+,K+-ATPase, which depletes intracellular ATP and in turn stimulates PFK, the principal rate-limiting enzyme of glycolysis.53 The resulting net production by glycolysis of 2 molecules each of ATP and lactate per molecule of glucose would in theory supply enough energy for astrocytic uptake (1 ATP for the glutamate transporter) and conversion (1 ATP to drive amidation) of 1 molecule of glutamate to glutamine, thus leaving lactate in surplus of requirements.54 Herein lies one possible explanation for the well-documented early rise in lactate in cerebral tissue during cortical activation, as occurs in seizures.55,56 More importantly, this hypothesized stimulation of glycolysis by glutamate provides a mechanism for the observed coupling of excitation and metabolism in the brain. Cortical activation causes rapid glutamatergic signaling, which increases the glutamine/glutamate flux and therefore drives the increased astrocytic consumption of glucose by glycolysis. This logic is supported by evidence of coupling of the glutamate/glutamine shuttle to glucose energetics in the cerebral cortex in vivo.57,58
Astrocytes are capable of long-range signaling and buffering functions through the use of their gap junctions to form a large intercellular network, or syncytium. For example, increases in the extracellular K+ concentration secondary to neuronal activity leads to the entry of K+ into astrocytes through strong inwardly rectifying K+ (Kir) channels.59,60 The resulting local depolarization is propagated electrotonically through the glial cell network via the gap junctions, which leads to the efflux of K+ at distant cell processes that are not experiencing the elevated K+ concentration. The high density of K+ channels on astrocytic end-feet allows K+ to be deposited in the perivascular space, where it can be recycled when neural activity ceases. This so-called spatial buffering of K+ is important because even modest efflux of K+ from neurons can lead to considerable changes in the concentration of K+ in the ECS, with potentially detrimental effects on maintenance of neuronal membrane potential, activation and inactivation of voltage-gated channels, synaptic transmission, and electrogenic transport of neurotransmitters. The gap junctions of astrocytes also contribute to the propagation of intercellular Ca2+ waves, probably by enhancing the release of ATP, as well as by providing an intercellular pathway. Astrocyte membrane depolarization by glutamate causes the mobilization of Ca2+ from the endoplasmic reticulum via the inositol triphosphate (IP3) generated by the activation of metabotropic glutamate receptor 5.61 It has previously been thought that IP3, Ca2+, or both are propagated across gap junctions to create a “Ca2+ wave.” More contemporary evidence suggests that the Ca2+ wave is propagated through the paracrine actions of ATP released from astrocytes on purinergic receptors.62 Release of glutamate from astrocytes has been associated with this calcium wave. Taken together, it is clear that an increase in neuronal depolarization is coupled to an increase in astrocytic depolarization, which in turn influences local metabolic and electrical activity via K+, H+, and Ca2+ ions.
Finally, yet another distinct role of astrocytes in cerebral metabolism is regulation of the development and function of the endothelial cells of the BBB. Astrocytes have important influences on the BBB, which plays a crucial role in cerebral metabolism (see later). First, contact of astrocytic foot processes with endothelial cells of the BBB upregulates the formation of tight junctions in the latter, mainly by inducing the production of occludin, an integral protein of tight junctions.63,64 Second, astrocytes can also induce the expression in endothelial cells of membrane transporters and specialized enzymes such as γ-glutamyl transpeptidase.65,66 Astrocytes submitted to hypoglycemic conditions may release factors that increase glucose uptake through the BBB.67
Blood-Brain Barrier
The BBB is a protective structure formed by capillaries to restrict the exchange of solutes between the brain and blood and thus to ensure a chemically controlled intracerebral milieu for optimal cellular performance. Morphologically, the BBB is composed of a monolayer of specialized capillary endothelial cells surrounded by a thin basal lamina and closely invested by the foot processes of astrocytes and cells known as pericytes. Unlike endothelial cells elsewhere in the body, those of the BBB are characterized by specialized regions of circumferential intercellular contact known as tight junctions or zonula occludens, minimal pinocytotic activity, and the virtual absence of fenestrations, all of which combine to necessitate that molecules passing across the BBB take a transcellular rather than a paracellular route. Consequently, free diffusion through an intact BBB is limited to lipid-soluble substances such as CO2, O2, ethanol, and lipophilic drugs or to very small polar molecules with a radius of less than 0.8 nm.68 Passage of even the smallest charged molecules (i.e., inorganic ions) is severely restricted, so transendothelial electrical resistance, which is typically 2 to 20 Ω•cm2, can be increased to greater than 1000 Ω•cm2.69 Hence, ions are transported by channels or by active means, such as via Na+,K+-ATPase.70 Transport of ions (particularly Na+) is coupled to the obligatory flow of water via osmotic forces, and both the luminal Na+ transporter and abluminal Na+,K+-ATPase are implicated in the secretion of extracellular fluid by brain capillaries.68,70 There are specific transport systems for the transcellular traffic of small lipophilic nutrient and amino acid molecules across the BBB. For example, glucose and neutral amino acids are transferred by the GLUT1 and L-amino acid transporters, respectively. Large hydrophilic proteins such as insulin, transferrin, and insulin-like growth factor may be taken up by a saturable receptor-mediated transcytosis mechanism, whereas others, particularly polycationic proteins, may cross the BBB via a nonspecific, non–receptor-mediated process referred to as adsorptive transcytosis. The BBB also has active mechanisms for excluding potentially harmful substances from the brain. Physiologically expressed carriers such as P-glycoprotein actively transport lipophilic molecules across the BBB and out of the brain. In addition, a metabolic barrier is provided by a combination of intracellular and extracellular enzymes: ectoenzymes such as peptidases and nucleotidases for the degradation of peptides and ATP, respectively, and intracellular enzymes such as monoamine oxidase and cytochrome P-450 for the inactivation of neuroactive and toxic compounds.71 Finally many, if not most of the features of the BBB are dynamic. For instance, during hypoglycemia, upregulation of GLUT1 and MCT transporter expression allows transport of glucose and ketone bodies into the brain to be increased.72
Cerebral Blood Flow
Hemodynamics
Here, r refers to vessel radius, ΔP to pressure gradient, η to the coefficient of fluid viscosity, and L to vessel length.73 In practical terms, L and η can usually be regarded as effectively constant, and the implication of the Hagen-Poiseuille law is that blood flow not only varies proportionally with the pressure gradient but also with the fourth power of the vessel radius. This provides an explanation for the clinical observation of the large change in blood flow that can occur with only small changes in vessel diameter. However, it only approximates real life because contrary to the key assumptions behind the Hagen-Poiseuille law, normal blood flow is not continuous but pulsatile, and blood vessels are not rigid and branchless tubes. In addition, if the rate of flow is continuously increased, there comes a point when resistance to flow increases sharply and the flow ceases to be laminar, instead forming a turbulent pattern. The situation in the brain is made even more complex by the operation of cerebrovascular autoregulation (discussed in detail later), the possible presence of arterial stenosis, and the diameter and extent of arterial collaterals.74 The intracranial arteries of the circle of Willis represent the naturally existing site of collateral blood flow for the cerebral circulation. It should be noted, however, that when a major cerebral artery undergoes gradual occlusion, the extracranial arteries can also provide important collateral supply to the cerebral circulation via the ophthalmic, meningeal, and leptomeningeal arteries.
Under normal physiologic conditions, blood flow is regulated in the brain through changes in vascular resistance. By combining Equations 10 and 14, the significant relationship between vessel radius r and CVR is revealed:
Indeed, the resistance of the cerebral circulation is subject to dynamic changes in the contractile state of vascular smooth muscle (VSM), most of all at the level of the penetrating precapillary arterioles; that is, the principal cerebral resistance vessels are those that arise perpendicularly from the pial arteries on the brain surface before penetrating the parenchyma. However, up to 50% of total CVR arises from smaller pial arteries (150 to 200 µm in diameter) and arteries of the circle of Willis.73,75
Hemorheology
The composition and environment of blood confer on itself complex and anomalous viscous properties.76,77 Importantly, by being composed of a concentrated suspension of cells within proteinaceous plasma, blood is a particulate fluid. Furthermore, many of these cells are capable of altering their shape and forming physical interactions with each other or the glycocalyx on the endothelial wall.78,79 From the foregoing, it is intuitive that blood viscosity is not only a function of plasma viscosity but also depends on the concentration of cells, one measure of the latter being the hematocrit. Less obvious is that such factors as the deformability and aggregability of erythrocytes and the adherence or nonadherence of leukocytes to the endothelium80–82 can lead to marked deviations from Newton’s law. However, in blood vessels, where the internal diameter is very large in comparison to the size of the cells, blood of normal hematocrit approximates Newtonian behavior reasonably well.77
The non-Newtonian behavior of blood is best demonstrated in its passage through the microcirculation. In large arteries, where the shear rate is low, calculations using Poiseuille’s equation yield an “apparent” viscosity that is much higher than expected. This has been explained by the tendency of erythrocytes to form clumps or rouleaux at low shear rates, thereby increasing resistance to flow. With the higher shear rates typically found in the microvasculature, the apparent viscosity falls because any cell aggregates are dispersed into single cells that stretch and align themselves with the axial and fastest moving laminae of the bloodstream, thus leaving a slower, cell-depleted zone of plasma along the vessel margin. This marginal zone is thought to progressively dilute the hematocrit as the caliber of the blood vessel is reduced and may be accentuated by plasma skimming or cell screening at branching points. As a result, when blood flows through progressively diminishing arterioles or capillaries with a diameter of 300 µm or smaller, there is a linear fall in apparent viscosity known as the Fahraeus-Lindqvist effect. Eventually, however, when blood reaches capillaries with a diameter that is less than that of an erythrocyte (6 to 8 µm), a steep rise in blood viscosity and an inversion phenomenon (i.e., reversal of the Fahraeus-Lindqvist effect) take place.83,84 The anomaly represented by the Fahraeus-Lindqvist effect is of critical importance in counteracting the adverse influence of blood vessel geometry (number, length, and diameter) on resistance to microcirculatory blood flow.
Relationship between Cerebral Blood Flow and Intracranial Pressure
CBF and ICP are related by the Monro-Kellie doctrine, the modern version of which states that because the intracranial space is fixed and its principal components, the brain parenchyma, blood, and CSF, are nearly incompressible, any change in the volume of one component must lead to a reciprocal change in volume of the other components or ICP must rise. Cerebral blood volume (CBV) takes up a significant proportion of intracranial volume. At any point in time, changes in CBV are determined by changes in arterial inflow relative to venous drainage and are therefore reliant on CBF. If intracranial compliance is low, a rise in CBF can increase ICP. However, as ICP rises, there must be a compensatory rise in MAP or CPP will fall (see Equations 11 and 12), with a deleterious effect on CBF.
Regulation of Cerebral Blood Flow
Unlike other organs, regulation of blood flow in the brain is distinguished by the influence of astrocytes and neurons. Extracerebral blood vessels receive a rich “extrinsic” supply of perivascular fibers from the parasympathetic (mainly the sphenopalatine, otic, and internal carotid) and sympathetic (mainly from the superior cervical) ganglia, as well as the sensory roots of the trigeminal ganglia. On entering the brain parenchyma, cerebral arteries lose this ganglionic nerve supply and instead acquire “intrinsic” innervation from parenchymal neurons. The best characterized intrinsic neural pathways that project to cortical blood vessels are those from the nucleus basalis, locus caeruleus, and raphe nucleus. With electrical or chemical stimulation of these areas, increases or decreases in cortical CBF occur. Anatomic studies have shown that neurons in these areas send projection fibers to cortical blood vessels, as well as to astrocytes. In fact, noradrenergic afferents from the locus caeruleus target mainly perivascular astrocytes. Changes in perivascular astrocytic [Ca2+]i secondary to noradrenaline cause vasoconstriction of the adjacent arterioles.85 Hence, arterial tone is influenced by astrocytes, as well as by neurons.
Major Mediators
Nitric Oxide
Nitric oxide (NO) is a ubiquitous cellular messenger that was referred to as endothelium-derived relaxing factor (EDRF) on its discovery and remains known as one of the principal mediators of vasodilation. With regard to this vasodilator function, NO is the mediator for the action of mechanical stimuli and a large variety of agonists on the endothelium. The latter include acetylcholine, bradykinin, oxytocin, histamine, endothelin-1 (ET-1 via ETB receptors; see later), and prostaglandin F2α (PGF2α). Apart from vascular actions, NO signaling has been suggested or established in a wide variety of other physiologic functions, including neurotransmission, behavioral inhibition, prevention of platelet and neutrophil aggregation, promotion of gastrointestinal motility, and the immune response. Moreover, alterations in the NO system are implicated in the pathogenesis of many diseases as diverse as Alzheimer’s disease, cerebral vasospasm, pyloric stenosis, and nephrogenic diabetes insipidus.86–88 In terms of its vascular actions, which involve extensive crosstalk with a variety of other vasoactive systems, it is clear that the NO signaling system is a major mediator and possibly part of a “final common pathway” of vascular modulation.
Being a short-lived and freely diffusible gas, NO cannot be stored and must be synthesized close to its site of action by nitric oxide synthase (NOS) from L-arginine. There are actually three isoforms of NOS, each encoded by distinct and highly conserved genes: neuronal/type I (nNOS), inducible/type II (iNOS), and endothelial/type III (eNOS). The endothelial and neuronal isoforms are constitutively expressed in cerebral blood vessels, the latter occurring in the nerve plexus in the outer adventitial layer.89–91 Recently, constitutive expression of a posttranslationally modified variant nNOS isoform, nNOS-α or mtNOS, has been identified in the mitochondria (mt) of brain and other organs.92 By contrast, expression of iNOS is induced in cells, including macrophages, endothelial cells, and neurons, under specific conditions such as inflammation, trauma, and infection86 and appears to have no role in normal cerebrovascular function. The enzyme is active when dimerized. The catalytic reaction requires molecular oxygen and NADPH in addition to the cofactors of FAD, flavin mononucleotide, iron protoporphyrin IX, and tetrahydrobiopterin (BH4). Once formed, NO diffuses within or between cells, thus permitting it to function as both an autocrine and paracrine messenger. In its most common redox state, NO possesses an unpaired electron, which renders it highly reactive, particularly with metalloproteins containing iron or thiol groups. Indeed, much of NO signaling relies on avid binding of NO to the heme moiety of soluble guanylate cyclase, thereby leading to activation of the enzyme, followed by increased levels of cyclic guanosine monophosphate (cGMP). In VSM cells, cGMP in turn activates protein kinase G, which causes relaxation by opening K+ channels or decreasing the sensitivity of the contractile machinery to Ca2+.
NO has an important role in the regulation of cerebrovascular tone under normal, basal conditions, as shown by the constrictive effects of nonspecific NOS inhibitors on resting cerebral arteries both in vitro and in vivo, accompanied in the latter case by a reduction in CBF.93–96 The individual blockade of eNOS and nNOS causes a decrease in vascular tone, thus suggesting a tonic influence of both isoforms.97–99 This effect is confirmed in the former case by the phenotype of modest systemic hypertension in mice with targeted deletion of eNOS.100 This may not merely be due to a simple loss of vasodilatory influence inasmuch as eNOS-null animals demonstrate cerebral arteriolar hypertrophy that has been attributed to the lost inhibition of VSM proliferation,101 thus echoing previous hypotheses that eNOS-derived NO is a negative regulator of vascular remodeling.102,103 In contrast, tonic input is supplied to forebrain arteries by the nitroxidergic parasympathetic postganglionic fibers arising from the pterygopalatine ganglion.104 This is evidenced by ipsilateral cerebral vasoconstriction on denervation or ablation of this ganglion or its preganglionic afferents in dogs and monkeys.105–107
By and large, evidence suggests that the NO synthesized by nNOS is one of the mediators in the coupling of CBF to synaptic activity. Selective nNOS inhibitors such as 7-nitroindazole have been shown in animal models to inhibit increases in CBF induced by neuronal activity.108–111 Curiously, nNOS-null mice have an intact hyperemic response to hypercapnia112 and neural activation108,113,114 in the cerebral cortex but not in the cerebellum.115 However, unlike their wild-type brethren, the cerebral cortical hyperemia in mutant nNOS mice is not altered by pharmacologic NO inhibition, which provides a clue that normalcy can be maintained, at least in the supratentorial compartment, by the compensatory activity of NO-independent pathways. In light of the evolutionary conservation of NOS genes, such redundancy is noteworthy.
Regulation of NOS isoforms in the context of the cerebral circulation is extremely complex and occurs at multiple levels.116 In general, transcriptional regulation governs cell- and tissue-specific expression of all isoforms.117 In addition, eNOS transcription can be upregulated by shear stress on endothelial cells as a result of the presence in the promoter region of a shear stress responsive element.118,119 The occurrence of posttranscriptional control mechanisms, such as alterations in messenger RNA splicing and stability, is well described.120 Apart from the availability of substrate and cofactors, the activity of the enzyme itself is regulated by a variety of protein-protein interactions, as well as by phosphorylation. Binding of calmodulin is essential for the electron transfer function of all NOS isoforms,121 but eNOS and nNOS do so in a manner that is reversible and dependent on [Ca2+]i, whereas iNOS does so avidly and in an essentially Ca2+-independent manner.122 Ca2+-calmodulin has the additional important function of competing with caveolins in the allosteric modulation of eNOS and nNOS activity. Another apparent regulator of eNOS, with which it colocalizes, is the 90-kD heat shock protein (Hsp90).123 Better known as a molecular chaperone, Hsp90 enhances the activation of eNOS, possibly by facilitating the competitive displacement of caveolin-1 from eNOS by Ca2+-calmodulin.124,125 As eNOS is regulated by phosphorylation on serine and, in specific situations, on tyrosine and threonine residues,126,127 it is intriguing that Hsp90 has also been reported to assist and potentiate the phosphorylation of eNOS by the serine/threonine kinase Akt.128
Eicosanoids
Eicosanoids are a diverse group of 20-carbon mediators that are ubiquitously produced by the regulated oxygenation of arachidonic acid. Because arachidonic acid is sequestered at the sn-2 position of glycerophospholipids, it must be enzymatically released from membrane phospholipids before the synthesis of eicosanoids. This occurs principally through the action of phospholipases of the A2 variety (PLA2).129–131 Many isoforms of PLA2 are represented in the mammalian central nervous system, with wide expression in many cell types, especially astrocytes.132–134 After mobilization, arachidonic acid can be converted to eicosanoids by one of three major metabolic routes, namely, the cyclooxygenase (COX), lipoxygenase, or epoxygenase (a cytochrome P-450 monooxygenase) pathways. The metabolites generated by COX are also known as prostanoids. The COX pathway was the first to be elucidated and remains the best studied, but each pathway generates products with opposing vasomotor properties. For example, the two COX isoforms (constitutive COX-1 and inducible COX-2) produce potent vasoconstrictors such as PGF2α and thromboxane (TXA2), as well as potent vasodilators such as prostaglandin I2 (PGI2, prostacyclin) and prostaglandin E2 (PGE2). The relative concentrations of these prostanoids affect basal vascular tone by altering the balance between VSM contraction and relaxation. One of the important factors that determines the types of eicosanoid that are synthesized is the cell type that is under consideration. For instance, under normal conditions, endothelial cells tend to favor the release of vasodilatory eicosanoids, whereas aggregating platelets tend to favor the release of vasoconstrictors.131
Eicosanoids have been proposed to be one of many substances that modulate blood vessel diameter in response to neural activity. In rat cortical slices, vasodilation in response to neuronal stimulation can be blocked by the presence of the COX inhibitor acetylsalicylic acid.135 In further in vivo experiments, it has been demonstrated that this response is probably mediated by release of the potent vasodilator PGE2 from astrocytes after discharge of glutamate from neurons.136 More recently, epoxygenase products, possibly epoxyeicosatrienoic acids, have also been implicated in the vasodilation of cortical arterioles after astrocytic α-amino-3-hydroxy-5-methyl-4-isoxazolepropionic acid (AMPA) receptor stimulation.137 Yet, another study has proposed that under physiologic conditions, vasoactive neuronal projections govern the release of arachidonic acid by astrocytes, which is metabolized to the vasoconstrictor 20-hydroxyeicosatetraenoic acid (20-HETE) by cytochrome P-450 Ω-hydroxylase in smooth muscle cells.85
Endothelium-Derived Hyperpolarizing Factor
Apart from NO and prostanoids, there is at least one other endothelium-dependent dilator of cerebral vessels, referred to as endothelium-dependent hyperpolarizing factor (EDHF). For instance, agonists such as acetylcholine, UTP, and ATP continue to dilate cerebral arterioles after the complete inhibition of NOS and COX enzymes.138,139 In isolated vessels, the action of EDHF can be characterized by resistance to NOS and COX inhibition, the presence of an intact endothelium, hyperpolarization of VSM cells by approximately 15 mV, and blockade by inhibitors of calcium-activated potassium (KCa) channels.140
The identity and mechanism of action of EDHF remain speculative. In the rat middle cerebral artery, EDHF-mediated vasodilation is preceded by endothelial hyperpolarization caused by the stimulation of intermediate-conductance Ca2+-activated K+ channels (IKCa) by agonist-induced increases in endothelial cell Ca2+.141 It is not yet clear how endothelial hyperpolarization is then transformed into VSM cell hyperpolarization and vasorelaxation, but it is hypothesized to involve the transmission of a substance from the endothelium to VSM. Many studies, mainly on coronary blood vessels, have concluded that this substance is an epoxyeicosatrienoic acid derived from the metabolism of arachidonic acid by cytochrome P-450 epoxygenase in endothelium.142,143 Conversely, this conclusion has been disputed by other studies.144,145 K+ has found some favor as the putative EDHF substance inasmuch as K+ released into the ECS during endothelial hyperpolarization by IKCa channels has been shown to mediate VSM cell relaxation by activating barium (Ba2+)-sensitive Kir channels and particular Na+,K+-ATPase isoenzymes.146 Other suggested candidates for EDHF include C-type natriuretic peptide, lipoxygenase products, hydrogen peroxide, and anandamide.140 An alternative line of investigation has implicated the passage of electrical current through myoendothelial gap junctions in generation of the EDHF response.147,148 If electrical current is the transmitted “substance,” EDHF would not be an actual “factor” but a process. Part of the reason for the lack of consensus on EDHF is likely to be natural variation in the biologic mechanisms of vasodilation between different sexes, different vascular beds, and even different stimuli on the same vessel.149–151 Therefore, it is important that no conclusions about the nature of EDHF in the cerebral circulation be based on studies in the peripheral circulation.
EDHF appears to complement endothelium-derived NO in controlling the cerebral circulation. First, EDHF becomes more important than NO in dilation as cerebral vessel diameter decreases. For example, EDHF has been found to have a more prominent role than NO in vasodilation after luminally applied ATP in isolated penetrating arterioles, but the opposite was true in the middle cerebral artery.152 Second, in some pathologic states, the EDHF response is upregulated, whereas the NO response is compromised.153
Endothelins
The endothelium also produces a family of three 21–amino acid isopeptides known as endothelins. Of these, ET-1 is regarded as being the most relevant to the cerebral circulation. It is synthesized as a 203–amino acid form, which is first cleaved into a 39–amino acid intermediate (“big ET-1” or proendothelin) before proteolysis by ET-converting enzyme (ECE) into the 21–amino acid active form.154 Circulating concentrations of ET-1 are normally low, and it is not stored in cells but is synthesized on demand in response to a variety of factors, including phorbol esters, thrombin, and epinephrine. There are two receptors for ET-1: ETA receptors predominate in smooth muscle and are sensitive to ET-1 and ET-2,155,156 whereas ETB predominates in endothelium and is sensitive to all three endothelin isoforms.157–160 In vitro, ET-1 is known to have potent vasoconstrictive effects on human cerebral arteries and a strong growth-promoting action on cultured fibroblasts and VSM cells.160,161 The latter action can bring about long-term vascular changes. In vitro experiments have revealed that low concentrations of ET-1 can act on endothelial ETB receptors to cause vasodilation through the mediation of NO.158,162,163 However, when the endothelium is removed or disrupted or ET-1 is injected into the subarachnoid cisterns, ET-1 produces profound and long-lasting vasoconstriction via a direct interaction with VSM ETA receptors, possibly through the activation of a combination of signal transduction pathways, including phospholipase C (PLC)/IP3, Rho/Rho kinase, and extracellular signal–regulated kinase-1 (ERK1) and ERK2.161,164,165 Direct inhibition of NO signaling may also be involved.159 In vivo, the intravascular effect of ET-1 is rather variable and may cause vasoconstriction or vasodilation, depending on the species and vascular territory involved.157
Evidence has accumulated for a functional link between the synthesis and activity of NO and ET-1. In human brain microvascular endothelial cells, ET-1 secretion has been shown to be stimulated by angiotensin II, bradykinin, the calcium ionophore A23187, dopamine, norepinephrine, phorbol esters, serotonin, thrombin, and vasopressin.157 Many of these agents are linked to NO-mediated relaxation, thereby providing circumstantial evidence of a balancing mechanism between ET-1–mediated vasoconstriction and NO-mediated vasodilation. Like the NO gene, the promoter for the ET-1 gene has a shear stress responsive element, but unlike the NO gene, activation of this element decreases transcription.166 In an acute setting, endothelium-derived NO has been shown to inhibit the release of ET-1 in porcine aorta whereas inhibition of NO production has been shown to potentiate the constrictive effect of this agent.167 This manner of interaction between NO and ET-1 has been demonstrated in a variety of preparations, including human vascular tissue168 and cultured rat VSM cells.169 In a chronic setting, treatment of quiescent rat VSM cells with NO donors leads to upregulation of (vasoconstrictive) ETA receptor expression and an increase in the sensitivity of ET-1 for this receptor, effects mimicked by cGMP but attenuated in the presence of the NOS inhibitor L-nitroarginine methylester (L-NAME).165 Together, these findings support the notion of physiologic antagonism between ET-1 and NO.
ET-1 does not appear to have a substantial role in CBF except in pathologic states. Topical application of ET-1 to cerebral arteries in vivo causes significant vasoconstriction, an effect reversed in the presence of the selective ETA receptor antagonist BQ-123.157 However, topical application of ET receptor antagonists alone does not alter the diameter of cerebral vessels in vivo, thus suggesting that ET does not contribute to maintenance of basal cerebrovascular tone.158,170,171 Furthermore, because ET-mediated constriction is long lasting, it seems unlikely that ET contributes to the fine temporal regulation of CBF.157 Instead, it is likely that the vasoconstrictive action of ET-1 is considerably augmented in pathologic conditions such as cerebral ischemia and post–subarachnoid hemorrhage (SAH) vasospasm, where a defect in NO signaling is known to occur.
Adenosine
Adenosine is an endogenously produced purine nucleoside generated from the action of either 5′-nucleotidases on AMP or specific hydrolases on S-adenosylhomocysteine. Its physiologic activities in the brain include modulation of neuronal and synaptic activity and regulation of CBF.172 Based on microdialysis measurements in freely moving, unanesthetized animals, the best estimate of the free concentration of adenosine in the brain is 50 to 300 nM.173 The level of adenosine increases sharply and relatively rapidly in the ECS during increased physiologic neuronal activity and with seizures, hypoxia, and ischemia. Intracellularly, breakdown of AMP, a product derived from ATP and ADP hydrolysis during increased neuronal “work,” and the metabolism of S-adenosylhomocysteine and other molecules lead to the formation of adenosine, followed by its transport outside the cell via a nucleoside transporter.172 Additionally, adenosine may be formed extracellularly from ectoenzyme-mediated catabolism of ATP acting as a neurotransmitter. After binding of adenosine to N-methyl-D-aspartate (NMDA) receptors, the excitatory neurotransmitter glutamate can also elicit the release of adenosine.
At cell surfaces, adenosine can act via two classes of P1 (purinergic) receptors, A1 and A2.172,173 Activation of Gi/o-coupled A1 receptors on neurons inactivates adenylate cyclase and decreases cAMP levels, thereby reducing influx through Ca2+ channels and increasing efflux through K+ channels and causing hyperpolarization. The A1 receptors are therefore inhibitory; they are present presynaptically and function as negative feedback “autoreceptors” to decrease the release of glutamate and other neurotransmitters.174 Postsynaptically, A1 receptors also decrease neuronal excitability.172 Gs protein–coupled A2 receptors, in contrast, are present in both VSM and endothelial cells of cerebral blood vessels and elicit cAMP-mediated vasodilation via stimulation of adenylate cyclase. Stimulation of A2a receptors can also lead to the release of NO from endothelium and astrocytes,175,176 probably through the calcium-insensitive p42/44 mitogen-activated protein kinase (MAPK) pathway.177 Note that through these combined actions on A1 and A2 receptors, the adenosine that is released during neural activity can help minimize the energy deficit from CBF-metabolism mismatch. Hence, adenosine has been implicated not only as a mediator of flow-metabolism coupling but also as a neuroprotective agent in the setting of ischemia.178–181
Potassium Ions
Potassium ions (K+) are among the most potent vasodilatory signals in the brain. Evidence suggests that K+ is a key mediator of neurovascular or flow-metabolism coupling.182 K+ is released from neurons during physiologic neuronal activation and in large amounts during seizures, hypoxia, and ischemia.183 Excessive perineuronal K+ accumulation is prevented by its uptake into local astrocytes, followed by its shunting toward the perivascular foot processes of astrocytes, where release into the ECS occurs, probably through slow conductance (see K+ spatial buffering, earlier). Elevation of perivascular K+ in the range of 3 to 15 mM leads to hyperpolarization of the VSM cell membrane via increased conductance of Kir channels, particularly Kir2.1 and Na+,K+-ATPase, in the VSM cells of pial arteries.184,185 This facilitates smooth muscle relaxation and vasodilation through the closure of voltage-gated calcium channels (VGCCs). Above a perivascular concentration of approximately 15 mM, K+ is a vasoconstrictor in that excessive accumulation of this cation leads to depolarization-induced activation of smooth muscle VGCCs.186
Hydrogen Ions
As described earlier, the well-known vasodilatory action of CO2 is mediated mainly through the action of H+ on cerebral arteries.187 The origin of H+ ions includes local CO2 production from oxidative metabolism of glucose in neurons (followed by CO2 metabolism in and H+ extrusion from astrocytes) and production of the metabolic intermediate lactate. Active, healthy cells, in addition to energetically compromised cells, can lead to the production and extracellular accumulation of H+. Acidification of the ECS by the local accumulation of H+ results in vasodilation and reduced neuronal excitability. The precise mechanism of H+-induced vasodilation is not well understood, but attention has focused on the potential role of activation of ATP-sensitive potassium (KATP) channels or facilitation of NO release (or both). It is well established that openers of KATP channels produce dilation of cerebral blood vessels and that this response is inhibited by specific KATP blockers such as glibenclamide. In this context, it is of interest that cloned ATP has been found to possess a pH-sensitive site.188 A role for NO is suggested by the ability of NOS inhibitors to attenuate the vasodilation elicited both by moderate hypercapnia and by topical application of acidic CSF.158,189–191
Cerebral Autoregulation
The brain maintains its regional and total blood flow (and therefore perfusion) at a fairly constant rate over a wide range of systemic blood pressure by a vasomotor phenomenon known as cerebral autoregulation. Autoregulation involves the rapid and sustained constriction or dilation of cerebral resistance vessels, principally the precapillary arterioles, purely in response to changes in transmural pressure caused by variations in CPP. Recall that in normal individuals, CPP varies directly with MAP because ICP is constant. In effect, the brain is protected from fluctuations in perfusion over a MAP range of 60 to 150 mm Hg (Fig. 343-3). Beyond these limits, CPP passively follows MAP. It is important to note that the limits of autoregulation are by no means invariable. For example, they may be right-shifted in chronic hypertension (associated with increased sympathetic tone) and in states associated with increased renin release; conversely, a left shift may be observed in sleep, “physiologic hypotension” in athletes, “pathologic hypotension” associated with hemorrhage, and the presence of angiotensin-converting enzyme inhibitors, prolonged hypoxemia, or hypercapnia.192,193 Furthermore, autoregulation of CBF may be disturbed in acute, severe hypotension or hypertension and profoundly impaired or even abolished in severe cerebral ischemia, arteriovenous malformation beds, or brain injury and after aneurysmal SAH.73
Mechanisms
The precise mechanism of cerebral autoregulation is not known. Proposed mechanisms include intrinsic changes in VSM tone (myogenic hypothesis) and the release of a variety of vasoactive substances from the endothelium (endothelial hypothesis) or periadventitial nerves (neurogenic hypothesis) in response to changes in transmural pressure. In contradistinction to general cerebrovascular regulation, evidence argues against the involvement of “metabolic” or “humoral” factors in cerebral autoregulation. First, as measured by microdialysis, the extracellular and perivascular concentrations of H+, K+, and adenosine, key mediators in metabolic vasoregulation, normally do not change in response to alterations in CPP in the autoregulatory range.10,194–197 Second, the time course of autoregulation is too rapid to depend on the generation of metabolic products.192,194–197 Third, the autoregulatory response has been observed in isolated, perfused vessels in vitro (i.e., not subject to the influences of neuroglial metabolism).10,198,199 Taken together, these findings suggest that metabolic factors are unlikely to play a major role in autoregulation.
The “myogenic hypothesis” of autoregulation accords a key role to the inherent response of VSM to mechanical stimulation. Arterioles typically exhibit a state of partial contraction that is dependent on intraluminal pressure; an increase in pressure leads to increased contraction, whereas a decrease in pressure leads to decreased contraction. It is clear that this is a phenomenon that resides in the smooth muscle cell and involves the obligatory influence of Ca2+.200 It is currently understood that myogenic contraction follows a sequence of depolarization of VSM cells, entry of Ca2+ through L-type VGCCs, facilitation of “calcium-induced calcium release” from the sarcoplasmic reticulum, Ca2+-dependent activation of myosin light-chain phosphorylation, and finally, actin-myosin–based contraction.201 However, the mechanosensor and signaling mechanisms for pressure-induced VSM cell depolarization are uncertain. The possible transduction mechanisms have been covered thoroughly elsewhere.202 As in other excitable cells, VSM cell membrane potential is a consequence of ion gradients, and patch-clamp investigations on VSM cells have revealed the presence of stretch-activated channels (SACs) whose deformation causes depolarization by allowing the influx of cations.203,204 Evidence has emerged that certain members of the diverse superfamily of mammalian homologues of the Drosophila transient receptor potential channel (TRP), including TRPC6, TRPV2, and TRPM4, share the biophysical properties of SACs. Downregulation of the expression of these TRP genes by antisense oligonucleotides leads to a significant attenuation in pressure-induced VSM cell depolarization and thus myogenic constriction.205–208 It has been suggested that VSM integrins can also serve as mechanotransducers through their physical associations with the extracellular matrix, cytoskeleton, and protein tyrosine kinases at cell structures known as dense plaques. The most compelling evidence for this hypothesis is that peptides containing integrin-specific amino-acid sequences are potently vasoactive. Furthermore, integrin signaling regulates VSM [Ca2+]i, the primary trigger for contraction, by altering L-type Ca2+ current.209,210
An “endothelial hypothesis” for cerebral autoregulation is supported by evidence that the endothelium can respond to physical stimuli such as pulsatile stretch and shear stress.198,211,212 It is clear that the endothelium is by no means a passive and inert barrier between the blood and VSM but rather has a critical vasomodulatory role through the synthesis, elaboration, and release of EDRFs such as NO, PGI2, and EDHF, as well as endothelium-derived contracting factors (EDCFs) such as ET-1 and TXA2. An increase in flow rate and shear stress without an increase in transmural pressure can induce vasodilation, most likely through the endothelial release of NO.198 It has been said that endothelium-dependent arteriolar contractions can be demonstrated in response to increased transmural pressure. This has been considered to be due to depression of EDRF release or an increase in EDCF release (or both).198,213,214 Yet some studies have found that disruption of the endothelium does not impair transmural pressure–induced arteriolar constriction.215
The “neurogenic hypothesis” of cerebral autoregulation attributes the resistance of cerebral blood vessels to release of neurotransmitters from perivascular nerve fibers.192 Before discussing the “neurogenic hypothesis,” it is important to appreciate that perivascular nerve fibers of diverse origin arborize throughout the adventitial layer of cerebral arteries and release a wide variety of neurotransmitter substances.10,90,94,216–218 The sources of cerebrovascular innervation may be either extrinsic (i.e., from remote neurons) or intrinsic (i.e., involving local neurons) and are detailed in Table 343-1. There is a wealth of morphologic and functional evidence supporting an active role for perivascular nerves in the regulation of vascular tone.219 Apart from the dense innervation of cerebral arteries, the presence of specific receptors for neurochemical transmitters such as biogenic amines and peptides on vascular endothelial and smooth muscle cells has been widely established by both pharmacologic and immunochemical means.192,194,220–222 Furthermore, nerve stimulation studies have demonstrated a functional correlation between electrical stimulation of isolated arteries (even those subject to mechanical de-endothelialization) and altered vasomotor tone.223,224 During such studies, the inhibitory effects of specific receptor antagonists suggest that the vasomotor effects of nerve stimulation are mediated by release of neurotransmitters from adventitial nerve fibers. Isometric force recording of isolated cerebral arteries exposed to a variety of neurotransmitters such as acetylcholine, calcitonin gene–related peptide (CGRP), diethylamine NONOate (an NO donor), norepinephrine, serotonin, and substance P has provided additional functional evidence of the importance of nerves in regulating vasomotor function.94,194,220,222,225 Certainly, the time course of neurotransmitter release, binding, and action could be comparable to the relatively rapid time course of autoregulation.
The function of extrinsic and intrinsic innervation of cerebral arteries and arterioles is poorly understood. The innervation of extracerebral arteries by sympathetic nerve ganglia probably serves to shift the upper limit of the autoregulatory curve to higher pressures. This is believed to be partly mediated by neuropeptide Y and would serve to protect the brain against hypertension secondary to sympathetic activation.216 Strangely, although stimulation of the parasympathetic ganglia elicits strong vasodilation via acetylcholine-mediated endothelial-dependent release of NO, it does not seem to play any role in autoregulation or any other physiologic cerebrovascular response. The trigeminovascular system releases the powerful vasodilator CGRP in the setting of disturbed cortical activity but again has no known role in autoregulation.226 If there are mechanisms for triggering neurotransmitter release from intrinsic perivascular nerves in the setting of increased transmural pressure, they have not been determined. Although conjectural, the presence of nonspecific SACs in perivascular nerve fibers, akin to those found in VSM cells, could provide the local Na+– and Ca2+-mediated depolarization and Ca2+ entry required for release of transmitters from perivascular nerve terminals.
Regulation of Cerebral Blood Flow by Blood Gases
Carbon Dioxide
CO2 is considered to be the most important physiologic variable in chemoregulation. The arterial partial pressure of CO2 (PaCO2) exerts profound effects on CBF, particularly across the physiologic range of 30 to 50 mm Hg (Fig. 343-4). In mammals of all ages, hypercapnia (increased PaCO2) is found to cause cerebral vasodilation, whereas hypocapnia causes the reverse. In fact, inhalation of 5% to 7% CO2 is associated with an almost exponential increase in CBF of 50% to 100%, thus rendering CO2 one of the most potent vasodilatory influences on the cerebral circulation.227 When alterations in PCO2 have been sustained for 3 to 5 hours, there is an adaptive return of CBF toward baseline levels.
It is most likely that extracellular acidosis rather than intracellular acidosis or molecular CO2 itself is the major determinant of hypercapnic hyperemia.187,228,229 A direct effect of CO2 on brain vessels has been disproved by experiments showing that manipulations of PCO2 are not vasoactive when the pH of CSF is kept constant. The “pH hypothesis” of hypercapnic vasodilation states that the actions of CO2 on CBF are mediated by the effects of H+ on VSM. When PaCO2 increases, molecular CO2 but not HCO3− or H+ diffuses across the BBB. Perivascular PCO2 rises, which leads astrocytes to convert the CO2 to HCO3− and H+ via carbonic anhydrase, thereby increasing the extracellular or perivascular concentration of H+ (i.e., there is a fall in local pH).227 This is thought to cause cerebral vasodilation via a sequence of effects on VSM cells consisting of increased potassium conductance, increased K+ efflux, hyperpolarization, closure of VGCCs, and reduced cytosolic Ca2+, a process culminating in relaxation.230 The exact mechanism of increased potassium conductance has not been resolved and may involve the opening of KATP or KCa channels231,232 or increased H+/K+ exchange in VSM cells.179,233–235 The adaptation of cerebrovascular tone to hypercapnia is associated with a gradual return of CSF pH and HCO3− ion concentration toward normal values.236,237
There is no consensus on the mechanism or mechanisms linking changes in extracellular pH to cerebral VSM tone, and some research has even suggested that there may be significant differences between adults and neonates. Prostanoids, NO, and cyclic nucleotides have all been implicated in mediation of the aforementioned changes in plasma membrane calcium and potassium conductance, but the literature is confusing. Inhibition of prostanoid synthesis by indomethacin has been found to abolish the vasodilatory response to hypercapnia in newborn pigs but not in adult humans.238 Some studies have reported that NOS inhibition significantly attenuates the vascular reactivity to hypercapnia in adult animals.158,239–241 Yet this effect of NOS inhibition has been disputed in newborn animals and adult humans.96,242 Data comparing newborn and adult animals are insufficient to make any firm conclusions about possible differences in the mechanism of vascular responsiveness. Suggested explanations for the inconsistent data in adults are that NO may have a permissive role in facilitating the action of other mediators243,244 or that multiple redundant pathways may interact in CO2-induced vasodilation.245–247 Alternatively, it could well reflect the variable specificity and other pitfalls of pharmacologic NOS blockade.116
Oxygen
Arterial oxygen is another important determinant of CVR and hence CBF. Elevated inspired oxygen concentrations elicit CVR and decrease CBF. Conversely, a fall in PaO2 results in vasodilation. It is generally held that the response of CBF to PaO2 is a threshold phenomenon that is evident only when PaO2 falls below the normal physiologic range (Fig. 343-5), although some research suggests otherwise.248
Hypoxia is thought to elicit vasodilation by both direct effects on cerebral resistance vessels and indirect effects on nonvascular components. It has been proposed that hypoxia elicits VSM relaxation by inhibiting sarcoplasmic Ca2+ uptake and stimulating the production of EDRF over EDCF. Hypoxia also causes alterations in cellular metabolism that lead to increased generation and release of the vasoactive tissue factors K+, H+, and adenosine. The influence of K+ on CBF is discussed later in the context of flow-metabolism coupling. Cortical CBF has been shown to increase with increasing H+ concentration or acidity over a PaO2 range of 40 to 300 torr (1 torr ≅ 1 mm Hg).248 Likewise, increasing blood flow has been seen after the infusion of increasing concentrations of adenosine or adenosine analogues into the brain.249 The preponderance of evidence suggests that arterial chemoreceptors do not contribute to hypoxic vasodilation because it persists after the deafferentation of cranial nerves IX and X. However, it has been proposed that hypoxia-induced stimulation of oxygen-sensing neurons in the rostral ventrolateral medulla may increase CBF via neurogenic vasodilation.193,250 There is an overall consensus that NO signaling is probably not involved in mild to moderate hypoxia-induced vasodilation inasmuch as NOS inhibitors do not attenuate vasodilation in this setting.251,252 However, NO signaling may be involved in severe hypoxia (PaO2 <35 mm Hg) because the NOS inhibitor L-NAME has been found to attenuate vasodilation in these circumstances.191
Cerebral Neurovascular Coupling
Maintenance of cerebral homeostasis depends on the dynamic regulation of oxygen and glucose supply to match changes in metabolic demand. Since the enunciation of this essential concept by Roy and Sherrington more than a century ago,253 it has become well established that increased cerebral activity is accompanied by rapid (within 1 to 2 seconds) and regionally specific increases in oxygen and glucose uptake and metabolism.11,254 The oxygen and glucose for this purpose must be delivered by increased blood flow at the capillary level, hence the designation functional hyperemia or neurovascular coupling.
Mechanisms
Despite much research, exactly how increased neuronal activity triggers enhanced capillary blood flow remains conjectural. Any proposed mechanism or mechanisms must be capable of inducing rapid vasodilation along the lines of what is observed in vivo and have a clearly defined site of action within the vasculature that can account for the effects of neuronal activity on the cerebral circulation. Originally, Roy and Sherrington proposed that blood flow through the microvasculature increases under the local influence of a buildup of metabolites from a relative energy deficit. Such metabolites, primarily K+, H+ (largely related to lactate and CO2 levels), and adenosine, can affect VSM tone directly or indirectly through altered neurovascular transmission (see later). However, this theory is clearly inadequate in that it fails to anticipate any effect on the upstream resistance vessels or arterioles, an omission that goes against basic hemodynamic principles and laboratory observations in vascular beds throughout the body.255 The ability of functional activity to elicit coordinated changes in the precapillary resistance arterioles, as well as the microvessels in a simple model of the cerebral circulation, suggested that the vascular response must somehow be retrogradely propagated. This prompted speculation that the metabolic and electrical signals for vasodilation may be conducted upstream through gap junctions between endothelial cells or between endothelial and VSM cells or through flow-mediated vasodilation.256–258 More recent investigations have suggested several potential mechanisms whereby synaptic activity may be incorporated as a trigger for neurovascular coupling.
The rich innervation of cortical microvessels by several neurotransmitter systems suggests that perivascularly released neurotransmitters are important in adaptations of flow to neuronal activity. Somatosensory cortical hemodynamic responses have been shown to correlate with local field potentials, thus implying that vascular changes reflect the incoming neuronal input and local processing in a given area.259 GABA interneurons are candidates for such a neural processing role because they provide a particularly rich innervation to microvessels. In this context, it has been of interest that selected activation of single cortical GABA interneurons can not only increase but also decrease the diameter of neighboring microvessels through the expression of VIP, NOS, and somatostatin.260,261 This provides a plausible mechanism for the redistribution of perfusion to activated neuronal networks to occur over a backdrop of stable perfusion to the entire brain.
Through painstaking research, a picture has gradually emerged of astrocytic end-feet serving as individual vasoregulatory units through neurotransmitter-evoked Ca2+-dependent signaling events. It has been demonstrated that arteriolar vasodilation occurs in a similar time frame to the rise in astrocytic [Ca2+]i induced by glutamatergic neuron activity or the application of metabotropic glutamate receptor (mGluR) agonists.135 Depending on the resting state of the arteriole, similarly evoked astrocytic increases in [Ca2+]i have also been found to be temporally related to vasoconstriction. However, arteriolar tone is affected by increases in astrocytic [Ca2+]i only when it is large and propagates into end-feet.85 Currently, it is thought that binding of mGluR by glutamate activates PLC and generates IP3, which not only promotes cytosolic release of Ca2+ from the endoplasmic reticulum but also causes a separate, spatially restricted rise in [Ca2+]i through Ca2+ release channels associated with end-feet IP3 receptors. Hence, the spatially restricted liberation of IP3 within an astrocyte end-foot has been linked to the production of a localized large-amplitude [Ca2+]i signal that immediately precedes vasodilation of an approximately 30-µm segment of arteriole within a cortical slice.262 Although most experimental work has been done with glutamate, it is probable that other neurotransmitters such as GABA, somatostatin, and VIP may likewise produce vasoactive effects through the generation of astrocytic Ca2+ signals, as well as direct innervation of vessels.260,262 In light of the evidence for a specialized function of the end-foot in neurovascular coupling, it is of interest that the local interneurons that so richly innervate cerebral arterioles make extensive contacts with end-feet.216
It is probable that astrocytic Ca2+ signals cause vasodilation through mediators that bring about alterations in Ca2+ signaling processes in arteriolar VSM cells. Studies on brain slices have found that induction of synaptic activity leads to an increase in astrocyte [Ca2+]i, followed closely (<1 second) by the inhibition of spontaneous VSM cell [Ca2+]i oscillation and arteriolar vasodilation.263 The leading candidates in mediation of this process are K+ and arachidonic acid metabolites. Elevation of astrocytic [Ca2+]i can lead to Ca2+-dependent activation of PLA and liberation of arachidonic acid, which is subject to metabolism by a host of enzymes in astrocytes and endothelial cells to form vasoactive substances.264 Alternatively, a rise in astrocytic [Ca2+]i might activate end-foot big conductance Ca2+-sensitive K+ (BKCa) channels and lead to the release of K+ into the ECS and VSM cell depolarization as a result of the activation of Kir channels.265
One aspect that has been neglected in the account of the astrocytic vasoregulatory unit just presented is the responsiveness of cerebrovascular tone to the dynamic metabolic state of the local brain environment. Therefore, it is of interest that a mechanism whereby metabolites can modulate the vasomotor influence of astrocytes has been newly revealed in the rat brain.266 When oxygen availability is low and astrocyte [Ca2+]i is elevated, astrocyte glycolysis is maximized, thereby leading to extracellular accumulation of lactate and adenosine. This in turn can attenuate transporter-mediated uptake of the vasorelaxant PGE2 from the ECS and block astrocyte-mediated constriction, both of which facilitate vasodilation.
Clinical Measurement of Cerebral Blood Flow and Metabolism
Historical Background
Measurement of CBF and metabolism in humans is invaluable for understanding brain function in normal and abnormal states and, in clinical practice, has found diagnostic and prognostic applications in many areas of medicine. Although the theoretical groundwork was laid by Fick in the latter half of the 19th century, it was not until 1945 that measurement of CBF was first described. In this groundbreaking publication, Kety and Schmidt applied the Fick principle to determine global CBF in conscious human subjects by measuring the cerebral uptake of inhaled nitrous oxide, an inert and freely diffusible gas, through repeated chemical analysis of arterial and jugular venous blood samples.267 These CBF values, when combined with measurements of cerebral arteriovenous differences in glucose and oxygen concentration, permitted a crude determination of some parameters of cerebral metabolism as well.268 This early technique is a far cry from the sophistication of the methods available today.
Clinical Techniques
Currently, it is possible to use numerous techniques with a variety of underlying principles and unique sets of advantages, limitations, and indications for the clinical measurement of CBF and metabolism.254,269–280 At best, laser Doppler flowmetry, transcranial Doppler, and electroencephalography provide qualitative assessment of CBF. Quantitative CBF measurement techniques can be divided into two principal groups that use either a diffusible or nondiffusible tracer and rely on entirely different mathematical models. The former group involves calculation of the uptake of inert and highly diffusible tracers by the brain via some modification of the Fick equation. Because these tracers are freely diffusible in the brain parenchyma, the CBF values obtained reflect cerebral perfusion. The remaining techniques are based on the central volume theorem and require the construction of a time-density curve after the injection of nondiffusible contrast agents. Because these agents are confined to the vasculature, the values obtained reflect intravascular flow rather than perfusion.
The ideal clinical technique should be based on widely available and relatively inexpensive technology, be noninvasive, not require anesthesia, and permit accurate and reproducible measurements with a high degree of spatial and temporal resolution. Stable xenon–enhanced computed tomography (Xe-CT) comes closest to meeting these criteria for CBF, but its availability is constrained by equipment and gas requirements. Positron emission tomography (PET) has the benefit of allowing both CBF and metabolic measurements but has largely been confined to the research setting because of the requirement for an in-house cyclotron. By comparison, the equipment and materials needed for CBF measurement by single-photon emission computed tomography (SPECT) and intracarotid 133Xe studies are relatively accessible to clinicians, thus making these the current modalities of choice in most centers.280 This status quo will inevitably be challenged in due course by the emergence of new techniques. Of these, computed tomographic perfusion (CTP) imaging has emerged as a promising means of rapidly evaluating cerebral perfusion in various clinical scenarios, although the accuracy, reproducibility, and reliability of the quantitative results are still in question.281,282 There is also keen interest in extending the clinical applications of magnetic resonance imaging (MRI) to quantitative physiologic imaging.283 However, at the present time, MRI is not routinely used for perfusion imaging because of the difficulty of obtaining absolute quantification of perfusion parameters and comparing even relative MRI perfusion data between centers as a result of the lack of standardization of MRI units.284 Furthermore, CT-based assessment of cerebral perfusion offers several significant advantages over MRI in that the technology can simply be added to existing routine CT scanners in centers that do not possess MRI, is less time-consuming, is not contraindicated in patients with ferromagnetic implants, and allows safer imaging of patients with respiratory and hemodynamic instability.
Computed Tomographic Perfusion Imaging
The basic principle underlying CTP imaging is that the standard iodinated contrast material is an intravascular tracer, which allows the CT scanner to be a detector of brain blood flow. The concept itself is not new, but the high-speed continuous data acquisition that is essential for the detection of cerebral tracer kinetics has become possible only with modern helical or spiral CT scanners incorporating slip ring technology. Two imaging techniques have been developed; they differ distinctly in the volume of brain coverage and the data obtained. The slow-infusion technique can provide high-resolution whole-brain CT angiography and mapping of CBV but not mean transit time or CBF. Voxel-by-voxel subtraction of the unenhanced attenuation values from the enhanced attenuation values provides the CBV map of the entire brain. In contrast, the first-pass technique allows quantitative determination of CBV, mean transit time, and CBF, but in only a limited region. There is a linear relationship between the concentration of the contrast agent and the degree of attenuation. The attenuation data for each voxel in the scanned area and the progressive changes in density in regions of interest overlying a selected input artery and input vein are the data required for the “deconvolution algorithm” to make contrast agent time-concentration curves for each voxel. The software then generates color-coded parametric CBF, CBV, and mean transit time maps. It is possible to combine both slow-infusion and first-pass techniques in one sitting.284
The advantages of CTP imaging are that it does not require any special equipment, is fast, and barring any contrast reactions, is well tolerated. It requires only dedicated postprocessing software. However, even with a multidetector CT unit, CTP using the first-pass technique differs from Xe-CT in not providing whole-brain CBF coverage; in a typical protocol, only two 10-mm cerebral CT slabs are examined. This is mainly because the kinetics of iodinated contrast material is much quicker than that of stable xenon, as well as the necessity of limiting radiation exposure to acceptable levels. In any event, uncertainties exist about how quantitative CBF values should be obtained with CTP imaging because the results can vary markedly depending on such variables as the choice of input artery and size of the arterial region of interest.281 At present, the accuracy, reliability. and reproducibility of quantitative CTP imaging remains to be thoroughly established.
Single-Photon Emission Computed Tomography
SPECT determines the three-dimensional distribution of a single gamma ray–emitting radiotracer in the body. Hence, SPECT machines are designed to detect single gamma rays (photons) and determine their point of origin from their trajectory. Most modern SPECT studies use the radioisotope technetium 99m attached to a carrier that easily crosses the BBB, is distributed in the brain in proportion to regional CBF, and is temporarily retained there, thus allowing detection of the radioisotope anytime within the subsequent few hours. A variety of such carriers are in clinical use, each with its own advantages and disadvantages. The most commonly used SPECT brain blood flow tracer is technetium 99m-hexamethyl-propyleneamine oxime (99mTc-HMPAO [Ceretec]), a lipid-soluble macrocyclic amine. It is extracted on first pass by the brain, where it becomes fixed for several hours by conversion to a hydrophilic compound in the presence of intercellular glutathione. With 99mTc-HMPAO, CBF quantification is not straightforward, and a semiquantitative approach is generally used in which counts in a region of interest are determined and compared with those in an analogous region in the opposite, presumably normal hemisphere.285 By contrast, 133Xe-SPECT is ideally suited for quantitative CBF determination with the inert gas clearance technique. The rapid clearance of 133Xe from the brain has the advantage of allowing repeated studies within a short interval but unfortunately requires dynamic instrumentation, which has the significant disadvantage, when combined with the low energy of the emitted photons, of rendering poor spatial resolution of the resulting images. Also to be considered are the same concerns about the adverse effects of xenon inhalation that were mentioned for Xe-CT.
Positron Emission Tomography
The type of information that can be obtained with PET relies on the radiotracer that is administered. PET can be used to study regional cerebral glucose utilization through the use of intravenous [18F]fluorodeoxyglucose (FDG). Active brain regions take up FDG as though it were glucose. Once inside the cell, it is phosphorylated by hexokinase into FDG 6-phosphate, which is not a substrate for glucose transport and cannot be metabolized by phosphohexose isomerase, the enzyme catalyzing the next step in glucose metabolism. Thus, labeled FDG 6-glucose becomes trapped within the cell and contributes to a pattern of radioactivity that reflects local glucose uptake and metabolism. 15O can be inhaled for assessment of oxygen metabolism. The most commonly used tracer for PET CBF imaging is radiolabeled water (H215O) because it distributes freely in blood. This tracer has a very short half-life (≈2 minutes), so a bolus injection provides a snapshot that can be repeated, if desired, every 12 to 15 minutes.286
Magnetic Resonance Imaging and Spectroscopy
Two approaches can be used for the measurement of CBF with MRI. Akin to first-pass CTP imaging, the more established approach involves administration of the paramagnetic agent gadolinium and is variously referred to as dynamic susceptibility contrast, first-pass, or bolus perfusion MRI. Of note, the contrast agent remains wholly intravascular and only indirectly alters MRI signal intensity, thus hindering absolute quantification of hemodynamic parameters. The other MRI approach for imaging CBF is called arterial spin labeling and uses radiofrequency pulses to “tag” arterial blood water molecules, thereby obviating the need for exogenous contrast agent. Changes in the amplitude of the MRI signal can be used to construct quantitative images of cerebral perfusion, although there are still outstanding issues with absolute quantification.287
Cerebral Ischemia
Global versus Focal Cerebral Ischemia
Cerebral ischemic disorders fall into two broad categories: global and focal.288–290 A global insult, such as occurs in cardiac arrest, affects CBF to all brain areas similarly. However, if blood flow is not restored promptly, the cell damage seen in global ischemia does not affect all regions uniformly as a result of inherent differences in vulnerability between neuronal populations. In rodents, such populations are found in the hippocampus, particularly the CA1 sector; the neocortex (layers 3 and 5-6); and the caudoputamen.291,292 Global ischemia is usually dense, or complete; hence, the duration of ischemia that is compatible with survival is relatively brief. For example, the longest period of experimental cardiac arrest that can be sustained with good neurological recovery is 12 minutes.293 Focal cerebral ischemia is a distinctly different entity that occurs when an artery supplying a region of the brain is occluded, most commonly from thrombosis in situ or thromboembolism, and it typically produces a less severe but more sustained insult than that caused by nonfatal global ischemia.289,290,294,295 If prolonged, it will produce a contiguous mass of damaged cells, designated an infarct, in the territory of the occluded artery. An infarct is not homogeneous; there is a centrifugal gradation from dense ischemia in a so-called core area that is supplied solely by the occluded artery to less dense ischemia in the periphery because of supplemental blood supply from collateral vessels. Focal ischemia is sometimes permanent, but spontaneous lysis of an occluding thrombus may lead to reperfusion of an occluded artery. A dramatic illustration of such reperfusion is provided by cases of transient ischemic attack. Experiments in animals suggest that it is possible for short-lived focal ischemia to produce subtle lesions in which there is only selective loss of individual neurons.296–298 Although it has a duration and heterogeneity of flow disturbance that results in a more complex lesion than seen with global ischemia, focal ischemia represents the dominant pattern in human disease. Accordingly, the following discussion is confined to focal cerebral ischemia.
Flow Thresholds and the Origin of the Concept of the Penumbra
As CBF and tissue oxygen levels decline, cellular functions fail in a sequence that is determined by their relative energy requirements and biologic significance. Before the 1970s, cell death was thought to be a relatively sudden and inevitable consequence of focal cerebral ischemia, even though the phenomenon of cerebral transient ischemic attack had been described and suggested the existence of a critical level or threshold of reduction in CBF for cell death to occur.299 Only when techniques for quantitative regional CBF measurements became available could the relationship between CBF values and brain cell function be properly investigated. In a groundbreaking paper, Symon and coauthors reported their observations of three zones of flow disturbance in a baboon model of middle cerebral artery occlusion (MCAO): a peripheral zone of only mildly reduced blood flow without discernible adverse cellular effect; an intermediate zone of moderate reduction of blood flow to below 20 mL/100 g per minute, in which an isoelectric electroencephalogram and absent evoked potentials indicated “electrical failure”; and an inner “core” zone of severe reduction of blood flow to below 6 to 10 mL/100 g per minute, in which the signs of “electrical failure” were accompanied by a dramatic increase in the extracellular K+ concentration, indicative of “membrane failure.”300 Crucially, Symon and colleagues also found that when the flow values or thresholds for these failures were recrossed by prompt elevation of blood pressure, the tissue in the intermediate zone could be rescued, thereby ultimately resulting in a smaller infarct. Hence arose the original concept of the “ischemic penumbra” as a region of potentially salvageable brain tissue that is perfused in a narrow range between the thresholds for electrical impairment and morphologic integrity. The upper and lower of these principal flow thresholds have accordingly become known as the “penumbral” and “infarction” thresholds, respectively.301
Falling CBF levels have now been correlated with biochemical and genetic events as well, which has led to the description of “molecular penumbras.” Biochemical changes are observed well before ATP levels are significantly reduced. Protein synthesis begins to fail at less than 50 mL/100 g per minute, tissue lactate levels start to rise at 35 mL/100 g per minute, neurotransmitter release and disturbance of energy metabolism occur at approximately 20 mL/100 g per minute, and finally, terminal depolarization and concomitant massive potassium efflux occur at 6 to 15 mL/100 g per minute (Table 343-2).295 Microarray analysis has revealed that focal ischemia is accompanied by the upregulated or downregulated expression of dozens of genes, including those for heat shock proteins, antioxidative enzymes, RNA metabolism, inflammation, and cell signaling, as well as immediate early genes.302 Among these genes, those whose products appear to distinguish reversible from irreversible ischemic injury are said to define molecular penumbras. Examples include Hsp70 and hypoxia-inducible factor-1 (HIF-1).303,304
ISCHEMIC THRESHOLD | RATE OF CBF (mL/100 g/min) | NEUROLOGICAL MANIFESTATION |
---|---|---|
Normal | 50-60 | No deficit |
First | 25-30 | Mild to moderate deficit from electrical impairment |
Second | 16-20 | Severe deficit from electrical failure |
Third | 10-12 | Profound deficit from pump failure and cytotoxic edema |
Fourth | <10 | Impending death from gross metabolic failure |
Further Characterization of the Ischemic Penumbra
The concept of the penumbra has undergone some revision and refinement since it was originally formulated. It is generally accepted that in focal ischemia there is a peripheral area of the initial lesion that normally becomes part of the final infarct but may be prevented from doing so by reperfusion or pharmacologic intervention. However, this area may not necessarily be electrically silent, nor may it have completely preserved ion homeostasis.290 It is also a dynamic entity. The penumbra has maximal volume at the onset of ischemia, after which it progressively shrinks with time. This time dependency was emphasized in a classic study on awake macaques, in which the infarction threshold rose from approximately 5 mL/100 g per minute for 2-hour MCAO to about 18 mL/100 g per minute for permanent MCAO.305 This result reflects the rise in infarction threshold toward the penumbral threshold with increasing duration of flow disturbance as the penumbral area is progressively subsumed into the irreversibly damaged core.306 The penumbra’s precarious existence is further manifested as electrophysiologic instability. Despite the critical decline in perfusion in the penumbra, its level of local glucose metabolism tends to be maintained at normal levels, which results in marked elevation of the local glucose metabolism/blood flow ratio—a sign of metabolism-flow uncoupling.307 This leads to metabolic stress manifested as repetitive displays of so-called peri-infarct depolarizations and is associated with a need for ATP to restore the membrane potential. When tissue ATP is completely depleted, the penumbra becomes irreversibly depolarized and indistinguishable from the infarction core.
Modes of Neuronal Death in Ischemia
The occurrence of at least two distinct modes of cerebral ischemic death has been described: apoptosis and necrosis (Fig. 343-6). Apoptosis is triggered in response to a wide range of physiologic and pathologic stimuli. The morphology of apoptosis, which is best demonstrated by transmission electron microscopy, is typified by cell shrinkage, chromatin condensation, nuclear compaction, plasma membrane blebbing, disorganized cytoplasmic organelles, and finally, cellular fragmentation into smaller membrane-bound vesicles containing cytoplasmic organelles with or without nuclear fragments.308 These vesicles, or apoptotic bodies, are gradually removed by phosphatidylserine-directed phagocytosis with minimal evidence of damage or inflammatory change in the surrounding tissue.309 There are two classic pathways to apoptosis, those initiated by either mitochondria (intrinsic pathway) or cell surface “death” receptors (extrinsic pathway), and both are distinguished by the sequential activation of proteases known as caspases.308 Another of the unique identifying features of apoptosis is double-stranded DNA cleavage at internucleosomal sites to yield a laddered pattern of DNA on gel electrophoresis, with each band in the ladder differing in size by about 180 base pairs.310 In contrast, necrosis is a dissipative form of cell death triggered by extreme stress in the form of depletion of ATP or membrane damage. It has the characteristic morphologic features of cellular swelling, formation of extensive cytoplasmic vacuoles, distended or disrupted mitochondria, dilation of the endoplasmic reticulum, and eventual lysis of the cell membrane (see Fig. 343-6). The resultant release of cellular contents into the intercellular space damages neighboring cells and elicits an accompanying inflammatory response (a feature of necrosis, not apoptosis; Fig. 343-7).308,311 Autophagy is a third form of cell death that has been described in cerebral ischemia. It is a pathway of degradation that involves the encirclement of damaged proteins and organelles by a membrane-delimited structure, an autophagosome, the contents of which are then degraded by fusion with a lysosome.312 Like apoptosis, autophagy occurs in physiologic situations, involves ordered processes and a stereotypical progression toward cell elimination, and is regarded as a form of programmed cell death. However, autophagy is believed to promote cell survival in many scenarios, and the question of whether autophagic activity is the cause of death or an attempt to prevent it is still unresolved.313 The distinct differences between these three modes of cell death suggest that ischemic cell death follows mechanistically stereotypical patterns.
However, on closer scrutiny, there does not appear to be such a clear delineation of ischemic cell death into these stereotypical patterns. In this regard, comparatively more data are available on necrosis and apoptosis in ischemic brain than on autophagy. Dying neurons can exhibit composite characteristics of necrotic and apoptotic morphologic features.314 The necrosis and apoptosis pathways can share effector molecules such as cathepsins and caspases.315,316 Stimuli that are typically associated with necrosis can induce the apoptotic phenotype and vice versa.317–321 A cell may switch between apoptosis and necrosis even when it appears to have committed to one particular fate. For instance, cells with apoptotic morphology may undergo lysis and culminate in a phenotype more typical of necrosis. Such secondary necrosis has been observed in the liver and also in in vitro models.322 It seems that the intensity of the insult is one determinant of the final mode of death, with more severe insults favoring necrosis over apoptosis.323 Certainly, a number of studies have reported a greater prominence of necrotic morphology in the densely ischemic core than in the less ischemic penumbra. Other determinants of the type of cell death appear to be factors such as mitochondrial function, the availability of ATP, and the integrity of effectors of apoptosis such as caspases.317,321,324 In other words, the final form of a cell’s death depends on the physiologic context at the time of the death signal.
There is emerging consensus that degenerate cell death of all causes is represented by a spectrum of patterns of programmed and nonprogrammed cell death, sometimes involving novel pathways.312,325,326 For instance, in one study it was noted that the DNA fragments seen on gel electrophoresis of rat ischemic neurons differed in size from those seen in archetypal apoptosis and, rather than being blunt ended, had staggered ends with a 3′ recess of 8 to 10 nucleotides. This suggests that the endonucleases that produce DNA damage in ischemic neurons are different from those of classic apoptosis.327 A further example is provided by paraptosis, a nonapoptotic programmed cell death that superficially resembles necrosis but is characterized by the presence of cytoplasmic vacuolation. It was observed in a third of fibroblasts that had been induced to overexpress the human insulin-like growth factor 1 receptor and was found to be mediated by a previously unknown functional site of caspase-9. Degenerate neural cell death with morphologic similarities to paraptosis has been reported, thus suggesting the particular relevance of this form of death in the central nervous system.328
Mechanisms of Ischemic Brain Injury
A great deal of our knowledge about the pathophysiology of stroke is derived from experimental research on animals and cell cultures. Such research has revealed that cerebral ischemic injury is the culmination of an extremely complex process involving a cascade of biochemical pathways evolving over a period of many hours or even days. Much remains to be elucidated, but it is clear that the elaborate interaction between these pathways of the ischemic cascade can lead to many potential routes to brain cell death.294,329–332 Certain observations are worth noting. First, in contradistinction to the initial events, later events in the ischemic cascade may progress unabated even when CBF and the bioenergetic state of the cell improve.294 Second, more irreversible cellular damage may be caused by the occurrence of postischemic reperfusion, especially if delayed, than by the ischemic event itself.333 Hence, therapeutic interventions aimed purely at reperfusing acutely ischemic brain may be doomed to fail. Third, the different tempo and responses to the neuroprotective strategies of cell death in the infarct core than in the penumbra indicate that the underlying mechanisms are certain to be different.329
Regulation of Cellular Calcium
[Ca2+]i is tightly regulated within narrow limits by multiple mechanisms.334 Under normal conditions, entry of Ca2+ into the cell from the ECS is favored by a 10,000-fold higher extracellular calcium concentration ([Ca2+]e) than [Ca2+]i and a negative resting cell membrane potential. One determinant of [Ca2+]i is the balance between passive influx and active extrusion across the plasma membrane. Ca2+ enters cells by voltage-sensitive and agonist-operated calcium channels. At the level of the plasma membrane, the extrusion mechanisms consist of ATPase-driven export of Ca2+ and a 3Na+-Ca2+ (NCX) exchanger that indirectly derives its energy from the Na+ gradient. Because the NCX is electrogenic and carries 3 Na+ (in) for 1 Ca2+ (out), it is driven not only by the Na+ gradient but also by the membrane potential. In some circumstances, such as collapse of the Na+ gradient and membrane depolarization, Ca2+ translocation by NCX can be reversed and cause cell calcium loading.
Other regulatory mechanisms control movement of Ca2+ between the endoplasmic reticulum and the cytosol and between mitochondria and the cytosol.335,336 The endoplasmic reticulum is the main compartment for Ca2+ storage in the cell. Sequestration of free cytosolic Ca2+ within the endoplasmic reticulum depends on sarcoplasmic-endoplasmic reticulum Ca2+-ATPase (SERCA), whereas release back to the cytoplasm depends on signaling mediated by ryanodine and IP3 receptors.337,338 The calcium-binding proteins calreticulin and calnexin enhance this buffering capacity of the endoplasmic reticulum.339 Mitochondrial influx of calcium is driven principally by a uniporter (i.e., by the mitochondrial membrane potential Δψm), which being negative inside, exerts electrophoretic drag on the positively charged calcium ion.335,336,340–342 The rate of influx through the uniporter is proportional to [Ca2+]i, which has a large capacity (Vmax). Conversely, the normal efflux pathway for mitochondrial calcium involves Na+-dependent and Na+-independent secondary active transporters, which are substantially slower than the uniporter.343 Hence, the intramitochondrial calcium concentration ([Ca2+]m) can acutely reach excessive levels if [Ca2+]i is elevated.
Depletion of Adenosine Triphosphate and Terminal Depolarization
The primary insult of cerebral ischemia is loss of ATP as a result of a reduction in oxidative phosphorylation. The degree and duration of the ischemia determine the severity of the energy impairment. In complete and prolonged ischemia, energy metabolism ceases altogether. Whether an interruption in glucose supply from blood depletes cellular ATP depends on the availability of alternative glycolytic and oxidative substrates and on the cell’s rate of consumption of ATP. More commonly, ischemia is incomplete, and when the oxygen supply is critical, greater reliance is placed on anaerobic metabolism. Recall that astrocytes possess their own glycogen stores and, by converting these stores to glucose, can, at least in theory, temporarily continue to supply a limited quantity of ATP by anaerobic glycolysis.344 Meanwhile, interruption of oxidative phosphorylation causes ATP synthase to run in reverse, and this can accelerate the depletion of residual ATP.345
In the core of a focal ischemic lesion, severe or complete reduction of ATP levels to 25% or less of basal levels initiates an early disturbance in ion gradients.329 Of foremost importance is a reduction in the activity of plasma membrane Na+,K+-ATPase. This affects neurons before astrocytes because the former have higher energy demands.346 Because Na+,K+-ATPase counters the inherent “leakiness” of the cell membrane, the membrane potential gradually rises, which leads to the opening of voltage-gated ion channels. This permits the influx of Na+, Ca2+, and Cl− and the efflux of K+ along concentration gradients previously established by primary and secondary active transport. The influx of Na+ and Cl− is accompanied by osmotically obligated water, thereby resulting in cell swelling or osmolytic damage. This cell swelling is not considered to be a principal cause of cell death in vivo because of the limited Na+, Cl−, and water content of extracellular fluid (about 20% of tissue volume). More importantly, profound dissipation of ion gradients ensues, and with insufficient Na+,K+-ATPase activity to reestablish them, neurons become terminally depolarized and electrically silent.347 This sequence of events probably underlies cell death in the ischemic core.
In the penumbra, the catastrophic events of the core do not occur because tissue energy status is nearly normal or relatively well preserved. The suppression of electroencephalographic activity along with the supply of sufficient substrates for ongoing anaerobic synthesis of ATP avoids the rapid onset of extreme ionic disturbances seen in the core. Nevertheless, cell death eventually ensues if blood flow is persistently depressed. There is experimental evidence from rodents that the occurrence of repetitive peri-infarct depolarization may ultimately lead to terminal depolarization.348 These depolarizations occur very abruptly, last between 3 and 5 minutes, have the characteristic form of spreading depression, and are associated with K+ efflux and Ca2+ influx. Their frequencies are halved by NMDA or AMPA antagonists, and hence they are believed to originate from glutamate release in the ischemic core.349 They probably aggravate the energy demands on penumbral brain because they necessitate the consumption of ATP to restore normal transmembrane gradients. Certainly, biochemical measurements confirm the episodic depletion of ATP, and factors that reduce the frequency of these depolarizations also reduce the final infarct size.348–351 Nonetheless, it remains to be established that such depolarization waves occur in primates or in humans. Instead, cell death in the penumbra has been attributed to a host of secondary phenomena triggered by acidosis, excess glutamate signaling, and cytosolic Ca2+ overloading.
Acidosis
As a consequence of increased anaerobic glycolysis, the proton and lactate levels of the brain rise during ischemia, with a corresponding reduction in intracellular and extracellular pH.346,352 The pH of ischemic brain may fall below 6.2 in normoglycemia and below 5.6 in the presence of preischemic hyperglycemia.353 Evidence has been presented that acidosis inhibits glycolysis, thus compromising the brain’s attempt to maintain ATP levels.354 Acidotic conditions during ischemia also reduce the activity of endogenous antioxidants and favor the liberation of ferrous iron from binding sites on ferritin and other protein complexes, which catalyzes hydroxyl radical (·OH−) formation from hydrogen peroxide (H2O2) (the so-called Fenton or Haber-Weiss reaction).355
Glutamate-Dependent and Glutamate-Independent Cellular Calcium Overload
The depolarization of neurons by ischemia triggers the release of glutamate and other excitatory neurotransmitters. Glutamate can activate two types of ionotropic glutamate receptors on neurons—those preferentially activated by AMPA or NMDA. Glutamate acts on the AMPA receptor to open a channel that allows the passage of monovalent cations (Na+, K+, and H+). When Na+ enters this channel down its electrochemical gradient, it depolarizes the postsynaptic membrane and allows the influx of Ca2+ through L- and T-type VGCCs on the postsynaptic membranes of the dendrites and cell body. In addition, it relieves the magnesium (Mg2+) block on postsynaptic NMDA-gated channels, thereby allowing Ca2+ to pass through these particularly high-conductance channels. Although AMPA-gated channels are generally considered to conduct monovalent cations only, it has been established that they may permit an inward Ca2+ current with certain combinations of receptor subunits (GluR1 to GluR4) and the degree of RNA editing.356,357 According to the GluR2 hypothesis, the assembly of AMPA receptors containing the GluR2 subunit, which are impermeable to Ca2+, is preferentially suppressed relative to that of other AMPA receptors after cerebral ischemia.358 Additionally, activation of metabotropic glutamate receptors results in the mobilization of intracellular endoplasmic reticulum calcium stores via activation of PLC and the production of second messengers such as IP3.
The glutamate-stimulated increase in free [Ca2+]i is normally promptly terminated by reuptake of glutamate into astrocytes by Na+-glutamate cotransporters. Recall that glutamate uptake by GLT1 and GLAST is driven by the electrochemical gradient of Na+, which is maintained by Na+,K+-ATPase (see earlier). Because of the failure of Na+,K+-ATPase, uptake of glutamate by these transporters is inhibited. It is also possible that glutamate uptake by glial GLT1 and the excitatory amino acid transporter EAAT2 can be downregulated by free radical–mediated oxidation of a redox site on these transporters.359 Furthermore, because these transporters are electrogenic (i.e., normally transferring a positive charge inward), membrane depolarization can lead to a reversal of the direction of Na+ transport and actively contribute to excess of glutamate in the ECS. Thus, impaired glutamate uptake and enhanced glutamate release combine to cause increasing concentrations of extracellular glutamate, abnormal prolongation of glutamate signaling, further cellular Ca2+ influx, and ever increasing elevations in free [Ca2+]i. Initiation of this positive feedback loop is crucial to the theory of glutamate-mediated excitotoxicity.
Researchers have also identified an important role for ischemic cation influx secondary to glutamate-independent mechanisms,360 including TRPM7, a nonselectively permeable member of the melastatin subfamily of TRP channels that is activated by oxidative stress; acid-sensitive ion channel 1a (ASIC1a), which carries an inward Na+ and Ca2+ current in response to extracellular acidification; Na+/K+/2Cl− cotransporter isoform 1 (NKCC-1); and NCX, which can work as an antiporter in either the Ca2+ efflux/Na+ influx (forward) or Na+ efflux/Ca2+ influx (reverse) modes. The first two have been found to contribute to intracellular Na+ and Ca2+ overload in cerebral ischemia.361–363 With regard to NCX, investigators have previously found evidence of both detrimental and beneficial functions in the setting of ischemia.364 NCX has three isoforms that are encoded by three different genes. Significantly, although NCX1 and NCX2 require ATP to function, NCX3 does not. Intracellular sodium overload through channels such as TRP, ASIC1a, and NKCC-1 has the potential to cause NCX to function in reverse mode, thereby exacerbating the cytosolic Ca2+ load.365 Alternatively, there is evidence that cleavage of NCX proteins by the Ca2+-activated protease calpain promotes the excitotoxic death of neurons,366,367 which suggests that the forward function of NCX may indeed be operating to reduce the cytosolic Ca2+ level. In this regard, it is of interest that ischemic brain damage is much worsened in NCX3-null mice.368
There is convincing evidence that mitochondria actively accumulate most of the Ca2+ that enters neurons during pathologic glutamate signaling in ischemic regions. In this situation, many of the mechanisms for the reduction of cytosolic Ca2+ are disabled because of ATP depletion. In fact, under extreme ischemic stress, endoplasmic reticulum Ca2+ stores are discharged into the cytoplasm, thereby aggravating the cytosolic Ca2+ overload.369,370 Hence, there is an increase in [Ca2+]i and a proportional rise in activity of the uniporter responsible for the mitochondrial Ca2+ influx. This usually serves the physiologic purpose of stimulating the activity of matrix Ca2+-sensitive dehydrogenase, thus increasing the supply of reducing equivalents to the respiratory chain and enhancing ATP synthesis. However, because the mitochondrial Ca2+ efflux mechanisms are substantially slower than the uniporter, they are readily overwhelmed by excessive Ca2+ influx through the uniporter, with significant adverse consequences on mitochondrial function (see later).334
Activation of Inflammation
A number of proinflammatory genes, including those for tumor necrosis factor-α (TNF-α) and interleukin-1β (IL-1β) and the transcription factors HIF-1, nuclear factor κB (NF-κB), and interferon-regulatory factor-1, are activated in response to the hypoxia, superoxide radical (·O2−) formation, and Ca2+ influx in the acute phase of cerebral ischemia.371,372 These products influence the expression of adhesion proteins such as intercellular adhesion molecule-1 (ICAM-1), P-selectins, and E-selectins, which permit neutrophils to accumulate in the vasculature.373 A major role in inflammation is played by resident microglia, whose proliferation and activation are a striking feature of the ischemic penumbra.374 In addition, chemokines such as monocyte chemoattractant protein-1 (MCP-1) induce the transmigration of leukocytes and monocytes into ischemic brain.375 About a third of these monocytes differentiate into cells that are indistinguishable from locally present microglia (Fig. 343-8).
Inflammation has many potentially deleterious effects in the ischemic brain. The accumulation of neutrophils, platelets, and red blood cells can be so intense that it leads to capillary plugging and results in focal areas of downstream microvascular perfusion defects (the so-called no-reflow phenomenon).376 Soluble factors released from neutrophils have been shown to activate endothelial matrix metalloproteinases (MMPs).377 Another source of MMPs is activated microglial cells.378 MMPs cleave protein components of the extracellular matrix, as well as process some cell surface receptors and soluble proteins.375 Studies suggest that MMP-2 and MMP-9 have in vivo activity in the conversion of pro-IL-1β into the mature proinflammatory cytokine IL-1β, which is known to be a crucial mediator of neurodegeneration in experimental models of cerebral ischemia.379 Both MCP-1 and MMPs have been found to cause BBB breakdown with disorganization of tight junctions as reflected by the redistribution and loss of key proteins such as occludin and zonula occludens-1.380–382 The permeability of the BBB is further exacerbated by hypoxia and neutrophil-induced activation of the contractile apparatus of the endothelial cell, which causes the formation of paracellular gaps.380,383 Destruction of the BBB permits the transmigration of leukocytes, promotes the extravasation of albumin and other osmotic compounds leading to vasogenic edema, and increases the risk of hemorrhagic conversion of an infarct.384 In addition, the elaboration of oxygen free radicals by inflammatory cells contributes to oxidative stress and vascular injury.385
Enhanced Activity of Free Radical Species
Ischemia causes an increase in the generation of both ROSs and reactive nitrogen species (RNSs) to levels that cannot be managed by endogenous antioxidant enzymes and factors. In this scenario, mitochondria are strongly implicated in the production of excessive superoxide production.386 An initial burst is triggered by the interruption of oxidative phosphorylation at complex IV in the mitochondrial respiratory chain by the lack of oxygen.345 Later mitochondrial free radical generation is under the influence of high Ca2+, Na+ and ADP in ischemic cells.387 Invading inflammatory cells also make an important contribution to ROS and RNS formation in several in vivo animal models of cerebral ischemia.388,389 Besides their direct cellular toxicity, these ROSs and RNSs can trigger the release of proinflammatory cytokines such as TNF-α, IL-1β, and IL-6, with subsequent upregulation of ICAM-1 and vascular cell adhesion molecule (VCAM), thereby leading to positive feedback.390
Ca2+ provides a major stimulus for the production of ROSs and RNSs in several ways. During the ischemic period, excessive consumption of ATP leads to accumulation of the purine metabolites xanthine and hypoxanthine in the brain, which on subsequent reperfusion are metabolized by xanthine oxidase to yield massive amounts of H2O2 and ·O2−. Ca2+ influences this process by inducing the activation of a cytosolic serine protease that converts xanthine dehydrogenase (which does not produce ROSs) to xanthine oxidase by limited proteolysis. In other cases, Ca2+ may trigger a reaction sequence that secondarily gives rise to ROS production. This occurs when Ca2+ activates PLA2, which causes hydrolysis of phospholipids and accumulation of arachidonic acid. It is during the metabolism of arachidonic acid by COX and lipoxygenase that ROSs, notably ·O2−, are formed.391,392 Elevations in [Ca2+]i can also produce several free radical species through stimulating the synthesis of NO by calcium-dependent nNOS. NO synthesis is particularly linked to Ca2+ influx via the NMDA receptor–operated channel through coupling of nNOS to the NMDA receptor by postsynaptic density 95 protein (PSD-95).393 NO itself has limited radical reactivity, and its half-life is brief because of its rapid reaction with ·O2− and possibly H2O2 to produce neurotoxic peroxynitrite (ONOO−), which can in turn decompose to another toxic species, ·OH−, the hydroxyl radical.394 This important reaction sequence is described by the following three equations:
Of all the NOS isoforms, nNOS also has a propensity for generating ·O2− through the oxidation of NADPH at the expense of O2. Such uncoupling of O2 reduction from NO formation is more likely under the acidic pH of the ischemic brain.395
It has been demonstrated in numerous studies that free radicals are directly involved in the damage to cellular macromolecules, especially lipids, proteins, and nucleic acids,396 and influence redox signal transduction pathways. With regard to the latter, free radicals can trigger the translocation of cytochrome c from the mitochondria to the cytoplasm and give rise to apoptosis.397 Through reduction of the redox state of the cell, free radicals can also rapidly reduce levels of the constitutively expressed DNA repair enzyme apurinic/apyrimidic endonuclease-1/redox factor-1 (APE/Ref-1) and activate transcription factors involved in the upregulation of proinflammatory genes.398 Experiments have implicated this reduction of APE/Ref-1 in the fragmentation of cellular DNA in focal cerebral ischemia, which may then precipitate apoptotic pathways.398 In addition, ROSs such as superoxide have the capacity to alter the vascular response to CO2, and endothelium-dependent vasodilators such as acetylcholine increase platelet aggregability, increase endothelial and BBB permeability, and disrupt endothelial membranes.385,399
DNA Damage
DNA damage in ischemia is primarily mediated by free radicals. Like other free radicals, ONOO− exerts toxic effects via a multiplicity of mechanisms.400,401 However, ONOO− is unique among the free radical species in having a long enough half-life to travel within and between cells and eventually entering the cell nucleus to trigger single-strand breaks in DNA.402 In this respect it is regarded as the key pathophysiologic species in the oxidation of and damage to DNA, with subsequent activation of poly(ADP-ribose) polymerase-1 (PARP-1), which as elaborated later, is believed to be a key mediator of cell death.403,404 In addition, intraneuronal Ca2+ overload or acidification can activate Ca2+/Mg2+-dependent endonucleases, or DNase II, and lead to internucleosomal cleavage of DNA.405
Generation of Lipid Mediators
Lipid catabolism is enhanced in ischemia, both because of insufficient ATP and cytidine triphosphate to catalyze the resynthesis of phospholipids in the course of their normal turnover and because calcium activates PLA2 and PLC.406,407 Hence, ischemia leads to a marked increase in free fatty acid levels, particularly arachidonic acid. Once reperfusion is initiated, oxidative metabolism of the accumulated arachidonic acid leads to the formation of COX and lipoxygenase products, some of which are active in promoting inflammatory responses, including the chemotactic accumulation of leukocytes in ischemic or postischemic tissue, whereas others promote cerebral edema, vasoconstriction, and platelet aggregation.408 PLA2 activation also gives rise to the formation of platelet-activating factor (PAF) from glycerophosphocholine (a minor phospholipid component of the membranes). The PLA2-activated reaction produces lyso-PAF, but this is converted to PAF by an endogenous acetyltransferase.409 PAF itself is a potent vasoconstrictor that may worsen flow reduction in the ischemic brain.410 Furthermore, PAF can promote transcriptional activation of primary response genes, including c-jun, c-fos, and the gene for the inducible form of prostaglandin synthase (PGS-2), which leads to the elaboration of a spectrum of vasoactive eicosanoids.406
Proteolysis by Calpains and Cathepsins
The elevations in [Ca2+]i resulting from ischemia can cause toxicity through the mediation of calcium-dependent proteases known as calpains. Calpains cleave α-spectrin, actin, and fodrin-α, thereby causing collapse of the cytoskeleton.411,412 In itself, this can cause serious problems for intracellular signaling, which depends on the integrity of the cytoskeleton. In addition, it has been proposed that damage to cytoskeletal proteins can contribute to subsequent damage to mitochondria. To be specific, many proteins in mitochondria, including mitochondrial RNA polymerase and most of the 13 subunits that make up cytochrome oxidase (complex IV) for the respiratory chain, are encoded only by nuclear DNA. Hence, neuronal mitochondria need to be periodically shuttled to and from the perikaryon by the cytoskeletal proteins cytoplasmic dynein and kinesin to obtain their full complement of proteins. Because this shuttle system is essential to mitochondrial function, intra-ischemic and postischemic damage to the motor proteins (dynein and kinesin) may be a cause of delayed neuronal damage, simply because mitochondria in regions far from the perikaryon run out of proteins to sustain oxidative phosphorylation.413
Calpains have also been implicated in cell death through the liberation of destructive proteases from the lysosome, the “suicide bags” of the cell.414 The lysosomal proteases cathepsin B and L have been found in the cytoplasm of ischemic cells, presumably after injury to the lysosomal membrane by free radicals or extralysosomal hydrolytic enzymes.315 With regard to the latter, involvement of calpains has been hypothesized because they have been found to rapidly localize to lysosomal membranes in primate models of ischemia.415,416 Among various targets, cathepsin B can activate the proinflammatory caspase-1 (also known as interleukin-1β–converting enzyme [ICE]) and caspase-11, which play important roles in brain ischemia by triggering inflammation and apoptosis.370,417 In addition, the proteolytic effects of cathepsin B include the activation of Bax and Bak, two proapoptotic members of the B-cell lymphoma (Bcl-2) family, and the subsequent release of proapoptotic cytochrome c through permeabilization of the mitochondrial membrane.
Secondary Energy Failure
Some evidence suggests that free radical overproduction causes ischemic death by compromising mitochondrial energy metabolism on a number of levels. The NO produced by nNOS, as well as by mtNOS, reversibly binds to the Cu2+ center of cytochrome oxidase and consequently inhibits electron transfer to O2 and mitochondrial O2 uptake.418 The free radical species ONOO−, ·OH, and ·O2− have been shown to cause direct inactivation of essential enzymes of the TCA cycle and metalloprotein complexes of the electron transport chain. For example, the Fe-S centers in electron transfer complexes are exquisitely sensitive to radical-mediated inactivation, as are pyruvate and succinate dehydrogenases, succinate oxidase, complex I, and F0F1-ATPase.419,420 ONOO− can also trigger the high conductance state of the mitochondrial permeability transition pore (MPTP), thus dissipating the mitochondrial membrane potential and resulting in cessation of electron transport and ATP synthesis.401 It is possible, too, that alterations in the fluidity of membranes subsequent to the peroxidation of their lipid constituents by free radicals could undermine electron flow by affecting lateral diffusion within the inner mitochondrial membrane.421
Yet another important contribution to secondary energy failure occurs through the activation of PARP-1 by the damaging actions of ONOO− on DNA. Evidence of PARP-1 activation is prominent in human cerebral infarcts.422 PARP-1 is an abundant nuclear enzyme whose activation after DNA damage is an early event elicited by very small amounts of DNA damage. The activated PARP-1 binds to DNA and hydrolyzes NAD+ to form polymers of ADP-ribose on acceptor proteins. Activation of PARP-1 is energetically expensive. For every mole of ADP-ribose transferred from NAD+, one mole of NAD+ is consumed and four free energy equivalents of ATP are required to regenerate NAD+ to normal cellular levels. This process can potentially result in a dramatic decline in cellular NAD+, particularly when NAD+ biosynthesis by the ATP-dependent NAD synthetase is inhibited. Once the NAD+ concentration falls below the approximately 1-mM level necessary to sustain the glycolytic glyceraldehyde-3-phosphate dehydrogenase reaction or the approximately 0.1-mM level necessary for intramitochondrial dehydrogenase reactions, the rate of ATP production is impaired, and a vicious cycle results that if not reversed will lead to permanent metabolic failure and necrotic death.423
In addition, PARP-1 can catalyze the activation of proinflammatory pathways via enhancement of NF-κB–mediated transcription, which plays a central role in the expression of inflammatory cytokines, chemokines, adhesion molecules, and inflammatory mediators.424 Hence, activation of PARP-1 can result in the migration of macrophages and microglial cells, with the potential for production of large quantities of free radicals and toxic cytokines. Alternatively, PARP-1 activation can also act as a signal for the initiation of a caspase-independent apoptotic program through the nuclear translocation of apoptosis-inducing factor (AIF).
Mechanisms of Apoptosis
Intrinsic Pathway: Mitochondrial Release of Proapoptotic Molecules
Current understanding of mitochondrial involvement in apoptosis is that mitochondria have a key role in initiation of the process through the release of a number of apoptogenic proteins from the intermembrane space. These proteins include cytochrome c, the second mitochondrial activator of apoptosis/direct inhibitor of apoptosis (IAP) binding protein with low pI (smac/DIABLO), and AIF. Experimental evidence suggests that release of these mitochondrial proteins can occur through two main mechanisms: nonspecific rupture of the outer mitochondrial membrane (OMM) after the induction of an MPTP and selective OMM permeabilization by Bcl-2 proteins (Fig. 343-9).
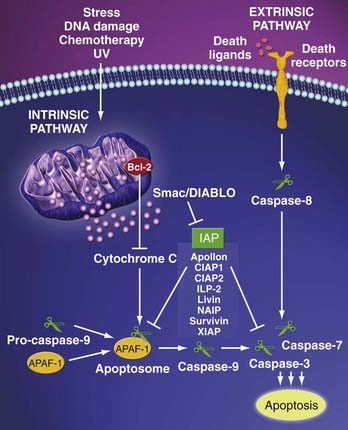
(Downloaded from http://www.imgenex.com/emarketing/081606_LivinorSurvivin/LivinorSurvivin_forweb.htm on 3/3/09.)
The MPTP is a multiprotein complex situated in the inner mitochondrial membrane; it was originally discovered as a mechanism by which mitochondrial calcium overload is relieved. The MPTP has been shown to be a key player in the cell death caused by Ca2+ overload, as well as by oxidative stress, high Pi levels, and decreased ATP, conditions that are created by ischemia.425,426 There is ongoing debate over the composition of the MPTP, but one crucial component is known to be cyclophilin D, which is so named because it is bound and inhibited by the drug cyclosporine. Cyclophilin D is thought to facilitate a calcium-triggered conformational change in the component that forms the transmembrane channel by converting it to an “open” pore.427,428 This causes indiscriminate discharge of Ca2+ and other molecules with a molecular mass of up to 1500 kD, dissipation of the mitochondrial electrochemical hydrogen ion gradient (Δψm), loss of matrix pyridine nucleotides, and rupture of the OMM, thus having an adverse impact on both the function and structural integrity of the organelle.
The MPTP was originally proposed to be the universal mechanism for mitochondrial involvement in apoptosis, but more recent studies have supported the provision by Bcl-2 molecules of a more important MPTP-independent mechanism, as well as regulatory mechanisms for the MPTP.428,429 Proteins in the Bcl-2 family share up to four Bcl-2 homology domains (BH1 to BH4) and are divided between proapoptotic and antiapoptotic members.430 It has been proposed that BH3-only proteins induce oligomerization of the multidomain proapoptotic Bax and Bak, which then insert themselves into the OMM and form large pores through which proteins may be able to leave the intermembrane space.431 Unlike the MPTP, this Bax- and Bak-mediated mitochondrial membrane permeabilization appears to be facilitated by Mg2+ and insensitive to cyclosporine.432 In contrast, Bcl-2 and Bcl-X are well-characterized inhibitors of apoptosis, and evidence suggests that they can inhibit opening of the MPTP.433,434
Release of cytochrome c from the confines of mitochondria is the commitment step in both the intrinsic and extrinsic pathways of apoptosis.324,435 In the cytosol, cytochrome c binds to deoxy-ATP and apoptotic peptidase-activating factor (APAF-1), which then oligomerizes into a septameric apoptosome. This process exposes APAF-1’s caspase activation and recruitment domain (CARD), thereby leading to the formation of a holoenzyme complex with caspase-9 and the subsequent activation of caspase-3 and other caspases such as types 2, 6, 8, and 10.436 Caspase-3 is known as an executioner of apoptotic cell death because it initiates the final common pathway in apoptosis that ultimately causes the morphologic and biochemical changes seen in apoptotic cells. Caspase-3 cleaves a range of critical cellular substrates and destroys their functions in the process, including the DNA repair enzyme PARP, the plasma membrane Ca2+-2H+ pump, the cytoskeletal components gelsolin and fodrin, and nuclear lamins.324,437,438 Caspase-3 can also activate proteins such as caspase-activated DNAse (CAD), which is responsible for the distinctive electrophoretic DNA ladder of apoptosis. Apoptosis can be blocked by binding of the X-linked inhibitor of apoptosis (XIAP) to APAF-1/caspase-9. However, XIAP is negatively regulated by smac/DIABLO, which is released from injured mitochondria together with cytochrome c.
By contrast, excitotoxic stimulation of NMDA receptors leads to the generation of free radicals and induction of apoptosis through PARP-1 activation. After PARP-1 activation, the flavoprotein AIF translocates from the mitochondria to the nucleus, preceding the release of cytochrome c and activation of caspase-3, the major executioner caspase. At the nucleus, AIF initiates nuclear condensation and large-scale chromatin fragmentation, thus dooming the cell to death. After AIF translocation, collapse of the mitochondrial membrane potential and initiation of the release of cytochrome c take place.439 However, the apoptogenic actions of AIF appear to be independent of caspase.439 Exactly how PARP-1 activation leads to the release of AIF is unclear, but the depletion of NAD+ after PARP-1 activation or some product of PARP-1 could serve as the signal.
Extrinsic Pathway: Signaling through Cell Surface Receptors
Stimulation of cell membrane death receptors belonging to the TNF receptor gene superfamily by virtue of a cytoplasmic domain of about 80 amino acids called the “death domain” plays a critical role in transmitting the death signal from the cell surface to the intracellular signaling pathways. The sequence of events that define the extrinsic phase of apoptosis is best characterized for the Fas ligand (FasL) and its receptor (FasR). Binding of FasL to FasR causes trimerization of Fas with the cytoplasmic adapter protein—Fas-associating protein with death domain (FADD)—and procaspase-8. At this point, a death-inducing signaling complex (DISC) is formed and results in the autocatalytic activation of procaspase-8. In turn, caspase-8 activates caspase-3, which triggers the execution phase of apoptosis as described earlier (see Fig. 343-9).440
When compared with the intrinsic apoptotic pathway, there is limited evidence implicating activation of the extrinsic apoptotic pathway in focal cerebral ischemia. Involvement of this pathway has been suggested by the expression of FasL and TNF-related apoptosis-inducing ligand (TRAIL) in the brain after reversible MCAO in adult rats. Furthermore, mice expressing dysfunctional FasL suffered reduced infarct volumes after MCAO.441 In another study, Fas mRNA was found to be induced after hypoxia-ischemia in the adult rat brain.442 Finally, striking Fas and FasL immunoreactivity was found in penumbra neurons in postmortem human brains.443
Amantea D, Nappi G, Bernardi G, et al. Post-ischemic brain damage: pathophysiology and role of inflammatory mediators. FEBS J. 2009;276:13-26.
Attwell D, Laughlin SB. An energy budget for signaling in the grey matter of the brain. J Cereb Blood Flow Metab. 2001;21:1133-1145.
Benarroch EE. Neuron-astrocyte interactions: partnership for normal function and disease in the central nervous system. Mayo Clin Proc. 2005;80:1326-1338.
Bian K, Murad F. Nitric oxide (NO)—biogeneration, regulation, and relevance to human diseases. Front Biosci. 2003;8:d264-278.
Bredesen DE, Rao RV, Mehlen P. Cell death in the nervous system. Nature. 2006;443:796-802.
Brown AM, Ransom BR. Astrocyte glycogen and brain energy metabolism. Glia. 2007;55:1263-1271.
Chipuk JE, Green DR. How do Bcl-2 proteins induce mitochondrial outer membrane permeabilization? Trends Cell Biol. 2008;18:157-164.
Friedman JA, Anderson RE, Meyer FB. Techniques of intraoperative cerebral blood flow measurement. Neurosurg Focus. 2000;9(5):e4.
Gordon GR, Choi HB, Rungta RL, et al. Brain metabolism dictates the polarity of astrocyte control over arterioles. Nature. 2008;456:745-749.
Hoeffner EG, Case I, Jain R, et al. Cerebral perfusion CT: technique and clinical applications. Radiology. 2004;231:632-644.
Jones TH, Morawetz RB, Crowell RM, et al. Thresholds of focal cerebral ischemia in awake monkeys. J Neurosurg. 1981;54:773-782.
Kety SS, Schmidt CF. The determination of cerebral blood flow in man by the use of nitrous oxide in low concentrations. Am J Physiol. 1945;143:53-66.
Kristian T, Siesjo BK. Calcium in ischemic cell death. Stroke. 1998;29:705-718.
Latchaw RE, Yonas H, Hunter GJ, et al. Guidelines and recommendations for perfusion imaging in cerebral ischemia: a scientific statement for healthcare professionals by the Writing Group on Perfusion Imaging, from the Council on Cardiovascular Radiology of the American Heart Association. Stroke. 2003;34:1084-1104.
Leist M, Jaattela M. Four deaths and a funeral: from caspases to alternative mechanisms. Nat Rev Mol Cell Biol. 2001;2:589-598.
Lipowsky HH. Microvascular rheology and hemodynamics. Microcirculation. 2005;12:5-15.
Magistretti PJ. Neuron-glia metabolic coupling and plasticity. J Exp Biol. 2006;209:2304-2311.
McKenna MC, Gruetter R, Sonnewald U, et al. Energy metabolism of the brain. In: Siegel GJ, Albers RW, Brady S, editors. Basic Neurochemistry: Molecular, Cellular, and Medical Aspects. Boston: Academic Press; 2006:531-558.
Nichols WW, McDonald DA, O’Rourke MF. McDonald’s Blood Flow in Arteries: Theoretical, Experimental, and Clinical Principles. London: Hodder Arnold; Distributed in the United States of America by Oxford University Press; 2005.
Pacher P, Beckman JS, Liaudet L. Nitric oxide and peroxynitrite in health and disease. Physiol Rev. 2007;87:315-424.
Siesjo BK. Pathophysiology and treatment of focal cerebral ischemia. Part I: Pathophysiology. J Neurosurg. 1992;77:169-184.
Symon L. The ischemic penumbra—the beginning. In: Donnan GA, Baron JC, Davis SM, editors. The Ischemic Penumbra. New York: Informa Healthcare; 2007:21-36.
Weinstein PR, Hong S, Sharp FR. Molecular identification of the ischemic penumbra. Stroke. 2004;35:2666-2670.
Zonta M, Angulo MC, Gobbo S, et al. Neuron-to-astrocyte signaling is central to the dynamic control of brain microcirculation. Nat Neurosci. 2003;6:43-50.